- 1Laboratory of Ecology, Department of Earth and Marine Sciences (DiSTeM), University of Palermo, Palermo, Italy
- 2Department of Biological and Environmental Sciences and Technologies (DiSTeBA), University of Salento, Lecce, Italy
- 3National Interuniversity Consortium for Marine Sciences (CoNISMa), Rome, Italy
Temperature is a fundamental variable for all biological processes. It influences the metabolism and tolerance limits of all living organisms, affecting species phenology and distribution patterns. It also facilitates the spread of non-indigenous species and the proliferation and expansion of native outbreak-forming species. Pennaria disticha is a colonial benthic cnidarian reported to be invasive in different Indian and Pacific coastal areas, as well as a harmful member of fouling communities found in Mediterranean marine aquaculture farms. Using the most basal functional trait (i.e., thermal tolerance), we explored the potential of P. disticha to colonize different habitats across the Mediterranean Sea in future warming scenarios. Respiration rate was measured as a proxy of P. disticha metabolism under 12 different experimental temperatures. The obtained thermal tolerance dataset was used to create a thermal performance curve (TPC). We then scaled modeled curve to occurrence probability to map species potential metabolic habitat suitability and phenological shifts within the Mediterranean Sea when subjected to different warming scenarios. Prediction maps for future climatic conditions showed a potential temporal and spatial expansion of P. disticha in the Western and Central Mediterranean. The present data increases our understanding of the ecological performance and potential distribution of an invasive and outbreak-forming species. This information will contribute to the development of early warning systems and to the design and implementation of risk assessment and management plans.
Introduction
The combination of climate change and local anthropogenic stressors can facilitate the spread and recruitment of non-indigenous species (Sarà et al., 2018; Geraldi et al., 2020) and the proliferation and expansion of native outbreak-forming species, such as jellyfish (Purcell et al., 2007), exacerbating their overall ecological impacts and economic consequences worldwide. Indeed, as documented by ongoing species range shifts and changes in phenology (Parmesan, 2006; Elith and Leathwick, 2009; Hoegh-Guldberg and Bruno, 2010; Sunday et al., 2015), climate change and ocean warming are expected to increasingly affect the structure and function of marine ecosystems, whose species need to adapt or to shift their geographical or vertical distribution to preserve their climatic niche (Coll et al., 2012; Garcia Molinos et al., 2016). For instance, global warming—combined with other anthropogenic impacts (Purcell et al., 2007; Boero et al., 2008; Richardson et al., 2009)—may amplify the spread of several jellyfish species by increasing their reproduction rate, reproductive period and/or their range of distribution toward higher latitudes (Purcell, 2005; Lucas et al., 2014a,b; Milisenda et al., 2018). Temperature, however, remains a key factor influencing the metabolism and phenology of cnidarians, despite the paradigmatic potential for life cycle adjustments and physiological plasticity bestowed upon this group (Boero et al., 2016; Foo and Byrne, 2016).
The increasing likelihood of negative interactions between marine gelatinous organisms and humans has also been reported in recent years (Purcell et al., 2007; Brotz and Pauly, 2012; Lucas et al., 2014b; Bosch-Belmar et al., 2020), driven by the coinciding rise of human activities at sea and cnidarians proliferative potential (Gibbons and Richardson, 2013). Marine aquaculture sprawl is known to facilitate the spread of jellyfish and other cnidarians as it provides additional suitable habitats such as artificial benthic substrates (Sarà et al., 2007) for the asexual proliferation of sessile life stages, i.e., the polyps (Boero et al., 2008; Richardson et al., 2009; Duarte et al., 2013). Specifically, hydroids (i.e., the sessile polyp colonies of the class Hydrozoa) represent a significant component within marine fouling communities on natural and artificial substrates. Common underwater net and submerge structures cleaning activities in aquaculture facilities, as well as seasonal hydroids reproductive events, may release thousands of free-living stinging propagules (such as tiny jellyfish, larvae, or fragmented tentacles) into the water column that may directly and repeatedly contact with fish skin and gills (Baxter et al., 2011; Bosch-Belmar et al., 2017). The toxic compounds delivered by their stinging cells threaten the health of farmed organisms, negatively affecting the effectiveness and productivity of aquaculture operations and related revenues (Baxter et al., 2012; Fitridge and Keough, 2013; Sievers et al., 2013; Bosch-Belmar et al., 2020).
Among the most common and injuring components of the fouling community, the conspicuous colonial hydroid Pennaria disticha is not only able to affect farmed organisms but also to produce painful stings and severe dermatitis to field aquaculture operators and bathers (Tezcan and Sarp, 2013; Bosch-Belmar et al., 2017). It may pose a significant threat since some of its main traits (e.g., warm-water affinity, high growth rate, iteroparous reproduction, and the persistence of a resting stage when environmental conditions are not optimal) conferred the species a significant invasiveness potential (Schuchert, 2006; Knapp et al., 2011; Bosch-Belmar et al., 2019; Vaga et al., 2020).
Despite its potential impact on marine ecosystems and relevant economic activities, there is a lack of knowledge on the ecological performance of harmful species (such as stinging cnidarians, like P. disticha) and the potential influence of future temperature conditions on their metabolism, phenology, and distribution. Ecologists and biologists may count on functional traits to assist stakeholders in predicting the potential distribution of harmful species driven by environmental (including climatic) change. These traits usually include important aspects such as tolerance and sensitivity to environmental conditions, driving the ability of each species to adapt its own metabolic machinery and biological performance in space and time (Sarà et al., 2013). Here, we used the “simplest” and the most basal functional trait, thermal tolerance, to explore if and how P. disticha is expected to colonize different habitats across the Mediterranean Sea in different future warming scenarios. Individual respiration rate was used as a metabolic proxy (Angilletta et al., 2002; Sokolova et al., 2012; Gould et al., 2021) to study the effects of temperature on the organismal performance of P. disticha and link the species potential habitat suitability based on this functional trait with the IPCC temperature projections under different climatic scenarios (Butenschön et al., 2016). We generated metabolic-based maps that can be considered as an informative baseline and used to implement adaptive measures for management and conservation of biodiversity and natural resources. They provide a useful predictive tool for stakeholders and entrepreneurs of the Blue Economy, who are faced with increased ecosystem vulnerability due to constantly changing environmental conditions.
Materials and Methods
Laboratory Experiments
A total of 90 colony specimens of Pennaria disticha was sampled along the coast of Palermo (Sicily, Central Mediterranean Sea) (38°11′12.786″N; 13°21′41.1336″E). Hydroid colonies were collected by gently removing the creeping stolons (hydrorhiza) from the substrate to preserve the integrity of the erect colonial stem (hydrocaulus) and its lateral branches (hydrocladia). Samples were immediately brought back to the wet enclosure of the Laboratory of Ecology at the University of Palermo and prepared for the experiments. Hydrocauli ranging between 8 and 12 cm in length were carefully inspected with a stereomicroscope, and all epibiotic organisms were manually removed using forceps. Prior to the experiments the hydroids were starved and acclimated for 24 h at the temperature recorded during sampling (23°C).
To measure oxygen consumption at each investigated temperature, nine different colonies were randomly collected from the tanks and placed individually in a respirometric chamber (130 ml) containing filtered (Whatman GF/C 0.45 μm) saturated-air, 38 psu seawater. Three respirometric chambers were filled with filtered seawater only and they were used as controls. To ensure the constant mixing of water, each chamber was stirred with a bar magnet and an individual stirring device (Bosch-Belmar et al., 2016). Respirometric chambers (12 in total: 3 controls and 9 containing P. disticha specimens) were randomly divided into three temperature-controlled water baths and the concentration of dissolved oxygen was measured simultaneously by means of 3 optical oxygen meters (Pyro Science Firesting O2), each equipped with four optodes. Measurements were performed in continuum for 1 h. Temperatures were kept stable by means of a circulated thermal bath (Grant Optima TX150). To investigate the thermal tolerance of P. disticha, respiration rate (RR, mg O2 h–1 DW-g–1) was measured at 11 different temperatures as follows: 13, 15, 17, 19, 21, 23, 25, 27, 29, 31, and 33°C. Temperature range was based on the areal extremes of the Pennaria disticha spatial distribution. In other words, minimum and maximum temperatures were obtained from the species’ occurrence reported in the GBIF online database1 for the Mediterranean Sea. Starting from the water acclimation temperature, the temperature in each group was increased or decreased to the next level at a rate of 1°C per hour (e.g., Schröer et al., 2011; Prusina et al., 2014; Montalto et al., 2017). Respiration rate was calculated according to Sarà et al. (2013):
where Ct0 was the oxygen concentration at the beginning of the measurement, Ct1 was the oxygen concentration at the end of the measurement and Volr was the volume of water in the respirometric chamber. At the end of the measurement session, the organisms from each replicate were dried at 105°C for 24 h and then weighed to determine the Dry Weight (DW, g).
Thermal Performance Model
By using the “rTPC” R package (Padfield et al., 2021), a total of 23 thermal performance models were performed and compared, allowing us to identify the Sharpe-Schoolfield model as the best fit with our respiration rate (RR) data (i.e., presenting the lowest AICc score; see Supplementary Table 1 and Supplementary Figure 1). The Sharpe-Schoolfield is a non-linear regression model describing the thermal performance curves (TPC) of an organism based on enzymatic deactivation at high temperatures (Schoolfield et al., 1981). This model estimated four distinct parameters used in the prediction of the monthly presence of the species based on its RR values (Supplementary Figure 2). The model equation is:
where “k” is the Boltzmann’s constant with a value of 8.62e-05; “rtref” is the rate at the standardised temperature; “e” is the activation energy (eV); “eh” is the high temperature de-activation energy (eV); “th” is the temperature (°C) at which enzyme is 1/2 active and 1/2 suppressed due to high temperatures; “rtref” is the standardization temperature in degrees Celsius (temperature at which rates are not inactivated by high temperatures); “temp” was the temperature in degrees Celsius.
Bootstrapping was used to calculate 95% prediction limits for the selected TPC model and confidence intervals around its temperature optimum.
Environmental Data
Sea surface temperature (SST) was selected to perform the model since P. disticha is a species usually habiting shallow waters where light and temperatures drive its reproduction (Schuchert, 2006). Mediterranean monthly mean SST raster files were created based on current daily SST data downloaded from the Copernicus Marine Service Information.2 To predict future distribution, we used forecasted daily SST for the year 2050 (Assis et al., 2018) within modeling projections forced by Representative Concentration Pathways RCP4.5 and 8.5 (IPCC, 2014), which represented a likely range of future global greenhouse gas and aerosol emissions. RCP4.5 scenario represents moderate emissions while RCP8.5 forecasts more extreme effects of climate change. All SST raster layers were upscaled to a resolution of 0.08 × 0.08 decimal degrees.
Metabolic Suitability Prediction Maps
The final parameters of the TPC model (Supplementary Figure 2) were used to predict the potential occurrence of the active colonial stage of Pennaria disticha in the present-day climatic conditions and in two 2050 future scenarios of greenhouse gas (GHG) emissions (RCP4.5 and RCP8.5). In the Mediterranean and other temperate seas, P. disticha has an iteroparous, multiannual life cycle, with a sharply seasonal phenology (Brinckmann-Voss, 1970); the active colonial stage (i.e., the erect polyp colony) arise and sexually reproduce in the warmest months of the year. After reproduction, the polyps shrink, shedding all feeding and reproductive hydranths, to rest throughout the coldest period in a quiescent form, i.e., the creeping stage (the hydrorhiza). This remains anchored to the substrate until suitable environmental conditions return that trigger the re-growth of the erect polyp colony (Gili and Hughes, 1995). This is the life stage that represents a major multiannual source of impact on Mediterranean marine fish farms. At the same time, the hydroid colony plays a role as a seasonal habitat former in shallow rocky bottom communities.
To create thermal habitat suitability maps based on the species metabolism, data on experimentally measured respiration rate was converted into probability of occurrence at different temperatures. We scaled the different oxygen consumption values to the RR value at the temperature optimum predicted by the model, using the following formula:
where OP was the occurrence probability, RRt was the respiration rate value at temperature t, and RROpt was the value of respiration rate at the optimum temperature predicted by the Sharpe-Schoolfield model.
Spatial occurrence probability based on RR values was calculated at pixel level using the environmental values reported in the (a) current, (b) 2050 RCP 4.5, and (c) 2050 RCP 8.5 raster layers using the following formula:
where SOPim was the spatial occurrence probability in the spatial pixel “i” for the month “m,” PRRim was the predicted respirartion rate in the spatial pixel “i” and the month “m,” and RROpt was the value of respiration rate at the optimum temperature predicted by the model.
To signal the temporal and spatial difference between the present and the future predictions for the species habitat suitability, subtractions between future and present raster files were carried out for each month and RCP scenario. In this way, new raster layers were created, where increasing or decreasing spatial occurrence probability of the species at pixel level was represented.
Results
Thermal Performance Curve
Across the temperature range tested (from 13 to 33°C), the amount of consumed oxygen changed from 1.22 ± 0.68 mg h–1 g–1 DW (estimated at 15°C) to 5.70 ± 1.30 mg h–1 g–1 DW (estimated at 23°C). At the warmest temperatures, a sharp increase in the hydroid respiration rate was measured from 27°C (1.54 ± 0.30 mg h–1 g–1 DW) to 29°C (6.46 ± 0.95 mg h–1 g–1 DW) and maintained up to 31 and 33°C (5.02 ± 1.07 and 6.49 ± 5.15 mg h–1 g–1 DW, respectively). Particularly, at 27 and 29°C, a regression of hydranths (polyp tentacles contraction) was observed, and at 31 and 33°C autotomy of hydrants was repeatedly recorded in all experimental individuals. This response may be related to the transition from the optimal to the pejus temperature range (Sokolova et al., 2012), or even to a direct switch to the pessimum range, as has been observed for some marine organisms at high temperatures (Jost et al., 2012). Consequently, data from 29, 31, and 33°C were considered uninformative for the TPC model and associated with an increasing likelihood of reproductive failure and/or ongoing mortality of the colonies. Respiration rate data were then fitted using the Sharpe-Schoolfield model, and the obtained TPC predicts a temperature optimum for the species at 24.1°C (Figure 1). Furthermore, the fitted model identifies a maximum (27.6°C) thermal limit for the species, in accordance with the temperature value corresponding to the morphological changes observed during the experiments (Figure 2). The model estimated four distinct parameters used in the prediction of the monthly presence of the species based on its RR values (Supplementary Figure 2).
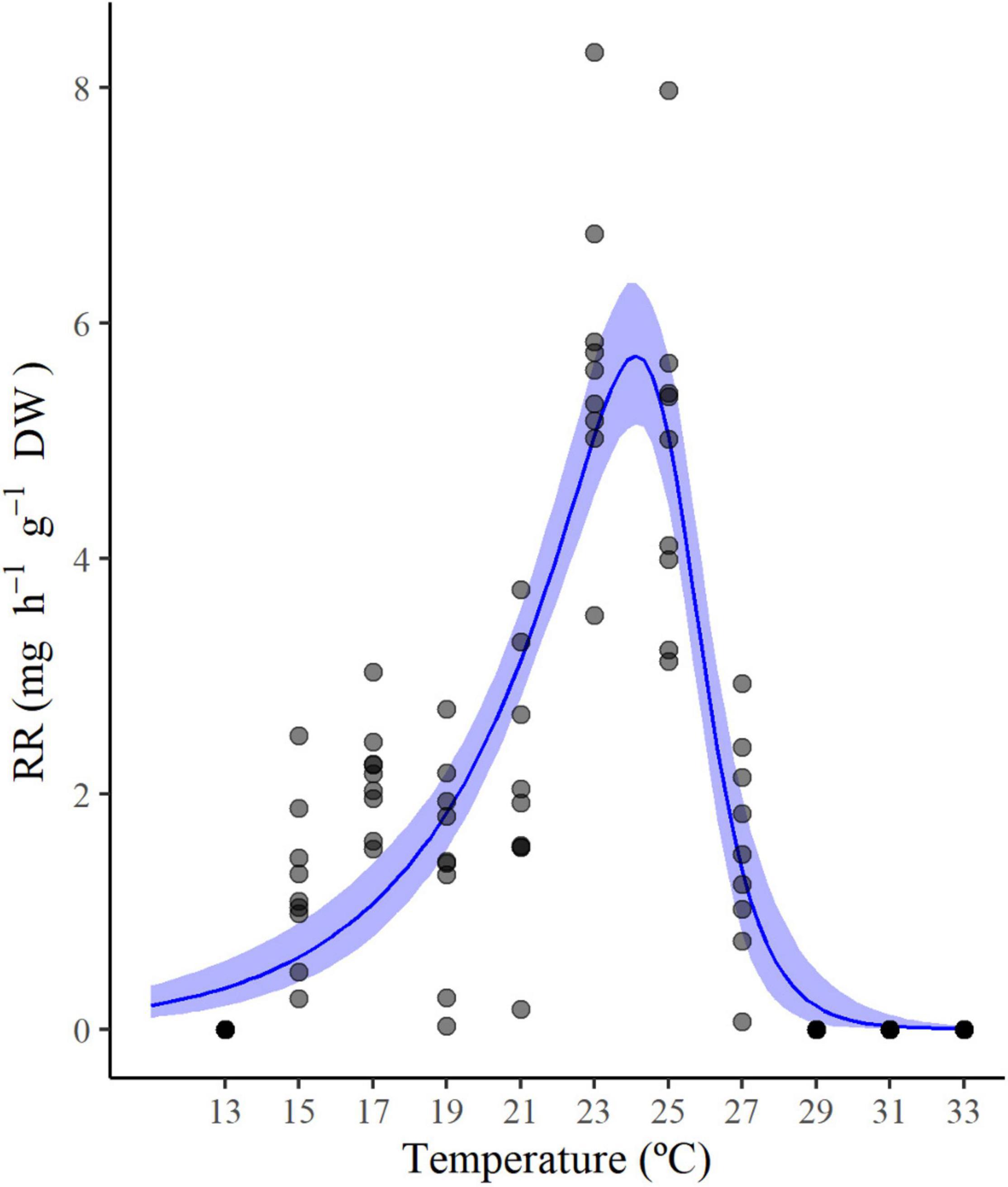
Figure 1. Thermal performance curve of Pennaria disticha according to the selected model (Sharpe-Schoolfield). Shaded area corresponds to the confidence intervals. Darker dots represent overlapped individual respiration rate values.
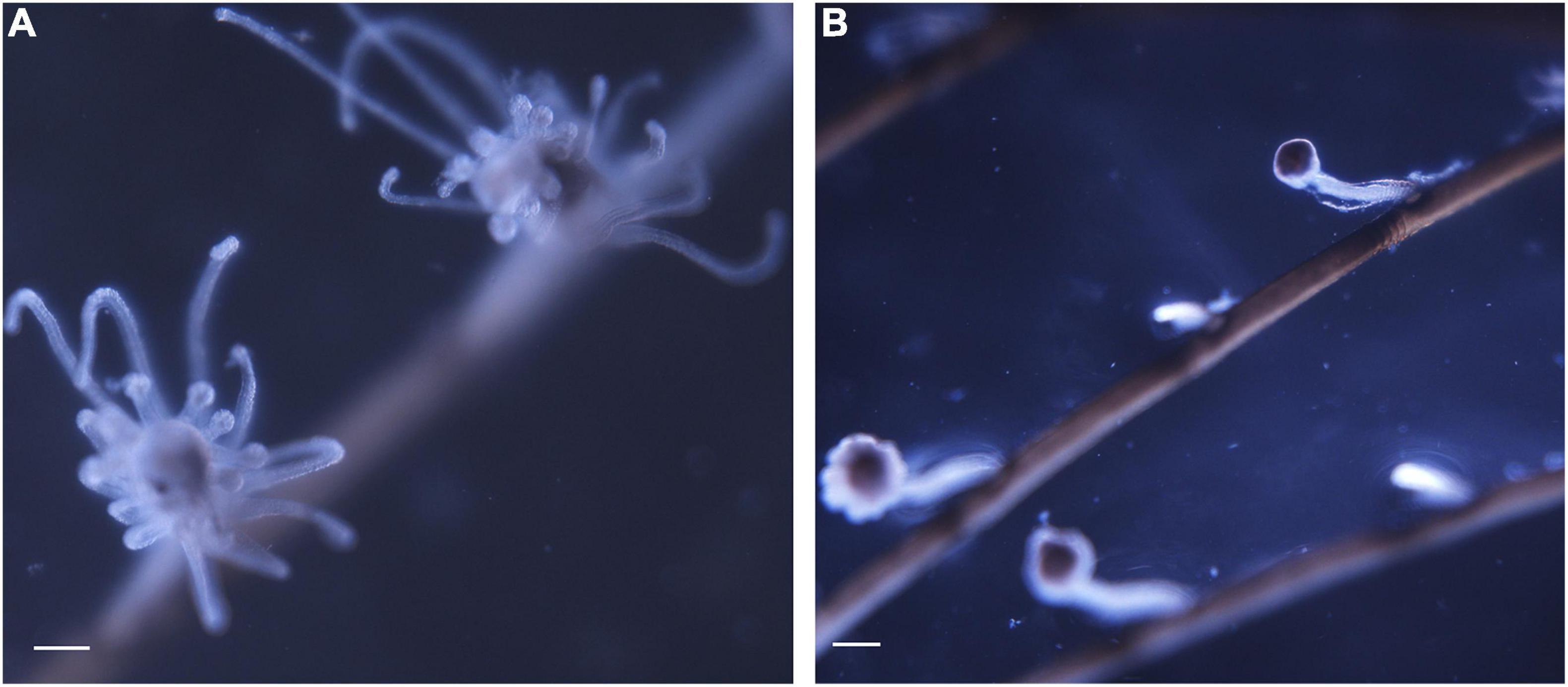
Figure 2. Morphological features of experimental colonies at different tested temperatures: (A) healthy and performant polyp at 23°C and (B) damage hydrants at 29°C. Scale bar = 500 μm.
Metabolic Suitability Prediction Maps
To create prediction maps, measured respiration rates were converted into probability of occurrence. This allows to graphically identify the different ranges of habitat suitability probability on the TPC (Figure 3). Thus, low probability values (from 0 to 0.2) would correspond to low RR placed in the final part of the modeled curve tails, representing the low performance of the species at those temperatures (ideally closer to the lower and higher lethal limits). Based on metabolic rates (i.e., the optimum thermal conditions for the species), our modeling effort suggested that the whole Mediterranean basin may have suitable conditions for the growth and reproduction of Pennaria disticha hydroid colonies. Prediction metabolic maps showed that the potential seasonal occurrence of active polyp colonies of P. disticha in the Mediterranean Sea may vary among different basin sections. In the Adriatic Sea and in the Western and central sub-regions, the highest habitat suitability (>0.6) for the hydroid is restricted between June to October, while in the Eastern basin it may span from May to December (Figure 4). Prediction maps illustrating the distributions under the RCP 4.5 and 8.5 (2050) scenarios showed a potential temporal and spatial expansion of P. disticha in the Central and Western Mediterranean. The habitat suitability of the species is increased by 2 months, potentially from May to November in the western and central parts of the basin (Figures 5, 6). Specifically, the species occurrence would increase in May (up to 0.6) in southern Tunisia and the Greek coasts, and slightly (up to 0.4) in the northern Spanish and Sardinian areas. Also, a potential marked enlargement of the metabolic-based habitat suitability for P. disticha is predicted in November for the whole basin, increasing latitudinally from 0.6 to 0.8 and 1 in the southern Mediterranean coasts to 0.6 and 0.8 in the Spanish coasts, the North Tyrrhenian and the Adriatic seas. No remarkable expansion can be detected between present-day and future predictions of spatial distribution and/or monthly occurrence of P. disticha in the Eastern Basin. Even if future climatic conditions may facilitate the spread of P. disticha and may increase its occurrence probability within the Mediterranean, a decrease in the potential thermal suitability of the species is predicted in the warmer months (July, August, and September), under both RCP 4.5 and 8.5 across the Adriatic and the Aegean Seas, as well as in the eastern Tunisian coast (Figures 7, 8).
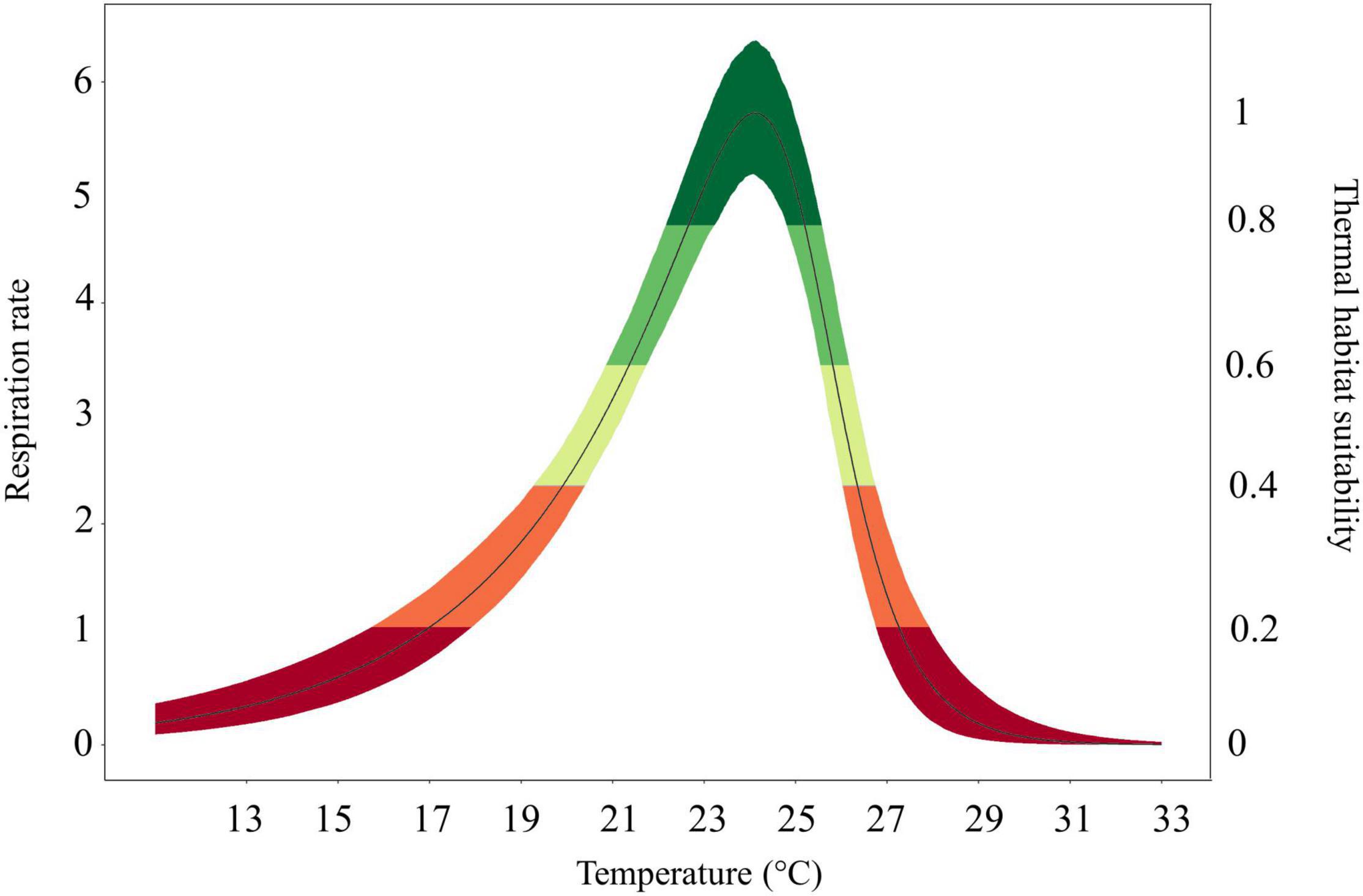
Figure 3. Thermal habitat suitability scale within the thermal performance curve of the species ranging from low (0) to high (1) species occurrence probability. Different colors (representing the species thermal habitat suitability) would correspond to: optimum temperature range (dark green colored), lower and upper pejus ranges (light green and yellow colored), pessimum (orange colored), and lethal ranges (red colored) according to Sokolova et al. (2012).
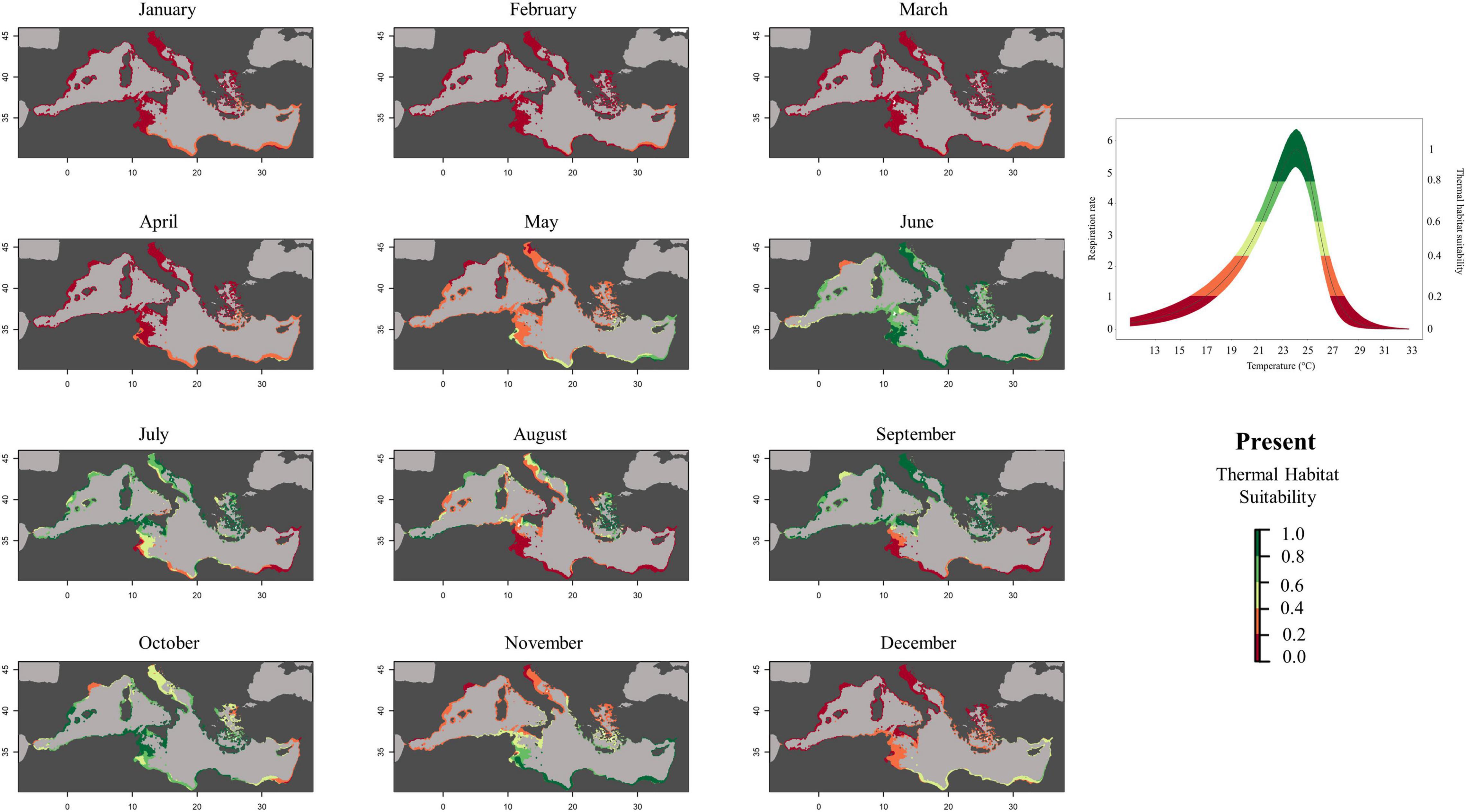
Figure 4. Current predicted habitat suitability probability of Pennaria disticha based on respiration rate values (mg h– 1 g– 1 DW).
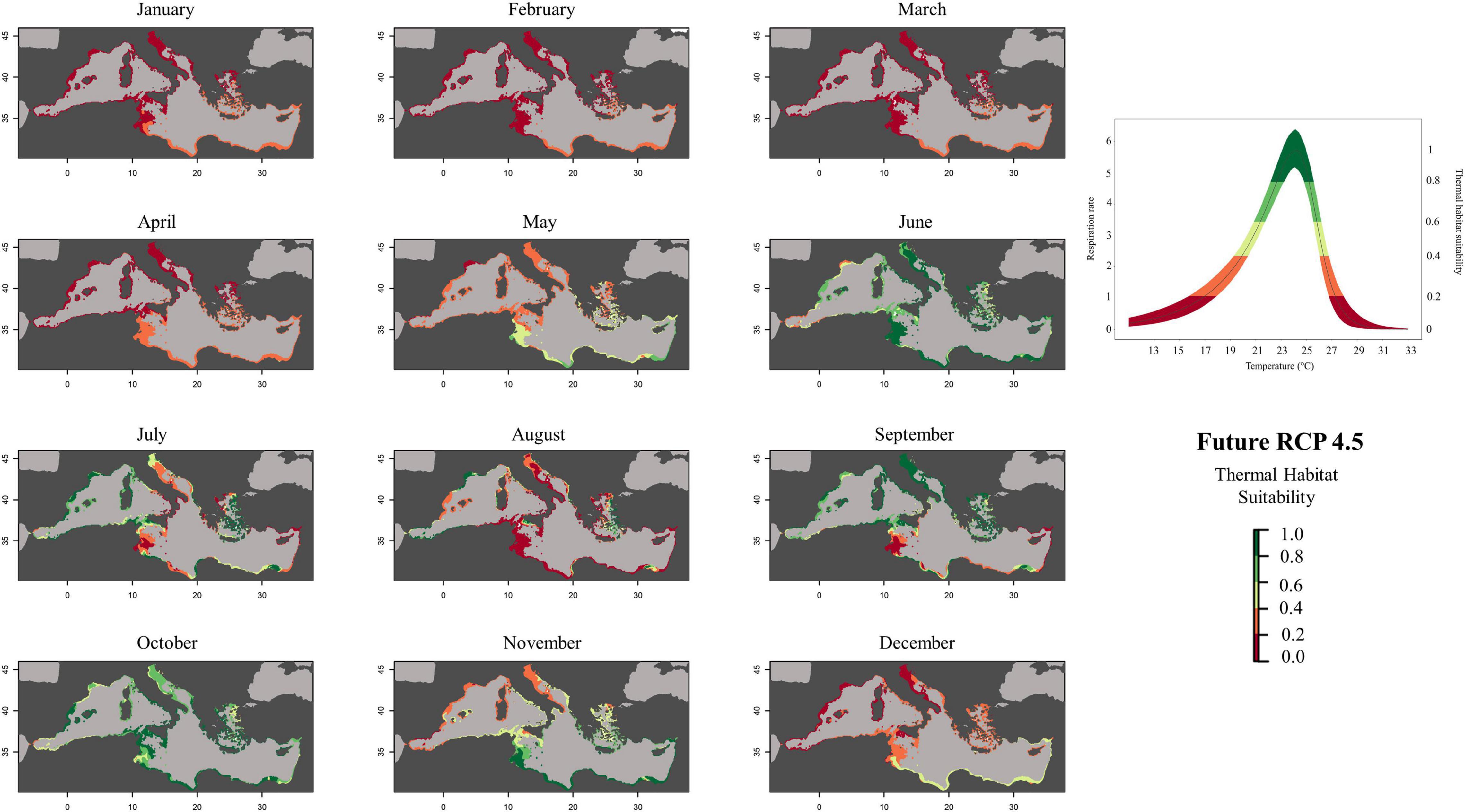
Figure 5. Future (RCP 4.5, 2050) predicted habitat suitability probability of Pennaria disticha based on respiration rate values (mg h– 1 g– 1 DW).
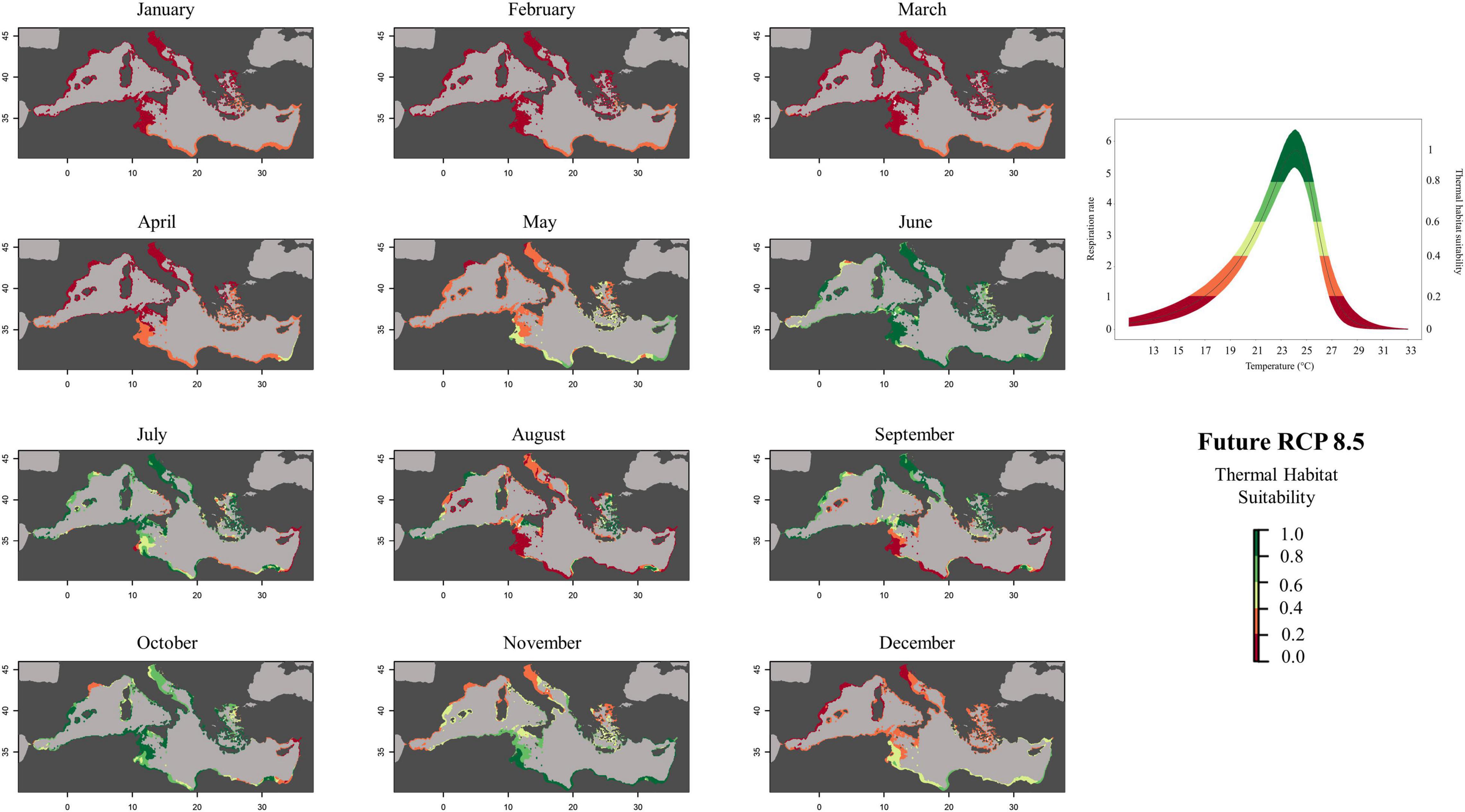
Figure 6. Future (RCP 8.5, 2050) predicted habitat suitability probability of Pennaria disticha based on respiration rate values (mg h– 1 g– 1 DW).
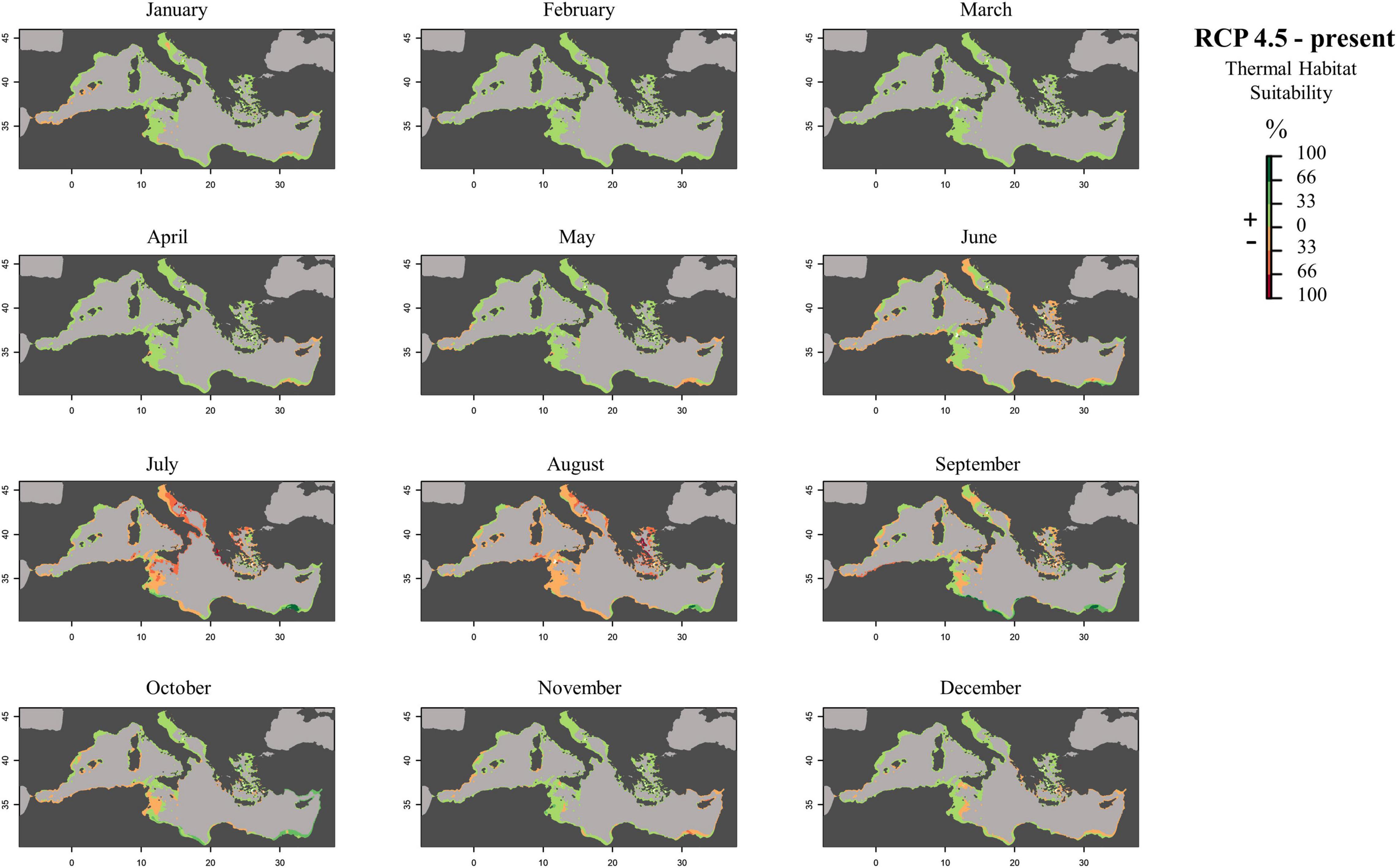
Figure 7. Monthly prediction maps representing the subtraction between future (RCP 4.5) and present habitat suitability raster files: positive percentage values represent an increase in the suitability of the habitat in the future scenario, while negative percentage values represent a decrease in the occurrence probability of the species in that area.
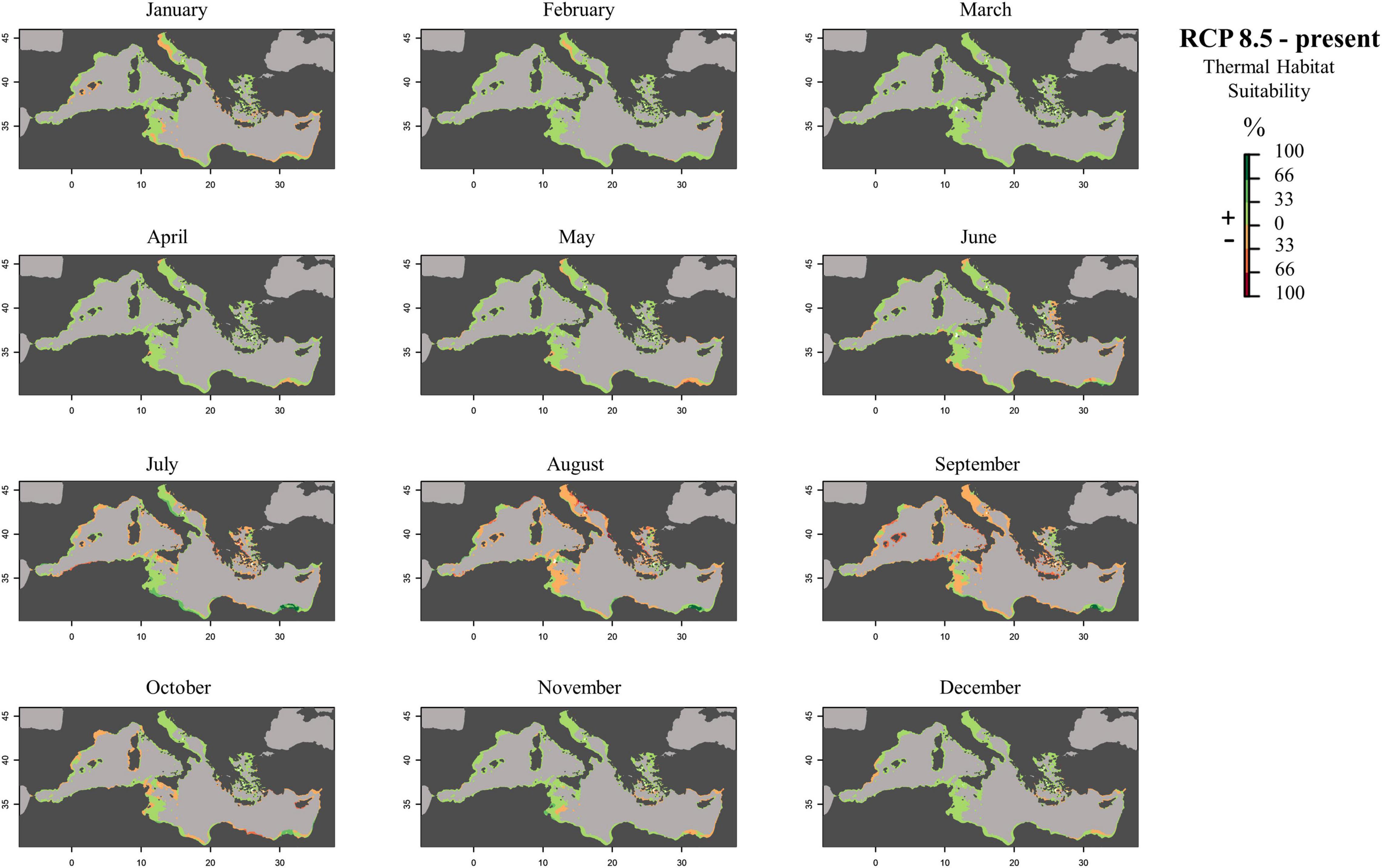
Figure 8. Monthly prediction maps representing the subtraction between future (RCP 8.5) and present raster files: positive percentage values represent an increase in the suitability of the habitat in the future scenario, while negative percentage values represent a decrease in the occurrence probability of the species in that area.
Discussion
Temperature is the main factor regulating biological processes across all levels of ecological organization (Brown et al., 2004; Kooijman, 2010; Yvon-Durocher et al., 2012). Even small increases of SST may significantly influence individual metabolic rates and population growth. In turn, metabolic shifts may lead to changes in species ontogeny, phenology, geographical distribution, and biotic interactions (Levy et al., 2016). Species distribution, coexistence, and degree of resilience to stressful or changing environmental conditions, critically depend on species-specific reproductive traits and outputs (Angilletta, 2009). Seasonal hydroids, such as P. disticha, enter their active phenotype (the polyp colony) when conditions are optimal for energy capture (feeding) and for the initiation of sexual reproduction. Pennaria colonies also exhibit a prolonged resting dormancy period preceded by regression of hydranths, loss of the erect stem and branches, and the accumulation of stem cells in creeping stolons; making this thermophilic species resistant to prolonged periods (months) of adverse conditions, i.e., able to overwinter at low water temperatures (Gili and Hughes, 1995). Temperature, as the main regulator of Pennaria’s complex life cycle, is the trigger for the activation and termination of each of those stages.
Pennaria disticha was originally described in the Mediterranean Sea (Goldfuss, 1820) but it is now commonly recorded worldwide in temperate and tropical waters, encountered on rocky coasts at shallow depths (Brinckmann-Voss, 1970; Schuchert, 2006). Coinciding with the rise of water temperature in the Ligurian Sea over the last 40 years, P. disticha has been increasingly recorded in the Northern Tyrrhenian Sea (Bianchi and Morri, 1994; Puce et al., 2009; Parravicini et al., 2015). The species has also been recorded in the Southern and Central Adriatic, and recently, for the first time, within the fouling community of mussel farms near Cattolica (Emilia Romagna region) in the southern part of the Northern Adriatic (Di Camillo et al., 2018). The current prediction maps (Figure 4) faithfully described the annual period of the colonial stage occurrence reported by scientific literature (Schuchert, 2006; Bosch-Belmar et al., 2019), and showed a large thermal suitability area for the species within the entire Mediterranean Sea, even in areas where the species has only been rarely recorded or not yet recorded (i.e., the upper part of the northern Adriatic Sea). The forecasted rise in water temperature will boost the ecological success of Pennaria disticha in the Mediterranean basin, through enlargement of its geographical distribution and phenological shifts. According to the metabolic predictions, the species should shift its active stage (the polyp colony) into a prolonged period (reflected by the increasing occurrence probability), from spring to late autumn.
Our integrated experimental and modeling effort showed that P. disticha should reach “maximum performance” at 24.1°C, with a left skew curve characterized by lower performance at lower temperatures. This trait is usually representative of a “high-temperature” specialist (Angilletta, 2009). This means that above the species-specific optimum value of T, any further increase may lead to energy reallocation, rapid reduction in growth rate, reproductive failure, and even mortality (Matzelle et al., 2015). Accordingly, our laboratory experiments highlighted a typical sequence of temperature-dependent morphological changes. At T = 28–29°C, the polyps displayed tentacle contraction. At T ≥ 30°C, autotomy of polyps (i.e., detachment of the upper part of the polyp including mouth and tentacles) was commonly observed in all experimental colonies. Autotomized polyps were unable to regenerate the colonial stem and eventually died. This suggests increased likelihood—in scenarios with high GHG level—of reproductive failure and mortality, with direct negative consequences at population level. This detrimental morphological change, recorded under mesocosm conditions, is mirrored by the metabolic-based model maps, which predicted a future “unlikely” thermal suitability for the species during the warmest months in the Southern and Eastern Mediterranean areas (Figures 5, 6), where mean monthly temperatures are expected to exceed the maximum thermal tolerance limits of the species. Thus, in warmer months, P. disticha colonies would be subjected to “thermal stressful status” with significant effects on reproduction and species dispersal. Similar to other hydroids (e.g., Ectopleura spp.) that may respond to increasing temperature through autotomy and dispersal of polyps bearing germ cells (Boero, 1984), P. disticha might also cope with and acclimate to warming seas by bringing forward the development and maturation of the free-living, planktonic sexual stage (short-living eumedusoids). Nevertheless, further research is needed to investigate the potential of P. disticha to adapt to the cumulation of multiple human-driven impacts.
The present results indicate, that in the future, we can expect an increasing detrimental role of P. disticha in Mediterranean waters given the predicted changing conditions in thermal profiles. As this species is a structural, deciduous habitat-former able to modify the microhabitats where it attaches affecting substrate morphology and water circulation, the potential effect on local biodiversity of natural rocky and artificial hard substrates such as those of aquaculture facilities will be amplified. The species’ invasive potential, facilitated by rising temperature, will increasingly negatively impact the fish aquaculture sector. As a result, aquaculture management plans will need to be implemented with increased awareness of all potential threats, including the seasonal occurrence of invasive and outbreak-forming species. Temperature thresholds in performance of P. disticha can be associated with the vulnerable temperature range for Mediterranean fish marine aquaculture and used to inform fish farm operators of the periods when the active (and potentially harmful) colonial stage may be present, and of the temperature range when production of the free-living, medusoid sexual stages can be foreseen (around 25°C, Bosch-Belmar et al., 2019). Increased information on problematic species biology and potential distribution would contribute to the development of early warning systems, informed risk assessments, and more effective management plans. These actions are considered essential for conservation policymakers and marine economic sector managers who are faced with dynamic environmental change and increased ecosystem vulnerability.
Data Availability Statement
The original contributions presented in the study are included in the article/Supplementary Material, further inquiries can be directed to the corresponding author/s.
Author Contributions
MB-B and GS conceived the study and designed it through the different steps. MB-B performed the experiments and developed the metabolic suitability prediction maps. MB-B drafted the early version of this manuscript and with GS and SP prepared the present version. All authors contributed to the article and approved the submitted version.
Funding
This study was funded by the Italian University and Research Minister (MIUR) through the PRIN MAHRES (cod. 2017MHHWBN) project.
Conflict of Interest
The authors declare that the research was conducted in the absence of any commercial or financial relationships that could be construed as a potential conflict of interest.
Publisher’s Note
All claims expressed in this article are solely those of the authors and do not necessarily represent those of their affiliated organizations, or those of the publisher, the editors and the reviewers. Any product that may be evaluated in this article, or claim that may be made by its manufacturer, is not guaranteed or endorsed by the publisher.
Acknowledgments
We are grateful to G. Milisenda for valuable statistics and modeling advice and C. Giommi and M. Tantillo for their kind assistance during the laboratory experiments.
Supplementary Material
The Supplementary Material for this article can be found online at: https://www.frontiersin.org/articles/10.3389/fmars.2022.810555/full#supplementary-material
Footnotes
References
Angilletta, M. (2009). Thermal Adaptation: A Theoretical and Empirical Synthesis, 1st Edn. New York, NY: Oxford University Press Inc. doi: 10.1093/acprof:oso/9780198570875.001.1
Angilletta, M. J., Niewiarowski, P. H., and Navas, C. A. (2002). The evolution of thermal physiology in ectotherms. J. Therm. Biol. 27, 249–268. doi: 10.2741/e148
Assis, J., Tyberghein, L., Bosch, S., Verbruggen, H., Serrão, E. A., and De Clerck, O. (2018). Bio-ORACLE v2.0: extending marine data layers for bioclimatic modelling. Glob. Ecol. Biogeogr. 27, 277–284. doi: 10.1111/geb.12693
Baxter, E. J., Rodger, H. D., McAllen, R., and Doyle, T. K. (2011). Gill disorders in marine-farmed salmon: investigating the role of hydrozoan jellyfish. Aquac. Environ. Interact. 1, 245–257. doi: 10.3354/aei00024
Baxter, E. J., Sturt, M. M., Ruane, N. M., Doyle, K., Mcallen, R., and Rodger, H. D. (2012). Biofouling of the hydroid Ectopleura larynx on aquaculture nets in Ireland: implications for finfish health. Fish Vet. J. 13, 17–29.
Bianchi, C. N., and Morri, C. (1994). Southern species in the Ligurian Sea (Northern Mediterranean): new records and a review. Boll. Mus. Ist. Biol. Univ. Genova 58–59, 181–197.
Boero, F. (1984). The ecology of marine hydroids and effects of environmental factors: a review. Mar. Ecol. 5, 93–118. doi: 10.1111/j.1439-0485.1984.tb00310.x
Boero, F., Bouillon, J., Gravili, C., Miglietta, M. P., Parsons, T., and Piraino, S. (2008). Gelatinous plankton: irregularities rule the world (sometimes). Mar. Ecol. Prog. Ser. 356, 299–310.
Boero, F., Brotz, L., Gibbons, M., Piraino, S., and Zampardi, S. (2016). “Ocean warming 3.10 impacts and effects of ocean warming on jellyfish,” in Explaining Ocean Warming: Causes, Scale, Effects and Consequences, eds D. Laffoley and J. M. Baxter (Gland: IUCN), 460.
Bosch-Belmar, M., Azzurro, E., Pulis, K., Milisenda, G., Fuentes, V., Kéfi-Daly Yahia, O., et al. (2017). Jellyfish blooms perception in Mediterranean finfish aquaculture. Mar. Policy 76, 1–7. doi: 10.1016/j.marpol.2016.11.005
Bosch-Belmar, M., Escurriola, A., Milisenda, G., Fuentes, V. L., and Piraino, S. (2019). Harmful fouling communities on fish farms in the SW mediterranean sea: composition, growth and reproductive periods. J. Mar. Sci. Eng. 7:288. doi: 10.3390/jmse7090288
Bosch-Belmar, M., Giomi, F., Rinaldi, A., Mandich, A., Fuentes, V., Mirto, S., et al. (2016). Concurrent environmental stressors and jellyfish stings impair caged European sea bass (Dicentrarchus labrax) physiological performances. Sci. Rep. 6:27929. doi: 10.1038/srep27929
Bosch-Belmar, M., Milisenda, G., Basso, L., Doyle, T. K., Leone, A., and Piraino, S. (2020). Jellyfish impacts on marine aquaculture and fisheries. Rev. Fish. Sci. Aquac. 29, 242–259. doi: 10.1080/23308249.2020.1806201
Brinckmann-Voss, A. (1970). Anthomedusae/athecata (Hydrozoa, Cnidaria) of the Mediterranean. Part I. Capitata. Fauna Flora Golfo Napoli 39, 1–96.
Brotz, L., and Pauly, D. (2012). Jellyfish populations in the Mediterranean Sea. Acta Adriat. 53, 211–230.
Brown, J. H., Gillooly, J. F., Allen, A. P., Savage, V. M., and West, G. B. (2004). Toward a metabolic theory of ecology. Ecology 85, 1771–1789. doi: 10.1890/03-9000
Butenschön, M., Clark, J., Aldridge, J. N., Icarus Allen, J., Artioli, Y., Blackford, J., et al. (2016). ERSEM 15.06: a generic model for marine biogeochemistry and the ecosystem dynamics of the lower trophic levels. Geosci. Model Dev. 9, 1293–1339. doi: 10.5194/gmd-9-1293-2016
Coll, M., Piroddi, C., Albouy, C., Ben Rais Lasram, F., Cheung, W. W. L., Christensen, V., et al. (2012). The Mediterranean Sea under siege: spatial overlap between marine biodiversity, cumulative threats and marine reserves. Glob. Ecol. Biogeogr. 21, 465–480. doi: 10.1111/j.1466-8238.2011.00697.x
Di Camillo, C. G., Pica, D., Puce, S., and Cerrano, C. (2018). Fouling Hydroids on Mussel Farm: a Possible Model in the Study of Temporal Niche Dynamics in Marine Environment. Cesenatico: Società Italiana Di Biologia Marina.
Duarte, C. M., Pitt, K. A., Lucas, C. H., Purcell, J. E., Uye, S. I., Robinson, K., et al. (2013). Is global ocean sprawl a cause of jellyfish blooms? Front. Ecol. Environ. 11, 91–97. doi: 10.1890/110246
Elith, J., and Leathwick, J. R. (2009). Species distribution models: ecological explanation and prediction across space and time. Annu. Rev. Ecol. Evol. Syst. 40, 677–697. doi: 10.1146/annurev.ecolsys.110308.120159
Fitridge, I., and Keough, M. J. (2013). Ruinous resident: the hydroid Ectopleura crocea negatively affects suspended culture of the mussel Mytilus galloprovincialis. Biofouling 29, 119–131. doi: 10.1080/08927014.2012.752465
Foo, S. A., and Byrne, M. (2016). Acclimatization and adaptive capacity of marine species in a changing ocean. Adv. Mar. Biol. 74, 69–116. doi: 10.1016/bs.amb.2016.06.001
Garcia Molinos, J., Halpern, B. S., Schoeman, D. S., Brown, C. J., Kiessling, W., Moore, P. J., et al. (2016). Climate velocity and the future global redistribution of marine biodiversity. Nat. Clim. Change 6, 83–88. doi: 10.1038/nclimate2769
Geraldi, N. R., Anton, A., Santana-Garcon, J., Bennett, S., Marbà, N., Lovelock, C. E., et al. (2020). Ecological effects of non-native species in marine ecosystems relate to co-occurring anthropogenic pressures. Glob. Change Biol. 26, 1248–1258. doi: 10.1111/gcb.14930
Gibbons, M. J., and Richardson, A. J. (2013). Beyond the jellyfish joyride and global oscillations: advancing jellyfish research. J. Plankton Res. 35, 929–938. doi: 10.1093/plankt/fbt063
Gili, J. M., and Hughes, R. G. (1995). The ecology of marine benthic hydroids. Oceanogr. Mar. Biol. Annu. Rev. 33, 351–426.
Gould, K., Bruno, J. F., Ju, R., and Goodbody-Gringley, G. (2021). Upper-mesophotic and shallow reef corals exhibit similar thermal tolerance, sensitivity and optima. Coral Reefs 40, 907–920. doi: 10.1007/s00338-021-02095-w
Hoegh-Guldberg, O., and Bruno, J. F. (2010). The impact of climate change on the world’s marine ecosystems. Science 328, 1523–1528. doi: 10.1126/science.1189930
IPCC (2014). Climate Change 2014: Synthesis Report. Contribution of Working Groups I, II and III to the Fifth Assessment Report of the Intergovernmental Panel on Climate Change. Core Writing Team. Geneva: IPCC, 151.
Jost, J. A., Podolski, S. M., and Frederich, M. (2012). Enhancing thermal tolerance by eliminating the pejus range: a comparative study with three decapod crustaceans. Mar. Ecol. Prog. Ser. 444, 263–274. doi: 10.3354/meps09379
Knapp, I. S., Godwin, L. S., Smith, J. E., Williams, C. J., and Bell, J. J. (2011). Records of non-indigenous marine species at Palmyra Atoll in the US Line Islands. Mar. Biodivers. Rec. 4:e30. doi: 10.1017/S1755267211000078
Kooijman, B. (2010). Dynamic Energy Budget Theory for Metabolic Organisation, 3rd Edn. Cambridge: Cambridge University Press.
Levy, O., Buckley, L. B., Keitt, T. H., and Angilletta, M. J. (2016). Ontogeny constrains phenology: opportunities for activity and reproduction interact to dictate potential phenologies in a changing climate. Ecol. Lett. 19, 620–628. doi: 10.1111/ele.12595
Lucas, C. H., Gelcich, S., and Uye, S.-I. (2014a). “Living with jellyfish: management and adaptation strategies,” in Jellyfish Blooms, eds K. A. Pitt and C. Lucas (Dordrecht: Springer Science+Business Media), 129–150. doi: 10.1007/978-94-007-7015-7
Lucas, C. H., Jones, D. O. B., Hollyhead, C. J., Condon, R. H., Duarte, C. M., Graham, W. M., et al. (2014b). Gelatinous zooplankton biomass in the global oceans: geographic variation and environmental drivers. Glob. Ecol. Biogeogr. 23, 701–714. doi: 10.1111/geb.12169
Matzelle, A. J., Sarà, G., Montalto, V., Zippay, M., Trussell, G. C., and Helmuth, B. (2015). A bioenergetics framework for integrating the effects of multiple stressors: opening a “black box” in climate change research. Am. Malacol. Bull. 33, 150–160. doi: 10.4003/006.033.0107
Milisenda, G., Martinez-Quintana, A., Fuentes, V. L., Bosch-Belmar, M., Aglieri, G., Boero, F., et al. (2018). Reproductive and bloom patterns of Pelagia noctiluca in the Strait of Messina, Italy. Estuar. Coast. Shelf Sci. 201, 29–39. doi: 10.1016/j.ecss.2016.01.002
Montalto, V., Bagarella, R., Rinaldi, A., Sarà, G., and Mirto, S. (2017). Thermal adaptation and physiological responses to environmental stress in tunicates. Aquat. Biol. 26, 179–184. doi: 10.3354/ab00685
Padfield, D., O’Sullivan, H., and Pawar, S. (2021). rTPC and nls.multstart: A new pipeline to fit thermal performance curves in r. Methods. Ecol. Evol. 12, 1138–1143. doi: 10.1111/2041-210X.13585
Parmesan, C. (2006). Ecological and evolutionary responses to recent climate change. Annu. Rev. Ecol. Evol. Syst. 37, 637–669. doi: 10.1146/annurev.ecolsys.37.091305.110100
Parravicini, V., Mangialajo, L., Mousseau, L., Peirano, A., Morri, C., Montefalcone, M., et al. (2015). Climate change and warm-water species at the north-western boundary of the Mediterranean Sea. Mar. Ecol. 36, 897–909. doi: 10.1111/maec.12277
Prusina, I., Sarà, G., De Pirro, M., Dong, Y. W., Han, G. D., Glamuzina, B., et al. (2014). Variations in physiological responses to thermal stress in congeneric limpets in the Mediterranean Sea. J. Exp. Mar. Biol. Ecol. 456, 34–40. doi: 10.1016/j.jembe.2014.03.011
Puce, S., Bavestrello, G., Di Camillo, C. G., and Boero, F. (2009). Long-term changes in hydroid (Cnidaria, Hydrozoa) assemblages: effect of Mediterranean warming? Mar. Ecol. 30, 313–326. doi: 10.1111/j.1439-0485.2009.00283.x
Purcell, J. E. (2005). Climate effects on formation of jellyfish and ctenophore blooms: a review. J. Mar. Biol. Assoc. U. K. 85, 461–476.
Purcell, J. E., Uye, S., and Lo, W.-T. (2007). Anthropogenic causes of jellyfish blooms and their direct consequences for humans: a review. Mar. Ecol. Prog. Ser. 350, 153–174.
Richardson, A. J., Bakun, A., Hays, G. C., and Gibbons, M. J. (2009). The jellyfish joyride: causes, consequences and management responses to a more gelatinous future. Trends Ecol. Evol. 24, 312–322. doi: 10.1016/j.tree.2009.01.010
Sarà, G., Lo Martire, M., Buffa, G., Mannino, A. M., and Badalamenti, F. (2007). The fouling community as an indicator of fish farming impact in Mediterranean. Aquac. Res. 38, 66–75. doi: 10.1111/j.1365-2109.2006.01632.x
Sarà, G., Palmeri, V., Montalto, V., Rinaldi, A., and Widdows, J. (2013). Parameterisation of bivalve functional traits for mechanistic eco-physiological dynamic energy budget (DEB) models. Mar. Ecol. Prog. Ser. 480, 99–117. doi: 10.3354/meps10195
Sarà, G., Porporato, E. M. D., Mangano, M. C., and Mieszkowska, N. (2018). Multiple stressors facilitate the spread of a non-indigenous bivalve in the Mediterranean Sea. J. Biogeogr. 45, 1090–1103. doi: 10.1111/jbi.13184
Schoolfield, R. M., Sharpe, P. J., and Magnuson, C. E. (1981). Non-linear regression of biological temperature-dependent rate models based on absolute reaction-rate theory. J. Theor. Biol. 88, 719–731. doi: 10.1016/0022-5193(81)90246-0
Schröer, M., Saphörster, J., Bock, C., and Pörtner, H. O. (2011). Oxygen and capacity limited thermal tolerance of the lugworm Arenicola marina: a seasonal comparison. J. Exp. Mar. Biol. Ecol. 409, 300–309. doi: 10.1016/j.jembe.2011.09.011
Schuchert, P. (2006). The European athecate hydroids and their medusae (Hydrozoa, Cnidaria): capitata part 1. Rev. Suisse Zool. 113, 325–410.
Sievers, M., Fitridge, I., Dempster, T., and Keough, M. J. (2013). Biofouling leads to reduced shell growth and flesh weight in the cultured mussel Mytilus galloprovincialis. Biofouling 29, 97–107. doi: 10.1080/08927014.2012.749869
Sokolova, I. M., Frederich, M., Bagwe, R., Lannig, G., and Sukhotin, A. A. (2012). Energy homeostasis as an integrative tool for assessing limits of environmental stress tolerance in aquatic invertebrates. Mar. Environ. Res. 79, 1–15. doi: 10.1016/j.marenvres.2012.04.003
Sunday, J. M., Pecl, G. T., Frusher, S., Hobday, A. J., Hill, N., Holbrook, N. J., et al. (2015). Species traits and climate velocity explain geographic range shifts in an ocean-warming hotspot. Ecol. Lett. 18, 944–953. doi: 10.1111/ele.12474
Tezcan, Ö. D., and Sarp, S. (2013). An unusual marine envenomation following a rope contact: a report on nine cases of dermatitis caused by Pennaria disticha. Toxicon 61, 125–128. doi: 10.1016/j.toxicon.2012.10.019
Vaga, C. F., Kitahara, M. V., Nascimento, K. B., and Migotto, A. E. (2020). Genetic diversity of the Pennaria disticha Goldfuss, 1820 (Cnidaria, Hydrozoa) complex: new insights from Brazil. Mar. Biodivers. 50:68. doi: 10.1007/s12526-020-01102-7
Keywords: harmful species, thermal tolerance, metabolic-based maps, early warning system, thermal habitat suitability
Citation: Bosch-Belmar M, Piraino S and Sarà G (2022) Predictive Metabolic Suitability Maps for the Thermophilic Invasive Hydroid Pennaria disticha Under Future Warming Mediterranean Sea Scenarios. Front. Mar. Sci. 9:810555. doi: 10.3389/fmars.2022.810555
Received: 07 November 2021; Accepted: 24 February 2022;
Published: 04 April 2022.
Edited by:
Stelios Katsanevakis, University of the Aegean, GreeceReviewed by:
Simone Mirto, National Research Council (CNR), ItalyConor McManus, Division of Marine Fisheries, Rhode Island Department of Environmental Management, United States
Copyright © 2022 Bosch-Belmar, Piraino and Sarà. This is an open-access article distributed under the terms of the Creative Commons Attribution License (CC BY). The use, distribution or reproduction in other forums is permitted, provided the original author(s) and the copyright owner(s) are credited and that the original publication in this journal is cited, in accordance with accepted academic practice. No use, distribution or reproduction is permitted which does not comply with these terms.
*Correspondence: Mar Bosch-Belmar, bWFyaWFkZWxtYXIuYm9zY2hiZWxtYXJAdW5pcGEuaXQ=