- 1Department of Geosciences, University of Malta, Msida, Malta
- 2Department of Earth and Environmental Sciences, University of Milano-Bicocca, Milan, Italy
- 3National Oceanography Centre, Southampton, United Kingdom
- 4Helmholtz Centre for Ocean Research, GEOMAR, Kiel, Germany
The mesophotic domain is a poorly explored part of the oceans, notably in the Mediterranean Sea. Benthic communities in these depths are not well documented and as such are under higher risk from anthropogenic impacts. Hard substrate habitats in this depth window are not common and are a key ecotope. The Malta Plateau in the central Mediterranean, which is characterized by low sedimentation rates, offers a potentially unexplored domain for these features. Bathymetric and backscatter data offshore of the eastern coast of the island of Malta were used to identify > 1,700 small structures in mesophotic depths. These structures were verified to be biogenic mounds by dives. The mounds extend from several meters to tens of meters in diameter and occur in two main depth windows −40 to 83 meters below present sea level (mbpsl) and 83–120 mbpsl—each formed probably in a different stage during the last glacial cycle. The mounds are composed of interlocking bioconstruction by encrusting organisms and are colonized by sponges and various cold water corals (most of which are protected; e.g., Madrepora oculata). This unique and important habitat is currently under grave threat by human activity, most immediately by trawling and anchoring activity.
Introduction
Biogenic Mounds
The term “mound” is commonly used in literature to indicate biogenic-mediated features forming submerged positive reliefs (from a few to hundreds of meters in height), composed of a variety of dominant organisms capable of trapping or baffling fine sediments (e.g., cold-water corals, oysters, bryozoans, polychaetes). Mounds occur in a variably composed sedimentary matrix, and display rounded, semicircular, ellipsoidal or elongated planforms (Riding, 2002; Henriet et al., 2014). The terminology used to describe mound-like biogenic submarine landforms in cold water is inconsistent across literature. Terms like “patch reefs,” “pinnacle reefs,” “reef mounds,” “carbonate mounds” and others are frequently used to refer to “mounded” bioconstructions developed in temperate seas (Greene et al., 1999; Wood, 2001; Riding, 2002; Bare et al., 2010). Comparative analysis of these features, is critical but difficult. The different origin, composition and structure of mounds is often missing. In order to avoid adding confusion to the terminologies already in use, and to document the environmental relevance that biogenic landforms seem to hold in mesophotic depths (here defined as 30–150 mbpsl, meters below the present sea level), we use the general terms “biogenic mound” to describe the observations or “mounded bioconstruction” when referring to the growth process.
Biogenic mounds have been detected all over the world, although a precise description is often overlooked. Indeed, the study of such bioconstructions has benefitted from the increased availability of seafloor acoustic maps and Remotely Operated Vehicle (ROV) imagery. In the literature, cold-water coral carbonate mounds are the most well-documented structures. Hovland et al. (1994) were some of the first to describe sub-photic carbonate build-ups, referred to as “seabed mounds,” offshore western Ireland. Since then, several studies have revealed thousands of deep-water carbonate mounds. Biogenic mounds have developed in a wide depth range, from coastal areas to deeper environments (> 1,000 m), and they can be formed by a variety of organisms. The dimensions and shapes of mounds may vary a lot, with heights ranging from a few meters to > 300 m, and lateral extensions of up to tens of kilometers (Ramos et al., 2017; Wienberg et al., 2018; Hebbeln et al., 2019). The bioconstructions are normally grouped into provinces, which may comprise tens to hundreds of individual mounds (Hebbeln et al., 2014; Glogowski et al., 2015). These morphologies have been observed and documented along the Atlantic margins (White and Dorschel, 2010; Hebbeln et al., 2019) from northern Norway to Mauritania and from Brazil to Canada, and the Mediterranean Sea (Lo Iacono et al., 2008; Wienberg and Titschack, 2015; Corbera et al., 2019; Taviani et al., 2005a, 2019; Wienberg, 2019).
There is no consensus in the literature regarding the timing and mechanism of mounded bioconstruction formation. Mounds have been related to hydrocarbon seepage (Wheeler et al., 2007; Foubert and Henriet, 2009) or autogenic processes stimulated by high current speeds and food supply (Mienis et al., 2007; White, 2007; Foubert and Henriet, 2009). Some authors (Abbey et al., 2013) ascribed the formation of mounds to reef development during short-lived periods of slow sea-level rise during the Holocene transgression, whereas subsequent drowning halted their growth. Other authors (Locker et al., 2016) ascribe their origin to reef development during periods of low sea-level amplitude during the last glacial cycles, which were terminated due to a combination of emersion and drowning during the glacial maximum and subsequent transgression. Both models are based on the water depth of the features and their correspondence to past sea-levels (Miller et al., 2020).
Direct dating of such mounds is difficult. Their outer crust, which divers and ROVs can sample, may be entirely coated by encrusters that colonized after the formation of the core of the mounds. Meanwhile, drilling the biogenic mounds is technologically challenging and expensive, and destructive methods may not be suitable for such an environmentally sensitive environment that is characterized by slow growth rate (Benedetti et al., 2016; Groves et al., 2018). Furthermore, the features themselves might be, in part, the product of erosion, complicating dating even further. As such, all age estimations for these mounds are limited and at times speculative.
Biogenic Mounds in the Mediterranean Sea
Subdivision of modes of carbonate production (carbonate factories) shows the Mediterranean carbonate production in euphotic to mesophotic depths to be unique because it is dominated by a light-governed C-type factory (Reijmer, 2021). The production in the light-governed C-type factory (Schlager, 2005; Reijmer, 2021) is defined as dominated by phototrophs that are not corals. This type of production is characterized by a small production center. Mounds reported in the Mediterranean Basin tend, for the most part, to be significantly smaller than their Atlantic counterparts (Matos et al., 2017). Mediterranean biogenic mounds are typified by cold-water coral growth at their tops and/or flanks. Mounds have been reported in the Alboran Sea, Tyrrhenian Sea, the Ionian Sea and the Levantine Sea, with varied construction including microbial, cold water corals and others (Aloisi et al., 2000; Remia and Taviani, 2005; Taviani et al., 2005b; Savini et al., 2009, 2014; Mastrototaro et al., 2010; Corbera et al., 2019; Angeletti et al., 2020). Most of these mounds occur at depths of > 200 m. Increased exploration of the mesophotic domain has led to discovery of biogenic mounds in such depths as well, revealing a diverse range of bioconstructions (Ingrosso et al., 2018). Among the most representative habitats is the coralligenous, with a wide range of morphotypes (Ballesteros et al., 2006; Bracchi et al., 2017), which are generally not associated with mound-like features. Biogenic mounds < 100 m in diameter and located in sub-euphotic depths have only been described in a few locations in the Mediterranean Sea (e.g., Savini et al., 2014; Violante et al., 2020). Notably these studies do not report coralligenous or algal reefs components. The assemblage building the mounds vary depending on depth, temperature and trophic state. It may be dominated by bryozoans (James et al., 2004), cold water corals such as Desmophyllum pertusum (= Lophelia pertusa) and Madrepora oculata (Dorschel et al., 2007; Foubert et al., 2008), sponges (Carvalho et al., 2016; Goren et al., 2021) or a combination of corals and coralline algae (Amado-Filho et al., 2016; Dumalagan et al., 2019). In some cases, based on geochemical and morphological evidence, it was possible to hypothesize the origin of some of the deeper of these mounds to hydrocarbon seepage (Cangemi et al., 2010; Loher et al., 2018). The ecology of these seeps is mostly susceptible to the activity state of the seep than other environmental parameters (Dando, 2010). However, the origin of the biogenic mounds is less clear in shallower waters where phototrophic calcifiers are present at the surface of the mound (Violante et al., 2020). In addition, the Mediterranean also hosts cold-water coral reefs (Taviani et al., 2005b; Schembri et al., 2007; Fink et al., 2015) and microbial carbonate chemoherms (Omoregie et al., 2009; Taviani et al., 2013; Rubin-Blum et al., 2014), which are outside the scope of this work.
This Study
Here, we report on the extensive occurrence of biogenic mounds offshore the Maltese Islands (Strait of Sicily). The objectives of our study are to quantify the geometry and distribution of these biogenic mounds. Based on this information, we discuss the possible origin of these features, as well as the risks they presently face.
Regional Setting
The Maltese archipelago (Figure 1) is located in the central Mediterranean Sea, between Sicily and North Africa, in an elevated region called the Malta Plateau. The latter is a 150 km long and 120 km wide ridge located in the north-eastern part of the Pelagian Platform, which separates the eastern and western Mediterranean. The Maltese Islands comprise one of the few emergent areas of the Pelagian carbonate platform. Outcropping across the Maltese Islands are shallow (< 500 m) water, Oligo-Miocene pre- to syn-rift sedimentary successions (Pedley et al., 1976). The entire sequence is disrupted by two normal fault systems (Illies, 1980; Gardiner et al., 1995). The first is a system of syn-depositional ENE-WSW trending faults, which were active intermittently between the Early Miocene and mid-Pliocene. The second system consists of NW-SE trending faults that were formed or re-activated in the late Pliocene to Quaternary. The seafloor around the archipelago, is characterized by a smooth and gently sloping seabed, predominantly 100–150 m in depth, although it locally reaches depths of 400 m. The seafloor is mostly covered by a thin layer of sand to silt sized biogenic sediments with local baffling by seagrass or red algae (Micallef et al., 2013). Seepage of methane and carbon dioxide has been documented across the Malta Plateau (Holland et al., 2003; Savini et al., 2009; Cangemi et al., 2010) also in proximity of the Maltese Islands (Micallef et al., 2011, 2019). Buried bodies of freshened groundwater have been reported offshore eastern Malta; the source of this groundwater is likely topographically-driven meteoric recharge during sea-level lowstands (Haroon et al., 2021). The water column is stratified in the boreal summer and remixes in the boreal winter (Drago et al., 2003). Winter temperature in the upper 200 m is around 15°C while the upper 100 m can warm to ∼25°C in summer. The warmer water comes from the south-east and is driven by anticyclonic flow forced by northwestern cool water flowing into the eastern Mediterranean (Drago et al., 2003).
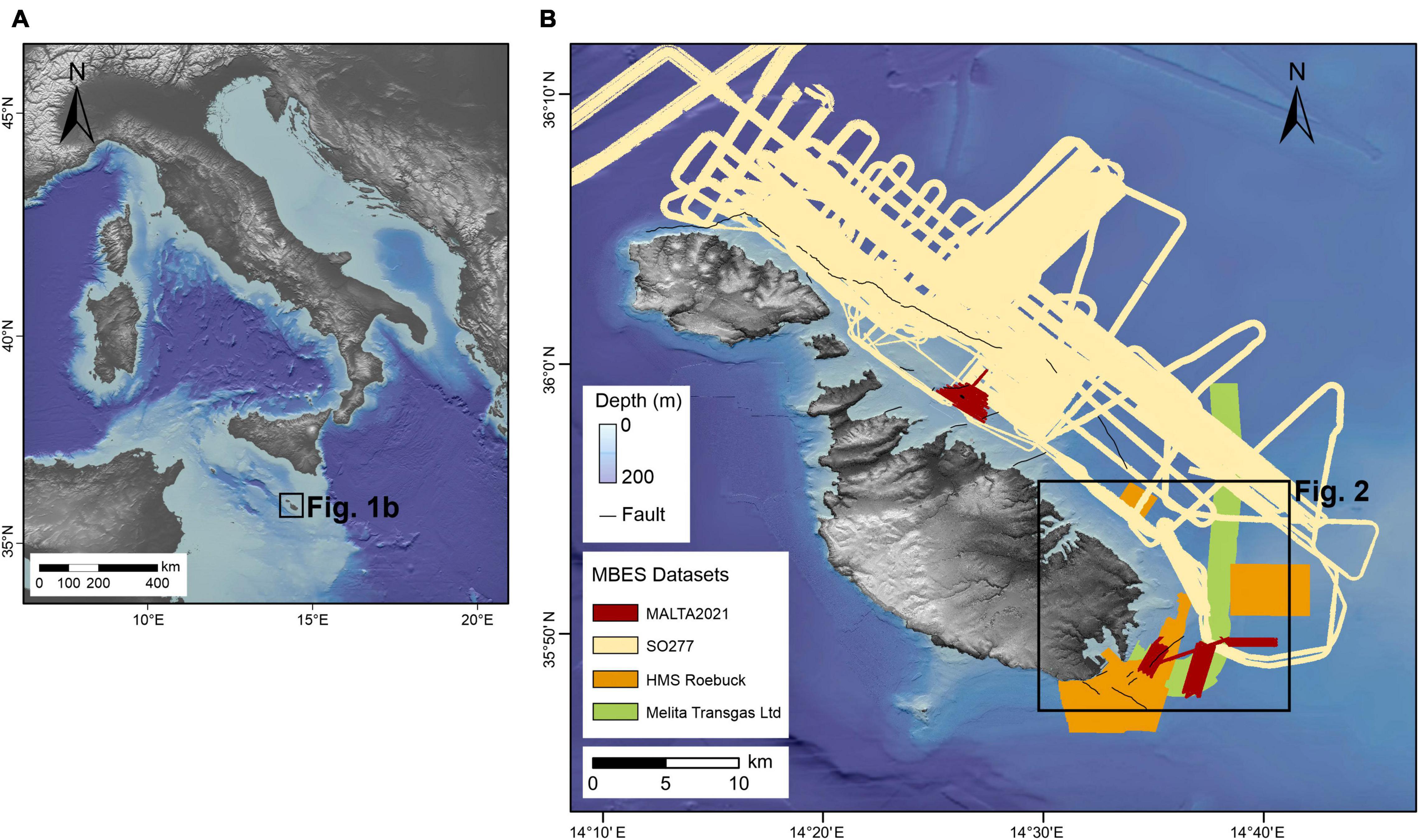
Figure 1. Location map. (A) Overview map (upper left, ETOPO) shows the location of Malta, (B) available bathymetric data from the seafloor surrounding the Maltese Island, research area for this study is marked with a red box. “© Crown Copyright and/or database rights. Reproduced by permission of The Keeper of Public Records and the UK Hydrographic Office (www.GOV.uk/UKHO).”
Materials and Methods
The study area is located offshore of the south-eastern coast of Malta (Figure 1). It comprises 250 km2 of seafloor ranging between 0 and 140 m in depth, with a substrate of maerl, gravel, sand and bedrock outcrops. The key morphological features in the study area are seven fault-related escarpments.
Two types of seafloor data were collected from the study area between 2006 and 2021, as described below.
Multibeam Echosounder Data
The multibeam echosounder data available for this study were acquired during four different oceanographic expeditions: (i) MALTA2021 cruise conducted on board the MV Goldfinder in 2021 using a Norbit iWBMS system operating at a frequency of 400 kHz. (ii) SO277 cruise on board the R/V Sonne in 2020 using a Kongsberg EM 710 system. (iii) Gas pipeline reconnaissance survey carried out by Melita Transgas Ltd. in 2018, using a R2Sonic 2024 system. (iv) HMS Roebuck cruise in 2006 using a Kongsberg EM-1002 system.
Post-processing of the multibeam bathymetry data from these surveys, which included correction for heading, depth, pitch, heave and roll, was conducted using Qimera. Tide and sound velocity profile corrections were applied and the sounding data cleaned to remove erroneous soundings. Bathymetric data were gridded to 1–2 m digital elevation models (DEM). Backscatter data were processed using Fledermaus FMGT at 0.2–1 m.
Mapping of mounds in the study area was carried out using the BGS Seabed Mapping Toolbox developed in 2017 by the British Geological Survey (Gafeira et al., 2018). This toolbox contains four main tools: Feature Delineation Tool, BPI-based Delineation Tool, Feature Short Description Tool, and Feature Full Description Tool. We decided to use the Feature Delineation Tool to delineate confined positive features directly from the bathymetric data. To run the tool, five values—Cutoff Vertical Relief, Minimum Vertical Relief, Minimum Width, Minimum Width/Length Ratio and Buffer Distance—had to be defined. The Cutoff Vertical Relief defines the contour line that will be used to delineate the features; the values set for the Minimum Vertical Relief, Minimum Width and Minimum Size Ratio define the thresholds above which features are going to be mapped. Finally, the Buffer Distance compensates for the fact that the delineation process is based on the features’ internal contour line, which corresponds to the Cutoff Vertical Relief threshold. For this study we used a Cutoff Vertical Relief of 0.2 m, a Minimum Width of 10 m, a Minimum Width/Length Ratio of 0.2, and a Buffer Distance of 5 m; these values have been kept fixed for each mapping operation. The Minimum Vertical Relief was the only parameter that was varied. We tried different values—10, 15, 20, and 30 m—in order to detect morphologies having different extents. The output of this tool was a shapefile of polygons that delineated the mapped features, and a text file reporting the information about the script, input data and parameters used. The extracted polygons were visually compared with the available bathymetry and backscatter data to eliminate features resulting from acquisition artifacts.
Imagery and Samples
ROV surveys were carried out in 2014 and 2021. The 2014 survey was carried out on the R/V Hercules using a Seaeye Panther Plus equipped with a Kongsberg High-Definition color camera. The 2021 survey was carried out on the Delfino and utilized a Shandong Future Robot Co. VVL-V400-4T Underwater Inspection ROV with a Digital HD color 2 megapixel camera. During these surveys, spot dives were carried out at 60 m deep mounds located east of Delimara. Technical dives were carried out in the summer of 2021, during which photographs and samples were collected from mounds at 90–100 m depths. These samples included hand samples of the exposed rock and adjacent sediment in random locations that were easily accessible for the divers. Hand samples were washed with fresh water and air dried before being described. One large rock sample was cut and polished. Sediment samples were visually described.
Results
Multibeam Echosounder Data
Mounds
Automated mapping has identified 2,332 mounds across the surveyed area (Figure 2). An area near the port of Valletta is a known spoil ground and was excluded from our analysis, leaving 1,770 mounds. These features, identified as mounded bioconstructions by subsequent dives (see section “Imagery and Samples”), range in area from a few m2 to > 10,000 m2 (Figure 3a). Mounds have a mean area of ∼600 m2 and an average diameter of ∼20 m. Most mounds inhabit swaths of 500–1,000 m of relatively flat seafloor and are found between 60 and 120 mbpsl. The mounds can be grouped into two main clusters based on depth (Figure 3b), from 40 to 83 mbpsl (Figure 4A) and from 83 to 120 mbpsl (Figure 4B). Spatially, the mounds are clumped together, often aligning along isobaths (Figure 2). There is no clear relation between the mound size and water depth, and all clusters exhibit similar size distributions. The mean distance between adjacent mounds is 2.9 ± 102.5 m for the entire population. Densities in the in the 40–83 mbpsl cluster are higher than in the 83–120 mbpsl cluster, with the mean distance between mounds being 97.5 ± 131.3 to 51.5 ± 97.5 m, respectively. In some places, mounds are aligned and exhibit some linearity or conformity to isobaths (Figure 2). Notably, at around 80 mbpsl the mounds are located on or near escarpments. Due to their clustering and the adjacency of some mounds and structures to such escarpments, elevation extraction was not very reliable. Individual mounds that are not part of clusters, have elevations of 1–5 m above the local topography. On multibeam backscatter data (Figure 5), the mounds are characterized by high acoustic reflectivity, with the surrounding seafloor having intermediate acoustic reflectivity. The seafloor away from the mounds is characterized by low acoustic reflectivity.
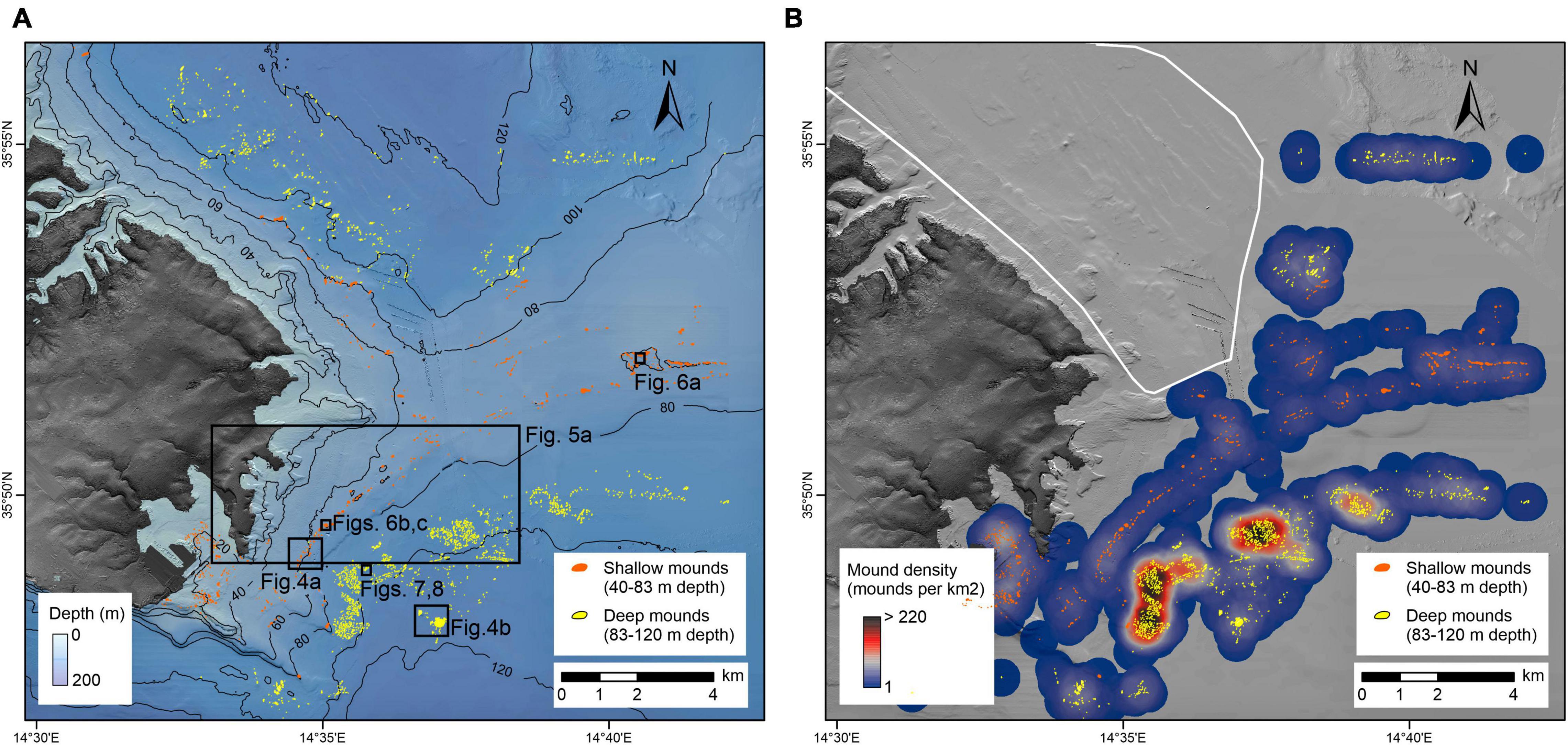
Figure 2. (A) Output of automated mapping showing the locations of the mounds. (B) Density map with 1,000 m search radius highlighting areas of high mound density and locations of spoil grounds.
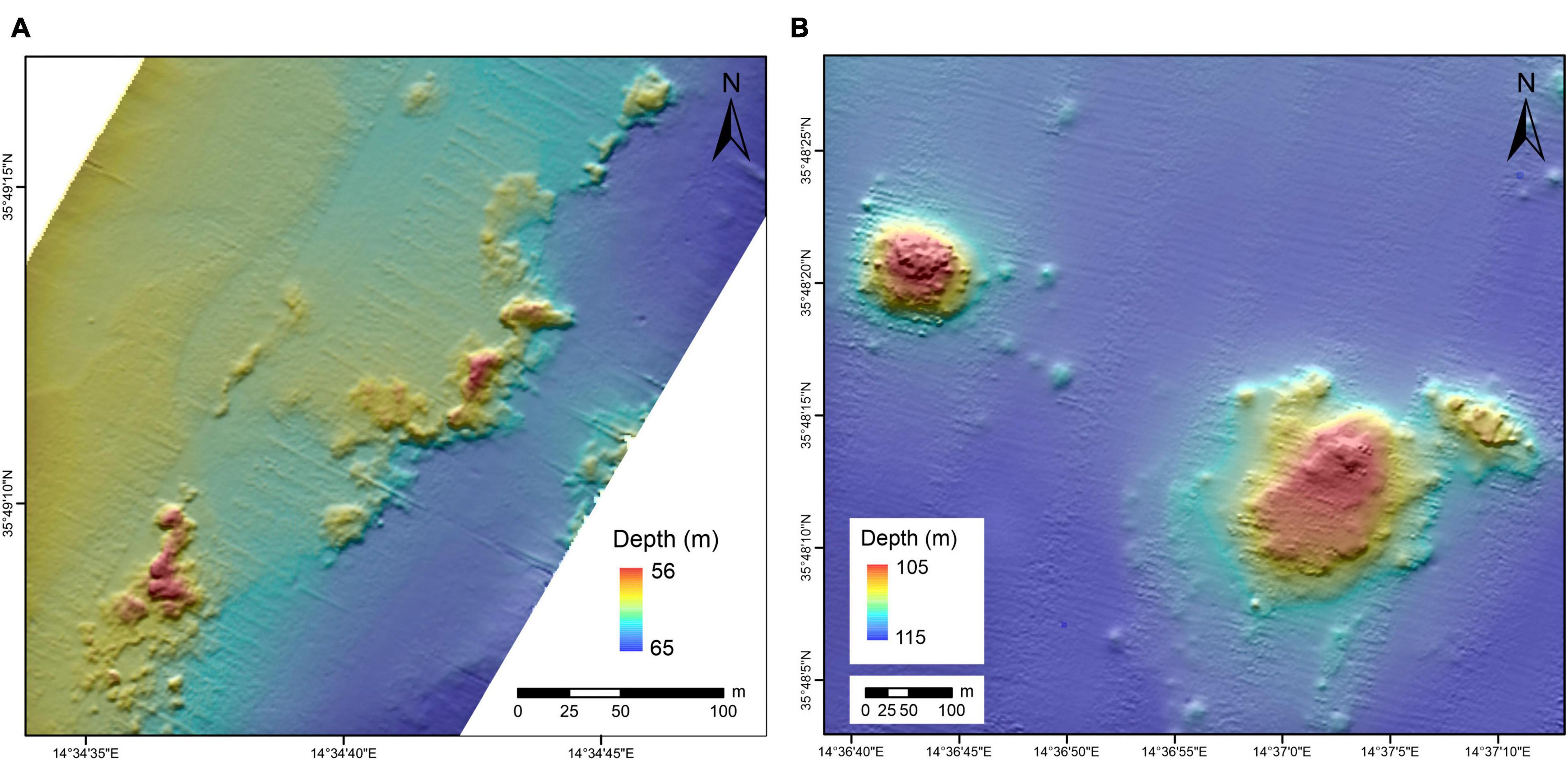
Figure 4. Bathymetric maps of the mounds. (A) Case example for mounds in the 40–83 mbspl clusters and (B) case example for mounds in the 83–120 mbspl cluster.
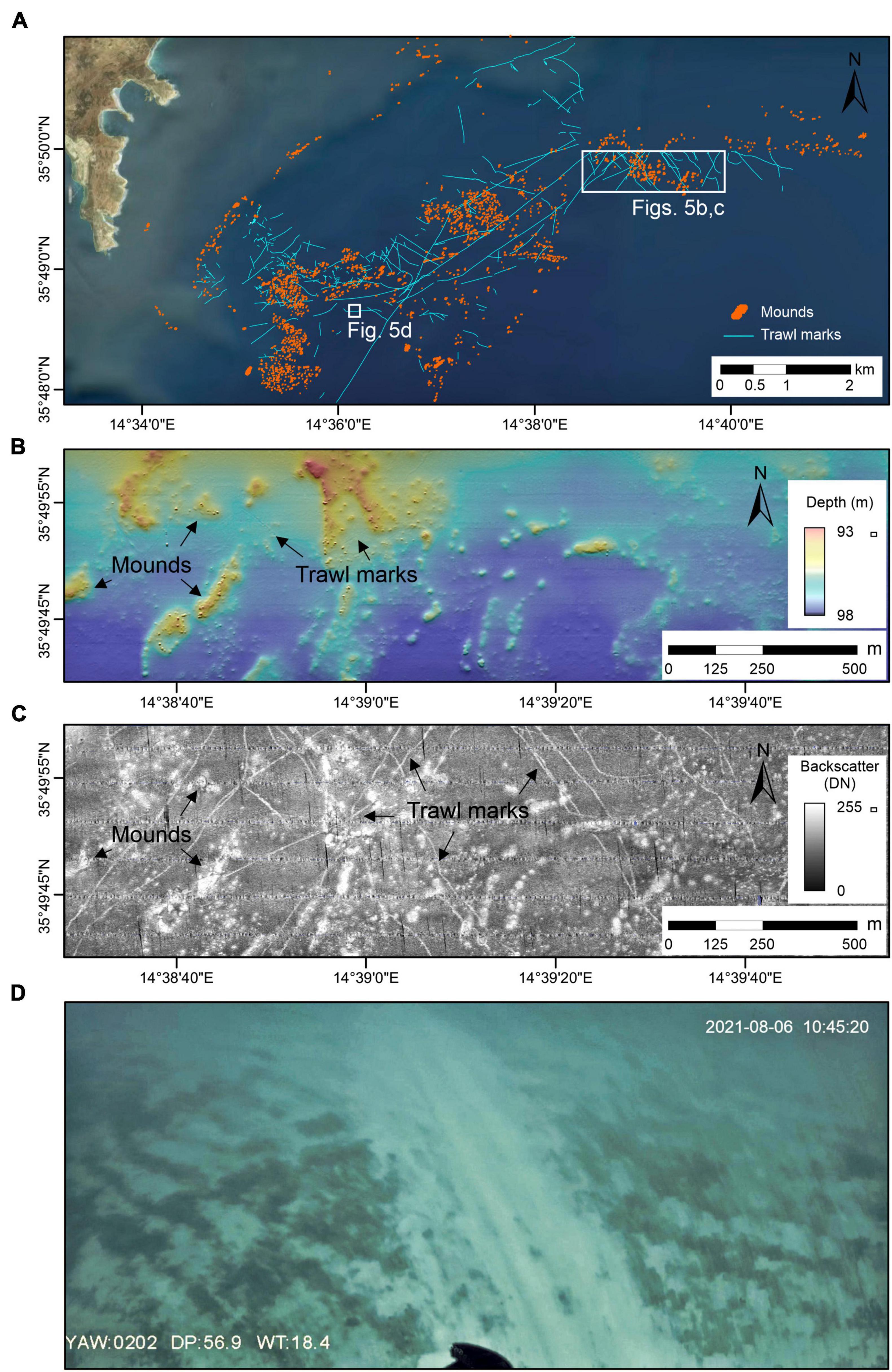
Figure 5. Evidence of trawl marks in the study area. (A) Map showing trawl marks occurrence in the study area. (B) Shaded-relief color multibeam bathymetry and (C) backscatter imagery of an area of mounds highly impacted by trawling; (D) ROV image of a trawl mark probably caused by a trawl door.
Trawl Marks
The multibeam bathymetry and backscatter data reveal the presence of trawl marks across the entire study area (Figure 5A). In the bathymetric data, some trawl marks are visible as narrow furrows that can be up to 20 cm deep (Figure 5B). In the backscatter imagery, trawl marks are displayed as narrow (∼4 m wide) areas of higher backscatter values compared to the surrounding seafloor (Figure 5C). Trawl marks range from about 20 m to over 4.5 km in length, having both straight and curved forms. They generally appear as individual features, although parallel marks are also visible with a mean distance of 60–70 m. In some trawl marks, positive relief (∼5 cm high) can be identified along both sides of the furrow, indicating pushed up sediments.
Imagery and Samples
In all locations surveyed by ROV, the mounds were found to be rock outcrops composed of an agglomeration of smaller rocky elements spread across a small area (Figures 6A,B, 7A). A fair number of the rocks are taller than wide. In most cases, they do not appear to be detached boulders but extend into the subsurface. The rocks are for the most part rounded, although locally jagged and with sharp edges, particularly at around 60 mbpsl. The surface of the rocks forming the mounds is irregular with some crevasses and an overall rugose texture where the rock surface is exposed with abundant associated organisms (the most relevant of which are listed in Table 1).
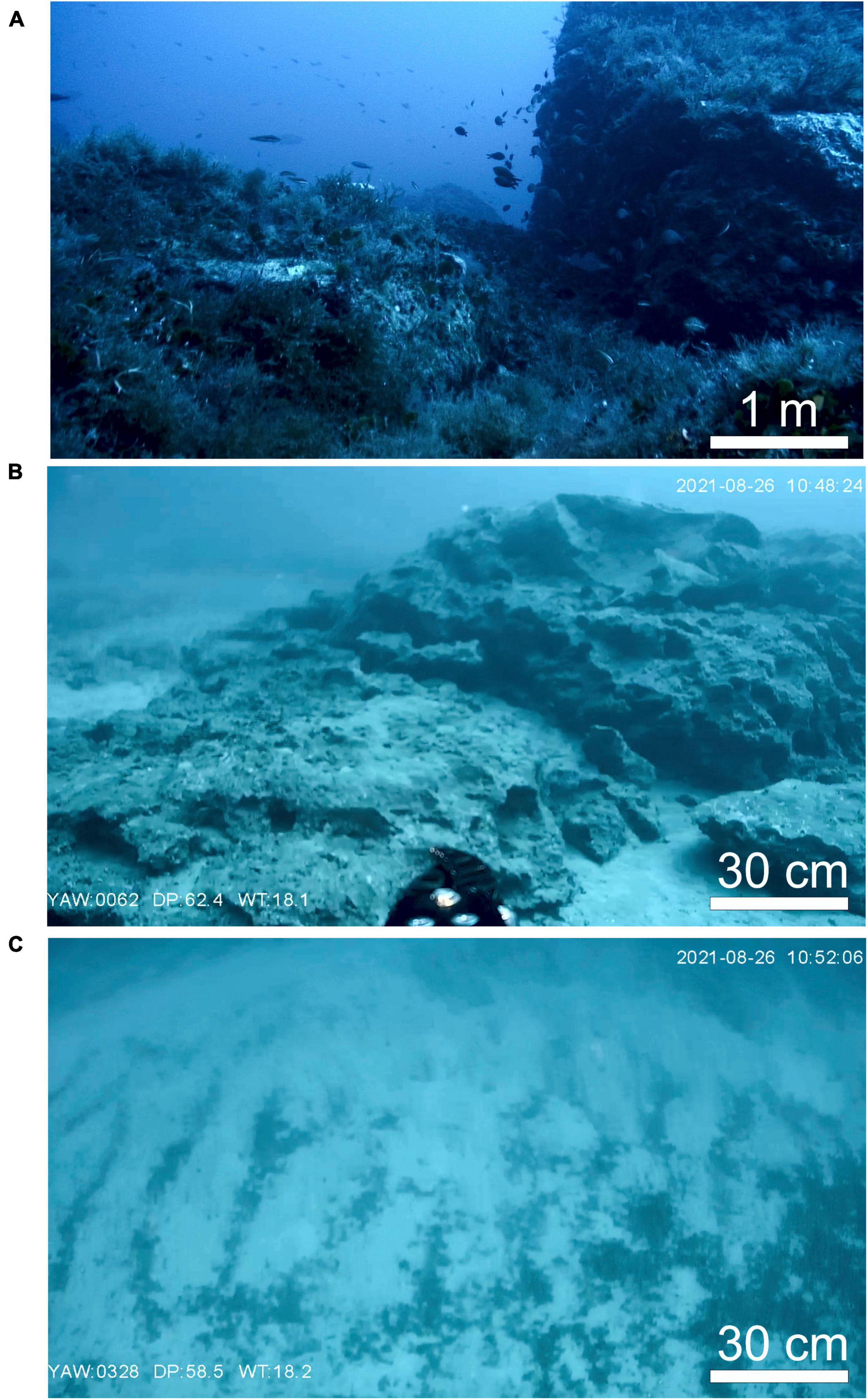
Figure 6. Imagery of mounds from the 40 to 83 m cluster located at 60 mbpsl. (A) Rock outcrops in mounds in the northern part of the study area; (B) rock outcrops in mounds in the southern part of the study area. (C) Sediment ripples adjacent to a mound from the southern part of the study area.
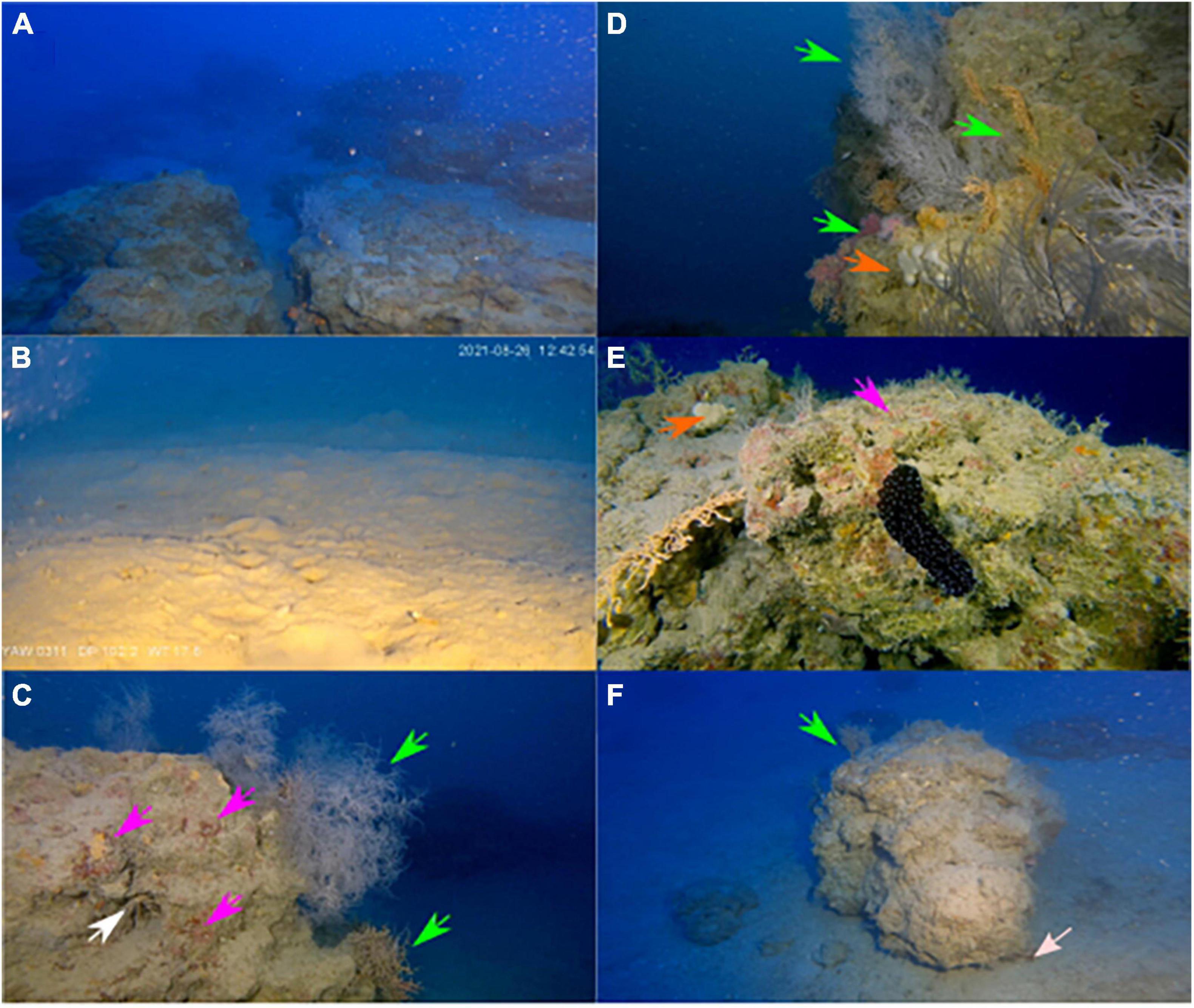
Figure 7. Imagery from mounds at 90 mbpsl. (A) Rock outcrops in mounds from in the southern part of the study area; (B) sediments adjacent to a mound in the southern part of the study area; (C) rock outcrop from a mound in the southern part of the study area with red alga (magenta arrows), Anthipatarians (green arrows) and sea urchin (white arrow); (D) rock outcrop from a mound in the southern part of the study area with Anthipatarians and gorgonian (green arrows, white and orange specimens, respectively) and sponges (orange arrow); (E) rock outcrop from a mound in the southern part of the study area with an Holoturia, note also sponges (orange arrow) and red alga (magenta arrows). (F) Rock outcrop from a mound in the southern part of the study area with a notch at the base (pinkish-white arrow).
Sediment adjacent to the mounds at around 60 mbpsl (Figure 6C) is arranged in small ripples with a dark crest. Trawl marks are present and visible within a short distance of the mounds explored during the dives (Figures 5A,D). Mounds found around a depth of 60 mbpsl appear in two forms: in the north of the study area, they are extensively covered by soft body organisms, as well as possible hydrozoans and macroalgae, although no clear calcifiers are present (Figure 6A). In the south of the study area, the mounds are barren with some sediment coating (Figure 6B).
Sediment adjacent to mounds at around 90 mbspl also exhibit some ripples, but these do not express any consistent character. The seafloor, however, does exhibit significantly more bioturbation activity (Figure 7B) relative to shallower waters (Figure 6C). The sediment around the mounds shows variability in grain size over short distances. On some sides of the mound, the sediment consists of the sediment appears as a fine to medium sand, whereas on other sides the sediment ranges from fine to very coarse sand. The coarser grains have poor roundness and sphericity and are composed of fragments of branching bryozoans, shell fragments and other unidentified bioclasts. Based on the sediment samples and optical imagery, the higher acoustic reflectivity in the backscatter data (Figure 5C) appears to be associated with coarse-grained material whereas the lower reflectivity is finer-grained sediment.
The crust of the mounds was sampled from a mound at ∼90 mbpsl. The crust is calcareous (Figure 8a) and composed of interlocking meshes of different encrusting organisms, including coralline algae (Figure 8b), vermiforms, bryozoans and foraminifera. These appear to trap fine micritic material and exhibit areas with iron oxides (Figure 8c). The vermiforms are varied in form and appear to include serpulid worms as well as possible vermetid gastropods that occur in association with encrusting foraminifera (Figure 8d). The outer coating of the crust is covered by encrusters such as vermiforms, crustose coralline algae and small attached bivalves. The mounds found between 90 and 100 mbpsl exhibit different levels of fouling (Figures 7C–F). The mounds’ surfaces show multiple reddish patches (Figure 7C), potentially formed by crustose coralline algae and possibly encrusting foraminifera. Additionally, the surface is covered by different types of vermiforms (probable serpulids). Anthipatarians and gorgonians, echinoderms (Astrospartus mediterraneus, NE), sea urchins, sea cucumber [Holothuria (Panningothuria) forskali, LC] and sponges (Chondrosia reniformis, NE) (Figures 7C–F). Some spots of barren rocks and with a thin sediment coating are also present. In some locations, there is a notch at the base of the mounds (Figure 7F), but it is not clear if it is erosive or the product of differential growth of mounds crust. A diverse fish fauna was observed in the proximity of the mounds at all depths.
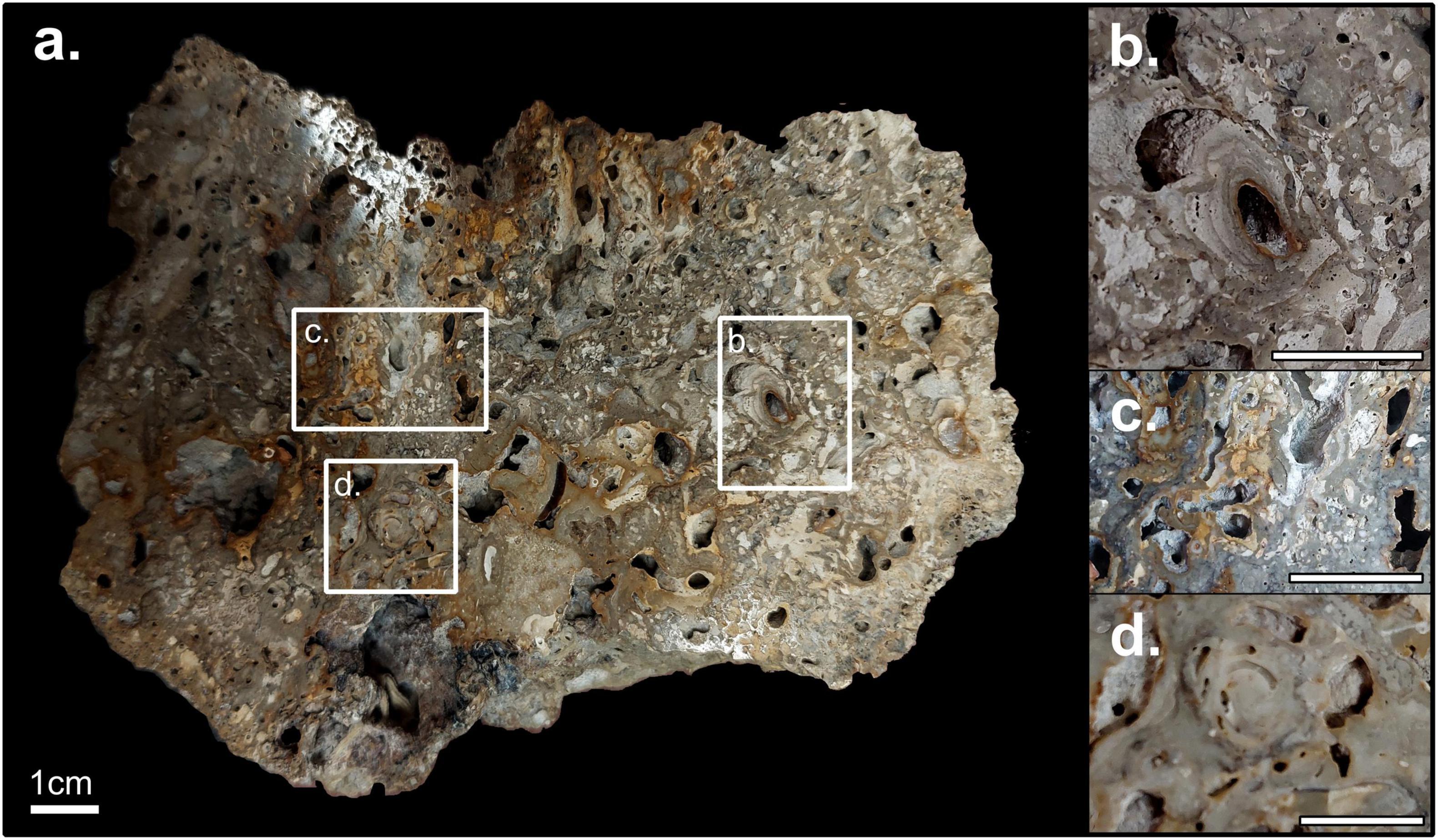
Figure 8. (a) Polished slab of surface crust samples of a mound from the at 90 mbpsl (Figure 4). (b) Coralline algae rounded encrustation. (c) Iron oxide staining. (d) Serpulid. All scale bars are 1 cm.
Discussion
Origin of Mounds
Presently > 1,700 features can be found in water depths of 40–120 mbpsl offshore SE Malta. These are mounded bodies composed of bioconstruction, which we identify as biogenic mounds. When inspected these mounds were found to be elevated carbonate edifices with minor biogenic coating. These structures, several meters in height, are much smaller and denser than scleractinian cold water coral mounds described in greater depths in the Mediterranean (Remia and Taviani, 2005; Savini et al., 2014; Corbera et al., 2019). As such, their genesis is probably somewhat different. The carbonate is a collection of encrusting organisms that have accumulated over time and make the outer crust of the mound. However, the inner structure is unknown. We consider three potential origins for the underlying core of the mounds:
(i) Geological boulders: The identified mounds are comparable in shape to boulders found along the present Maltese shoreline, which have been attributed to extreme storm events or tsunamis (Biolchi et al., 2016). However, our mounds are larger, more numerous, and not clustered near any steep topography (onshore and offshore), but rather far from them or even above. As such, the mounds are probably not boulder accumulations with subsequent encrusting.
(ii) Ancient shallow-water biogenic reefs: In high latitudes and deep waters, the growth of mounds is limited to interglacial periods as glacier growth limits their development during glacial periods (Rüggeberg et al., 2007). However, in lower latitudes and shallower depths, such as our study area, the opposite may occur. Mounds may develop in what are now mesophotic depths as euphotic reefs during glacial times and then drown during interglacial periods (Webster et al., 2004; Beaman et al., 2008). These drowned reefs often form or reside atop terraces formed during prolonged periods of relatively stable sea-level over the last glacial cycle (Carter et al., 1986; Grigg et al., 2002). Mounds found between 40 and 83 mbpsl inhabit surfaces formed during MIS5 and MIS3-4 lowstands (Figure 9). As the substrate of the mounds, under a thin sediment coating, is probably geological rock, extensive truncation is needed to clear such a surface. Coastal retreat of rocky shores in the North Atlantic (where tides and weather are more abrasive than the central Mediterranean) has been estimated at ∼7 cm/yr (Lim et al., 2010). As such, abrasion of the terraces inhabited by the mounds in the 40–83 mbpsl cluster will be in the order of thousands of years old, much longer than the short standstills of post-glacial transgression, in this case from the last glacial minimum, and more likely related to earlier stages such as MIS 5–3. Colonization likely occurred both during that time and during the post-glacial transgression.
(iii) Authigenic carbonate edifices related to seepage: The above explanation (ii) does not apply well to the more numerous features between 83 and 120 mbpsl. This is because they reside in depths that do not correspond to any distinct standstill in the last glacial cycle or the preceding one (Figure 5). These could, instead, be the product of seepage of two types of fluids: meteoric groundwater or hydrocarbons (notably methane). The scenario for the former would entail meteoric groundwater discharging at the coast or shallow seafloor (submarine groundwater discharge). Such discharge would have been enhanced during glacial drawdowns due to the development of higher hydraulic head (Faure et al., 2002). The seeps would lead to the accumulation of authigenic carbonates or alternatively provide a local nutrient spike (Knee et al., 2016; Gierlowski-Kordesch et al., 2021) that could promote colonization. These initial edifices could be colonized at later times and continue to accumulate in the available hard substrate (Hovland and Risk, 2003). Such a scenario is feasible in view of the occurrence of coastal discharge at present (Sustainable Energy and Water Conservation Unit, 2015) and the occurrence of freshened groundwater offshore the coast of SE Malta, which is thought to have been emplaced during sea-level lowstands (Haroon et al., 2021). Extension of the groundwater body underneath the Malta Plateau across the entire depth range of the mounds during glacial periods is plausible. A second scenario entails the escape of methane at the seafloor. Micallef et al. (2019) documented an active fluid system responsible for degassing of methane onshore and offshore the Maltese Islands, with the fluid flowing preferentially along faults and, occasionally, pipe structures. The localization of these seeps on preexisting flow paths such as faults (including blind faults) could explain some of the linearity observed in some of the clusters.
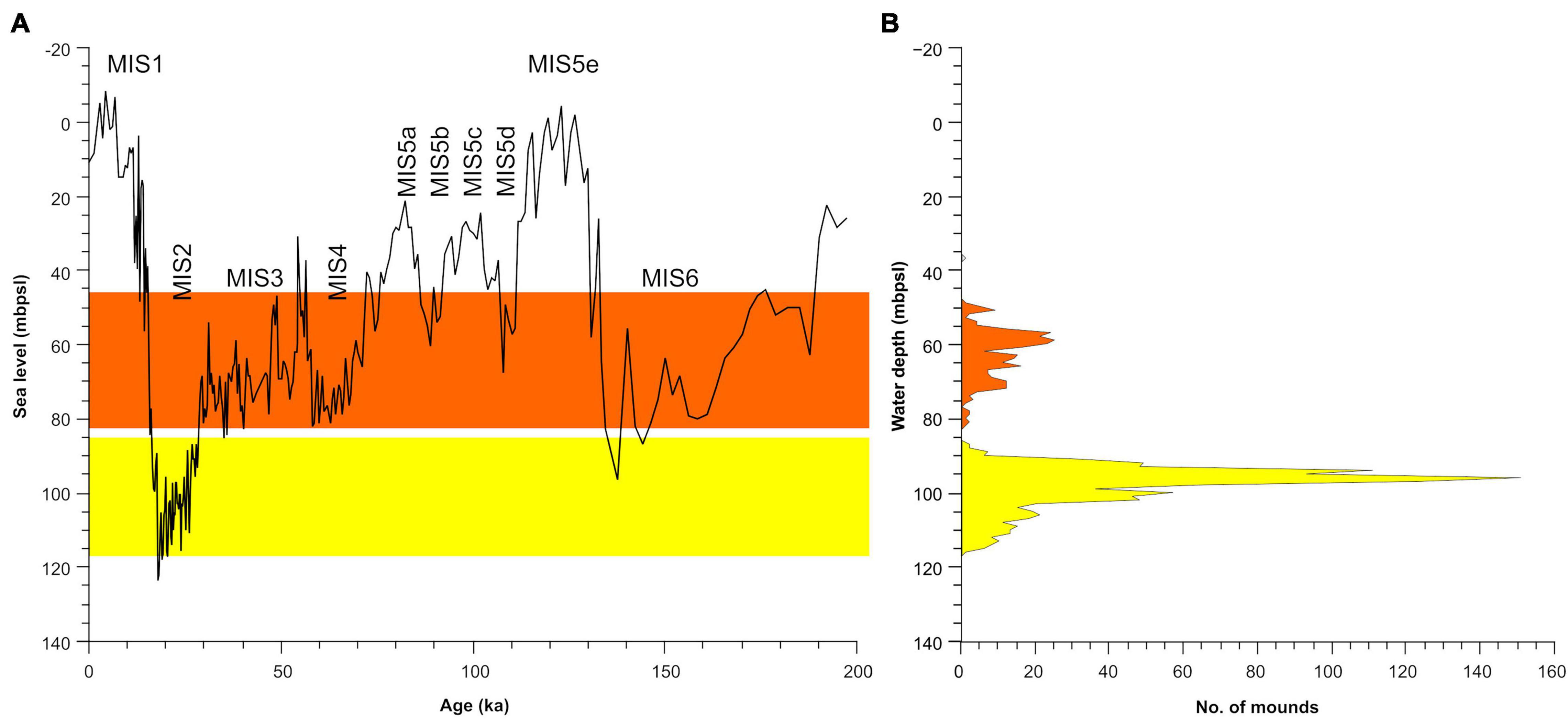
Figure 9. (A) Sea-level curve of the last glacial cycle (left, Miller et al., 2020) and (B) water depth ranges of the mounds. Note that most of the mounds relate to water depths associated with low stands. Gray gradient illustrates relative density of mounds in a given depth.
At present, the mounds appear to be living mesophotic reefs, offering refuge and feeding grounds for pelagicand benthic organisms as well as a hard substrate for recruitment (Figure 6). Assuming a conservative 1 mm/year growth rate based on the coralline algae for their accretion (Caragnano et al., 2016), had their current phase of accretion begun in the post Last Glacial Maximum transgression, that would allow for ∼1.5 m of accumulation for the 83–120 mbpsl cluster. Given the strong vertical component of some of the observed elements (e.g., Figure 7F), it is likely much of this growth was vertical. However, this vertical growth could not keep up with the rapid rate of sea-level rise during the last glacial cycle (Lambeck et al., 2011). If the sedimentation rate on the Malta Plateau were higher than the growth rate, it is reasonable to assume many of these mounds would have been buried and not exposed at the surface. The mounds appear to be local sources of sediment, as evident by the larger grains size and kind of sediment near the mounds. As such, it is likely that the low sedimentation rate in the area was key to the extensive occurrence and preservation of the mounds.
Risks to Biogenic Mounds
The mounds offshore SE Malta and the species they host (see species list, Table 1) deserve protection. Unfortunately, the mounds are exposed to intensive anthropogenic stress. The trawl marks that crisscross across the study area, which we predominantly associate to fishing and anchoring activities (Figure 5), have damaged the mounds directly and may negatively affect the filter-feeding organisms that build them via sediment resuspension, as had occurred in other cases (Caddy, 1973; Rogers, 1999; De Juan et al., 2007).
Summary and Conclusion
We have registered hundreds of small elevated features offshore eastern Malta which we identified as biogenic mounds. These mounds are clustered in two main groups, between 40–83 and 83–120 mbpsl. The mounds are rocky, composed of interlocking biogenic encrustation by calcifiers. They are a few meters in height and up to a few tens of meters in diameter, occurring mostly as relatively tight clusters. Their origin is unclear; given their composition, these structures likely took several thousands of years to reach their current size, or possibly longer. It is not unlikely that these two clusters formed by different mechanisms. We propose that the 40–83 mbpsl cluster comprises drowned reefs formed upon terraces excavated in the last glacial cycle. Contrarily, the formation of the 83–120 mbpsl cluster was likely related to the seepage of fluid (groundwater and/or methane). Presently, the surface of the mounds is colonized by a mesophotic hard bottom community including endangered species (see species list). These structures are ecologically relevant and a unique ecotope that would have potentially required prolonged stable conditions to grow, but that is currently under risk from anthropogenic activity. Mostly directly, trawling activity is extensive around these features and may lead to their imminent destruction. Urgent legislative action is therefore required to declare the seafloor offshore SE Malta as a Marine Special Area of Conservation similar to other reef and biogenic buildup areas offshore Malta (ERA, 2021).
Data Availability Statement
The original contributions presented in the study are included in the article/supplementary material, further inquiries can be directed to the corresponding author/s.
Author Contributions
Research conceptualization and principle writing was done by AM, OB, RD, TL, and AG also took part in field work and data collection. OB, RD, TL, and AV did the Data processing. AS provided the additional input. AM, OB, and RD provided funding for this work. All authors contributed to the article and approved the submitted version.
Funding
This research was supported by the Marie Skłodowska-Curie grant agreements no. 101003394 (RhodoMalta) and 867471 (TrawledSeas), and the European Research Council (ERC) (grant agreement no. 677898; MARCAN), under the European Union’s Horizon 2020 Research and Innovation Programme, the University of Malta and the SMART Project through the Helmholtz European Partnering Initiative (Project ID No. PIE-0004). Part of this study was supported by U.K. NERC National Capability funding (NE/R015953/1).
Conflict of Interest
The authors declare that the research was conducted in the absence of any commercial or financial relationships that could be construed as a potential conflict of interest.
Publisher’s Note
All claims expressed in this article are solely those of the authors and do not necessarily represent those of their affiliated organizations, or those of the publisher, the editors and the reviewers. Any product that may be evaluated in this article, or claim that may be made by its manufacturer, is not guaranteed or endorsed by the publisher.
Acknowledgments
We kindly acknowledge the captain, crew and scientists on the R/V Sonne expedition SO277 for their assistance with data collection, and Kevin Sciberras for organizing the dive surveys onboard the Delfino. We are grateful to the UK Hydrographic Office and Melita Trangas Co., Ltd. for providing access to their data. The surveys were possible following permits issued by the Department of Continental Shelf, the Environment and Resources Authority, the Superintendence of Cultural Heritage and the Ports and Yachting Directorate.
References
Abbey, E., Webster, J. M., Braga, J. C., Jacobsen, G. E., Thorogood, G., Thomas, A. L., et al. (2013). Deglacial mesophotic reef demise on the Great Barrier Reef. Palaeogeogr. Palaeoclimatol. Palaeoecol. 392, 473–494. doi: 10.1016/j.palaeo.2013.09.032
Aloisi, G., Pierre, C., Rouchy, J. M., Foucher, J. P., and Woodside, J. (2000). Methane-related authigenic carbonates of Eastern Mediterranean Sea mud volcanoes and their possible relation to gas hydrate destabilisation. Earth Planet. Sci. Lett. 184, 321–338. doi: 10.1016/S0012-821X(00)00322-8
Amado-Filho, G. M., Moura, R. L., Bastos, A. C., Francini-Filho, R. B., Pereira-Filho, G. H., Bahia, R. G., et al. (2016). Mesophotic ecosystems of the unique South Atlantic atoll are composed by rhodolith beds and scattered consolidated reefs. Mar. Biodivers. 46, 933–936. doi: 10.1007/s12526-015-0441-6
Angeletti, L., Castellan, G., Montagna, P., Remia, A., and Taviani, M. (2020). The “Corsica Channel Cold-Water Coral Province” (Mediterranean Sea). Front. Mar. Sci. 7:661. doi: 10.3389/fmars.2020.00661
Ballesteros, E., Avançats, E., and Csic, D. B. (2006). Mediterranean coralligenous assemblages: a synthesis of present knowledge. Communities 44, 123–195. doi: 10.1016/j.jenvman.2012.07.024
Bare, A. Y., Grimshaw, K. L., Rooney, J. J., Sabater, M. G., Fenner, D., and Carroll, B. (2010). Mesophotic communities of the insular shelf at Tutuila, American Samoa. Coral Reefs 29, 369–377. doi: 10.1007/s00338-010-0600-y
Beaman, R. J., Webster, J. M., and Wust, R. A. J. (2008). New evidence for drowned shelf edge reefs in the great barrier reef Australia. Mar. Geol. 247, 17–34. doi: 10.1016/j.margeo.2007.08.001
Benedetti, M. C., Priori, C., Erra, F., and Santangelo, G. (2016). Growth patterns in mesophotic octocorals: timing the branching process in the highly-valuable Mediterranean Corallium rubrum. Estuar. Coast. Shelf Sci. 171, 106–110. doi: 10.1016/j.ecss.2015.12.026
Biolchi, S., Furlani, S., Antonioli, F., Baldassini, N., Causon Deguara, J., Devoto, S., et al. (2016). Boulder accumulations related to extreme wave events on the eastern coast of Malta. Nat. Hazards Earth Syst. Sci. 16, 737–756. doi: 10.5194/nhess-16-737-2016
Bracchi, V. A., Basso, D., Marchese, F., Corselli, C., and Savini, A. (2017). Coralligenous morphotypes on subhorizontal substrate: a new categorization. Cont. Shelf Res. 144, 10–20. doi: 10.1016/j.csr.2017.06.005
Caddy, J. F. (1973). Underwater observations on tracks of dredges and trawls: some effects of dredging on a scallop ground. J. Fish. Res. Bd. Can. 30, 173–180.
Cangemi, M., Di Leonardo, R., Bellanca, A., Cundy, A., Neri, R., and Angelone, M. (2010). Geochemistry and mineralogy of sediments and authigenic carbonates from the Malta Plateau, Strait of Sicily (Central Mediterranean): relationships with mud/fluid release from a mud volcano system. Chem. Geol. 276, 294–308. doi: 10.1016/j.chemgeo.2010.06.014
Caragnano, A., Basso, D., and Rodondi, G. (2016). Growth rates and ecology of coralline rhodoliths from the Ras Ghamila back reef lagoon, Red Sea. Mar. Ecol. 37, 713–726. doi: 10.1111/maec.12371
Carter, R. M., Carter, L., and Johnson, D. P. (1986). Submergent shorelines in the SW Pacific: evidence for an episodic post-glacial transgression. Sedimentology 33, 629–649. doi: 10.1111/j.1365-3091.1986.tb01967.x
Carvalho, M. S., Lopes, D. A., Cosme, B., and Hajdu, E. (2016). Seven new species of sponges (Porifera) from deep-sea coral mounds at Campos Basin (SW Atlantic). Helgol. Mar. Res. 70:10. doi: 10.1186/s10152-016-0461-z
Corbera, G., Lo Iacono, C., Gràcia, E., Grinyó, J., Pierdomenico, M., Huvenne, V. A. I., et al. (2019). Ecological characterisation of a Mediterranean cold-water coral reef: cabliers coral mound province (Alboran Sea, western Mediterranean). Prog. Oceanogr. 175, 245–262. doi: 10.1016/j.pocean.2019.04.010
Dando, P. R. (2010). “Biological communities at marine shallow-water vent and seep sites,” in The Vent and Seep Biota. Topics in Geobiology, 33, ed. S. Kiel (Dordrecht: Springer), doi: 10.1007/978-90-481-9572-5_11
De Juan, S., Thrush, S. F., and Demestre, M. (2007). Functional changes as indicators of trawling disturbance on a benthic community located in a fishing ground (NW Mediterranean Sea). Mar. Ecol. Prog. Ser. 334, 117–129.
Dorschel, B., Hebbeln, D., Foubert, A., White, M., and Wheeler, A. J. (2007). Hydrodynamics and cold-water coral facies distribution related to recent sedimentary processes at Galway Mound west of Ireland. Mar. Geol. 244, 184–195. doi: 10.1016/j.margeo.2007.06.010
Drago, A. F., Sorgente, R., and Ribotti, A. (2003). A high resolution hydrodynamic 3-D model simulation of the malta shelf area. Ann. Geophys. 21, 323–344. doi: 10.5194/angeo-21-323-2003
Dumalagan, E. E., Cabaitan, P. C., Bridge, T. C. L., Go, K. T., Quimpo, T. J. R., Olavides, R. D. D., et al. (2019). Spatial variability in benthic assemblage composition in shallow and upper mesophotic coral ecosystems in the Philippines. Mar. Environ. Res. 150:104772. doi: 10.1016/j.marenvres.2019.104772
ERA (2021). Conservation Objectives & Measures for Malta’s Marine Natura 2000 Sites. Valletta: Environment & Resources Authority, 61.
Faure, H., Walter, R. C., and Grant, D. R. (2002). The coastal oasis: ice age springs on emerged continental shelves. Glob. Planet. Change 33, 47–56. doi: 10.1016/S0921-8181(02)00060-7
Fink, H. G., Wienberg, C., De Pol-Holz, R., and Hebbeln, D. (2015). Spatio-temporal distribution patterns of Mediterranean cold-water corals (Lophelia pertusa and Madrepora oculata) during the past 14,000 years. Deep Sea Res. Part I Oceanogr. Res. Pap. 103, 37–48. doi: 10.1016/j.dsr.2015.05.006
Foubert, A., and Henriet, J.-P. (2009). Nature and significance of the recent carbonate mound record. The mound challenger code. Lect. Notes Earth Sci. 126, 298. doi: 10.1007/978-3-642-00290-8
Foubert, A., Depreiter, D., Beck, T., Maignien, L., Pannemans, B., Frank, N., et al. (2008). Carbonate mounds in a mud volcano province off north-west Morocco: key to processes and controls. Mar. Geol. 248, 74–96. doi: 10.1016/j.margeo.2007.10.012
Gafeira, J., Dolan, M., and Monteys, X. (2018). Geomorphometric characterization of pockmarks by using a GIS-based semi-automated toolbox. Geosciences 8:154. doi: 10.3390/geosciences8050154
Gardiner, W., Grasso, M., and Sedgeley, D. (1995). Plio-pleistocene fault movement as evidence for mega-block kinematics within the Hyblean—Malta Plateau Central Mediterranean. J. Geodyn. 19, 35–51. doi: 10.1016/0264-3707(94)00006-9
Gierlowski-Kordesch, E. H., Rothwell, G. W., Stockey, R. A., and Finkelstein, D. B. (2021). “Submarine groundwater discharge as a catalyst for eodiagenetic carbonate cements within marine sedimentary basins,” in Limnogeology: Progress, Challenges and Opportunities: A Tribute to Elizabeth Gierlowski-Kordesch, eds M. R. Rosen, D. Finkelstein, L. Park Boush, and S. Pla-Pueyo (Berlin: Springer Nature), 445–468.
Glogowski, S., Dullo, W.-C., Feldens, P., Liebetrau, V., von Reumont, J., Hühnerbach, V., et al. (2015). The Eugen Seibold coral mounds offshore western Morocco: oceanographic and bathymetric boundary conditions of a newly discovered cold-water coral province. Geo Mar. Lett. 35, 257–269. doi: 10.1007/s00367-015-0405-7
Goren, L., Idan, T., Shefer, S., and Ilan, M. (2021). Macrofauna inhabiting massive demosponges from shallow and mesophotic habitats along the Israeli Mediterranean Coast. Front. Mar. Sci. 7:612779. doi: 10.3389/fmars.2020.612779
Greene, H. G., Yoklavich, M. M., Starr, R. M., O’Connell, V. M., Wakefield, W. W., Sullivan, D. E., et al. (1999). A classification scheme for deep seafloor habitats. Oceanol. Acta 22, 663–678. doi: 10.1016/S0399-1784(00)88957-4
Grigg, R., Grossman, E., Earle, S., Gittings, S., Lott, D., and McDonough, J. (2002). Drowned reefs and antecedent karst topography, Au’au Channel, S.E. Hawaiian Islands. Coral Reefs 21, 73–82. doi: 10.1007/s00338-001-0203-8
Groves, S. H., Holstein, D. M., Enochs, I. C., Kolodzeij, G., Manzello, D. P., Brandt, M. E., et al. (2018). Growth rates of Porites astreoides and Orbicella franksi in mesophotic habitats surrounding St. Thomas, US Virgin Islands. Coral Reefs 37, 345–354. doi: 10.1007/s00338-018-1660-7
Haroon, A., Micallef, A., Jegen, M., Schwalenberg, K., Karstens, J., Berndt, C., et al. (2021). Electrical resistivity anomalies offshore a carbonate coastline: evidence for freshened groundwater? Geophys. Res. Lett. 48:e2020GL091909. doi: 10.1029/2020GL091909
Hebbeln, D., Bender, M., Gaide, S., Titschack, J., Vandorpe, T., Van Rooij, D., et al. (2019). Thousands of cold-water coral mounds along the Moroccan Atlantic continental margin: distribution and morphometry. Mar. Geol. 411, 51–61. doi: 10.1016/j.margeo.2019.02.001
Hebbeln, D., Wienberg, C., Wintersteller, P., Freiwald, A., Becker, M., Beuck, L., et al. (2014). Environmental forcing of the Campeche cold-water coral province, southern Gulf of Mexico. Biogeosciences 11, 1799–1815. doi: 10.5194/bg-11-1799-2014
Henriet, J. P., Hamoumi, N., Da Silva, A. C., Foubert, A., Lauridsen, B. W., Rüggeberg, A., et al. (2014). Carbonate mounds: from paradox to World Heritage. Mar. Geol. 352, 89–110. doi: 10.1016/j.margeo.2014.01.008
Holland, C. W., Etiope, G., Milkov, A. V., Michelozzi, E., and Favali, P. (2003). Mud volcanoes discovered offshore Sicily. Mar. Geol. 199, 1–6. doi: 10.1016/S0025-3227(03)00125-7
Hovland, M., and Risk, M. (2003). Do Norwegian deep-water coral reefs rely on seeping fluids? Mar. Geol. 198, 83–96. doi: 10.1016/S0025-3227(03)00096-3
Hovland, M., Croker, P. F., and Martin, M. (1994). Fault-associated seabed mounds (carbonate knolls?) off western Ireland and north-west Australia. Mar. Pet. Geol. 11, 232–246. doi: 10.1016/0264-8172(94)90099-X
Illies, J. (1980). “Form and function of graben structures: the Maltese Islands,” in Mobile Earth: International Geodynamics Project Final Report of the Federal Republic of Germany, eds H. Closs et al. (Boppard: Boldt), 161–184.
Ingrosso, G., Abbiati, M., Badalamenti, F., Bavestrello, G., Belmonte, G., Cannas, R., et al. (2018). Mediterranean bioconstructions along the Italian coast. Adv. Mar. Biol. 78, 61–136. doi: 10.1016/bs.amb.2018.05.001
James, N. P., Feary, D. A., Betzler, C., Bone, Y., Holbourn, A. E., Li, Q. Y., et al. (2004). Origin of late pleistocene bryozoan reef mounds; Great Australian Bight. J. Sediment. Res. 74, 20–48. doi: 10.1306/062303740020
Knee, K. L., Crook, E. D., Hench, J. L., Leichter, J. J., and Paytan, A. (2016). Assessment of Submarine Groundwater Discharge (SGD) as a source of dissolved radium and nutrients to moorea (French Polynesia) coastal waters. Estuaries Coasts 39, 1651–1668. doi: 10.1007/s12237-016-0108-y
Lambeck, K., Antonioli, F., Anzidei, M., Ferranti, L., Leoni, G., Scicchitano, G., et al. (2011). sea-level change along the Italian coast during the Holocene and projections for the future. Quat. Int. 232, 250–257. doi: 10.1016/j.quaint.2010.04.026
Lim, M., Rosser, N. J., Allison, R. J., and Petley, D. N. (2010). Erosional processes in the hard rock coastal cliffs at Staithes North Yorkshire. Geomorphology 114, 12–21. doi: 10.1016/j.geomorph.2009.02.011
Lo Iacono, C., Gràcia, E., Diez, S., Bozzano, G., Moreno, X., Dañobeitia, J., et al. (2008). Seafloor characterization and backscatter variability of the Almería Margin (Alboran Sea, SW Mediterranean) based on high-resolution acoustic data. Mar. Geol. 250, 1–18. doi: 10.1016/j.margeo.2007.11.004
Locker, S. D., Reed, J. K., Farrington, S., Harter, S., Hine, A. C., and Dunn, S. (2016). Geology and biology of the “Sticky Grounds”, shelf-margin carbonate mounds, and mesophotic ecosystem in the eastern Gulf of Mexico. Cont. Shelf Res. 125, 71–87. doi: 10.1016/j.csr.2016.06.015
Loher, M., Marcon, Y., Pape, T., Römer, M., Wintersteller, P., dos Santos Ferreira, C., et al. (2018). Seafloor sealing, doming, and collapse associated with gas seeps and authigenic carbonate structures at Venere mud volcano, Central Mediterranean. Deep Sea Res. Part I Oceanogr. Res. Pap. 137, 76–96. doi: 10.1016/j.dsr.2018.04.006
Mastrototaro, F., D’Onghia, G., Corriero, G., Matarrese, A., Maiorano, P., Panetta, P., et al. (2010). Biodiversity of the white coral bank off Cape Santa Maria di Leuca (Mediterranean Sea): an update. Deep Sea Res. Part II Top. Stud. Oceanogr. 57, 412–430. doi: 10.1016/j.dsr2.2009.08.021
Matos, L., Wienberg, C., Titschack, J., Schmiedl, G., Frank, N., Abrantes, F., et al. (2017). Coral mound development at the Campeche cold-water coral province, southern Gulf of Mexico: implications of Antarctic intermediate water increased influence during interglacials. Mar. Geol. 392, 53–65. doi: 10.1016/j.margeo.2017.08.012
Micallef, A., Berndt, C., and Debono, G. (2011). Fluid flow systems of the Malta Plateau, Central Mediterranean Sea. Mar. Geol. 284, 74–85. doi: 10.1016/j.margeo.2011.03.009
Micallef, A., Camerlenghi, A., Georgiopoulou, A., Garcia-Castellanos, D., Gutscher, M.-A., Lo Iacono, C., et al. (2019). Geomorphic evolution of the Malta Escarpment and implications for the Messinian evaporative drawdown in the eastern Mediterranean Sea. Geomorphology 327, 264–283. doi: 10.1016/j.geomorph.2018.11.012
Micallef, A., Georgiopoulou, A., Le Bas, T., Mountjoy, J. J., Huvenne, V. A. I., and Lo Iacono, C. (2013). Processes on the precipice: seafloor dynamics across the upper Malta-Sicily escarpment. Rapp. Comm. Int. Mer Méditerr. 40:39.
Mienis, F., de Stigter, H. C., White, M., Duineveld, G., de Haas, H., and van Weering, T. C. E. (2007). Hydrodynamic controls on cold-water coral growth and carbonate-mound development at the SW and SE Rockall Trough Margin, NE Atlantic Ocean. Deep Sea Res. Part I Oceanogr. Res. Pap. 54, 1655–1674. doi: 10.1016/j.dsr.2007.05.013
Miller, K. G., Browning, J. V., Schmelz, W. J., Kopp, R. E., Mountain, G. S., and Wright, J. D. (2020). Cenozoic sea-level and cryospheric evolution from deep-sea geochemical and continental margin records. Sci. Adv. 6:eaaz1346. doi: 10.1126/sciadv.aaz1346
Omoregie, E. O., Niemann, H., Mastalerz, V., de Lange, G. J., Stadnitskaia, A., Mascle, J., et al. (2009). Microbial methane oxidation and sulfate reduction at cold seeps of the deep Eastern Mediterranean Sea. Mar. Geol. 261, 114–127. doi: 10.1016/j.margeo.2009.02.001
Pedley, M. H., House, M. R., and Waugh, B. (1976). The geology of Malta and Gozo. Proc. Geol. Assoc. 87, 325–341. doi: 10.1016/S0016-7878(76)80005-3
Ramos, A., Sanz, J. L., Ramil, F., Agudo, L. M., and Presas-Navarro, C. (2017). “The giant cold-water coral mounds barrier off Mauritania,” in Deep-Sea Ecosystems Off Mauritania, eds A. Ramos, F. Ramil, and J. Sanz (Dordrecht: Springer Netherlands), 481–525. doi: 10.1007/978-94-024-1023-5_13
Reijmer, J. J. G. (2021). Marine carbonate factories: review and update. Sedimentology 68, 1729–1796. doi: 10.1111/sed.12878
Remia, A., and Taviani, M. (2005). Shallow-buried Pleistocene Madrepora-dominated coral mounds on a muddy continental slope, Tuscan Archipelago, NE Tyrrhenian Sea. Facies 50, 419–425. doi: 10.1007/s10347-004-0029-2
Riding, R. (2002). Structure and composition of organic reefs and carbonate mud mounds: concepts and categories. Earth Sci. Rev. 58, 163–231. doi: 10.1016/S0012-8252(01)00089-7
Rogers, A. D. (1999). The biology of Lophelia pertusa and other deep-water reef forming corals and impacts from human activities. Int. Rev. Hydrobiol. 84, 315–406.
Rubin-Blum, M., Shemesh, E., Goodman-Tchernov, B., Coleman, D. F., Ben-Avraham, Z., and Tchernov, D. (2014). Cold seep biogenic carbonate crust in the Levantine basin is inhabited by burrowing Phascolosoma aff. turnerae, a sipunculan worm hosting a distinctive microbiota. Deep Sea Res. Part I Oceanogr. Res. 90, 17–26.
Rüggeberg, A., Dullo, C., Dorschel, B., and Hebbeln, D. (2007). Environmental changes and growth history of a cold-water carbonate mound (Propeller Mound, Porcupine Seabight). Int. J. Earth Sci. 96, 57–72. doi: 10.1007/s00531-005-0504-1
Savini, A., Malinverno, E., Etiope, G., Tessarolo, C., and Corselli, C. (2009). Shallow seep-related seafloor features along the Malta plateau (Sicily channel – Mediterranean Sea): morphologies and geo-environmental control of their distribution. Mar. Pet. Geol. 26, 1831–1848. doi: 10.1016/j.marpetgeo.2009.04.003
Savini, A., Vertino, A., Marchese, F., Beuck, L., and Freiwald, A. (2014). Mapping Cold-water coral habitats at different scales within the Northern Ionian Sea (Central Mediterranean): an assessment of coral coverage and associated vulnerability. PLoS One 9:e87108. doi: 10.1371/journal.pone.0087108
Schembri, P. J., Dimech, M., Camilleri, M., and Page, R. (2007). Living deep-water Lophelia and Madrepora corals in Maltese waters (Strait of Sicily, Mediterranean Sea). Cah. Biol. Mar. 48, 77–83.
Schlager, W. (2005). Carbonate Sedimentology and Sequence Stratigraphy. SEPM Concepts in Sedimentology and Paleontology Series No. 8, Tulsa, OK: SEPM, 208. doi: 10.2110/csp.05.08
Sustainable Energy and Water Conservation Unit (2015). The 2nd Water Catchment Management Plan for the Malta Water Catchment District 2015 - 2021. Valletta: Sustainable Energy and Water Conservation Unit.
Taviani, M., Angeletti, L., Ceregato, A., Foglini, F., Froglia, C., and Trincardi, F. (2013). The Gela Basin pockmark field in the strait of Sicily (Mediterranean Sea): chemosymbiotic faunal and carbonate signatures of postglacial to modern cold seepage. Biogeosciences 10, 4653–4671. doi: 10.5194/bg-10-4653-2013
Taviani, M., Freiwald, A., and Zibrowius, H. (2005a). “Deep coral growth in the Mediterranean Sea: an overview,” in Cold-Water Corals and Ecosystems, eds A. Freiwald and J. M. Roberts (Berlin: Springer-Verlag), 137–156. doi: 10.1007/3-540-27673-4_7
Taviani, M., Remia, A., Corselli, C., Freiwald, A., Malinverno, E., Mastrototaro, F., et al. (2005b). First geo-marine survey of living cold-water Lophelia reefs in the Ionian Sea (Mediterranean basin). Facies 50, 409–417. doi: 10.1007/s10347-004-0039-0
Taviani, M., Vertino, A., Angeletti, L., Montagna, P., and Remia, A. (2019). “Paleoecology of Mediterranean cold-water corals,” in Mediterranean Cold-Water Corals: Past, Present and Future. Coral Reefs of the World, Vol. 9, eds C. Orejas and C. Jiménez (Berlin: Springer), 15–30. doi: 10.1007/978-3-319-91608-8_2
Violante, C., De Lauro, M., and Esposito, E. (2020). “Fine-scale seabed habitats off Capri Island, southern Italy,” in Seafloor Geomorphology as Benthic Habitat, eds P. T. Harris and E. Baker (Amsterdam: Elsevier), 439–450. doi: 10.1016/B978-0-12-814960-7.00024-5
Webster, J. M., Clague, D. A., Riker-Coleman, K., Gallup, C., Braga, J. C., Potts, D., et al. (2004). Drowning of the -150 m reef off Hawaii: a casualty of global meltwater pulse 1A? Geology 32:249. doi: 10.1130/G20170.1
Wheeler, A. J., Beyer, A., Freiwald, A., de Haas, H., Huvenne, V. A. I, Kozachenko, M., et al. (2007). Morphology and environment of cold-water coral carbonate mounds on the NW European margin. Int. J. Earth Sci. 96, 37–56. doi: 10.1007/s00531-006-0130-6
White, M. (2007). Benthic dynamics at the carbonate mound regions of the Porcupine Sea Bight continental margin. Int. J. Earth Sci. 96, 1–9. doi: 10.1007/s00531-006-0099-1
White, M., and Dorschel, B. (2010). The importance of the permanent thermocline to the cold water coral carbonate mound distribution in the NE Atlantic. Earth Planet. Sci. Lett. 296, 395–402. doi: 10.1016/j.epsl.2010.05.025
Wienberg, C. (2019). “A deglacial cold-water coral boom in the Alborán Sea: from coral mounds and species dominance,” in Mediterranean Cold-Water Corals: Past, Present and Future. Coral Reefs of the World, Vol. 9, eds C. Orejas and C. Jiménez (Berlin: Springer), 57–60. doi: 10.1007/978-3-319-91608-8_7
Wienberg, C., and Titschack, J. (2015). “Framework-forming scleractinian cold-water corals through space and time: a late quaternary North Atlantic perspective,” in Marine Animal Forests, eds S. Rossi, L. Bramanti, A. Gori, and C. Orejas Saco del Valle (Cham: Springer International Publishing), 1–34. doi: 10.1007/978-3-319-17001-5_16-1
Wienberg, C., Titschack, J., Freiwald, A., Frank, N., Lundälv, T., Taviani, M., et al. (2018). The giant Mauritanian cold-water coral mound province: oxygen control on coral mound formation. Quat. Sci. Rev. 185, 135–152. doi: 10.1016/j.quascirev.2018.02.012
Keywords: biogenic mounds, reef, natural heritage, coralline algae, trawl marks, seafloor mapping, Malta Plateau
Citation: Bialik OM, Varzi AG, Durán R, Le Bas T, Gauci A, Savini A and Micallef A (2022) Mesophotic Depth Biogenic Accumulations (“Biogenic Mounds”) Offshore the Maltese Islands, Central Mediterranean Sea. Front. Mar. Sci. 9:803687. doi: 10.3389/fmars.2022.803687
Received: 28 October 2021; Accepted: 25 January 2022;
Published: 25 February 2022.
Edited by:
Cristina Gambi, Marche Polytechnic University, ItalyReviewed by:
Giorgio Castellan, Institute of Marine Science (CNR), ItalySalvatore Giacobbe, University of Messina, Italy
Copyright © 2022 Bialik, Varzi, Durán, Le Bas, Gauci, Savini and Micallef. This is an open-access article distributed under the terms of the Creative Commons Attribution License (CC BY). The use, distribution or reproduction in other forums is permitted, provided the original author(s) and the copyright owner(s) are credited and that the original publication in this journal is cited, in accordance with accepted academic practice. No use, distribution or reproduction is permitted which does not comply with these terms.
*Correspondence: Or M. Bialik, b3IuYmlhbGlrQHVtLmVkdS5tdA==; b2JpYWxpa0BjYW1wdXMuaGFpZmEuYWMuaWw=