- 1Institute of Marine Sciences (LABOMAR), Federal University of Ceará (UFC), Fortaleza, Brazil
- 2Reef Systems Group, Leibniz Center for Tropical Marine Research (ZMT), Bremen, Germany
- 3Federal University of Rio Grande (FURG), Rio Grande, Brazil
- 4Brazilian Network on Global Climate Change Research (Rede CLIMA), São José dos Campos, Brazil
- 5Instituto Federal de Educação, Ciência e Tecnologia do Ceará (IFCE), Acaraú, Brazil
- 6Department of Marine Chemistry, Leibniz Institute for Baltic Sea Research, Rostock, Germany
In this article, we discuss knowledge and gaps regarding blue carbon ecosystems (BCEs) in Brazil, considering the urgency to apply protection actions and policies to safeguard their biodiversity and associated ecosystem services. We also indicate areas of further research to improve carbon stocks and sequestration rate estimates. We call attention to the shortage of studies on Brazilian BCEs relative to the growing knowledge on the Blue Carbon Framework accumulated worldwide over the last decade. Considering the extensive Brazilian Economic Exclusive Zone (known as “Blue Amazon”), knowledge concerning blue carbon stocks is vital at regional and global scales for mitigating global increases in atmospheric carbon dioxide (CO2). The Blue Amazon has at least 1,100,000 ha of vegetated and non-vegetated coastal ecosystems (mangroves, salt marshes, seagrass meadows, and hypersaline tidal flats) that collectively contain vast amounts of stored carbon, making Brazil an ideal place to test mechanisms for evaluating, conserving, and restoring BCEs. Other poorly understood potential sinks and sources of carbon are macroalgal and rhodolith beds, mudflats, continental shelf sediments, and marine animal forests in shallow, mesophotic, and deep waters. The carbon fluxes between diverse environmental compartments, such as soil–air, soil–water, groundwater–water–surface water, air–water, and land–ocean, in BCEs across the Blue Amazon must be studied. We emphasize the importance of assessing the total carbon stock and the recent dismantling of environmental laws that pose great risks to these important BCEs. The conservation and recovery of these areas would enhance the carbon sequestration capacity of the entire country. Furthermore, we highlight priorities to improve knowledge concerning BCEs and their biogeochemical cycles in the Blue Amazon and to provide information to assist in the reduction of atmospheric levels of CO2 for the United Nations Decade of Ocean Science (2021–2030).
1 Introduction
Global climate change is perhaps the greatest challenge of modern civilization, and several efforts are being made towards impact adaptation and mitigation of excessive greenhouse gases (Steffen et al., 2018; Bertram et al., 2021). In addition to lowering emissions and decarbonizing the economy, nature-based solutions have been increasingly proposed owing to their low cost, efficiency, and associated co-benefits (McLeod et al., 2011; Gattuso et al., 2018; Taillardart et al., 2018). While terrestrial ecosystems have been the focus of nature-based solutions, the role of coastal and marine ecosystems remains unaccounted for in several national emission inventories and not included in the National Determined Contributions (NDC) (Duarte, 2017). Over the last decade, ocean and terrestrial ecosystems have sequestered approximately 52% of anthropogenic CO2 emissions, with average rates of approximately 2.5 ± 0.6 and 3.4 ± 0.9 GtC year−1, respectively (Friendlingstein et al., 2019). However, some processes and ecosystems, such as coastal areas, are not fully accounted for in the global carbon budget. The CO2 that is captured from the atmosphere and sequestered in coastal and marine environments, mostly vegetated ecosystems such as mangroves, salt marshes, and seagrass meadows, is collectively known as blue carbon (BC) and consists of both organic and inorganic forms (Nellemann et al., 2009).
Coastal ecosystems sequester and stock larger amounts of carbon per unit area than terrestrial ecosystems. For this reason, the conservation and restoration of BC ecosystems (BCEs) are recognized among the key ocean-based activities towards climate change mitigation, adding to global efforts to limit global warming and achieve the goals of the Paris Agreement, with numerous co-benefits (Hoegh-Guldberg et al., 2019). The conservation and restoration of BCEs is crucial for marine CO2 removal (Lezaun, 2021). Therefore, the loss and degradation (e.g., land use changes, trawling activities, changes in water quality, and increasing pollution) of BCEs lead to increases in atmospheric CO2 emissions (Lovelock et al., 2017; O´Connor et al., 2019) and reductions in biosphere C sinks (net C accumulation in the long term) (Kauffmann et al., 2020), ultimately accelerating global climate change.
Mangroves, salt marshes, and seagrasses cover approximately ~36-185 million ha worldwide (Macreadie et al., 2021). However, some important marine ecosystems have not yet met the key criteria for inclusion within the BC framework (e.g., coral and oyster reefs) or show gaps in the scientific understanding of carbon stocks or greenhouse gas fluxes, with limited potential for management or accounting of carbon sequestration rates (e.g., macroalgal beds). Although the scale of greenhouse gas removal or emissions by plankton (Guidi et al., 2016), macroalgae forests (Krause-Jensen et al., 2018), mudflats, and marine animal forests (MAFs) (Rossi et al., 2017; Rossi et al., 2019) may be high, the long-term storage of fixed CO2 is not completely understood for these systems (Lovelock & Duarte, 2019). However, as BC science is multifaceted and has a broad scope, it must explore all potential opportunities for climate change mitigation and adaptation in marine ecosystems, including large and poorly studied tropical regions such as Brazil.
The Blue Amazon is a concept created by the Brazilian Navy to draw attention to the vast Brazilian economic exclusive maritime zone (larger than the Brazilian Amazon) and existing natural resources (including BCEs), which are essential for economy and society (Wiesebron, 2013). However, while the roles of the Amazon Rainforest in biodiversity maintenance, carbon sequestration, and climate regulation are well understood and recognized globally (Gatti et al., 2021), the roles of the Blue Amazon are not. Brazil currently lacks plans to strengthen its climate change and conservation policies and management in this Blue Amazon. Brazil has a vital role in global climate change mitigation, mainly focusing on terrestrial carbon (Gatti et al., 2021). However, when devising climate actions and mitigation strategies, no considerable importance is given to the ocean and coast. Brazil has one of the world’s longest coastlines (between 9th and 15th in rank) and maritime territories (11th in rank); it contains the second largest mangrove forest in a single country and has a high diversity of coastal and marine systems (Lacerda et al., 2019; Magris et al., 2020). However, the potential for carbon storage and sequestration in Brazil is not completely understood (Soares et al., 2017). As a result, Brazilian BCEs are not included in the country’s climate-mitigation commitments (e.g., NDC documents) and adaptation plans.
Therefore, in this perspective article, we aim to discuss the knowledge gaps in BCEs and possible solutions for their protection and restoration in Brazil. First, we present a broad and general characterization of the Brazilian coast and its recognized BCEs, focusing on the distribution and stocks of vegetated coastal systems, such as mangroves, salt marshes, and seagrasses. We discuss the carbon stocks of other important coastal systems [e.g., macroalgal and rhodolith beds, hypersaline tidal flats (HTFs), mudflats, continental shelf, and MAFs] and their potential to be included as BCEs. Next, we discuss the main ongoing impacts and conservation policies that threaten carbon stocks. Finally, we conclude with suggestions for further research to improve carbon estimates, considering the urgency of applying environmental protection actions and policies to safeguard BCE biodiversity and associated ecosystem services, including for climate change mitigation.
2 Blue Carbon Ecosystems in Brazil
Brazil is recognized as a “mega-diverse country” by the United Nations Enviromental Program (UNEP). Brazil´s high biodiversity extends to multiple coastal and marine habitats observed across the long coastline (~9,000 km) and the vast maritime territory (up to 5,700,00 km2), ranging from equatorial (5°N) to warm-temperate latitudes (33°S) (Figure 1). One of the Brazilian sites of global conservation relevance is the Amazon Rainforest. However, the carbon sink of the Amazon Rainforest is declining due to deforestation and climate change, including the recent documentation of the occurrence of carbon sources to the atmosphere through burning in southeastern Amazonia, where deforestation, warming, and moisture stress have been particularly enhanced (Gatti et al., 2021).
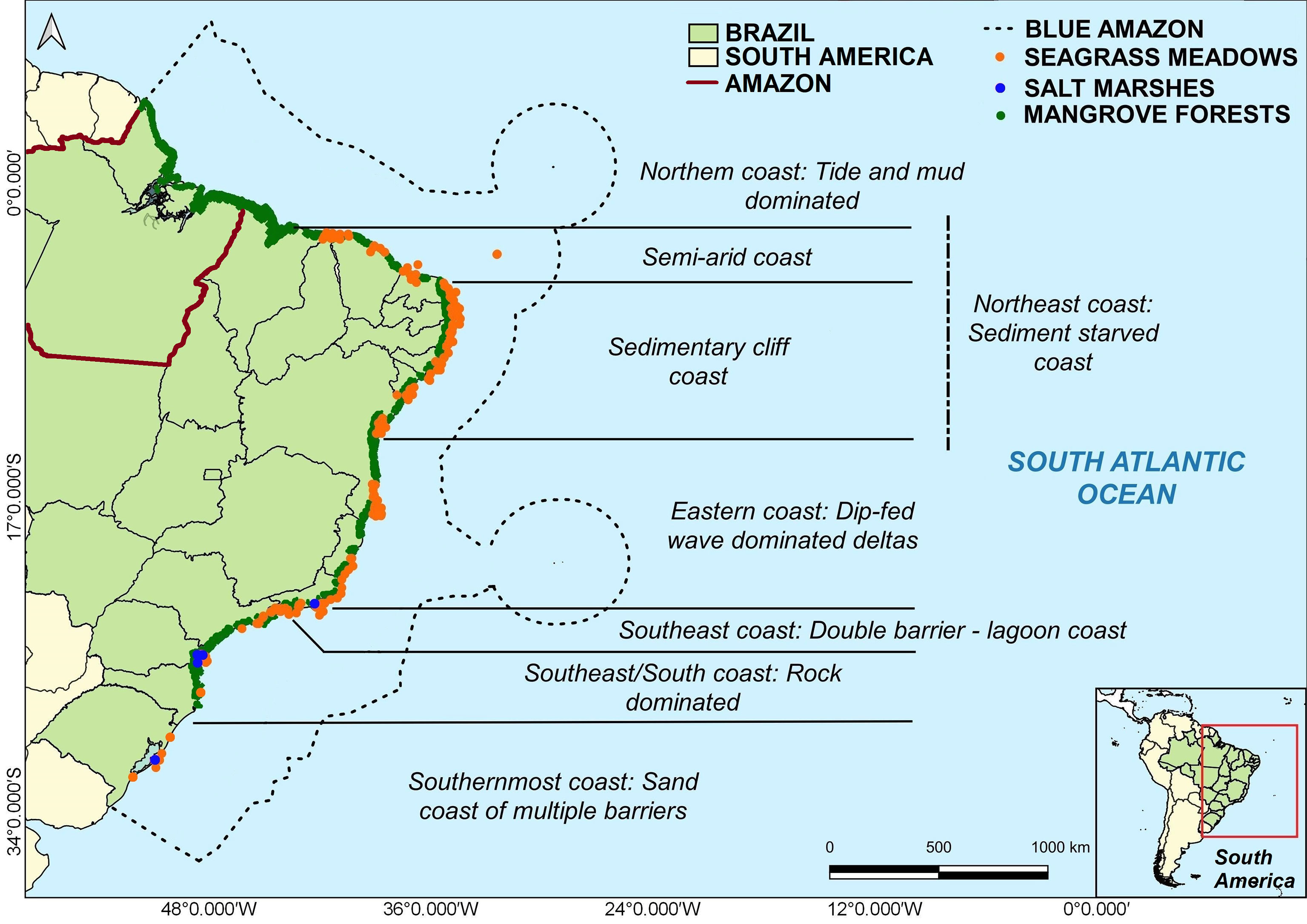
Figure 1 Map showing the spatial distribution of major blue carbon ecosystems (BCEs) in the Brazilian coast. The dashed line presents the limits of the Blue Amazon (Brazilian Economic Exclusive Zone). The orange, blue, and green dots represent the seagrass meadows, salt marshes, and mangrove ecosystems, respectively. Sources: Wiesebron (2013); Copertino et al. (2016); Ferreira and Lacerda (2016); Schaeffer-Novelli et al. (2016), and Lacerda et al. (2019). Coastal geomorphological settlings according to Muehe (2010).
The jurisdictional maritime territory of Brazil, known as the “Blue Amazon” (Figure 1), contains vegetated and non-vegetated coastal and marine ecosystems (mangroves, salt marshes, seagrass meadows, MAFs, and other systems) (Table 1) that could collectively contain a vast amount of stored carbon, making Brazil an ideal place to test new mechanisms for evaluating, conserving, and restoring BCEs (Turra and Denadai, 2016; Bertram et al., 2021).
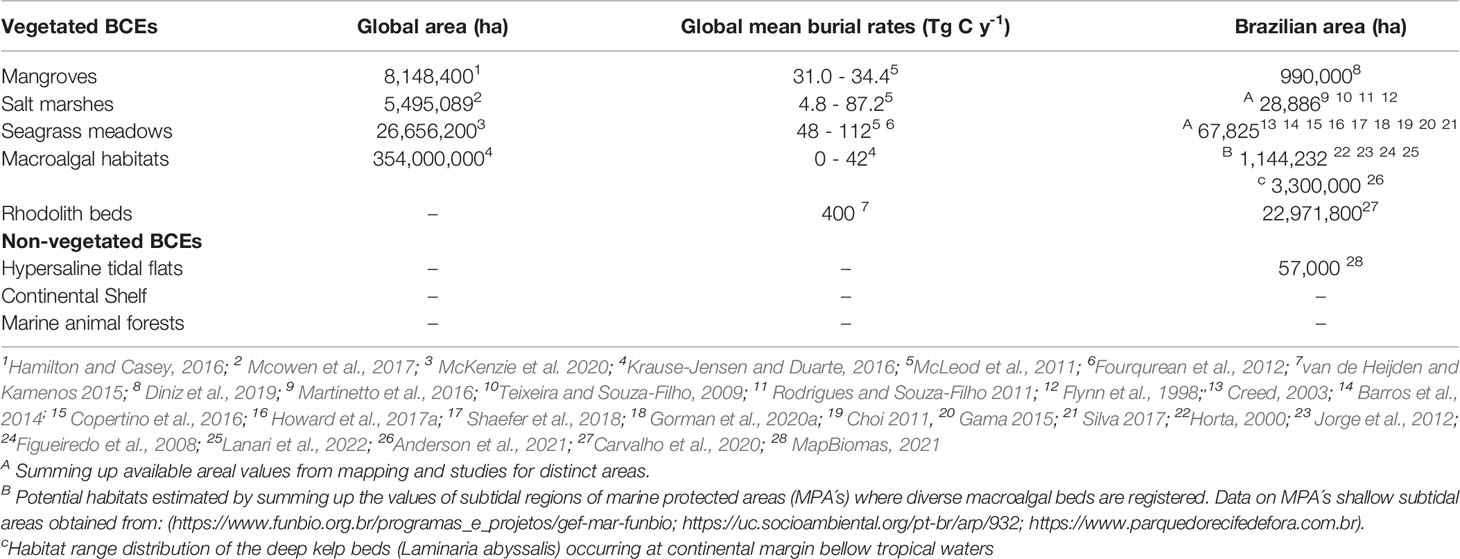
Table 1 Dimensions and carbon burial rates of established and potential BCEs. Estimates for global area, global mean burial rate, and Brazilian ecosystem areas.
The extension of recognized Brazilian BCEs (at least 1,100,000 ha) (Table 1) needs to be better understood, quantified, acknowledged, and protected because of increasing human pressures operating on different spatial scales (local, regional, and global) (Copertino et al., 2016; Horta et al., 2016; Schaeffer-Novelli et al., 2016; Soares et al., 2017; Magris et al., 2020; Soares et al., 2021). In this context, we present a characterization of three actionable BCEs (mangroves, salt marshes, and seagrass meadows) in Brazil (Figure 2).
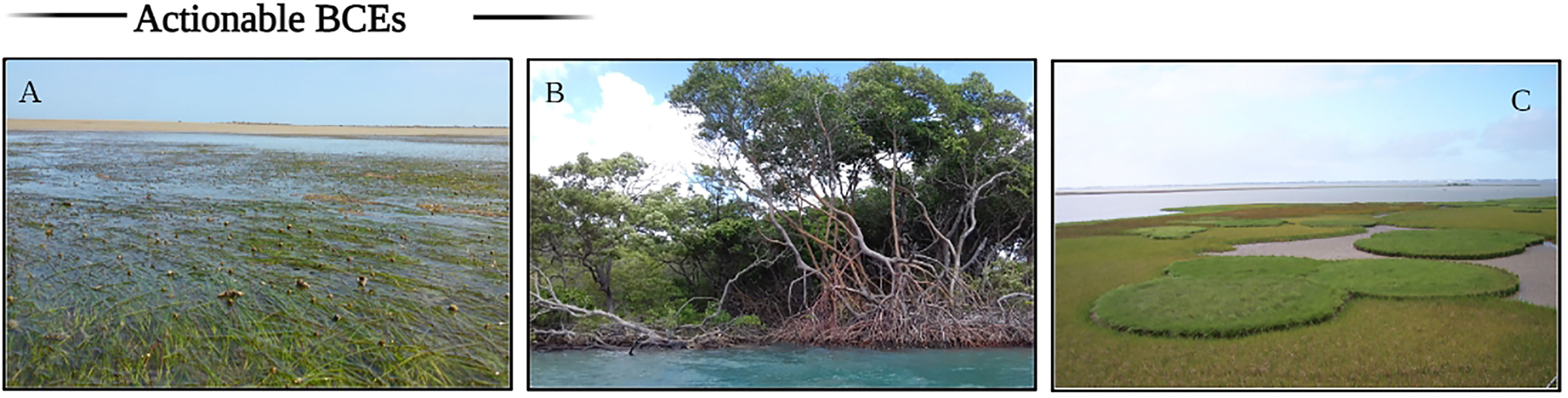
Figure 2 Blue carbon ecosystems in Brazil (South Atlantic). (A) Seagrass meadows - Cajueiro da Praia - Piaui state. Photo: K. Barros; (B) Mangrove forest - Rio Acarau - Ceara state. Photo: R. C. Maia; (C) Salt marshes - Lagoa dos Patos - Rio Grande do Sul state. Photo: C.S.B. Costa.
2.1 Mangrove Forests
Mangroves have a mean total ecosystem carbon stock (TECS) of approximately 738.9 MgC ha−1 (varying widely by site) and a total global mean C stock of 6.17 PgC. Tropical forests, on the other hand, have a mean TECS of approximately 314 MgC ha−1 and a total global mean carbon stock of 553 PgC (Alongi, 2020). Therefore, per plot, carbon stocks in mangrove forests are 2.35-fold those of in tropical rainforests. However, in terms of total carbon stocks, tropical forests have approximately 92-fold higher carbon levels than mangroves (Ahmed and Glaser, 2016; Kauffman et al., 2018a). For this review, we considered a global mangrove area of 8,148,400 ha, in which Brazil has the second largest mangrove area worldwide (Hamilton and Casey, 2016) (Table 1).
The aboveground (AGB) and belowground (BGB) biomass and TECS in Brazilian mangroves (Figure 2B) may present heterogeneity based on geomorphic and sedimentary settings, soil depth, tidal height, salinity, climate, environmental protection measures, and forest structure (Kauffman et al., 2018a; Kauffman et al., 2018b; Nóbrega et al., 2018; Rovai et al., 2018; Kauffmann et al., 2020; Hatje et al., 2020; Matos et al., 2020; Rovai et al., 2020; Rovai et al., 2022) (Table 2). The belowground portion (including soils and roots) represents approximately 70%–86% of the total carbon stocks in mangroves (Hamilton and Friess, 2018; Kauffman et al., 2018a; Kauffmann et al., 2020).
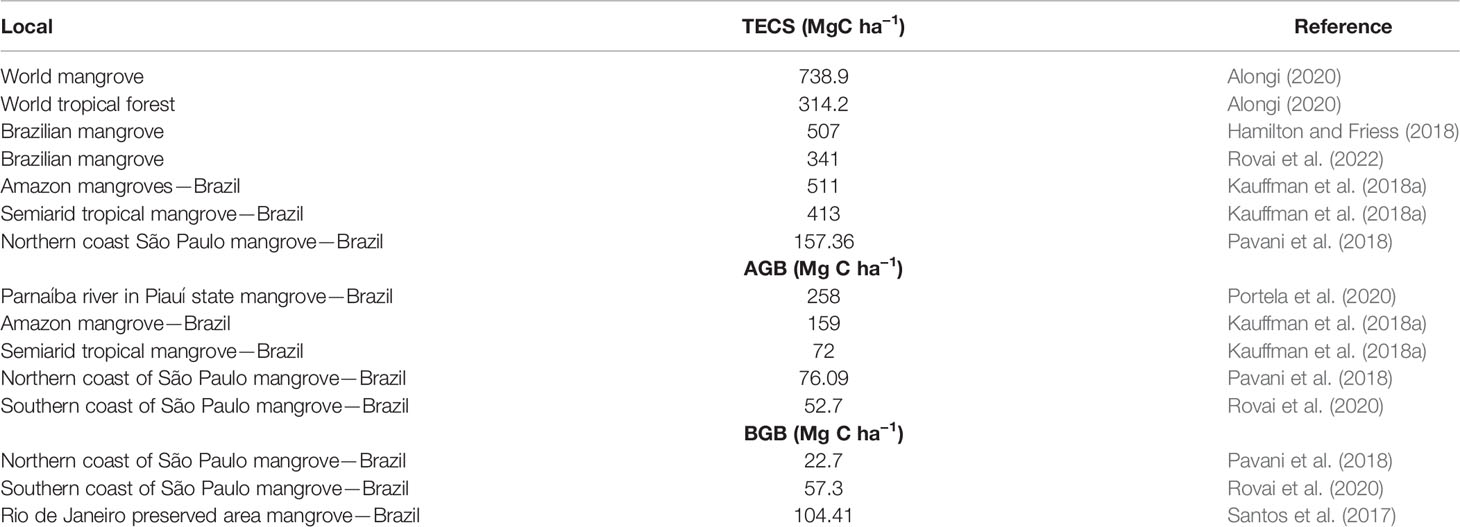
Table 2 Carbon stock in different compartments (TECS, AGB, and BGB) of mangrove and tropical forest ecosystem.
In the last 5 years (2017–2021), mangroves from seven of Brazil’s 15 coastal states have been studied and clarified the necessity of carbon research on the TECS, AGB, and BGB of Brazilian mangroves (Portillo et al., 2016; Kauffman et al., 2018a; Kauffman et al., 2018b; Pavani et al., 2018; Hamilton & Friess, 2018; Ferreira et al., 2019; Nóbrega et al., 2019; Portela et al., 2020; Rovai et al., 2020; Salum et al., 2020). There is also a shortage of information about TECS owing to the low spatial coverage of studies that include AGB and BGB portions simultaneously. Furthermore, the different biophysical typologies and the main drivers of the spatial heterogeneity of mangrove ecosystems along the Brazilian coast (Figure 1) remain poorly described.
Surveys of the TECS registered in Amazon mangroves (511 MgC ha−1) do not significantly differ from the mangroves of semiarid tropical coasts in Brazil [413 MgC ha−1 (Kauffman et al., 2018a) but differ from those of mangroves on the northern coast of São Paulo (157.36 MgC ha−1) (Pavani et al., 2018)]. At the national level, mean TECS varies from 341 MgC ha−1 and 507.83 MgC ha−1 (Hamilton and Friess, 2018; Rovai et al., 2022). Regarding specific mangrove portions, the AGB of the Amazon mangroves (159 MgC ha−1) is greater than that of semi-arid northeast mangroves (72 MgC ha−1) and those of the northern (76.09 MgC ha−1) and southern (52.7 MgC ha−1) coasts of São Paulo, but inferior to that of the Parnaíba River (258 MgC ha−1) (Kauffman et al., 2018a; Pavani et al., 2018; Portela et al., 2020; Rovai et al., 2020), illustrating matrix heterogeneity between sites (Table 2). Furthermore, the BGB portions of mangroves of the northern coast of São Paulo (22.07 MgC ha−1) differ from those of the southern coast (57.3 MgC ha−1) more than the Amazon mean for this compartment (104.41 MgC ha−1) (Kauffman et al., 2018a; Pavani et al., 2018; Rovai et al., 2020). These trends could be due to various factors, such as vegetal composition, hydrologic fluxes (wave exposure and riverine inputs), edaphic factors, anthropogenic influences, and microphytobenthic productivity (Hamilton and Friess, 2018; Rovai et al., 2018; Pavani et al., 2018; Nóbrega et al., 2019; Gorman et al., 2020b). In this regard, a systematic review of BC stocks in Brazilian mangroves is a priority to highlight the importance of sites that have not yet been studied and provide information about AGB and BGB at each site.
The combined carbon stocks of Pará mangroves in the Amazon region are twice those of the upland evergreen forests and almost fivefold those of tropical dry forests (Kauffman et al., 2018a; Kauffman et al., 2018b). Amazon mangroves represent 27.9% of the total mangrove area of the country (Ferreira & Lacerda, 2016). These results demonstrate the global importance of Brazilian mangroves as carbon hotspots (Rovai et al., 2022) (Table 1). Mangroves in North Brazil (Amazon mangroves) are part of the world’s largest continuous belt of mangroves (760,000 ha) (Souza Filho, 2005). In addition, mangroves between 5°N and 5°S represent almost 30% of the world’s mangroves (Giri et al., 2011). However, despite recent scientific efforts, mangroves are not adequately represented in global C budgets because the C cycle in mangrove ecosystems is the result of complex and poorly documented processes (Bouillon et al., 2008), particularly in Brazil. In addition, other greenhouse gases (e.g., methane and nitrous oxide) emitted from mangrove-dominated waters and soils could partially offset CO2 sequestration and organic C burial, especially in degraded/impacted mangroves (Rosentreter et al., 2018; Call et al., 2019).
Most of the research concerning C cycling in mangroves in Brazil and worldwide has focused on soil C stocks and burial rates, rather than on water fluxes. However, the fate of approximately 50% of the carbon fixed by mangrove vegetation is unknown (Bouillon et al., 2008). Studies have shown that mangrove carbon may be exported to the adjacent ocean (Maher et al., 2018; Santos et al., 2018). Thus, the C sequestration capacity of mangroves is likely underestimated.
The outwelling (lateral fluxes or horizontal exports) of dissolved and particulate carbon derived from BCEs has recently been described as a relevant process within the long-term BC sequestration mechanism (Santos et al., 2021). From a global perspective, mangroves account for >10% of terrestrially derived refractory organic carbon exported to the ocean (Dittmar et al., 2006). Mangrove export (outwelling) is the main source of terrigenous organic carbon in the ocean off northern Brazil (Dittmar et al., 2006). Further studies are required on the rates of organic carbon mineralization along the mangrove–estuary–continental shelf continuum to emphasize its importance as a vital atmospheric CO2 sink (Maher et al., 2018). In addition, the lateral export of dissolved inorganic carbon (DIC) and alkalinity can be greater than that of organic C burial as a long-term carbon sink (Maher et al., 2018; Santos et al., 2018). If the exported DIC remains in the water column after air–water equilibration, this carbon outwelling could represent an important and overlooked long-term carbon sink (Santos et al., 2018; Santos et al., 2021). Finally, this C outwelling along the land–ocean aquatic continuum has the potential to locally and/or regionally mitigate the process of ocean acidification by increasing coastal ocean pH (Sippo et al., 2016).
The northern and northeastern continental shelves of Brazil present widespread coral reef coverage (Leão et al., 2016; Soares et al., 2017; Soares et al., 2019) and the outwelling of carbon and alkalinity counteracting coastal acidification could be particularly relevant in shallow-water coral assemblages along the southwestern Atlantic coast (Cotovicz Jr. et al., 2020). However, estimates of carbon outwelling along the land–ocean continuum in Brazil (and elsewhere) are scarce (Cabral et al., 2021) and remain spatially and temporally limited. Therefore, it is important to calculate the outwelling from BCEs to correctly provide the actual sequestration capacities of BCEs, which may currently be underestimated. Future studies should examine the fate of carbon in coastal waters and oceans as long-term C reservoirs. The dissolved inorganic and organic C outwelling (lateral fluxes to the ocean) are potentially greater C sinks than organic C soil burial. Currently, these processes along the Brazilian coast are overlooked and require further investigation.
2.2 Salt Marshes
Salt marshes in Brazil occur along sheltered coastlines maybe associated or not with mangrove forests (Schaeffer-Novelli et al., 2016). Knowledge of salt marsh area extension (Figures 1, 2C), plant species composition, plant biomass standing crop, primary production, and associated surface soil parameters (carbon, organic matter, and grain size) is concentrated in subtropical and warm-temperate Brazilian estuaries and coastal bays (see Davy & Costa, 1992; Isacch et al., 2006; Martinetto et al., 2016; Schaeffer-Novelli et al., 2016 for reviews).
The most extensive salt marsh areas are found in southern Brazil, particularly in Rio Grande do Sul State (Figure 1), covering the margins of coastal lagoons and estuaries, and are dominated by mono-or multi-specific stands of Spartina (S. alterniflora and S. densiflora), Sacoccornia, Scirpus (S. maritimus and S. olney), and Juncus (J. kraussii and J. acutus) (Costa et al., 2003). Large areas of salt and brackish marshes are also associated with tropical mangrove forests (Teixeira & Souza-Filho, 2009; Rodrigues & Souza-Filho, 2011) dominated by Spartina spp., Disticlis spp., Eleocharis spp., and Schoenoplectus spp. (Kauffman et al., 2018a). According to available data on cover extension based on mapping studies (Teixeira & Souza-Filho, 2009; Rodrigues and Souza-Filho 2011; Martinetto et al., 2016), the total Brazilian salt marsh area is approximately 26,300 ha (Table 1). However, this number is underestimated as the total area occupied by tropical salt marshes in the SW Atlantic is unknown.
The total biomass standing crop (from <1 to 30 MgC ha−1) and surface soil organic matter (from <1% to 20%) content of these systems show high variability, depending on species composition, estuarine position, marsh level, terrestrial input, and climate. Most data on soil organic matter or carbon content are limited to surface or shallow core depths (up to 10–20 cm) and lack bulk density data and sedimentation rates. Moreover, data on carbon stocks of Brazilian salt marshes are restricted to two salt marsh areas more than 8,000 km apart that are influenced by distinct climates, tides, fluvial regimes, and community structures. The ecosystem carbon stocks (soil and biomass) observed in Amazonian tropical salt marshes (Pará, Brazil; for the top 1 m to 3 m soil depth) are between 196.7 ± 22.9 and 352.6 ± 29.0 (mean 257.3 Mg C ha−1), which are slightly higher than those used by the IPCC as a referential mean (Kauffman et al., 2018a). The contribution of aboveground biomass to these carbon pools was less than 2%. Higher values have been found for the extensive salt marshes in southern Brazil (Patos Lagoon, Rio Grande do Sul State) (Figure 1), ranging from 263 up to 440 MgC ha−1 (for the top 1 m), depending on the species and marsh level (Patterson et al., 2016). The aboveground biomass of these southern marshes (mean of 26.4 MgC ha−1) may account for 10%–20% of the ecosystem carbon pool. Therefore, further studies on carbon stocks and sequestration in Brazilian salt marshes are needed to provide better support for science-based policies to protect these unique systems.
2.3 Seagrass Meadows
Seagrasses are distributed worldwide, on both temperate and tropical coasts, covering a total area of 26,656,200 ha (McKenzie et al. 2020), up to 164,678,900 ha according to one modeling estimation (Jayathilake and Costello, 2018). There is a notable lack of data on seagrass carbon stocks and sequestration rates from most of the South Atlantic Ocean (Nóbrega et al., 2018; Gorman et al., 2020a), including the Brazilian coastline (Figure 1). Seagrass meadows are suggested to be hotspots for BC storage in soils and may contain at least twice as much carbon per unit area as terrestrial soils (Fourqurean et al., 2012). However, the sedimentary inorganic carbon as carbonate could be close to sedimentary Corg levels, and as the production of carbonates may represent a CO2 source, this can diminish the strength of seagrass sediments as CO2 sinks in some ecosystems, including Brazilian seagrass meadows (Howard et al., 2017; Gullström et al., 2018). Most available data and global reviews concerning seagrass carbon pools are based on the Mediterranean, Australia, and North Atlantic coastlines (Fourqurean et al., 2012; Ricart et al., 2020) and are biased towards large persistent species and dense meadows. Less knowledge is available regarding the stocks and sequestration capacity of small ephemeral species that dominate large extensions of the world´s coasts and estuaries including in Brazil.
Although seagrass meadows have been highlighted as important carbon sinks since the 1980s, they are still neglected in global carbon cycle models and emission inventories, as well as in NDCs, owing to gaps and uncertainties about their stocks, carbonate chemistry, and water–air CO2 fluxes (Duarte, 2017; Macreadie et al., 2014). The area of global seagrass meadows is highly controversial, with a large variability between estimates. Considering the range variability in worldwide seagrass area and the mean C stocks for the top meter of soil (108 Mg C ha−1), the global carbon pool is generally considered to lie between 4.2 and 8.4 Pg (Fourqurean et al., 2012); however, a less conservative approach produces estimates of between 8 and 19.0 Pg. These uncertainties are due to the scarcity of data on most parts of the world’s coasts, including South America. Approximately 50% of the seagrass soil carbon is derived from seagrass plants, with the remainder from other autochthonous and allochthonous sources (plankton, benthic algae, and terrestrial plants; Kennedy et al., 2010). Considering the loss rate of seagrass meadows (global loss rates accelerating from 0.9% year−1 in the 1940s to 7% year−1 towards the end of the 20th century) (Waycott et al., 2009; de los Santos et al., 2019), these systems may release an additional 11–90 Tg C year-1 into the atmosphere (Fourqurean et al., 2012; Pendleton et al., 2012).
Seagrass meadows in Brazil occur discontinuously along the coast (Figures 1, 2), with species distribution and abundance being strongly influenced by regional oceanography, coastal currents, river runoff, and regional and local geomorphology (Copertino et al., 2016). Despite the increasing number of studies on seagrass ecology in Brazil, few have investigated seagrass soil carbon stocks/sequestration or other soil characteristics (Patterson et al., 2016; Howard et al., 2017; Nóbrega et al., 2018; Gorman et al., 2020a; Gorman et al., 2020b). One study comparing seagrass soil across the Brazilian coast (NE semi-arid coast and Southeast/South Brazilian coast) found significant differences according to their morphological, chemical, and physical properties, with distinct total organic carbon (TOC) contents (Nóbrega et al., 2018). Available data show that the percentage of TOC in seagrass soils varies according to latitude, with higher values in the northeast semi-arid coast (Ceará and Piauí states), according to measurements conducted within dense estuarine meadows (2.5% ± 0.9%; Nóbrega et al., 2018).
Seagrass soil C stocks in Halodule spp. meadows measured from several sites across Eastern and Southeastern Brazil range from 0.2 to 1.7 Mg C ha−1 (Howard et al., 2017), with an average (0.67 Mg C/ha) lower than the global mean (1.65 Mg C ha -1 Fourqurean et al., 2012). Data from Northeast Brazil, taken from estuaries, show a higher mean (~2Mg C ha -1) and greater range of values (0.7–4.0 Mg C ha -1) (Nóbrega et al., 2018). The lack of seagrass mapping in most coastal regions hinders the accurate quantification of regional and global seagrass carbon pools. Summing up values from published and unpublished data and few mapped areas, the coastal seagrass area in Brazil covers at least 67,825 ha (Table 1). However, the total area coverage is likely to be greater because many shallow turbid waters and clear deep waters (>10 m) have not been surveyed (Copertino et al., 2016).
Brazilian seagrasses are dominated by small seagrass genera (Halodule, Halophila, and Ruppia) that occur in dynamic patches and have high seasonal and annual variability in their abundance and distribution patterns. While local losses have been reported due to anthropogenic and natural impacts (Copertino et al., 2016), H. wrightii and H. decipiens have expanded the southern limit range distribution (Sordo et al., 2011; Gorman et al., 2020a). Although the distribution of seagrass species along the Brazilian coast is relatively well known, the general lack of mapping and better distribution of soil C stocks for most regions (particularly for the densest meadows thriving along the northeastern region) limit the estimation at the national scale. Roughly, multiplying average C stocks found by Patterson et al. (2016); Howard et al. (2017), and Nóbrega et al. (2018) by the estimated areal extent (Table 1) gives a total soil reservoir ranging between 200 and 3000 Mg C. This wide range is due to the high variability in C densities among the regions and can be considered a hypothesis to be tested.
3 Other Potential Blue Carbon Ecosystems
The concept of BC and its rapidly growing scientific focus on mangroves, salt marshes, and seagrass ecosystems, justified by their large C reservoirs, high sequestration rates, long-term carbon storage, and potential to manage greenhouse gas emissions, support other adaptation policies (Lovelock & Duarte, 2019). In particular, mangroves and salt marshes are now recognized by the International Panel on Climate Change as potential nature-based solutions for atmospheric CO2 reduction (IPCC 2021) and are eligible for inclusion in the greenhouse gas accounting guidance of this panel (IPCC 2014). While these vegetated coastal systems are established BCEs, other relevant systems do not meet key criteria (e.g., coral reefs) or have gaps in scientific knowledge and limited potential for management and accounting (e.g., macroalgal beds and HTFs) (Figure 3).
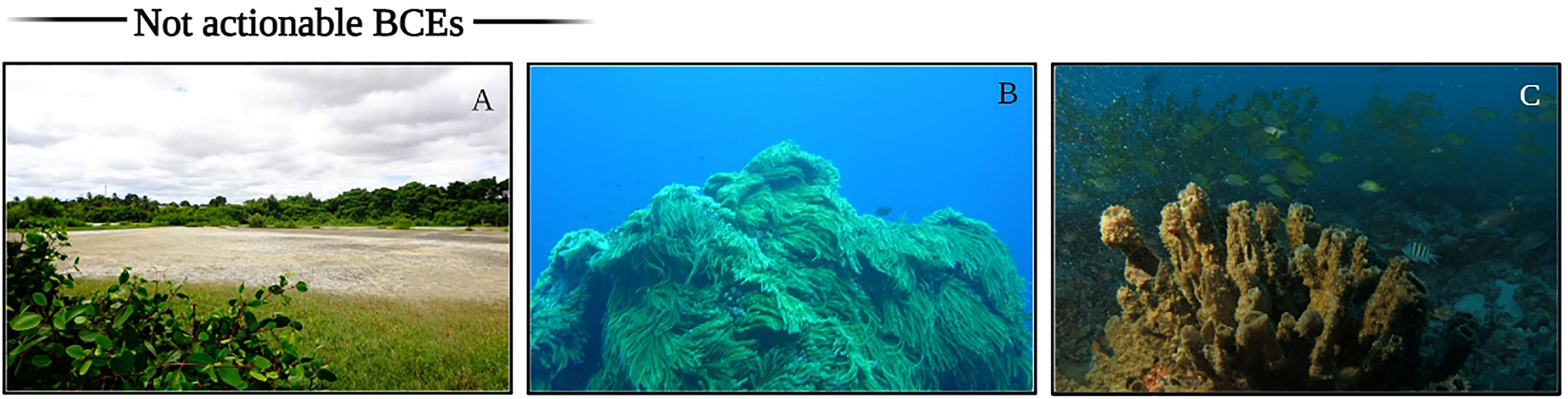
Figure 3 The Blue Amazon (Brazilian Economic Exclusive Zone, South Atlantic) contains potential and non-actionable BC ecosystems that could contain vast amounts of stored carbon. (A) Hypersaline tidal flat (E.g., Barra do Ceara-Ceara state. Photo: N. Beloto, 2020) (B) Macroalgal beds (E.g., Arquipelago de S.Pedro e S.Paulo-Pernambuco state. Photo:Dimas Gianuca, 2004); (C) Marine animal forest (E.g., Parque Estadual Marinho da Pedra da Risca do Meio - Ceara state. Photo: Marcus Davis, 2021).
The Blue Amazon has large extensions of overlooked potential BC sinks, such as macroalgae (Aued et al., 2018), rhodolith beds (Horta et al., 2016), phytoplankton-dominated ecosystems (Guidi et al., 2016), hypersaline tidal flats, mudflats (Gorman et al., 2020b), and marine animal forests (Soares et al., 2017; Barbosa et al., 2019; Soares et al., 2019). In their review, Lovelock and Duarte (2019) framed some of these ecosystems as non-actionable since they do not fulfill key criteria and because of insufficient knowledge about their potential as long-term sinks (Figure 3). If such systems provide important C stocks and sequestration, whether for the ecosystem or beyond, they must be investigated before they can be included in the BC framework. This BC question is long-standing and controversial and requires resolution using empirical evidence (Macredie et al., 2021). In this context, we present other potential BC sinks that should be investigated and protected in the Blue Amazon.
3.1 Hypersaline Tidal Flats (Apicuns)
Hypersaline Tidal Flats (HTF’s) are ecotones associated with mangrove ecosystems on arid and semiarid coasts (Figure 3). Despite carbon sequestration rates being lower in HTFs than in traditional BCEs (described in Section 2), worldwide HTF carbon stocks and long-term carbon sequestration rates can be substantial given their large area (Brown et al., 2021). Together with other vegetated systems, HTFs meet the requirements that define BC systems and can thus be included in global and regional management and mitigation policies (Lovelock & Duarte, 2019).
In Brazil, the HTFs, known as apicuns, occupy large areas in the supratidal zone (57,000 ha; MapBiomas 2021), in an intermediary position between mangroves (or salt marshes) and terrestrial environments (i.e., coastal plains), flooded monthly or with lower frequency (Schaeffer-Novelli et al., 2018; Albuquerque et al., 2014) (Figure 3). Low precipitation and tidal fluxes usually maintain hypersaline conditions (salinity > 100ppt). In Brazil apicuns and mangroves are contiguous ecological systems that present contrasting soils with very different physical, chemical, and mineralogical characteristics (Lacerda et al., 2019). The reduced tidal flooding associated with an evaporative environment and hydric deficit seems to be the primary controlling factor for the formation of these wetlands, which are primarily vegetated by extreme halophytes or devoid of vegetation (Hadlich and Ucha, 2009). Active pedogenetic processes (e.g., salinization, sulfidation, and bioturbation) controlled by the above factors have important ecological roles, specifically regarding carbon and nutrient dynamics (Albuquerque et al., 2014). A sandy mineral apicun soil is deposited over former mangroves.
Mangrove leaves and microphytobenthos have been identified as important sources of organic material accumulated along the sediment columns of these systems. The reported soil carbon content of Brazilian HTFs is lower than 1% (Hadlich et al., 2010; Albuquerque et al., 2014; Brown et al., 2021). Rates of organic carbon burial for a Brazilian HTF (Guaratiba Bay, Rio de Janeiro) during the last century were estimated at 17.8 (± 0.8) g C m−2 y−1 (Brown et al., 2021). These rates are considerably lower than the global averages reported for salt marshes (245 ± 26 g m−2 y−1), mangrove forests (163 ± 40 g m−2 y−1), and seagrasses (138 ± 38 g m−2 y−1). Nevertheless, the importance of HTFs as carbon sinks may be substantial given their extensive coverage along Brazil, particularly on northern and northeast coast (MapBiomas 2021; Soares et al., 2021). HTFs are essential for Brazilian mangrove forests, as they act as reservoirs of nutrients and buffer zones, particularly where mangroves are likely to move inland with sea-level rise due to climate change (Schaeffer-Novelli et al., 2018).
3.2 Macroalgal Beds
Environments dominated by macroalgae occupy a global area of 354,000,000 ha and, considering the high sequestration rates (173 T C year–1), play an essential role in BC budgets (Krause-Jensen and Duarte, 2016). In addition, the burial process of macroalgae-derived organic carbon in shelf sediments and the deep ocean requires that these environments be included in BC estimates (Krause-Jensen et al., 2018), including for the Blue Amazon (Figures 1, 3). While the C stocks of some algal species have been evaluated for some Brazilian regions (e.g., Sargassum; Gouvêa et al., 2020), the contribution of most benthic macroalgae to the C budget and burial is unknown in the SW Atlantic. Nevertheless, it is likely that a large fraction of the organic matter produced by macroalgae is exported and buried in adjacent sediments and deep oceans (Krause-Jensen and Duarte, 2016; Macreadie et al., 2019; Gouvêa et al., 2020).
Macroalgal habitats (mainly algal turfs, frondose macroalgae, rhodolith beds, shallow subtidal sargassum beds, and deep-water kelp beds) occupy the sea bottoms along the Blue Amazon (Aued et al., 2018). Deep-water kelp beds (dominated by the endemic Laminaria abyssalis) are predicted to cover 3,300,000 ha of the Brazilian continental margin, between depths of 20 and 120 m, under cold water temperatures (<15–19°C), and with a high nutrient supply of upwelling regimes (Anderson et al., 2021). Considering L. abyssalis demographic parameters (0.22–2.20 ind/m2) and individual dry weight (54 to 150 g) and the average C content for Laminaria species (30%–33%; King et al., 2020), we can expect average C stocks of up to 0.01 Mg C ha−1. The minimum values of net primary productivity for Laminaria species in the Atlantic range between 100 and 300 g C m-2 year−1 (King et al., 2020). Extrapolating to the area occupied by L. abyssalis in the southwestern Atlantic, it accounts for ecosystem sequestration rates of up to 312 Mg C year−1.
The rhodolith beds of the Blue Amazon span over 22,971,800 ha, equivalent to the area of Australia’s Great Barrier Reef (Horta et al., 2016; Carvalho et al., 2020). The most extensive area of rhodolith beds, covering 2,090,000 ha, is on the Abrolhos Bank (Amado-Filho et al., 2012). Distributed from 0 to ~250 m deep, these beds represent one of the largest carbonate depositional environments and play a fundamental role in the carbon cycle (Horta et al., 2016). The Abrolhos shelf boasts the world’s largest expansion of rhodoliths and is able to produce 1 kg of CaCO3 m-2 year−1, which represents approximately 5% of the CaCO3 burial/precipitation of the world’s carbonate banks (Amado-Filho et al., 2012). Although there is a large distribution and abundance of rhodoliths and seaweeds on the Brazilian coastline (Carvalho et al., 2020), the specific processes of the carbon cycle in these environments have not been completely modeled.
Coralline algae, which form rhodolith beds, may act as a CO2 sink in the processes of photosynthesis and CaCO3 dissolution, and as a CO2 source in the processes of respiration and CaCO3 production (Gattuso et al., 1998), which may offset the C sequestration capacity in the short term. Using a modeling approach, the potential burial rate has been estimated to be 0.4 × 109 Tg C year−1 (Van de Heidjen & Kamenos 2015). There is a need for more comprehensive studies involving the chemistry of coralline algae and refining the models with empirical data before evaluating the role of Brazilian rhodolith beds as true BCEs.
3.3 Plankton-Dominated Coastal Bays and Lagoons
Plankton (bacteria, viruses, phytoplankton, and zooplankton) represent communities in the biological carbon pump because they capture, transform, and export carbon from the upper layers of the ocean and coasts and transfer them for immobilization (Guidi et al., 2016) via benthic suspension feeders (e.g., sponges and octocorals) that compose MAFs (Rossi et al., 2017; Rossi and Rizzo, 2020), such as found across the Amazon Reef System (Soares et al., 2019). Other carbon may be deposited and sequestered through burial in sediments (Macreadie et al., 2019). However, only approximately 0.5% of the carbon fixed in the surface ocean is transferred and deposited on the sea floor.
In this way, higher rates of primary production associated with the eutrophication of Brazilian coastal bays in the tropics can generate large stocks of phytoplankton-derived dissolved and particulate organic carbon, which is permanently being produced, partially degraded, and buried in sediments (Cotovicz Jr. et al., 2018a). For example, the CO2 sink efficiency of Guanabara Bay (Brazil) may be enhanced by eutrophication owing to its location (near the largest city of Rio de Janeiro) and geomorphological characteristics, such as tropical coastal embayment (Cotovicz Jr. et al., 2015). Recent studies conducted in eutrophic Brazilian coastal lagoons have demonstrated the same pattern of atmospheric CO2 absorption and organic carbon storage in sediments (Cotovicz Jr. et al., 2021; Erbas et al., 2021). Large-scale exportation provided by plankton along the tropical and subtropical pelagic marine environments has not been analyzed; however, recent results provided by the Tara Oceans Consortium in the South Atlantic indicate that Radiolaria, alveolate parasites, Synechococcus, copepods, and Rhizaria are drivers of carbon exportation (Guidi et al., 2016) to seafloor sediments and MAFs in the SW Atlantic.
3.4 Marine Animal Forests
Other neglected carbon sinks in the Blue Amazon are MAFs, which capture carbon via benthic-pelagic coupling (Rossi et al., 2017; Rossi and Rizzo, 2020). MAFs are important carbon immobilizers. Various MAFs, including gorgonian forests (Coppari et al., 2019), sponge gardens (Coppari et al., 2016) (Figure 3C), and inactive and passive benthic suspension feeders (Rossi et al., 2017), have been recognized as important systems for considering the BC budget. Ascension Island (South Atlantic, United Kingdom) and the surrounding seamounts in the South Atlantic have shallow MAFs with depths of less than 1,000 m. These MAFs are estimated to capture at least 0.41 MgC ha−1, mainly in the form of deep coral reefs (Barnes et al., 2019). Thus, the Blue Amazon, which has seamounts, sponge gardens, octocoral forests, and an extensive seabed (Soares et al., 2017; Soares et al., 2019), should be considered during the estimation of carbon storage and immobilization in benthic ecosystems and associated sediments. These methodologies, applied to benthic suspension feeder communities (Barnes et al., 2019), mirror similar approaches used in terrestrial forestry studies (Coppari et al., 2016; Coppari et al., 2019) concerning C flux estimation (Rossi and Rizzo, 2020), and could be applied in the Blue Amazon to investigate the overlooked role of MAFs as carbon sinks in the SW Atlantic. However, the total area of the Brazilian MAFs has not been mapped (Table 1).
3.5 Continental Shelf
Perhaps the largest C sink, yet the most uncertain stock, lies within the Brazilian shelf sediments, which may receive a large part of the C exported from continental, coastal, and in situ pelagic biota inputs (e.g., Amazon River plume) (DeMaster et al., 1996; Sobrinho et al., 2021). Shelf sediments cover ~9% of global marine area and may account for more than 90% of stored carbon within some national inventories (Legge et al., 2020). Despite the large carbon pool, these marine areas are currently not protected by international agreements to promote their conservation.
Bottom trawling is likely to be the most widespread human pressure across the seabed globally, disturbing ecosystem functions and resuspending sediments (Rossi et al., 2019). Trawling affects up to 75% of continental shelf sediments globally, with almost 20 million km2 of sediments subject to this pressure (Kaiser et al., 2002). Globally, bottom trawling may release approximately 1.5 billion metric tons of aqueous CO2 annually, which is equal to that released on land through farming (Sala et al., 2021), plus the 22 Gt year−1 of suspended sediments have high direct impacts on biodiversity. The Brazilian economic exclusive zone is a relevant area for carbon storage and must be considered as a BC research priority and conservation target (Soares et al., 2017). Recent studies have estimated that the Amazon River Delta has an accumulated sedimentation rate above the global average. Moreover, the suspended material is transported horizontally by river plume fuel production to adjacent seafloor areas, which improves the relevance of the Amazon continental shelf to the global carbon budget (Sobrinho et al., 2021).
4 Impacts and Protection of the BCEs Sequestration
The extension and high biodiversity of the Blue Amazon provides great potential for carbon storage and sequestration, therefore contributing to climate mitigation and adaptation. However, their regional/global role as carbon sinks still needs to be better understood and recognized. Moreover, human impacts on these marine ecosystems must be avoided and managed because of the local and global relevance of ecosystem goods and services, including climate regulation. Climate change could modify the resistance or resilience of BCEs, potentially making them more sensitive to impacts from activities (e.g., bottom trawling and sewage pollution) and direct pressure may amplify climate change constraints in BCEs (Rossi et al., 2017).
In Brazil, BCEs are threatened by a plethora of human pressures such as coastal urbanization, heatwaves, global warming, oil and gas exploitation, eutrophication, ocean acidification, contamination by agricultural, industrial, and urban effluents, mining accidents, large oil spills, deforestation, and aquaculture activities (Pagliosa et al., 2012; Copertino et al., 2016; Soares et al., 2017; Lacerda et al., 2019; Magris et al., 2020; Soares et al., 2021). For example, deforestation for urbanization, salt exploration, and aquaculture has contributed to the loss of extensive mangrove areas and increased carbon emissions (Pagliosa et al., 2012; Kauffman et al., 2018b). In this way, aquaculture activities, such as shrimp farming, may remove 60% of the soil carbon stock and 85% of the living biomass carbon stock (Sasmito et al., 2020). Shifts in hydrology, tidal exchange, disposal of effluents into mangrove soils, and water changes also have negative effects on BCEs (Macreadie et al., 2017), including in the Blue Amazon (Soares et al., 2017; Magris et al., 2020). Recently, the most extensive oil spill detected in tropical oceans has reached large areas of BCEs, such as mangroves, seagrasses, salt marshes, and rhodolith beds (Magris and Giarrizo, 2020; Soares et al., 2020). For example, Brazilian estuaries (492,974 ha), mangroves (48,983 ha), seagrass meadows (32,477 ha), and tidal flats (6,364 ha) were severely affected by this oil spill along 3,000 km of coastline (Magris and Giarrizo, 2020).
In addition to these local and regional pressures, the predicted impacts of climate change on Blue Amazon ecosystems, such as warming (Magris et al., 2015), ocean and coastal acidification (Cotovicz Jr. et al., 2018b; Perretti et al., 2018), rising sea levels (Godoy and Lacerda, 2015), and droughts (Marengo et al., 2017; Soares et al., 2021), also enhance the risks associated with the degradation of BCEs (Magris et al., 2020) and changes in the coverage of BC areas (e.g., retraction and expansion of mangroves) (Lacerda et al., 2019). Thus, the impacts of climate change on BCEs and their C stocks are dependent on exposure to climate change factors and land use practices (Macreadie et al., 2019; O´Connor et al., 2019). These human activities in the Blue Amazon promote drastic changes in the rates and balance of carbon emissions due to the effects on the storage and sequestration of both the forest structure and burial in the soils (Pagliosa et al., 2012; Nóbrega et al., 2016; Kauffman et al., 2018b; Nóbrega et al., 2019).
Among BCEs, the only environments that have protection measures via legal instruments and coverage by coastal and marine protected areas (MPAs) are mangroves (Santos and Schiavetti, 2014). Other systems that are incorporated in MPAs (e.g., seagrass meadows within the Coral Coast MPA and Abrolhos Marine National Park) are also protected by instruments according to specific local, regional, or national laws. Coastal zones are formally protected by national and international legal provisions. For example, Brazilian mangroves are considered national heritage sites (Constitution of Brazil/1988) and permanent preservation areas (Forest Code/2012). Despite this apparent protection, the existing legal framework is insufficient as it does not require the development of public policies and management plans that ensure effective conservation (Ferreira and Lacerda, 2016). In recent years, the Brazilian federal government has made decisions that weakened and dismantled established environmental policies (Fearnside, 2019), modifying the protection of important areas (e.g., mangroves) and disregarding the inefficient use of natural resources (Bernardino et al., 2021).
Although protected by laws and MPAs, mangroves still suffer from illegal exploitation (Lacerda et al., 2019) and poor management effectiveness (Almeida et al., 2016). Shrimp aquaculture and urban development are likely to be the highest threats to mangrove forests in Brazil (Bernardino et al., 2021). The “Reducing Emissions from Deforestation and forest Degradation (REDD)” program could help to restore mangrove environments; however, technical and financial assistance and institutional support are required to implement REDD+ (Ahmed and Glaser, 2016). In addition to scientific gaps and uncertainties, the Blue Amazon suffers from a lack of political and a government agenda for downgrading already protected ecosystems. Therefore, the implementation of this type of instrument in coastal areas is still far from practical.
South America lost approximately 7.8% and 3.8% of its mangrove coverage between 1980–1990 and 1990–2000, respectively (Lacerda et al., 2019), including losses in Brazil (MAPBIOMAS 2021). In addition, it is important to highlight that Brazil has the third highest country-specific potential annual CO2-equivalent emissions from mangrove soils (Atwood et al., 2017). BCE payments can be implemented in countries with moderate fossil fuel emissions and extensive coastlines, potentially contributing towards climate change mitigation at a national scale (Taillardat et al., 2018). Brazil’s gross emission in 2020 was 2.16 billion tons of carbon dioxide equivalent (Gt CO2; SEEG Brasil, 2021). Discounting carbon removal by secondary forests and protected areas, the national net emissions in the same year were 1.52 Gt CO2. Considering only fossil fuel and cement production (land use change not included), Brazil emitted 467.38 million tons CO2, which represent 1.34% of global emissions (Ritchie and Roser, 2020).
In Brazil, 37.4% of emissions come from the energy sector, followed by the agriculture, land use change and forestry, industrial processes, and waste sectors, which contribute 32.6%, 22.6%, 4.2%, and 3.4% of greenhouse gas emissions, respectively (MCTIC – Ministério da Ciência e Tecnologia do Brasil 2017). In addition, the lack of knowledge regarding reliable and accurate estimates of BC cycling and national budgets is a lost opportunity for economic gains funding from voluntary carbon markets, non-carbon finance, and alternative livelihoods for local communities along the Brazilian coast (Zhao et al., 2020). Carbon credits can be generated for mangroves and coastal wetlands when degraded mangrove habitats are afforested or reforested. This could serve as a strategy to improve the quality of life of citizens and reduce social and economic inequalities using successful global case studies (Taillardat et al., 2018).
Despite the clear societal benefits of carbon sequestration, countries may wish to recognize the benefits in terms of economic value before making decisions about the protection of vulnerable ecosystems, aid policy decisions, widen the market for carbon management and trading (e.g., Nellemann et al., 2009; Luisetti et al., 2019), and include BC protection within national policies (Bertram et al., 2021). Under the increased human and climate pressures predicted for the next decades, damage costs caused by carbon release due to the disturbance of coastal and shelf sea sediments could be very high (e.g., up to US$ 12.5 billion in UK; Luisetti et al., 2019). However, efforts to manage socio-economic pressures to maintain seabed carbon storage need to account for trade-offs with social welfare benefits, such as food security. Therefore, more robust evidence is needed to develop effective incentive mechanisms to preserve these valuable ecosystems within a sustainability governance framework in Brazil.
5 BCEs National Priorities
Surveys on carbon stocks and sequestration in mangroves and other BCEs are fundamental, and defining values and understanding how these ecosystems work in relation to the carbon cycle are essential for climate change compensation projects. This does not mean that surveillance actions should be in the background; however, having observed the political management of Brazil, it is noticeable that there is an inversion of national environmental agencies. Inspection actions are incipient and the budgets for these actions are not being utilized. As a consequence of such environmental liabilities, deforestation and degradation have continued, along with worsening conditions for indigenous people (Scantimburgo, 2018; Fearnside, 2019; Missiatto et al., 2021). The ridge-to-reef concept (Carlson et al., 2019) of ecosystem management can also be applied to Brazilian coastal and marine environments, considering the huge amount of deforestation and pollutants originating from land activities that impact mangroves, seagrass beds, salt marshes, and shallow-water animal forests. Therefore, the priority actions for BCEs, and all terrestrial and marine areas of high importance, are political, especially for climate change mitigation and adaptation.
6 Urgent Recommendations and Future Directions
The current mismanagement of carbon in the Blue Amazon highlights the need for urgent improvements in research, conservation actions, and science-based policies in the United Nations Decade of Ocean Science (2021–2030). We highlight that the knowledge concerning the ecosystem services of the Blue Amazon, such as C stock/sequestration and protection measures, is still incipient and needs to be improved. Importantly, attempts to measure and set targets for carbon sequestration in BCEs should be kept separate from emissions targets in other economic sectors (e.g., transport, industry, and agriculture), to avoid using BC as an offset of other required emission reductions (Climate Analytics 2017). Although it is essential to prevent the degradation of BCEs, carbon sequestration is not the only or most valuable ecosystem service for local populations along the Blue Amazon.
The wide range of potential benefits that emerge from coastal ecosystem conservation and restoration, beyond carbon sequestration, always have sufficient justification for schemes to incentivize their immediate protection (e.g., payment for ecosystem services), with substantial adaptation benefits for local communities, in addition to other ecosystem services. To maximize these benefits, schemes should be designed with the full set of ecosystem services in mind, in addition to carbon sequestration.
Another urgent problem regarding carbon mismanagement in the Blue Amazon is that other BCEs such as seagrass, tidal salt marshes, HTFs, MAFs, and rhodolith beds still have little protection from MPAs or legal provisions that prevent intense economic exploitation (Copertino et al., 2016; Horta et al., 2016; Soares et al., 2017; Magris et al., 2020). Therefore, it is necessary to increase legal protection and MPA coverage in all environments and improve the efficiency of public policies, such as the management effectiveness of mangrove protected areas (Almeida et al., 2016). Moreover, conservation actions that are advantageous for both BC and the structural complexity and diversity of marine species, such as initiatives in terrestrial forests (Silveira et al., 2019), are preferable to carbon-focused initiatives in coastal and marine environments. Thus, it is important to identify and provide effective protection to BC diversity hotspots along the southwestern Atlantic coast.
7 Summary Actions for Researchers and Policymakers
● Promote research that provides information about C stocks, sequestration, and burial rates in Brazilian BCEs and that aims to understand carbon cycling in these environments.
● Reinforce surveillance actions in BCE areas, especially in those with high carbon sequestration and storage potential.
● Change the management of national and state environmental agencies to promote actions in BCEs and their dependent human communities favors.
● Establish goals for carbon sequestration in Brazilian BCEs together with economic sectors, considering carbon credit mechanisms.
● Consider all environmental goods and services provided by such unique environments, as well as carbon sequestration and storage, for the valorization of BCEs.
● Increase the legal protection of BCE areas, including environments that are still poorly measured or valued for their economic and environmental importance, such as seagrasses, HTFs, MAFs, and rhodolith beds.
● Establish the conservation and restoration of BCEs as a national priority for ocean-based carbon dioxide removal (CDR) and adaptation measures.
8 Conclusions
The Blue Amazon is potentially one of the largest carbon sinks on Earth but is largely overlooked. In addition, estimates of quantitative total carbon stocks, burial rates, and atmospheric exchanges of greenhouse gases in Brazilian BCEs are scarce. In this regard, systematic reviews and field/laboratory research on BC stocks in Brazilian coastal and marine ecosystems are a priority topic. It is important to publish future systematic reviews to demonstrate the gaps in current knowledge and original research to provide robust BC budgets of this extensive area.
It is necessary to improve the estimates of carbon stocks, fluxes, protection, and restoration of carbon both above- and belowground in already actionable BCEs (e.g., mangroves and seagrass meadows) and those still not-actionable (e.g., MAFs, HTFs, and macroalgal beds). However, the overall focus of current BC research on the estimations of total stocks and burial rates (habitat-bound carbon) has overlooked the mobile BC fraction, that is, carbon outwelling (lateral fluxes or horizontal exports of C to the ocean; Santos et al., 2021). Therefore, the sequestration capacity of BCEs can be largely underestimated (Santos et al., 2021; Rovai et al., 2022).
Understanding how climate change affects the carbon sequestration capacity in mature BC ecosystems (including Brazil) and during their restoration is a research priority (Macreadie et al., 2019). The role of rhodolith and fleshy macroalgal beds (sinks/donors) in BC cycling and the degree to which greenhouse gases are released following disturbance of BCEs are emerging scientific questions. Finally, we need to improve precision of the extent of BCEs, techniques to determine BC provenance, and understanding of the biotic and abiotic factors that influence sequestration in BCEs.
Author Contributions
MOS: conceptualization, writing—original draft, writing—review and editing, methodology, and formal analysis. LB: writing—original draft, writing—review and editing, and formal analysis. BL: writing—original draft, writing—review and editing, and formal analysis. KB: writing—original draft, writing—review and editing, and formal analysis. MC: writing—original draft, writing—review and editing, and formal analysis. RM: writing—original draft, writing—review and editing, and formal analysis. NB: writing—original draft, writing—review and editing, and formal analysis. LC: writing—original draft, writing—review and editing, and formal analysis. All authors contributed to the article and approved the submitted version.
Funding
This study was sponsored by Brazilian Long Term Ecological Research (Brazilian LTER) at Costa Semiárida do Brasil (PELD-CSB / CNPq Proc. 442337/2020-5), Alexander Von Humboldt Foundation (CAPES/AVH), and Brazilian Research Network on Global Climate Change (Rede CLIMA/ FINEP Grant 01.13.0353-00). MOS and LEAB are CNPq (Grants 313518/2020-3 and 310165/2020-2, respectively) and FUNCAP research fellows (Chief Scientist Program). MC was sponsored by CNPq (Edital Universal Process 433602/2018-0; PELD-ELPA Proc. 403805/2012-0). NB received a PhD scholarship from CAPES. LC receives a visiting professor grant from UFC (FUNCAP, No. INT-00159-00009.01.00/19).
Conflict of Interest
The authors declare that the research was conducted in the absence of any commercial or financial relationships that could be construed as a potential conflict of interest.
Publisher’s Note
All claims expressed in this article are solely those of the authors and do not necessarily represent those of their affiliated organizations, or those of the publisher, the editors and the reviewers. Any product that may be evaluated in this article, or claim that may be made by its manufacturer, is not guaranteed or endorsed by the publisher.
Acknowledgments
The authors thank the editor JP and the three reviewers for their constructive comments and recommendations that improved the manuscript. MC is deeply indebted to The Blue Carbon Initiative and Blue Carbon Scientific Working Group for all intellectual and motivational support and to Conservation International and IOC-UNESCO for international travel awards. This study is a jointed contribution of the Brazilian Research Network on Global Climate Change (Rede CLIMA/ FINEP Grant 01.13.0353-00), Brazilian LTER (PELD-CSB an PELD-ELPA) and Institutional Program for Internationalization (CAPES – PrInt) from Federal University of Ceará and Federal University of Rio Grande.
References
Ahmed N., Glaser M. (2016). Coastal Aquaculture, Mangrove Deforestation and Blue Carbon Emissions: Is REDD+ a Solution? Mar. Pollut. Bull. 66, 56–66. doi: 10.1016/j.marpol.2016.01.011
Albuquerque A. G. B. M., Ferreira T. O., Cabral R. L., Nóbrega G. N., Romero R. E., Meireles A. J. A., et al. (2014). Hypersaline Tidal Flats (Apicum Ecosystems): The Weak Link in the Tropical Wetlands Chain. Environ. Rev. 22, 1–11. doi: 10.1139/er-2013-0026
Almeida L. T., Olímpio J. L., Pantalena A. F., Almeida B. S., Soares M. O. (2016). Evaluating Ten Years of Management Effectiveness in a Mangrove Protected Area. Ocean Coastal Manag. 125, 29–37. doi: 10.1016/j.ocecoaman.2016.03.00810.1016/j.ocecoaman.2016.03.008
Alongi M. D. (2020). Global Significance of Mangrove Blue Carbon in Climate Change Mitigation. Sci 2 (3), 67. doi: 10.3390/sci2030067
Amado-Filho G. M., Moura R. L., Bastos A. C., Salgado L. T., Sumida P. Y., Guth A. Z., et al. (2012). Rhodolith Beds Are Major CaCo3 Bio-Factories in the Tropical South West Atlantic. PloS One 7 (4), e35171. doi: 10.1371/journal.pone.003517110.1371/journal.pone.0035171
Anderson A. B., Assis J., Batista M. B., Serrão E. A., Guabiroba H. C., Delfino S. D. T., et al. (2021). Global Warming Assessment Suggests the Endemic Brazilian Kelp Beds to be an Endangered Ecosystem. Mar. Environ. Res. 168, 105307. doi: 10.1016/j.marenvres.2021.105307
Atwood T. B., Connolly R. M., Almahasheer H., Carnell P. E., Duarte C. M., Lewis C. J. E., et al. (2017). Global Patterns in Mangrove Soil Carbon Stocks and Losses. Nat. Clim. Change 7, 523–528. doi: 10.1038/nclimate3326
Aued A. W., Smith F., Quimbayo J. P., Cândido D. V., Longo G. O., Ferreira C. E. L., et al. (2018). Large-Scale Patterns of Benthic Marine Communities in the Brazilian Province. PloS One 13(6), e0198452. doi: 10.1371/journal.pone.019845210.1371/journal.pone.0198452
Barbosa R. V., Davies A. J., Sumida P. Y. G. (2019). Habitat Suitability and Environmental Niche Comparison of Cold-Water Coral Species Along the Brazilian Continental Margin. Deep-Sea Res. Part I- Oceanographic Res. Papers 154, 103147. doi: 10.1016/j.dsr.2019.10314710.1016/j.dsr.2019.103147
Barnes D. K. A., Sands C. J., Richardson A., Smith N. (2019). Extremes in Benthic Ecosystem Services; Blue Carbon Natural Capital Shallower Than 1000m in Isolated, Small, and Young Ascension Island´s EEZ. Front. Mar. Sci. 6. doi: 10.3389/fmars.2019.0066310.3389/fmars.2019.00663
Barros K., Costa F., Rocha-Barreira C. (2014). A Halophila Baillonis Ascherson Bed on the Semiarid Coast of Brazil. Feddes Repert. 125 (3-4), 93–97.
Barros K. V. S., Rocha-Barreira C. A., Magalhães K. M. (2013). Ecology of Brazilian Seagrasses: Is Our Current Knowledge Sufficient to Make Sound Decisions About Mitigating the Effects of Climate Change? Iheringia Sér. Bot. 68 (1), 163–178. doi: 10.1016/j.marpol.2020.104389
Bernardino A. F., Nóbrega G. N., Ferreira T. O. (2021). Consequences of Terminating Mangroves Protection in Brazil Mar. Policy 125:104389. doi: 10.1590/1678-4766e2017048
Bertram C., Quaas M., Reusch T. B. H., Vafeidis A. T., Wolff C., Rickels W. (2021). The Blue Carbon Wealth of Nations. Nat. Clim. Chang. 11, 704–709. doi: 10.1038/s41558-021-01089-4
Bouillon S., Borges A. V., Castañeda-Moya E., Diele K., Dittmar T., Duke N. C., et al. (2008). Mangrove Production and Carbon Sinks: A Revision of Global Budget Estimates. Glob. Biogeochem. Cy. 22, GB2013. doi: 10.1029/2007GB003052
Brown D. R., Marotta H., Peixoto R. B., Enrich-Prast A., Barroso G. C., Soares M. L., et al. (2021). Hypersaline Tidal Flats as Important “Blue Carbon” Systems: A Case Study From Three Ecosystems. Biogeosciences 18 (8), 2527–2538. doi: 10.5194/bg-18-2527-2021
Cabral A., Dittmar T., Call M., Scholten J., de Rezende C. E., Asp N., et al. (2021). Carbon and Alkalinity Outwelling Across the Groundwater-Creek-Shelf Continuum Off Amazonian Mangroves. Limnol Oceanogr. Lett. 6, 369–378. doi: 10.1002/lol2.10210
Call M., Santos I. R., Dittmar T., Rezende C. E., Asp N. E., Maher D. T. (2019). High Pore-Water Derived CO2 and CH4 Emissions From a Macro-Tidal Mangrove Creek in the Amazon Region. Geochim. Cosmochim. Acta 247, 106–120. doi: 10.1016/j.gca.2018.12.029
Carlson R. R., Foo S. A., Asner G. P. (2019). Land Use Impacts on Coral Reef Health: A Ridge-To-Reef Perspective. Front. Mar. Sci. 6. doi: 10.3389/fmars.2019.00562
Carvalho V. F., Assis J., Serrão E. A., Nunes J. M., Anderson A. B., Batista M. B., et al. (2020). Environmental Drivers of Rhodolith Beds and Epiphytes Community Along the South Western Atlantic Coast. Mar. Environ. Res. 154, 104827. doi: 10.1016/j.marenvres.2019.104827
Climate Analytics (2017) Blue Carbon Briefing: The Dangers of Blue Carbon Offsetts: From Hot Air to Hot Water. Available at: https://climateanalytics.org/media/blue_carbon_briefing_16112017.pdf.
Choi K. F.. (2011). Áreas Prioritárias Para a Conservação Do Peixe-Boi Marinho Trichechus Manatus No Ceará e Rio Grande do Norte (2011). Dissertação (Mestrado em Ciências Marinhas e Tropicais), Instituto de Ciências do Mar, Universidade Federal do Ceará, Fortaleza 246f. Online access: https://repositorio.ufc.br/handle/riufc/14762.
Copertino M. S., Creed J. C., Lanari M. O., Magalhães K., Barros K., Lana P. C., et al. (2016). Seagrass and Submerged Aquatic Vegetation (VAS) Habitats Off the Coast of Brazil: State of Knowledge, Conservation and Main Threats. Braz. J. Oceanogr. 64 (2), 53–80. doi: 10.1590/S1679-875920161036064sp2
Copertino M., Seeliger U. (2010). “Habitats De Ruppia Maritima E De Macroalgas,” in O Estuário Da Lagoa Dos Patos: Um Século De Transformações, Ed. FURG, 92–98. Rio Grande: FURG (Federal Universidade do Rio Grande).
Coppari M., Gori A., Viladrich N., Saponari L., Canepa A., Grinyó J., et al. (2016). The Role of Mediterranean Sponges in Benthic-Pelagic Coupling Processes: Aplysina Aerophoba and Axinella Polypoides Case Studies. J. Exp. Mar. Biol. Ecol. 477, 57–68. doi: 10.1016/j.jembe.2016.01.004
Coppari M., Zanella C., Rossi S. (2019). The Importance of Coastal Gorgonians in the Blue Carbon Budget. Sci. Rep. 9, 13550. doi: 10.1038/s41598-019-49797-410.1038/s41598-019-49797-4
Costa C. S., Marangoni J. C., Azevedo A. M. G. Plant zonation in irregularly flooded salt marshes: relative importance of stress tolerance and biological interactions. J. Ecol., v. 91, p. 951965, 2003
Cotovicz L. C. Jr., Chielle R., Marins R. V. (2020). Air-Sea CO2 Flux in an Equatorial Continental Shelf Dominated by Coral Reefs (Southwestern Atlantic Ocean). Cont. Shelf Res. 204, 104175. doi: 10.1016/j.csr.2020.104175
Cotovicz L. C. Jr., Knoppers B. A., Brandini N., Costa Santos S. J., Abril G. (2015). A Strong CO2 Sink Enhanced by Eutrophication in a Tropical Coastal Embayment (Guanabara Bay, Rio De Janeiro, Brazil). Biogeosciences 12, 6125–6146. doi: 10.5194/bg-12-6125-2015
Cotovicz L. C. Jr., Knoppers B. A., Brandini N., Poirier D., Costa Santos S. J., Abril G. (2018b). Aragonite Saturation State in a Tropical Coastal Embayment Dominated by Phytoplankton Blooms (Guanabara Bay - Brazil). Mar. Pollut. Bull. 129, 729–739. doi: 10.1016/j.marpolbul.2017.10.064
Cotovicz L. C. Jr., Knoppers B. A., Brandini N., Poirier D., Costa Santos S. J., Cordeiro R. C., et al. (2018a). Predominance of Phytoplankton-Derived Dissolved and Particulate Organic Carbon in a Highly Eutrophic Tropical Coastal Embayment (Guanabara Bay, Rio De Janeiro, Brazil). Biogeochemistry 137, 1–14. doi: 10.1007/s10533-017-0405-y
Cotovicz L. C. Jr., Knoppers B. A., Régis C. R., Tremmel D., Costa Santos S. J., Abril G. (2021). Eutrophication Overcoming Carbonate Precipitation in a Tropical Hypersaline Coastal Lagoon Acting as a CO2 Sink (Araruama Lagoon, SE Brazil). Biogeochemistry 156, 231–254. doi: 10.1007/s10533-021-00842-3
Creed J. C. (2003). “The Seagrasses of South America: Brazil, Argentina, and Chile”, in World Atlas of Seagrasses. Eds. Green E. P., Short F. T. (Berkeley: The University California Press), 243–250.
Davy A., Costa C. S. B. (1992). “Development and Organisation of Salt Marsh Communities,” in Coastal Plant Communitites of Latin America. Ed. Seeliger U. (San Diego, USA:Academic Press), 157–178.
de los Santos C. B., Krause-Jensen D., Alcoverro T., Marbà N., Duarte C. M., van Katwijk M. M., et al. (2019). Recent Trend Reversal for Declining European Seagrass Meadows. Nat. Commun. 10, 3356. doi: 10.1038/s41467-019-11340-4
DeMaster D. J., Smith W. O. Jr., Nelson D. M., Aller J. Y. (1996). Biogeochemical Processes in Amazon Shelf Waters: Chemical Distributions and Uptake Rates of Silicon, Carbon and Nitrogen. Cont. Shelf Res. 16 (5-6), 617–643. doi: 10.1016/0278-4343(95)00048-8
Diniz C., Cortinhas L., Nerino G., Rodrigues J., Sadeck L., Adami M., et al. (2019). Brazilian Mangrove Status: Three Decades of Satellite Data Analysis. Remote Sens. 11 (7), 808. doi: 10.3390/rs11070808
Dittmar T., Hertkorn N., Kattner G., Lara R. (2006). Mangroves, a Major Source of Dissolved Organic Carbon to the Oceans. Global Biogeochem. Cy. 20, GB1012. doi: 10.1029/2005GB002570
Duarte C. M. (2017). Reviews and Syntheses: Hidden Forests, the Role of Vegetated Coastal Habitats in the Ocean Carbon Budget. Biogeosciences 14, 301–310. doi: 10.5194/bg-14-301-2017
Duarte C. M., Cebrian J. (1996). The Fate of Marine Autotrophic Production. Limnol. Oceanogr. 41 (8), 1758–1766. doi: 10.4319/lo.1996.41.8.1758
Duarte C. M., Middelburg J. J., Caraco N. (2005). Major Role of Marine Vegetation on the Oceanic Carbon Cycle. Biogeosciences 2 (1), 1–8. doi: 10.5194/bg-2-1-2005
Erbas T., Marques A., Abril G. (2021). A CO2 Sink in a Tropical Coastal Lagoon Impacted by Cultural Eutrophication and Upwelling. Estuar. Coast Shelf Sci. 263, 107633. doi: 10.1016/j.ecss.2021.107633
Fearnside P. M. (2019). “O Desmonte Da Legislação Ambiental”, In Movimentos Socioambientais. Ed. Weiss J. S. (Goiás: Editora Xapuri Socioambiental), 317–381.
Ferreira A. C., Bezerra L. E. A., Matthews-Cascon H. (2019). Aboveground Carbon Stock in a Restored Neotropical Mangrove: Influence of Management and Brachyuran Crab Assemblage. Wetlands Ecol. Manag. 27, 1–20. doi: 10.1007/s11273-019-09654-7
Ferreira A. C., Lacerda L. D. (2016). Degradation and Conservation of Brazilian Mangroves, Status and Perspectives. Ocean Coastal Manag. 125, 38–46. doi: 10.1016/j.ocecoaman.2016.03.011
Figueiredo M. A. O., Horta P. A., Pedrini A. G., Nunes J. M. C. (2008). Benthic Marine Algae of the Coral Reefs of Brazil: A Literature Review. Oecol. Bras. 12 (2), 258–269. doi: 10.4257/oeco.2008.1202.07
Flynn M. N., Wakabara Y., Tararam A. S. (1998). Macrobenthic associations of the lower and upper marshes of a tidal flat colonized by Spartina alterniflora in Cananeia lagoon estuarine region. Bull. Mar. Sci. 63 (2), 427–442.
Fourqurean J. W., Duarte C. M., Kennedy H., Marbà N., Holmer M., Mateo M. A., et al. (2012). Seagrass Ecosystems as a Globally Significant Carbon Stock. Nat. Geosci. 5 (7), 505–509. doi: 10.1038/ngeo1477
Friedlingstein P., Jones M. W., O'Sullivan M., Andrew R. M., Hauck J., Peters G. P., et al. (2019). Global Carbon Budget 2019. Earth Syst. Sci. Data 11 (7), 17831838. doi: 10.5194/essd-11-1783-2019
Gama L. R.. (2015). Ecologia trófica e áreas de alimentação de Chelonia mydas (LINNAEUS, 1758), no litoral do Paraná, Brasil. Dissertação de Mestrado, Universidade Estadual de Londrina, Londrina, 2015
Gatti L. V., Basso L. S., Miller J. B., Gloor M., Domingues L. G., Cassol H. L. G., et al. (2021). Amazonia as a Carbon Source Linked to Deforestation and Climate Change. Nature 595, 388–393. doi: 10.1038/s41586-021-03629-6
Gattuso J.-P., Frankignoulle M., Wollast R. (1998). Carbon and Carbonate Metabolism in Coastal Aquatic Ecosystems. Annu. Rev. Ecol. Syst. 29 (1), 405–434. doi: 10.1146/annurev.ecolsys.29.1.405
Gattuso J.-P., Magnan A. K., Bopp L., Cheung W. W. L., Duarte C. M., Hinkel J., et al. (2018). Ocean Solutions to Address Climate Change and its Effects on Marine Ecosystems. Front. Mar. Sci. 5. doi: 10.3389/fmars.2018.00337
Giri C., Ochieng E., Tieszen L. L., Zhu Z., Singh A., Loveland T., et al. (2011). Status and Distribution of Mangrove Forests of the World Using Earth Observation Satellite Data. Global Ecol. Biogeogr. 20, 154–159. doi: 10.1111/j.1466-8238.2010.00584.x
Godoy M. D. P., Lacerda L. D. (2015). Mangroves Response to Climate Change: A Review of Recent Findings on Mangrove Extension and Distribution. Anais Acad. Bras. Cienc. 87, 651–667. doi: 10.1590/0001-3765201520150055
Gorman D., Pavone C. B., Flores A. A. V. (2020a). Changes to the Structure of Tropical Seagrass Meadows (Halophila Decipiens) in the Warm-Temperate Waters of the Southwest Atlantic. Aquat. Bot. 161, 103174. doi: 10.1016/j.aquabot.2019.103174
Gorman D., Sumida P. Y. G., Figueira R. C. L., Turra A. (2020b). Improving Soil Carbon Estimates of Mudflats in Araçá Bay Using Spatial Models That Consider Riverine Input, Wave Exposure and Biogeochemistry. Estuar. Coastal Shelf Sci. 238(5), 106734. doi: 10.1016/j.ecss.2020.106734
Gouvêa L. P., Assis J., Gurgel C. F. D., Serrão E. A., Silveira T. C. L., Santos R., et al. (2020). Golden Carbon of Sargassum Forests Revealed as an Opportunity for Climate Change Mitigation. Sci. Total Environ. 729(10), 138745. doi: 10.1016/j.scitotenv.2020.138745
Guidi L., Chaffron S., Bittner L., Eveillard D., Larhlimi A., Roux S., et al. (2016). Plankton Networks Driving Carbon Export in the Oligotrophic Ocean. Nature 532, 465–470. doi: 10.1038/nature16942
Gullström M., Lyimo L. D., Dahl M., Samuelsson G. S., Eggertsen M., Anderberg E., et al. (2018). Blue Carbon Storage in Tropical Seagrass Meadows Relates to Carbonate Stock Dynamics, Plant–Sediment Processes, and Landscape Context: Insights From the Western Indian Ocean. Ecosystems 21, 551–566. doi: 10.1007/s10021-017-0170-8
Hadlich G. M., Celino J. J., Ucha J. M. (2010). Physical-Chemical Differentiation Between Supratidal Salt Flats, Mangroves and Hillsides in the Todos Os Santos Bay, Northeast Brazil. Geociências 4 (29), 633–641.
Hadlich G. M., Ucha J. M. (2009). Apicuns: Aspectos Gerais, Evolução Recente E Mudanças Climáticas Globais. Rev. Bras. Geomorf. 2 (10), 13–20. doi: 10.20502/rbg.v10i2.126
Hamilton S. E., Casey D. (2016). Creation of a High Spatio-Temporal Resolution Global Database of Continuous Mangrove Forest Cover for the 21st Century (CGMFC-21). Global Ecol. Biogeogr. 25 (6), 729–738. doi: 10.1111/geb.12449
Hamilton S. E., Friess D. A. (2018). Global Carbon Stocks and Potential Emissions Due to Mangrove Deforestation From 2000 to 2012. Nat. Climate Change 8, 240–244. doi: 10.1038/s41558-018-0090-4
Hatje V., Masqué P., Patire V. F., Dorea A., Barros F. (2020). Blue Carbon Stocks, Accumulation Rates, and Associated Spatial Variability in Brazilian Mangroves. Limnol. Oceanogr. 1 (1), 1–14. doi: 10.1002/lno.11607
Hoegh-Guldberg O., Caldeira K., Chopin T., Gaines S., Haugan P., Hemer M., et al. (2019). The Ocean as a Solution to Climate Change: Five Opportunities for Action (Washington, DC: World Resources Institute).
Hoeinghaus D. J., Vieira J. P., Costa C., Bemvenutti C. E., Winemiller K. O., Garcia A. M.. (2016). Estuary Hydrogeomorphology Affects Carbon Sources Supporting Aquatic Consumers Within and Among Ecological Guilds. Hydrobiologia. 673, 79–92. doi: 10.1007/s10750-011-0751-z
Horta P. A.. (2000). Macroalgas Do Infralitoral Do Sul e Sudeste Do Brasil: Taxonomia e Biogeografia. (2000). Tese de Doutorado, Universidade de São Paulo, São Paulo, 2000.
Horta P. A., Riul P., Amado Filho G. M., Gurgel C. F. D., Berchez F., Nunes J. M. C., et al. (2016). Rhodoliths in Brazil: Current Knowledge and Potential Impacts of Climate Change. Braz. J. Oceanogr. 64 (2), 117–136. doi: 10.1590/S1679-875920160870064sp2
Howard J. L., Creed J. C., Aguiar M. V. P., Fouqurean J. W. (2017a). CO2 Released by Carbonate Sediment Production in Some Coastal Areas may Offset the Benefits of Seagrass “Blue Carbon”. storage Limnol. Oceanogr. 63 (1), 160–172. doi: 10.1002/lno.10621
Howard J., Sutton-Grier A., Herr D., Kleypas J., Landis E., McLeod E., et al. (2017b). Clarifying the Role of Coastal and Marine Systems in Climate Mitigation. Front. Ecol. Environ. 15, 42–50. doi: 10.1002/fee.1451
Howard J. L.. (2018). "Patterns of Carbon Metabolism, Storage, and Remineralization in Seagrass Ecosystems". FIU Electronic Theses and Dissertations. 3719. doi: 10.25148/etd.FIDC004080
Intergovernmental Panel on Climate Change (IPCC) (2014). Supplement to the 2006 IPCC Guidelines for National Greenhouse Gas Inventories (Wetlands. Switzerland: IPCC).
Isacch J. P., Costa C. S. B., Rodríguez-Gallego L., Conde D., Escapa M., Gagliardini D. A., et al. (2006). Distribution of Saltmarsh Plant Communities Associated With Environmental Factors Along a Latitudinal Gradient on the South-West Atlantic Coast. J. Biogeogr. 33 (5), 888–900. doi: 10.1111/j.1365-2699.2006.01461.x
IPCC. (2021). Summary for Policymakers. Climate Change 2021: The Physical Science Basis. Contribution of Working Group I to the Sixth Assessment Report of the Intergovernmental Panel on Climate Change Masson-Delmotte V. P., Zhai A., Pirani S. L., Connors C., Péan S., Berger N., et al. Cambridge University Press.
Jayathilake D. R. M., Costello M. J. (2018). A Modelled Global Distribution of the Seagrass Biome. Biol. Conserv. 226, 120–126. doi: 10.1016/j.biocon.2018.07.009
Jorge R. R., Harari J., Fujii M. T. (2012). Macroalgal Composition and its Association With Local Hydrodynamics in the Laje De Santos Marine State Park, Southwestern Atlantic, São Paulo, Brazil. Braz. J. Oceanogr. Set 2012 60 (3), 405–419. doi: 10.1590/S1679-87592012000300012
Kaiser M. J., Collie J. S., Hall J. S., Jennings S., Poiner I. R. (2002). Modification of Marine Habitats by Trawling Activities: Prognosis and Solutions. Fish Fish 3, 114–136. doi: 10.1046/j.1467-2979.2002.00079.x
Kauffman J. B., Bernardino A. F., Ferreira T. O., Giovannoni L. R., Gomes L. E., Romero D. J., et al. (2018a). Carbon Stocks of Mangroves and Salt Marshes of the Amazon Region, Brazil. Biol. Lett. 14, 20180208. doi: 10.1098/rsbl.2018.0208
Kauffman J. B., Bernardino A. F., Ferreira T. O., Bolton N. W., Gomes L. E. O., Nóbrega G. N. (2018b). Shrimp Ponds Lead to Massive Loss of Soil Carbon and Greenhouse Gas Emissions in Northeastern Brazilian Mangroves. Ecol. Evol. 8, 1–11. doi: 10.1002/ece3.4079
Kauffmann J. B., Adame M. F., Arifanti V. B., Schile-Beers L. M., Bernardino A. F., Bhomia R. K., et al. (2020). Total Ecosystem Carbon Stocks of Mangroves Across Broad Global Environmental and Physical Gradients. Ecol. Monogr. 90 (2), e01405. doi: 10.1002/ecm.1405
Kennedy H., Beggins J., Duarte C. M., Fourqurean J. W., Holmer M., Marbà N., et al. (2010). Seagrass Sediments as a Global Carbon Sink: Isotopic Constraints. Global Biogeochem. Cycles 24 (4), GB4026. doi: 10.1029/2010GB003848
King N. G., Moore P. J., Pessarrodona A., Burrows M., Moore P. J. (2020). Ecological Performance Differs Between Range Centre and Trailing Edge Populations of a Cold-Water Kelp: Implications for Estimating Net Primary Productivity. Mar. Biol. 167, 137. doi: 10.1007/s00227-020-03743-5
Krause-Jensen D., Duarte C. M. (2016). Substantial Role of Macroalgae in Marine Carbon Sequestration. Nat. Geosci. 9 (10), 737–742. doi: 10.1038/ngeo2790
Krause-Jensen D., Lavery P., Serrano O., Marbà N., Masque P., Duarte C. M. (2018). Sequestration of Macroalgal Carbon: The Elephant in the Blue Carbon Room. Biol. Lett. 14 (6), 20180236. doi: 10.1098/rsbl.2018.0236
Lacerda L. D., Borges R., Ferreira A. C. (2019). Neotropical Mangroves: Conservation and Sustainable Use in a Scenario of Global Climate Change. Aquat. Conserv. Mar. Freshwater Ecosyst. 29, 1347–1364. doi: 10.1002/aqc.3119
Lanari M., Copertino M., Horta P.. (2022). Functional redundancy and stability in a subtidal macroalgal community in the Southwestern Atlantic coast. Mar. Environ. Res. 173, 105519. doi: 10.1016/j.marenvres.2021.105519
Leão Z. M. A. N., Kikuchi R. K. P., Ferreira B. P., Sovierzoski H. H., Oliveira M. D. M., Maida M., et al. (2016). Brazilian Coral Reefs in a Period of Global Change: a Synthesis. Braz. J. Oceanogr. 64, 97116. doi: 10.1590/S1679-875920160916064sp2
Legge O., Johnson M., Hicks N., Jickells T., Diesing M., Aldridge J., et al. (2020). Carbon on the Northwest European Shelf: Contemporary Budget and Future Influences. Front. Mar. Sci. 7. doi: 10.3389/fmars.2020.00143
Lezaun J. (2021). Hugging the Shore: Tackling Marine Carbon Dioxide Removal as a Local Governance Problem. Front. Clim. 3. doi: 10.3389/fclim.2021.684063
Lovelock C. E., Atwood T., Baldock J., Duarte C. M., Hickey S., Lavery P. S., et al. (2017). Assessing the Risk of Carbon Dioxide Emissions From Blue Carbon Ecosystems. Front. Ecol. Environ. 15 (5), 257–265. doi: 10.1002/fee.1491
Lovelock C. E., Duarte C. M. (2019). Dimensions of Blue Carbon and Emerging Perspectives. Biol. Lett. 15 (3), 20180781. doi: 10.1098/rsbl.2018.0781
Luisetti T., Turner R. K., Andrews J. E., Jickells T. D., Kröger S., Diesing M., et al. (2019). Quantifying and Valuing Carbon Flows and Stores in Coastal and Shelf Ecosystems in the UK. Ecosyst. Serv. 35, 67–76. doi: 10.1016/j.ecoser.2018.10.013
Macreadie P. I., Anton A., Raven J. A., Beaumont N., Connolly R. M., Friess D. A., et al. (2019). The Future of Blue Carbon Science. Nat. Commun. 10, 3998. doi: 10.1038/s41467-019-11693-w
Macreadie P. I., Baird M. E., Trevathan-Tackett S. M., Larkum A. W. D., Ralph P. J.. (2014). Quantifying and Modelling the Carbon Sequestration Capacity of Seagrass Meadows a Critical Appraisal? Mar Pollut Bull. 83, 430–439. doi: 10.1016/j.marpolbul.2013.07.038
Macreadie P. I., Nielsen D. A., Kelleway J. J., Atwood T. B., Seymour J. R., Petrou K., et al. (2017). Can We Manage Coastal Ecosystems to Sequester More Blue Carbon? Front. Ecol. Environ. 15 (4), 206–213. doi: 10.1002/fee.1484
Magalhães K. M., Barros K. V. S., Lima M. C. S., Rocha-Barreira C. A., Rosa Filho J., Soares M. O. (2020). Oil Spill + COVID-19: A Disastrous Year for Brazilian Seagrass Conservation. Sci. Total Environ. 764, 142872. doi: 10.1016/j.scitotenv.2020.142872
Magris R. A., Costa M. D. P., Ferreira C. E. L., Vilar C. C., Joyeux J.-C., Creed J. C, et al. (2020). A Blueprint for Securing Brazil´s Marine Biodiversity and Supporting the Achievement of Global Conservation Goals. Diversity Distrib 27(2), 198–215. doi: 10.1111/ddi.13183
Magris R. A., Giarrizo T. (2020). Mysterious Oil Spill in the Atlantic Ocean Threatens Marine Biodiversity and Local People in Brazil. Mar. Pollut. Bull. 153, 110961. doi: 10.1016/j.marpolbul.2020.11096110.1016/j.marpolbul.2020.110961
Magris R. A., Heron S. F., Pressey R. L. (2015). Conservation Planning for Coral Reefs Accounting for Climate Warming Disturbances. PLoS ONE 10(11), e0140828 . doi: 10.1371/journal.pone.0140828
Maher D. T., Call M., Santos I. R., Sanders C. (2018). Beyond Burial: Lateral Exchange is a Significant Atmospheric Carbon Sink in Mangrove Forests. Biol. Lett. 14, 20180200. doi: 10.1098/rsbl.2018.0200
MAPBIOMAS (2021) Manguezais, Praias, Dunas & Areais, Aquicultura E Apicuns Nos Últimos 36 Anos. Destaques do Mapeamento Anual Dos Manguezais, Praias, Dunas, Areais, Aquicultura E Apicuns No Brasil Entre 1985 a 2020. MapBiomas Coleção 6. Available at: https://mapbiomas-br-site.s3.amazonaws.com/MapBiomas_Zona_Costeira_Outubro_2021_30102021_OK.pdf (Accessed January 25, 2022).
Marengo J. A., Torres R. R., Alves L. M. (2017). Drought in Northeast Brazil – Past, Present, and Future. Theor. Appl. Climatol. 129, 1189–1200. doi: 10.1007/s00704-016-1840-8
Martinetto P., Montemayor D. I., Alberti J., Costa C. S. B., Iribarne O. (2016). Crab Bioturbation and Herbivory May Account for Variability in Carbon Sequestration and Stocks in South West Atlantic Salt Marshes. Front. Mar. Sci. 3(122), 1–12. doi: 10.3389/fmars.2016.00122
Matos C. R. L., Berrêdo J. F., Machado W., Sanders C. J., Metzger E., Cohen M. C. L. (2020). Carbon and Nutrient Accumulation in Tropical Mangrove Creeks, Amazon Region. Mar. Geol. 429, 106317. doi: 10.1016/j.margeo.2020.106317
McKenzie L. J., Nordlund L. N., Jones B. L., et al (2020). The Global Distribution of Seagrass Meadows. Environ. Res. Lett 15(7), 1–13. 074041. doi: 10.1088/1748-9326/ab7d06
McLeod E., Chmura G. L., Bouillon S., Salm R., Björk M., Duarte C. M., et al. (2011). A Blueprint for Blue Carbon: Toward an Improved Understanding of the Role of Vegetated Coastal Habitats in Sequestering CO2. Front. Ecol. Environ. 9(10), 552–560. doi: 10.1890/110004
Mcowen C., Weatherdon L., Bochove J., Sullivan E., Blyth S., Zockler C., et al. (2017). A Global Map of Saltmarshes. Biodivers. Data J. 5, e11764. doi: 10.3897/BDJ.5.e11764
MCTIC – Ministério da Ciência e Tecnologia do Brasil (2017) Estimativas Anuais Da Emissão Dos Gases De Efeito Estufa. Available at: http://educaclima.mma.gov.br/wp-content/uploads/2019/08/Estimativas-Anuais-4-2017.pdf.
Missiatto L. A. F., Silva L. G., Carvalho F. R., Denes D. M., Missiatto H. M. (2021). A Colonialidade Nas Políticas Ambientais do Governo Bolsonaro E a Inversão Dos Órgãos De Defesa do Meio Ambiente. Rev. Interdisciplinar. Margens - Dossiê Margens Poder Insurgência América Latina 15 (24), 85–102. doi: 10.18542/rmi.v15i24.10049
Muehe D.. (2010). Brazilian coastal vulnerability to climate change. Panam. J. Aquat Sci. 5(2), 173–183.
Nellemann C., Corcoran E., Duarte C. M., Valdés L., De Young C., Fonseca L., et al. (2009). Blue Carbon. A Rapid Response Assessmentt. GRID-Arendal: United Nations Environment Programme, GRID-Arendal (Norway: Birkeland Trykkeri AS).
Nóbrega G. N., Ferreira T. O., Neto M. S., Mendonça E. S., Romero R. E., Otero X. L. (2019). The Importance of Blue Carbon Soils in Tropical Semiarid Mangroves: A Case Study in Northeastern Brazil. Environ. Earth Sci. 78, 369. doi: 10.1007/s12665-019-8368-z
Nóbrega G. N., Ferreira T. O., Neto M. S., Queiroz H. M., Artur A. G., Mendonça E. S., et al. (2016). Edaphic Factors Controlling Summer (Rainy Season) Greenhouse Emissions (CO2 and CH4) From Semiarid Mangrove Soils (NE-Brazil). Sci. Total Environ. 542, A, 685–A, 693. doi: 10.1016/j.scitotenv.2015.10.108
Nóbrega G. N., Romero D. J., Otero X. L., Ferreira T. O. (2018). Pedological Studies of Subaqueous Soils as a Contribution to the Protection of Seagrass Meadows in Brazil. Rev. Bras. Cienc. Solo 42, 1–12. doi: 10.1590/18069657rbcs20170117
O´Connor J. J., Fest B. J., Sievers M., Swearer S. E. (2019). Impacts of Land Management Practices on Blue Carbon Stocks and Greenhouse Gas Fluxes in Coastal Ecosystems – A Meta-Analysis. Global Change Biol. 26 (3), 1354–1366. doi: 10.1111/gcb.14946
Pagliosa P. R., Rovai A. S., Fonseca A. L. (2012). Carbon Mismanagement in Brazil. Nat. Climate Change 2, 764. doi: 10.1038/nclimate1718
Patterson E., Johnson B., Dostie P., Copertino M. (2016). Stocks and Sources of Carbon Buried in the Salt Marshes and Seagrass Beds of Patos Lagoon Estuary, Southern Brazil. Geophys. Res. Abstr. 18, EGU2016–10792.
Pavani B. F., Sousa Júnior W. C., Inouye C. E. N., Vieira S. A., Mello A. Y. I. (2018). Estimating and Valuing the Carbon Release in Scenarios of Land-Use and Climate Changes in a Brazilian Coastal Area. J. Environ. Manag. 226, 416–427. doi: 10.1016/j.jenvman.2018.08.059
Pendleton L., Donato D. C., Murray B. C., Crooks S., Jenkins W. A., Sifleet S., et al. (2012). Estimating Global “Blue Carbon” Emissions From Conversion and Degradation of Vegetated Coastal Ecosystems. PloS One 7 (9), e43542. doi: 10.1371/journal.pone.0043542
Perretti A. R., Albergaria-Barbosa A. C. R., Kerr R., Cunha L. C. (2018). Ocean Acidification Studies and the Uncertainties Relevance on Measurements of Marine Carbonate System Properties. Braz. J. Oceanogr. 66 (2), 234–242. doi: 10.1590/s1679-87592018000706602
Portela M. G. T., de Espindola G. M., Valladares G. S., Amorim J. V. A., Frota J. C. O (2020). Vegetation Biomass and Carbon Stocks in the Parnaíba River Delta, NE Brazil. Wetlands Ecol. Manag 28, 607–622. doi: 10.1007/s11273-020-09735-y
Portillo J. T. M., Londe V., Moreira F. W. A. (2016). Aboveground Biomass and Carbon Stock are Related With Soil Humidity in a Mangrove at the Piraquê-Açu River, Southeastern Brazil. J. Coastal Conserv. 21 (1), 139–144. doi: 10.1007/s11852-016-0482-4
Ricart A. M., York P. H., Bryant C. V., Rasheed M. A., Ierodiaconou D., Macreadie P. I. (2020). High Variability of Blue Carbon Storage in Seagrass Meadows at the Estuary Scale. Sci. Rep. 10 (5865), 1–12. doi: 10.1038/s41598-020-62639-y
Ritchie H., Roser M. (2020) CO₂ and Greenhouse Gas Emissions. Available at: https://ourworldindata.org/co2-and-other-greenhouse-gas-emissions.
Rodrigues S., Souza-Filho P. W. M. (2011). Use of Multi-Sensor Data to Identify and Map Tropical Coastal Wetlands in the Amazon of Northern Brazil. Wetlands 31(1), 11–23. doi: 10.1007/s13157-010-0135-6
Rosentreter J. A., Maher D. T., Erler D. V., Murray R. H., Eyre B. D. (2018). Methane Emissions Partially Offset “Blue Carbon” Burial in Mangroves. Sci. Adv. 4, 6. doi: 10.1126/sciadv.aao4985
Rossi S., Coppari M., Viladrich N. (2017). ““Benthic-Pelagic Coupling: New Perspectives in the Marine Animal Forests”,” in Perspectives on the Marine Animal Forests. Eds. Rossi S., Bramanti L., Gori A., Orejas C. (Cham: Springer), 855–885. doi: 10.1007/978-3-319-21012-4_23
Rossi S., Isla E., Bosch-Belmar M., Galli G., Gori A., Gristina M., et al. (2019). Changes of Energy Fluxes in Marine Animal Forests of the Anthropocene: Factors Shaping the Future Seascape. ICES J. Mar. Sci. 76 (7), 2008–2019. doi: 10.1093/icesjms/fsz147
Rossi S., Rizzo L. (2020). ““Marine Animal Forests as C Immobilizers”,” in Perspectives on the Marine Animal Forest. Eds. Rossi S., Bramanti L., Gori A., Orejas C. (Cham: Springer), 333–400. doi: 10.1007/978-3-030-57054-5_11
Rovai A. S., Coelho-Jr C., de Almeida R., Cunha-Lignon M., Menghini R. P., Twilley R. R., et al. (2020). Ecosystem-Level Carbon Stocks and Sequestration Rates in Mangroves in the Cananéia-Iguape Lagoon Estuarine System, Southeastern Brazil. For. Ecol. Manag. 479, 118553. doi: 10.1016/j.foreco.2020.118553
Rovai A. S., Twilley R. R., Castãneda-Moya E., Riul P., Cifuentes-Jara M., Manrow-Villalobos M., et al. (2018). Global Controls on Carbon Storage in Mangrove Soils. Nat. Climate Change 8, 534–538. doi: 10.1038/s41558-018-0162-5
Rovai A. S., Twilley R. R., Worthington T. A., Riul P. (2022). Brazilian Mangroves: Blue Carbon Hotspots of National and Global Relevance to Natural Climate Solutions. Front. Collect. 4 (787533). doi: 10.3389/ffgc.2021.787533
Sala E., Mayorga J., Bradley D., Cabral R. B., Atwood T. B., Auber A., et al. (2021). Protecting the Global Ocean for Biodiversity, Food and Climate. Nature 592, 397–402. doi: 10.1038/s41586-021-03371-z
Salum R. B., Souza-Filho P. W. M., Simard M., Silva C. A., Fernandes M. E. B., Cougo M. F., et al. (2020). Improving Mangrove Above-Ground Biomass Estimates Using LiDAR. Estuar. Coastal Shelf Sci. 236, 106585. doi: 10.1016/j.ecss.2020.106585
Santos D. M. C., Estrada G. C. D., Fernandez V., Estevam M. R. M., Souza B. T. D., Soares M. L. G.. (2017). First Assessment of Carbon Stock in the Belowground Biomass of Brazilian Mangroves. Anais Da Academia Brasileira de Ciências 89 (3), 15791589. doi: 10.1590/0001-3765201720160496
Santos I. R., Burdige D. J., Jennerjahn T. C., Bouillon S., Cabral A., Serrano O., et al. (2021). The Renaissance of Odum’s Outwelling Hypothesis in ‘Blue Carbon’ Science. Estuar. Coastal Shelf Sci. 255, 107361. doi: 10.1016/j.ecss.2021.107361
Santos I. R., Maher D. T., Larkin R., Webb J., Sanders C. J. (2018). Carbon Outwelling and Outgassing vs. Burial in an Estuarine Tidal Creek Surrounded by Mangrove and Saltmarsh Wetlands. Limnol. Oceangr. Lett. 2, 1–18. doi: 10.1002/lno.11090
Santos C. Z., Schiavetti A. (2014). Spatial Analysis of Protected Areas of the Coastal/Marine Environment of Brazil. J. Nat. Conserv. 22 (5), 453–461. doi: 10.1016/j.jnc.2014.05.001
Sasmito S. D., Sillanpaa M., Hayes M. A., Bachri S., Saragi-Sasmito M. F., Sidik F., et al. (2020). Mangrove Blue Carbon Stocks and Dynamics are Controlled by Hydrogeomorphic Settings and Land-Use Change. Global Change Biol 26(5), 3028–3039. doi: 10.1111/gcb.15056
Scantimburgo A. (2018). O Desmonte Da Agenda Ambiental No Governo Bolsonaro. Perspectivas 52, 103–117.
Schaeffer-Novelli Y., Cintrón-Molero G., Reis-Neto A. S., Abuchahla G. M. O., Neta L. C. P., Lira-Medeiros C. F. (2018). The Mangroves of Araçá Bay through time: An interdisciplinary Approach for Conservation of Spatial Diversity at Large Scale. Ocean & Coastal Management 164, 60–67. doi: 10.1016/j.ocecoaman.2017.12.024
Schaeffer-Novelli Y., Soriano-Sierra E. J., Vale C. C., Bernini E., Rovai A. S., Pinheiro M. A. A., et al. (2016). Climate Changes in Mangrove Forests and Salt Marshes. Braz. Journ. Ocean. 64 (sp2), 83–98. doi: 10.1590/S1679-875920160919064sp2
SEEG–Brasil. (2016). Going against the world, Brazil increased emissions in the middle of the pandemic. https://seeg.eco.br/en/press-release
Shaefer C. E. G. R., Simas F. N. B., Albuquerque M. A., Souza E., Delpupo K. K. (2010). Fosfatização de solos e evolução da paisagem no arquipélago de Abrolhos, BA. Rem-Rev. Esc. Minas. 63(4), 727–34. doi: 10.1590/S0370-44672010000400019
Silva G. C. M. (2017). Mapeamento de Bancos de Algas e Fanerógamas na área de Proteção Ambiental Dos Recifes de Corais: RN utilizando geotecnologias. Tese de Doutorado em Desenvolvimento e Meio Ambiente, Centro de Biociências Universidade Federal do Rio Grande do Norte, Natal, 112 f. Online access: https://repositorio.ufrn.br/jspui/handle/123456789/24520
Silveira E. M. O., Terra M. C. N. S., Steege H. T., Maeda E. E., Júnior F. W. A., Scolforo J. R. S. (2019). Carbon-Diversity Hotspots and Their Owners in Brazilian Southeastern Savanna, Atlantic Forest and Semi-Arid Woodland Domains. For. Ecol. Manag. 452, 117575. doi: 10.1016/j.foreco.2019.117575
Sippo J. Z., Maher D. T., Tait D. R., Holloway C., Santos I. R. (2016). Are Mangroves Drivers or Buffers of Coastal Acidification? Insights From Alkalinity and Dissolved Inorganic Carbon Export Estimates Across a Latitudinal Transect. Glob. Biogeochem. Cycles 30, 753–766. doi: 10.1002/2015GB005324
Soares M. O., Campos C. C., Carneiro P. B. M., Barroso H. S., Marins R. V., Teixeira C. E. P., et al. (2021). Challenges and Perspectives for the Brazilian Semi-Arid Coast Under Global Environmental Changes. Perspect. Ecol. Conserv. 19 (3), 267–278. doi: 10.1016/j.pecon.2021.06.001
Soares M. O., Lotufo T. M. C., Vieira L. M., Salani S., Hajdu E., Matthews-Cascon H., et al. (2017). “Brazilian Marine Animal Forests: A New World to Discover in the Southwestern Atlantic,” in Marine Animal Forests. Eds. Rossi S., Bramanti L., Gori A., Orejas C. (Cham: Springer), 73–110. doi: 10.1007/978-3-319-21012-4_51
Soares M. O., Tavares T. C. L., Carneiro P. B. M. (2019). Mesophotic Ecosystems: Distribution, Impacts and Conservation in the South Atlantic. Diversity Distrib. 255 (2), 255–268. doi: 10.1111/ddi.1284610.1111/ddi.12846
Soares M. O., Teixeira C. E. P., Bezerra L. E. A., Paiva S. V., Tavares T. C. L., Garcia T. M., et al. (2020). Oil Spill in South Atlantic (Brazil): Environmental and Governmental Disaster. Mar. Policy 115, 103879. doi: 10.1016/j.marpol.2020.103879
Sobrinho R. L., Bernardes M. C., Rezende C. E., Kim J. H., Schouten S., Damsté J. S. S. (2021). A Multiproxy Approach to Characterize the Sedimentation of Organic Carbon in the Amazon Continental Shelf. Mar. Chem. 232, 103961. doi: 10.1016/j.marchem.2021.103961
Sordo L., Fournier J., Oliveira V. M., Gern F., Panizza A. C., Lana P. C. (2011). Temporal Variations in Morphology and Biomass of Vulnerable Halodule Wrightii Meadows at Their Southernmost Distribution Limit in the Southwestern Atlantic. Bot. Mar. 54 (1), 13–21. doi: 10.1515/BOT.2011.007
Souza Filho P. W. M. (2005). Costa De Manguezais De Macromaré Da Amazônia: Cenários Morfológicos, Mapeamento E Quantificação De Áreas Usando Dados De Sensores Remotos. Rev. Bras. Geofís. 23, 427–435. doi: 10.1590/S0102-261X2005000400006
Steffen W., Rockström J., Richardson K., Lenton T. M., Folke C., Liverman D., et al. (2018). Trajectories of the Earth System in the Anthropocene. PNAS 115 (33), 8252–8259. doi: 10.1073/pnas.1810141115
Taillardat P., Friess D. A., Lupascu M. (2018). Mangrove Blue Carbon Strategies for Climate Change Mitigation are Most Effective at the National Scale. Biol. Lett. 14 (10), 20180251. doi: 10.1098/rsbl.2018.0251
Teixeira S. G., Souza-Filho P. W. M. (2009). Mapping Tropical Coastal Environments (Gulf of Maranhão, Brazil) Using Satellite Remote Sensing Images. Rev. Bras. Geofís. 27, 69–82. doi: 10.1590/S0102-261X2009000500006
The Blue Carbon Initiative (2020) About Blue Carbon. Available at: https://www.thebluecarboninitiative.org/about-blue-carbon.
Turra A., Denadai M. R. (2016). Linking Biodiversity and Global Environmental Changes in Brazilian Coastal Habitats. Braz. J. Oceanogr. 64, 3–4. doi: 10.1590/S1679-87592016064sp2ed
Van der Heijden L. H., Kamenos N. A. (2015). Reviews and Syntheses: Calculating the Global Contribution of Coralline Algae to Total Carbon Burial.s. Biogeosciences 12 (21), 6429–6441. doi: 10.5194/bg-12-6429-2015
Waycott M., Duarte C. M., Carruthers T. J. B., Orth R. J., Dennison W. C., Olyarnik S., et al. (2009). Accelerating Loss of Seagrasses Across the Globe Threatens Coastal Ecosystems. PNAS 106 (30), 12377–12381. doi: 10.1073/pnas.0905620106
Wiesebron M. (2013). Blue Amazon: Thinking the Defense of Brazilian Maritime Territory. Austral: Braz. J. Strategy Int. Relat. 2 (3), 101–124. doi: 10.22456/2238-6912.35039
Keywords: mangrove, seagrass meadow, salt marsh, carbon burial, carbon sink, South Atlantic
Citation: Soares MO, Bezerra LEA, Copertino M, Lopes BD, Barros KVdS, Rocha-Barreira CA, Maia RC, Beloto N and Cotovicz LC Jr. (2022) Blue Carbon Ecosystems in Brazil: Overview and an Urgent Call for Conservation and Restoration. Front. Mar. Sci. 9:797411. doi: 10.3389/fmars.2022.797411
Received: 18 October 2021; Accepted: 11 March 2022;
Published: 29 April 2022.
Edited by:
Joanne S. Porter, Heriot-Watt University, United KingdomReviewed by:
Antoine De Ramon N’Yeurt, University of the South Pacific, FijiRebecca Borges, University of Bremen, Germany
Copyright © 2022 Soares, Bezerra, Copertino, Lopes, Barros, Rocha-Barreira, Maia, Beloto and Cotovicz. This is an open-access article distributed under the terms of the Creative Commons Attribution License (CC BY). The use, distribution or reproduction in other forums is permitted, provided the original author(s) and the copyright owner(s) are credited and that the original publication in this journal is cited, in accordance with accepted academic practice. No use, distribution or reproduction is permitted which does not comply with these terms.
*Correspondence: Marcelo O. Soares, bWFyY2Vsb3NvYXJlc0B1ZmMuYnI=