- 1Department of Geology and Geoenvironment, National and Kapodistrian University of Athens, Zografou Campus, Athens, Greece
- 2Hellenic Centre for Marine Research, Institute of Marine Biology, Biotechnology and Aquaculture, Heraklion Crete, Greece
- 3 Istituto Nazionale di Geofisica e Vulcanologia, Sezione di Palermo, Palermo, Italy
- 4Istituto Nazionale di Geofisica e Vulcanologia, Sezione di Milano, Milano, Italy
- 5GEOMAR - Helmholtz Center for Ocean Research Kiel, Kiel, Germany
- 6Department of Earth and Environmental Sciences, University of Ottawa, Ottawa, ON, Canada
- 7Laboratoire de Géologie – CNRS, UMR 8538, École Normale Supérieure, Paris Sciences Letters (PSL) University, Paris, France
- 8Remote Sensing Laboratory, National Technical University of Athens, Athens, Greece
- 9Department of Physics, National Kapodistrian University of Athens, Zografou Campus, Athens, Greece
- 10School of Creative Technologies, University of Portsmouth, Portsmouth, United Kingdom
- 11Department of Surveying and Geoinformatics Engineering, University of West Attica, Athens, Greece
Submarine hydrothermal systems along active volcanic ridges and arcs are highly dynamic, responding to both oceanographic (e.g., currents, tides) and deep-seated geological forcing (e.g., magma eruption, seismicity, hydrothermalism, and crustal deformation, etc.). In particular, volcanic and hydrothermal activity may also pose profoundly negative societal impacts (tsunamis, the release of climate-relevant gases and toxic metal(loid)s). These risks are particularly significant in shallow (<1000m) coastal environments, as demonstrated by the January 2022 submarine paroxysmal eruption by the Hunga Tonga-Hunga Ha’apai Volcano that destroyed part of the island, and the October 2011 submarine eruption of El Hierro (Canary Islands) that caused vigorous upwelling, floating lava bombs, and natural seawater acidification. Volcanic hazards may be posed by the Kolumbo submarine volcano, which is part of the subduction-related Hellenic Volcanic Arc at the intersection between the Eurasian and African tectonic plates. There, the Kolumbo submarine volcano, 7 km NE of Santorini and part of Santorini’s volcanic complex, hosts an active hydrothermal vent field (HVF) on its crater floor (~500m b.s.l.), which degasses boiling CO2–dominated fluids at high temperatures (~265°C) with a clear mantle signature. Kolumbo’s HVF hosts actively forming seafloor massive sulfide deposits with high contents of potentially toxic, volatile metal(loid)s (As, Sb, Pb, Ag, Hg, and Tl). The proximity to highly populated/tourist areas at Santorini poses significant risks. However, we have limited knowledge of the potential impacts of this type of magmatic and hydrothermal activity, including those from magmatic gases and seismicity. To better evaluate such risks the activity of the submarine system must be continuously monitored with multidisciplinary and high resolution instrumentation as part of an in-situ observatory supported by discrete sampling and measurements. This paper is a design study that describes a new long-term seafloor observatory that will be installed within the Kolumbo volcano, including cutting-edge and innovative marine-technology that integrates hyperspectral imaging, temperature sensors, a radiation spectrometer, fluid/gas samplers, and pressure gauges. These instruments will be integrated into a hazard monitoring platform aimed at identifying the precursors of potentially disastrous explosive volcanic eruptions, earthquakes, landslides of the hydrothermally weakened volcanic edifice and the release of potentially toxic elements into the water column.
Introduction
About 80% of volcanism on Earth is submarine (Crisp, 1984), mainly in subduction-related arc and mid-ocean ridge (MOR) geodynamic settings. The deep-seated mantle processes and associated volcanic activity are primary drivers of the chemical and biogeochemical evolution of the global oceans. Submarine volcanism is often associated with seafloor hydrothermal activity and degassing of high-temperature magmatic volatiles, presenting both potential hazards and opportunities for the future, such as mineral deposit formation that are the focus of intense debate over marine resources (e.g., Beaulieu et al., 2017).
Hydrothermal activity also supports an astonishing diversity of seafloor vent ecosystems influencing global carbon and nutrient cycles (Van Dover et al., 2002; Sander and Koschinsky, 2011; Hawkes et al., 2015), aspects that have been widely studied in different geodynamic settings (Lupton et al., 1990; Taran et al., 1992; Lilley et al., 1993; Tsunogai et al., 1994; von Damm, 1995; von Damm et al., 1995; Butterfield et al., 1997; Lupton et al., 2006; Lupton et al., 2008; Lupton et al.,2009; Caracausi et al., 2005a; Lan et al., 2010; Hannington et al., 2011; Kilias et al., 2013; Rizzo et al., 2016a; Rizzo et al., 2019; Bravakos et al., 2021). Most of the known modern seafloor hydrothermal systems, with their associated mineral deposits and chemosynthetic microbial biomes, occur at MORs and mature back–arc spreading centers, typically at water depths of 2000 to 4000m (e.g., Butterfield et al., 1990; Lilley et al., 1993; Von Damm, 1995; Von Damm et al., 1995; Lupton et al., 1999; Price and Giovanneli, 2017).
The least studied seafloor hydrothermal vent sites are associated with shallow submarine arc volcanoes and arc–related rifts in subduction–related settings (Taran et al., 1992; Tsunogai et al., 1994; Lupton et al., 2006; Lupton et al., 2008; Lan et al., 2010), which typically occur at a much shallower water depth (<1000m). These vigorously degassing, submarine hydrothermal systems are associated with significant and dangerous volcanic and seismic activity and are more likely to impact the marine environment near-coastal populations (Dando et al., 1995; Zimanowski and Büttner, 2003; Puzenat et al., 2021; Mei et al., 2022). The cabled sea-floor observatory deployed off the coast of Panarea hydrothermal system (Aeolian Arc, South Tyrrhenian Sea, it was exploded in November 2002) at a depth of 24m, is at the moment the only monitoring system installed in the Mediterranean Sea which automatically transmits data of chemical and physical signals (T, EC, pH, dissolved CO2, acoustics) to shore (Caracausi et al., 2005a; Caracausi et al., 2005b).
Research on shallow submarine arc volcanoes is still in its infancy despite their potentially severe hazards. In the Mediterranean, the Aeolian Island Arc of the Tyrrhenian Sea (Caliro et al., 2004; Caracausi et al., 2005a; Chiodini et al., 2006; Capaccioni et al., 2007; Heinicke et al., 2009; Tassi et al., 2009; Monecke et al., 2014; Petersen et al., 2014; Tassi et al., 2014; Tassi et al., 2015; Esposito et al., 2018) and the Hellenic Volcanic Arc of the Aegean Sea (Dando et al., 2000; Nomikou et al., 2012; Nomikou et al., 2013; Carey et al., 2013; Kilias et al., 2013; Cantner et al., 2014; Christopoulou, et al., 2016; Ulvrova et al., 2016; Rizzo et al., 2016a; Rizzo et al., 2019; Puzenat et al., 2021; Daskalopoulou et al., 2022; Kilias et al., 2022) have attracted lots of attention, as very little is known about their volcanic and hydrothermal activity and impacts over intermediate and longtime scales. Nevertheless geological and historical records point to many catastrophic events that have had a broad impact throughout Southern Europe (Druitt et al., 1999).
At global scale, in situ seafloor observatories for long-term monitoring of submarine volcanoes have been developed at a number of locations, such as the Azores node of European Multidisciplinary Seafloor and water column Observatory (EMSO: Colaco et al., 2011; Best et al., 2014; Escartin et al., 2015), Axial Seamount in the NE Pacific, which is part of the U.S. National Science Foundation (NSF)-funded Ocean Observatories Initiative (OOI) Cabled Array that captured the 2015 eruption (Nooner and Chadwick, 2016; Wilcock et al., 2016; Trowbridge et al., 2019; Cabaniss et al., 2020), the Ocean Networks Canada cabled observatory at Endeavour Ridge (Kelley et al., 2014), and also the Mayotte deep-sea eruption (North Mozambique channel) with an observatory being put in place by France (Feuillet et al., 2021). Several of these observatories have successfully captured changes in the dynamics and evolution of submarine volcanism. For example, the 2015 Axial Seamount eruption was successfully forecast within a 1-year time window on the basis of volcanic deformation, ascribed to pressurization of a magma reservoir at depth, and was captured in real time by the OOI Cabled Array (Nooner and Chadwick, 2016). A combination of deformation and seismic monitoring are being used to attempt to forecast the next eruption (Chadwick et al., 2022).
This paper is a design study, which reports on a joint effort of a multinational team of Earth and Ocean scientists to build a new seafloor observatory (SANTORini’s seafloor volcanic observatorY, SANTORY) that will be developed and installed within the crater of the submarine Kolumbo volcano (Nomikou et al., 2012; Nomikou et al., 2013) to monitor its activity and mitigate the hazards it poses to the neighboring densely populated volcanic island of Santorini.
SANTORY represents a new research priority for a combination of reasons:
Geochemical diversity: The Kolumbo shallow-seafloor massive sulfide (SMS) hydrothermal mineralization shows a range of elements and minerals, including those with security of supply issues (e.g., Sb, Sr, barite), and those that may have potential environmental implications (Tl, As, Sb, Hg), in areas exploited by fishing and tourism, if not managed. Understanding the geochemistry of these occurrences and the potential toxicity impact associated with their preservation and/or discharge, needs greater scientific focus.
Geological and Environmental diversity: The Kolumbo volcanic terrain includes nearly exposed volcanic flanks and sheltered crater within relatively shallow waters of European Exclusive Economic Zones (EEZs) making related geohazards more dangerous than those in international waters. Moreover, it is a dynamic environment with changing sediment inputs from islands and continental landmasses, and variable topographies and seismic activity which affect rates and levels of mass wasting. These factors affect seafloor toxic-metal budget and liberation potential.
Near-term submarine biotechnology potential: Environments such as Kolumbo are unique locations of high biological productivity, and high degrees of endemism, of added value to global biomedical research. The Hellenic (South Aegean) Volcanic Arc has been described as the largest ‘submarine volcanic ecosystems, a significant resource of novel genes and pathways with potential submarine biotechnological applications (Chrousos et al., 2020).
Study Site: SANTORINI-Kolumbo Volcanic Field
A linear feature in the southern Aegean Sea known as the Christiana–Santorini–Kolumbo (CSK) rift (Nomikou et al., 2019) (Figure 1) hosts one of the most important volcanic fields in Europe, having erupted more than 100 times in the last 400.000 years (Druitt et al., 1999). Running in a NE–SW direction, it includes several volcanic centers of late Pliocene to Pleistocene age as part of the larger east–west trending Hellenic subduction zone, north of the island of Crete. The CSK rift lies in a 100 km long, 45 km wide zone of en echelon NE–SW-trending rifts, including the Santorini–Amorgos Tectonic Zone (Nomikou et al., 2018). It hosts volcanic centers that include the extinct Christiana Volcano and associated seamounts, Santorini caldera with its intracaldera Kameni Volcano, Kolumbo Volcano, and 25 other submarine cones of the Kolumbo chain, which extends NE along the floor of the Anhydros Basin. Kolumbo is currently the most volcanically active part of the CSK (Nomikou et al., 2012; Hooft et al., 2017). The world-known Santorini volcano is a globally significant volcanic center, with numerous large-volume explosive eruptions over the last 600.000 years (Druitt et al., 1999). It is widely renowned for its 3600 BP Minoan eruption, which is thought to have had a significant impact on the homonymous Minoan civilization (Crete), in the Eastern Mediterranean Sea, because of the subsequent earthquakes and tsunamis (Dimitriadis et al., 2009; Ulvrova et al., 2016; Nomikou et al., 2016).
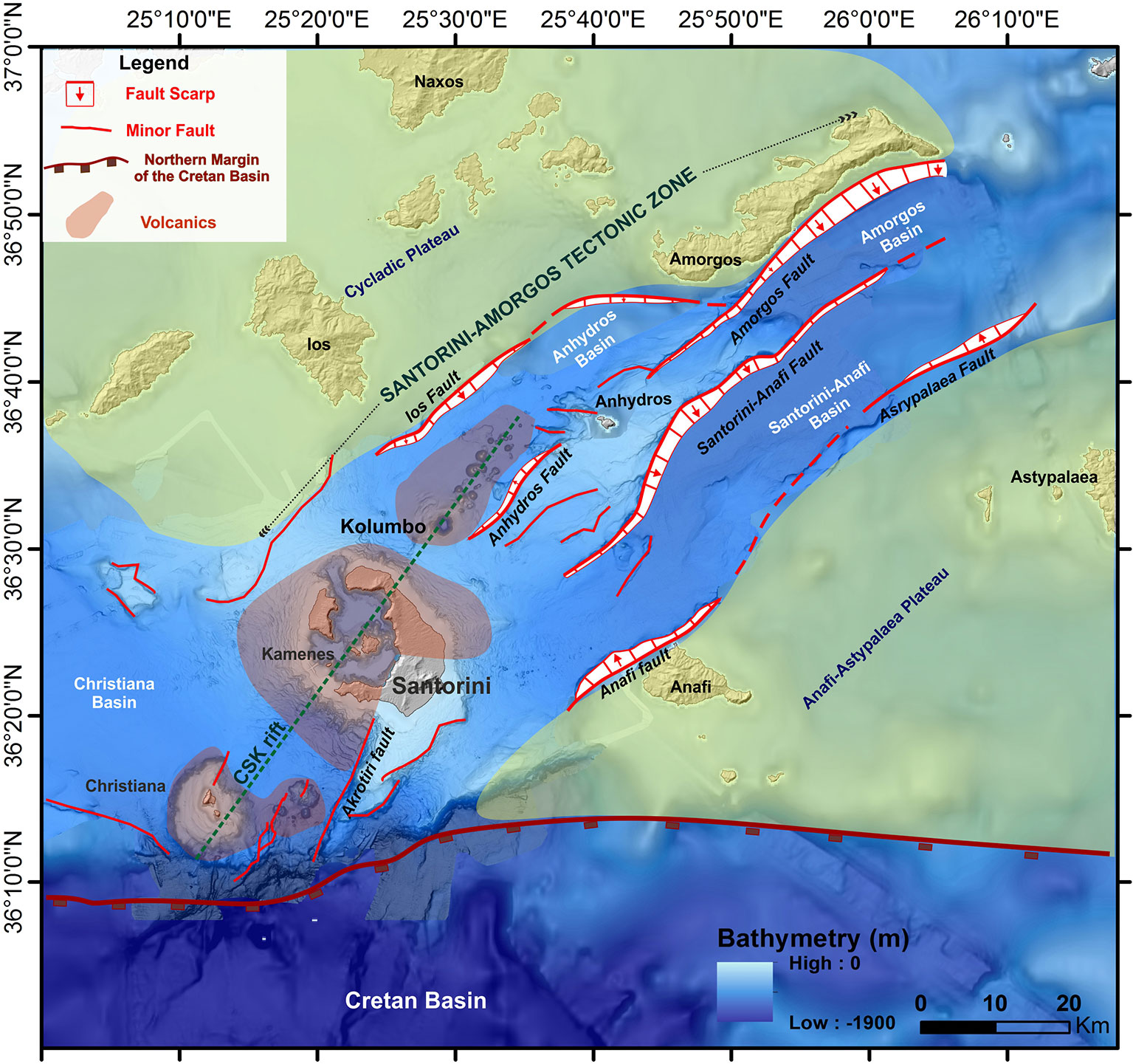
Figure 1 Christiana-Santorini-Kolumbo rift (South Aegean Sea, Greece) (Nomikou et al., 2018; Nomikou et al., 2019).
The Kolumbo volcano, a 3 km diameter cone with a 1700 m wide crater, is the most prominent entirely submarine volcanic feature of the CSK rift. The crater’s rim is currently as shallow as 18 m below sea level, and the flat crater floor is 505 m below sea level (Figure 2). At least seventy people who were either at sea or along the NE coastline of Santorini died of asphyxiation due to acidic gases released by an intense eruption from Kolumbo in 1650 AD (Cantner et al., 2014; Fuller et al., 2018). In addition, a large tsunami on the 29th of September 1650 caused widespread damage on Santorini and on other islands within a 150 km radius (Ulvrova et al., 2016).
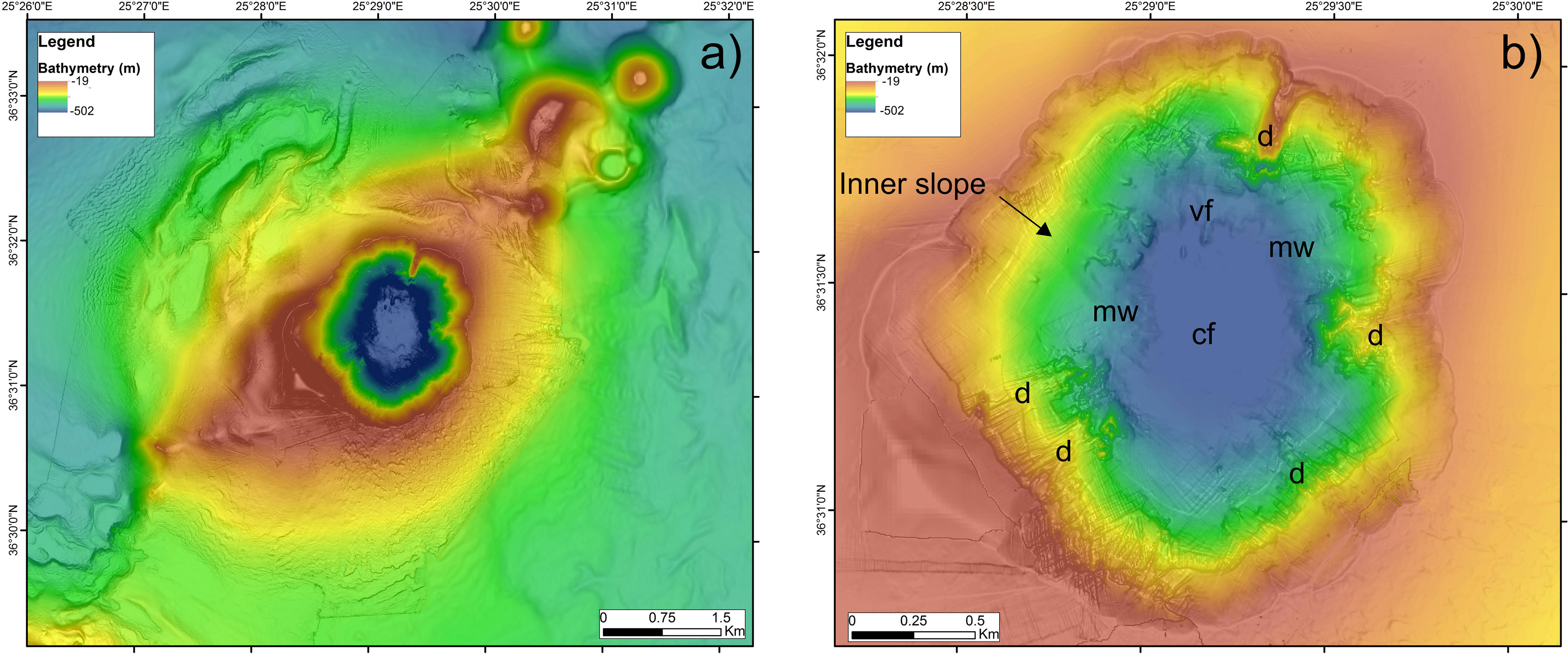
Figure 2 (A) An AUV high-resolution bathymetric map of Kolumbo volcano (B) An AUV high-resolution map of Kolumbo crater (High resolution bathymetric data were collected by GEOMAR’s AUV Abyss during mission POS 510, Hannington, 2018). d, dyke; cf, crater floor; mw, mass wasting deposits; vf, vent field.
The first detailed bathymetric map of the Kolumbo volcano was produced in 2001 using the 20 kHz SB2120 swath system on R/V Aegaeo (Nomikou et al., 2012; Nomikou et al., 2013). More recently in 2015, bathymetric data were also acquired on-board the R/V Marcus Langseth using the Simrad Kongsberg EM122, 12 kHz multibeam echo sounder. In 2017, high-resolution AUV (Autonomous Underwater Vehicle) data were collected during POS510 cruise, in 7 missions of AUV Abyss (GEOMAR) (Hannington, 2018), under the framework of the collaborative project “ANYDROS: Rifting and Hydrothermal Activity in the Cyclades Back-arc Basin” (Figure 2). The AUV mapping allows a 2m resolution that can identify seafloor geomorphological features that are not visible in conventional ship-based multibeam data. The new bathymetric map of Kolumbo volcano (Nomikou et al., 2019b) reveals: a) the abrupt inner slopes of Kolumbo crater, b) the almost flat seafloor surrounding the active vent field at the northern part of the crater floor (485 m depth) c) dykes exposed in the inner slopes, d) the mass-wasting deposits in the inner slopes, e) the curvilinear scarps with inward dipping faces at the W-NW rim of the crater.
Kolumbo is the most active volcanic center in the area and is considered the most dangerous submarine volcano in the Mediterranean Sea, partly because it is prone to explosive activity. At present, Kolumbo is only monitored sporadically during isolated oceanographic missions, and therefore nearby highly populated areas are vulnerable to significant risks from the volcano (such as earthquakes, tsunami, landslides). Current knowledge mainly concerns Kolumbo’s hydrothermal activity as a source of potentially toxic metal(loid)s, climate-critical gases, as well as natural radioactivity (Jamieson et al., 2013; de Ronde et al., 2019; Neuholz et al., 2020; Klose et al., 2021).
The hydrothermal system within its caldera (Sigurdsson et al., 2006; Kilias et al., 2013), emits an important but poorly quantified flux of mantle-derived gases together with aqueous fluids venting at 265°C (Carey et al., 2013; Klaver et al., 2016; Hannington, 2018; Rizzo et al., 2016a; Rizzo et al., 2019) (Figure 3). The emitted gases composed of nearly pure CO2 and other trace gases (e.g, H2S, CH4, H2, CO) common to hydrothermal systems at active volcanoes. Noble gases are present in trace concentrations, and helium (3He/4He) ratios of up to 7 Ra, with a Mid-ocean ridge basalts: MORB-like signature, are the highest reported along the Hellenic volcanic arc (Rizzo et al., 2016a; Rizzo et al., 2019). In Kolumbo gases, a remarkable concentration of Hg(O) has also been found (Rizzo et al., 2019), suggesting active magmatic-hydrothermal degassing activity of this volcano that poses several hazards for the area; this is confirmed by elevated contents of Hg fixed in hydrothermal sulfide minerals deposited in seafloor hydrothermal deposits (see below).
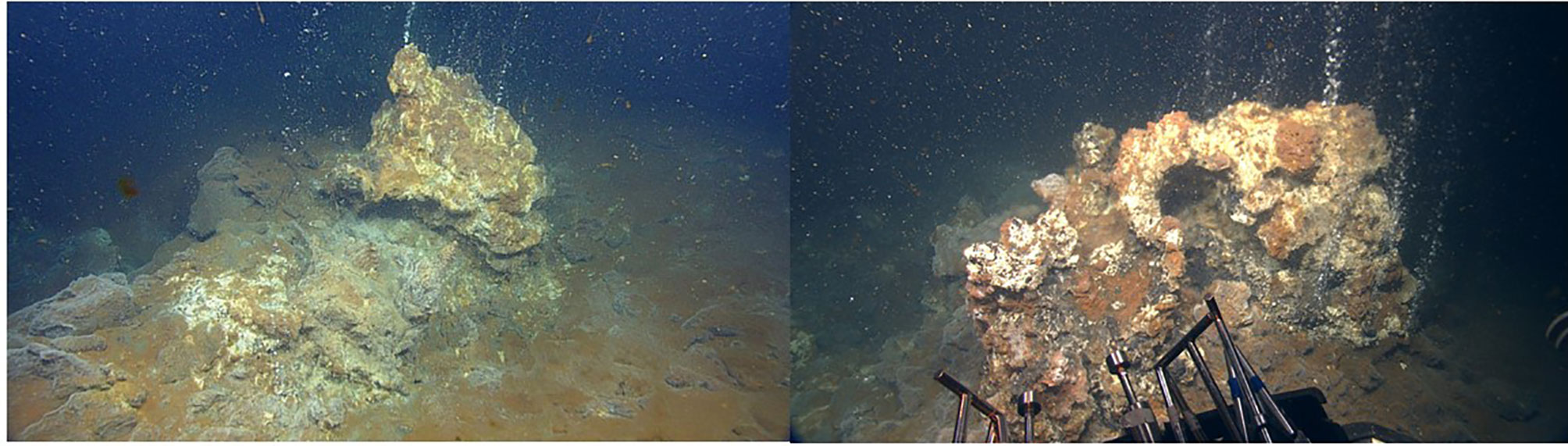
Figure 3 ROV captured photos (E/V Nautilus Leg NA-007) (Carey et al., 2011) of active high–temperature (max 265°C) Kolumbo hydrothermal sulfide mounds, vigorously discharging boiling fluids and gases (>99% CO2) (Carey et al., 2013b; Kilias et al., 2013).
The Kolumbo crater hosts an active shallow-marine, boiling hydrothermal system currently forming the only known polymetallic seafloor massive sulfide (SMS) deposits associated with continental margin volcanism, with high contents of potentially toxic, volatile metal(loid)s (VTML), i.e., Ag, Hg, As, Sb, Pb and Tl (Kilias et al., 2013; Kilias et al., 2016). The VTML are contributed to the Kolumbo hydrothermal system, possibly via active degassing of a shallow magma chamber and the shallow submarine hydrothermal venting (de Ronde et al., 2005; Hannington et al., 2005; Kilias et al., 2017). High VTML contents are variably distributed in sulfide minerals (pyrite, marcasite, galena, sphalerite, chalcopyrite, Pb-Sb sulfosalts, stibnite, and orpiment- and realgar-like As-sulfides) which constitute potential natural source of VTML to the overlying seawater column (Kilias et al., 2016; Fuchida et al., 2017; Zegkinoglou et al., 2019a; Fallon et al., 2019; Zitoun et al., 2021) (Figure 4).
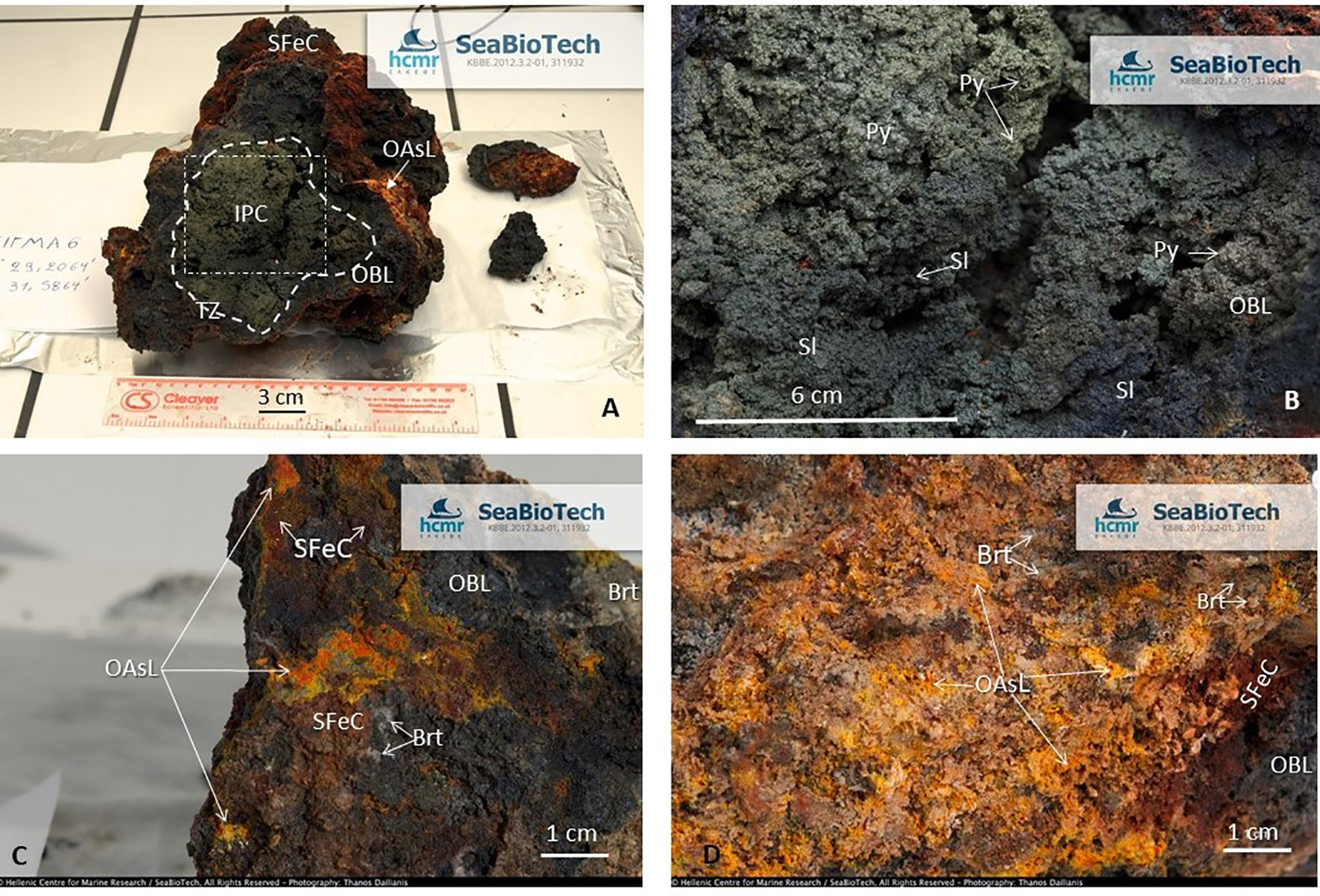
Figure 4 Ex situ photographs of a typical recovered Au-rich SMS chimney (Au up to 32 ppm; Au/(Cu+Zn+Pb) = 1.9), enriched in potentially toxic, volatile metal(loid)s (As, Sb, Pb, Ag, Hg, Tl) (VTML), Kolumbo hydrothermal vent field (Kilias et al., 2013). All sulfide phases are variably enriched in Au and VTML. (A). Basal cross section of chimney showing typical pattern of sulfide phase zonation. (B) Enlargement of the squared area of (A), showing the IPC texture that is dominated by fine-grained botryoidal masses of pyrite surrounding fluid flow channels. (C, D). Outermost skin of the OBL (dark bluish-black), composed of patches with red, orange, and yellow auriferous orpiment-, and realgar-, like phases (OAsL), associated with barite; these As-phases show evidence of oxidative weathering in the form of local, dark brown SFeC. Py, Auriferous As-pyrite; Brt, barite; Sl, polymetallic sulfide aggregates; IPC, Inner Pyrite Core; OBL, Outermost Barite Layer; TZ, Transition Zone; OAsL, Outer As-rich Layer; SFeC, Surface Fe-oxyhydroxide rich Crust. “SeaBioTech” EU-FP7 project (Grant No. 311932), are thanked for funding the sampling.
In terms of radioactivity, the amount being released by Kolumbo is largely unknown, as is the case with most submarine volcanic systems featuring hydrothermal activity around the world. Natural radioactivity stems mainly from naturally occurring long-lived radioactive uranium and thorium daughters, which are produced in the mantle and can be released to the water column by hydrothermal fluids. The presence of VTML may also suggest the presence of the heavier and radioactive Ra isotopes in the dynamic hydrothermal processes, despite the latter have not been studied in Kolumbo. Among these natural radioactive emitters, important radio tracers present in HVF are the gaseous radon (222Rn) and the thoron (220Rn), which can easily escape the mantle and the sediment layer via diffusion and transfer processes. The study of their kinetics in the marine environment can offer valuable information on the dynamics of the system, and radon and thoron releases may be seismic precursors (Hwa and Kim, 2015). With SANTORY, we expect to close this knowledge gap worldwide using long-term, in situ monitoring, overcoming the remoteness and the harsh conditions of Kolumbo, by installing and operating a novel, high-resolution γ-ray spectrometer.
The Kolumbo volcano has a number of unique attributes that make it attractive for an observatory: i) it is ideally located and very accessible; (ii) it shows significant magmatic and hydrothermal activity; iii) it hosts a unique physical and chemical environment, owing to its deep, nearly vertical-walled crater; iv) the high-temperature hydrothermal vent field is an extreme environment emitting high concentrations of VTML that are partly fixed in polymetallic hydrothermal sulfide chimneys and mounds, and also released into the seawater column in unknown quantities, causing also build-up of hydrothermally emitted CO2 resulting in persistent acidic conditions; v) the observed metal(loid) enrichment highlights the significance of shallow submarine hydrothermal vent activity as a potential source of toxic metal(loid)s in natural areas extensively exploited by tourism and fishing; vi) the hydrothermal vents are habitats for extremophiles that are not found or only detectable in very low numbers in other active vent fields; and (vii) of particular interest is the opportunity to assess changes in habitat conditions (e.g., via genomics) and the release of toxic elements due to volcanic and hydrothermal activity unrest.
Description of the Planned Observatory
The SANTORY observatory aims to monitor the current activity at Kolumbo volcano and link these findings to ongoing observations of the wider Santorini Volcanic Complex, by developing and integrating state-of-the-art technology for in situ monitoring along with discrete sampling and measurements (Figure 5).
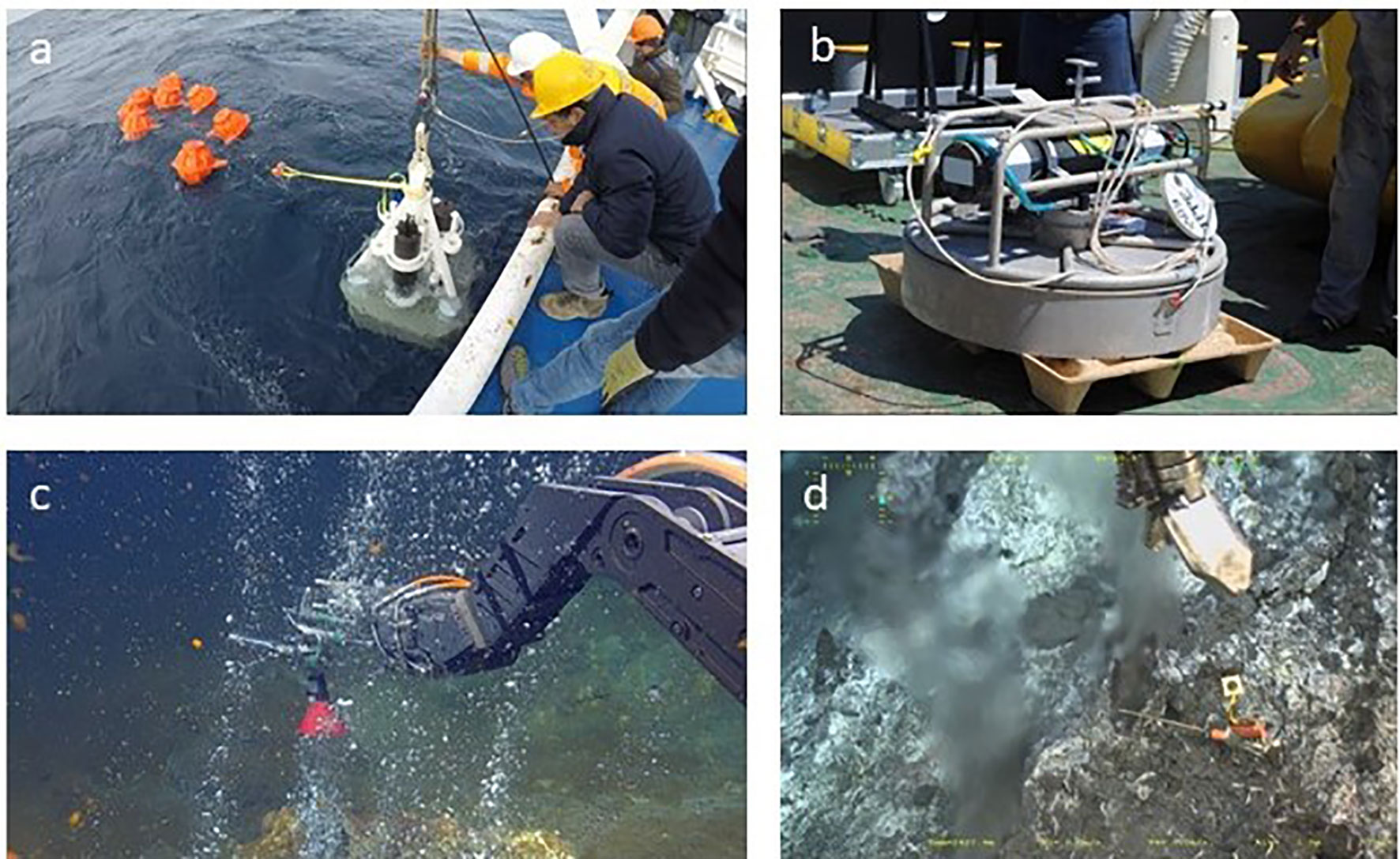
Figure 5 Some of the novel sensors and monitoring systems that will be deployed in the SANTORY observatory: (A) automated seafloor system for geochemical multi-parametric monitoring (i.e., dissolved CO2, H2S, O2, temperature, pressure, conductivity, pH, water column current, and turbidity, hydrophone) (Longo et al., 2021a), (B) IPGP (France) pressure gauge deployed at Santorini during the 2012 Caldera cruise (Vilaseca et al., 2016) measuring vertical seafloor movements, (C) discrete gas-tight sampler above Kolumbo vent (Carey et al., 2013), (D) Temperature sensor deployed at the Lucky Strike hydrothermal field during the Bathyluck 2009 cruise (Barreyre et al., 2012).
The heart of the observatory will include moorings with spectral imaging capabilities, in situ operating sensors, including chemical sensors, pressure gauges coupled with tiltmeters, fluid/gas samplers triggered by ROV’s, and purpose-built sensors to record physical and chemical parameters in diffuse hydrothermal flows. Chemical sensors will include autonomously operating mass spectrometers to characterize dissolved volcanic gases in the water column, while operating continuously different timescales (e.g., days to weeks). A stand-alone observatory deployment is planned at the crater bottom, together with an OBS, to collect high-frequency data form a wide range of probes (pH, T, EC, dissolved CO2, CH4 and O2, acoustics). Monitoring will be conducted through long-term deployments of the instrumentation, that will record continuously, and with instrument recovery during recurrent cruises (e.g., 1 yr). This approach will provide time series to investigate processes with temporal variabilities spanning from less than a day to over a year. The installation of the SANTORY sensors and monitoring systems will be done based on the morphology and distribution of hydrothermal features in the area. This fine-scale geological knowledge is required to both provide a precise context necessary to properly interpret the acquired time series of both data and sampling (fluids and ecosystems primarily), while securing that mesurements are performed at the same locations. The on-site submarine monitoring will be complemented with on-shore land-based seismographs installed on Santorini Island. The proximity of Kolumbo to Santorini ensures high accuracy in seismic monitoring without the need to install OBS inside the volcanic cone, which increases the cost and complexity of operation. Tables 1, 2 provide information of the planned activities.
Imaging Data
Observing the flow rates of active hydrothermal vents and chimney growth rates as well as correlating this information with the overall activity of the Kolumbo system is crucial for monitoring. Up to now flow rates have been measured quite sporadically during costly underwater expeditions with supporting surface vessels (Carey et al., 2011; Nomikou et al., 2012; Carey et al., 2013; Nomikou et al., 2013). In SANTORY the goal is to design, develop and deploy optical imaging systems to document active processes the seafloor, and in particular hydrothermal dynamics and links to associated ecosystems. Two type of imaging systems will be designed and deployed.
i) A stand-alone, underwater optical video system with associated light sources, will be developed to monitor the main Kolumbo hydrothermal vents. The system will be designed to capture short video sequences (a few minutes) at regular time intervals (e.g., every 4-6 hours), an approach successfully deployed at other deep sea hydrothermal observatories (e.g., EMSO-Azores).
ii) SANTORY will deploy an autonomous submarine imaging system comprising spectral and optical RGB color sensors, and deployable also by ROV, to characterized accurately seafloor reflectance, and hence infer physical and chemical properties to obtain detailed seafloor composition maps. The spectral imaging system will be developed based on integrated and synchronized high-end VNIR and high-resolution RGB video cameras. Distortion and vignetting of frames requires processing (Vakalopoulou and Karantzalos, 2014; Kandylakis et al., 2015) to reconstruct the spectral reflectance of the seafloor that accounts for ambient light conditions, ROV lighting sources and the inherent optical properties of the surrounding water column. This is achieved exploiting a combination of visible and near-infrared (VNIR) spectra through geometrically and spectrally consistent seafloor mosaics to accurately map biotic and abiotic cover.
Geochemical Parameters
iii) Santory will simultaneously measure key physical and geochemical parameters of the vent sites, including dissolved CO2, H2S, O2, temperature, pressure, salinity, conductivity, pH, water column current, turbidity and passive hydro-acoustics. The resulting time-series will be compared with other recordings such as volcanic tremor, changes in fluid flux, and chimney growth. These deployments will include a new stand-alone multi-parametric geochemical recording system will be deployed on the seafloor to collect these data consisting of a suite of probes with both slow and fast cycling times (Longo et al., 2021a; Longo et al., 2021b) insuring multi-parameter characterization of specific sites.
iv) Santory will specifically monitor in situ the concentration of dissolved CO2 in the water column above the hydrothermal vents and on the crater floor using highly accurate pCO2 sensors. Deployed both at the seafloor and on mobile platforms (e.g., ROVs during recovery cruises), these data will provide both the temporal variability and constrain the 3D pCO2 structure, that is required to his will allow us to map the distribution and variability of CO2 concentration dissolved in the water column above the vents and reconstruct the flux of gas emitted from the crater bottom, with implications for the budget of the emitted volcanic species. The flexible design allows deployment from different platforms (e.g. ROV, long-term deployments on seafloor observatories, buoys and moorings and profiling applications using water sampling rosettes). Samples stored in the gas-tight samples will be analyzed for major and trace elements in on-shore laboratories.
v) Complementing the continuous chemical monitoring, discrete gas-tight samplers deployed by ROVs will collect hydrothermal fluids from the vents at the crater bottom. Samples stored in glass and stainless-steel bottles will be analyzed onshore to study the chemistry of magmatic volatiles (CO2, N2 and CH4) and the isotopic composition of noble gases (He, Ne, Ar).
Combined chemical monitoring and sampling will: i) deepen our knowledge of the origin of the emitted fluids; ii) allow comparisons to recognize changes and determine the cause; iii) constrain changes in the state of activity of the volcano. The main goals are: (a) to decipher whether potentially toxic trace VTML elements are released in the seawater column.; (b) to investigate the partitioning of trace VTML elements between hydrothermal fluids and sulfide minerals; and (c) to investigate whether precious trace elements (i.e., Au) are possibly transported in colloidal suspensions in boiling diffuser hydrothermal fluids. Sampling protocols and analytical methods for major and trace element analysis of fluids and particulate solids are detailed in Gartman et al. (2018); Hannington and Garbe-Schönberg (2019) and Evans et al. (2020) (see also below “Shore-based mineralogical and geochemical analyses”).
Radionuclides Coupled With Chemistry
vi) In addition to variations in CO2, H2S, and O2, we expect measurable fluxes of radon and/or thoron commonly associated with volcanic emissions (Jamieson et al., 2013; Hwa and Kim, 2015; de Ronde et al., 2019; Neuholz et al., 2020; Klose et al., 2021). A prototype underwater γ–radiation spectrometer will be developed for operation on the seafloor observatory to monitor these fluxes together with other physical and chemical data. In addition to stand-alone detectors, SANTORY will deploy γ–detectors on ROV to provide real–time, in situ monitoring of radioactivity levels near the vents. In addition to monitoring dynamics of radon/thoron emanation from the volcanic vents, several applications for the instruments are envisioned, including radioisotope tracing experiments, (e.g., correlation of radiotracers to seismicity/venting), subseafloor hydrogeological studies, and sediment dating (Jamieson et al., 2013; de Ronde et al., 2019; Neuholz et al., 2020; Klose et al., 2021). The main effort in SANTORY is to introduce new types of detectors that are smaller, more efficient and less power–consuming, to offer continuous monitoring and new opportunities for scaling up the design and interoperability with other instruments.
Physical Parameters
The hydrothermal activity is influenced by both the dynamics of the overlying ocean, and by subseafloor geological processes. Monitoring of the hydrothermal activity will thus be coupled with monitoring of physical parameters, in order to understanding the links (or lack of) between the different time-series and identify the processes behind any hydrothermal temporal variability.
vii) Temperature variations of the hydrothermal outflow at different vents and areas of the crater floor will be measured with stand-alone low/high temperature recorders. Hydrothermal fluid temperature in these systems often shows fluctuations that may be related to volcanic processes, tectonic deformation, or seismicity (Sohn et al., 2009), in addition to tidal forcing (Barreyre et al., 2012; Barreyre et al., 2014), or variations in the rate of recurrent boiling and hydrothermal fluid-seawater mixing (Gartman et al., 2019). In particular, the temporal variability at tidal frequencies can be exploited to understand the subseafloor permeability structure of these systems (e.g., Crone and Wilcock, 2005; Barreyre et al., 2022).
viii) Pressure gauges and tiltmeters, placed at the rim and within the crater, and coupled to temperature sensors, will recorddifferential vertical displacements, while current meters will monitor currents water within the crater. A prior study in Santorini caldera recorded seiches and seasonal events (e.g., Vilaseca et al., 2016), and these diurnal and seasonal oceanographic fluctuations have been shown to modulate the temperature of the outflow at the seafloor, particularly in the case of diffuse hydrothermal flow (Barreyre, et al., 2014; Barreyre, et al., 2018). The bottom pressure gauges and tiltmeters will be placed on benchmarks and anchored to the seafloor using an ROV. The tiltmeters measure instrument inclination along two horizontal axes with high resolution (Fabian and Villinger, 2008). Internal temperatures of the instruments are also monitored to correct pressure measurements. These stations will be installed both within and outside the crater.
Shore-Based Mineralogical, Geochemical and Biological Analyses
SANTORY in-situ monitoring will be accompanied by shore-based measurements on samples collected. Laboratory work will provide details on various parameters that are not time-sensitive in terms of hazard risk estimation, but will clarify the effects of the active volcano on the formation of its ecosystem: geochemical processes, biomineralization, and more.
ix) Sulfide mineralogy (i.e., mineral abundances and their VTML content) is the main control on trace element distribution, chemical speciation and bioavailability of VTML, and is key to assessing subsequent oxidative weathering, dissolution and release of VTML and potential toxicity. Sulfide mineralogy and chemistry at a high spatial resolution and per-mineral basis will be determined using a combination of optical microscopy and scanning electron microscopy with energy dispersive X-ray (SEM-EDS) analysis, an electron microprobe (EMPA), micro-X-ray fluorescence (µXRF), LA-ICP-MS analysis, and secondary ion mass spectrometry (SIMS). Integrated micron-scale maps of the texture, chemistry and mineralogy of sulfides that will be produced, will allow determination of detailed paragenetic relationships, quantitative understanding of the mineralogical sequestration of VTML, VTML distribution among the main sulfides and accessory phases contained in SMS.
Such detailed mineralogical data will be used for the determination and quantification of the mineralogical changes through time seen between the pristine hypogene sulfide assemblages and degraded material from the inactive and extinct SMS deposits due to oxidative weathering. Mineral associations are important as contact between certain minerals could lead to galvanic reactions that are known to affect sulfide dissolution rates by more than an order of magnitude during oxidative weathering (Vera et al., 2013; Fuchida et al., 2017; Hauton et al., 2017; Fallon et al., 2017; Fallon et al., 2018; Fallon et al., 2019). Furthermore, detailed SEM and LA-ICPMS will be undertaken on secondary products to document the fate of sulfide-hosted trace metals released during the weathering process. This has been documented for terrestrial systems, but such studies are lacking in SMS on the seafloor. Whole-fluid sample treatment protocols and major and trace element analysis by inductively coupled plasma mass spectrometry (ICP-MS), and analysis of solid particles from nano-to-macro scale, by diffraction (XRD), Scanning electron microscopy/energy dispersive X-ray spectroscopy (SEM/EDS), and Transmission electron microscopy (TEM/ED), are detailed in Gartman et al. (2018; Gartman et al., 2019), Hannington and Garbe-Schönberg (2019) and Evans et al. (2020).
x) Moreover, the associated chemosynthetic microbial biomes and the presence and risks of potential pathogens are poorly known (e.g., Oulas et al., 2016; Christakis et al., 2018; Mandalakis et al., 2019; Bravakos et al., 2021). Together with the geochemical and isotopic characterization, samplers for water and microbial mat collection will be also deployed at the vents and the collected samples will be further processed in the lab. We will integrate genomic approaches in marine microbial observation by combining standardized protocols for sampling, environmental DNA extraction and analysis through specific gene markers such as the amplicon sequencing of the 16S rRNA gene which is a common taxonomic marker for both bacteria and archaea.
Expected Results-Discussion
With SANTORY, we expect to obtain high-frequency information on changes in the underlying permeability structure and fluid output of the Kolumbo volcanic system, including potential impacts on the local environment.
SANTORY will provide geochemical, mineralogical, physical and biological data, over an extended period of time, to address a broad range of scientific topics, including:
- Invest on innovative, next–generation technology and the latest developments in marine genomic observation, to monitor active shallow (<500m) hydrothermal field processes and assess volcanic and seismic hazards (e.g., landslides, tsunamis)
- Constrain the processes that modulate and control the temporal variability in hydrothermal activity
- Understand the interdependencies that this variability imparts on the associated ecosystems
- Determine the different parameters of the system that could be used to evaluate major changes that may indicate risks (e.g., enhanced magmatic and associated hydrothermal activity)
- Develop and adapt monitoring strategies coupled with instrumental development.
- Determine the largest controls of potentially toxic, volatile trace metal(loid) (VTML) distribution, with direct effect on subsequent dissolution and potential toxicity
- Determine the potential toxicity impact of the dissolution and release of VTML via the process of oxidative weathering of the Kolumbo SMS, i.e., mineralogical changes and diagenesis on the seafloor induced via protracted interaction of SMS with oxidizing seawater when hydrothermal activity ceases temporarily (inactivity) or permanently (extinction).
- Decipher the potential toxicity producing changes of fine SMS-bearing hydrothermal vent−detritus dispersed in the oxidizing seawater column by eruptive or landslide disturbances, i.e., submarine eruptions, steam-blast eruptions, failure of hydrothermally weakened volcanic edifices etc.
- Investigate the partitioning of volatile trace elements (As, Ag, Hg, Sb, Pb, Tl) between hydrothermal vent fluids and sulfide and sulfosalt minerals, and analysis of the fundamental controls of trace element fixing in modern SMS minerals.
- Constrain the rate of magmatic inputs using the geochemical features of the vented fluids.
- Follow the circulation of hydrothermal fluids in fractures and conduits using acoustic data.
While some of the above scientific objectives are shared across existing submarine observatories, SANTORY is unique in that it focuses on a summit crater of an active submarine volcano at a shallow water depth where continuous phase separation is taking place, and where phreatomagmatic processes can have significant impact in nearby areas. In addition to monitoring and sampling of volcanic gases, the dangers of shallow magmatic-hydrothermal activity, as recently observed in Tonga, will be explicitly addressed.
Establishing the nature of the threat will be achieved by observing long- and short-term fluctuations in (i) the thermo-barometric conditions of the hydrothermal system; (ii) hydrothermal influences on seawater (bio) geochemistry, and (iii) changing subseafloor permeability. The pressure gauges coupled with tiltmeters and an array of benchmarks will be the first geodetic network to monitor ground movements in this type of setting that can be compared to fluid and gas fluxes. Quantification of the budget of CO2 emitted from the vents coupled to the time variability of selected key tracers of magmatic degassing, will be compared with other active hydrothermal systems on Earth.
Furthermore, gases emitted from the degassing vents are made of nearly pure CO2 that dissolves in seawater within 10 meters above the vent (Carey et al., 2013). Therefore, the water column above the bottom of Kolumbo crater contains dissolved volcanic gases (mostly CO2, H2S, CH4, H2, CO, noble gases) with a concentration that progressively decreases toward the ocean surface.
Until the SANTORY observatory, gases emitted from Kolumbo have only been sampled sporadically, limiting the possibility of recognizing changes in the state of activity of the volcano. A higher frequency of sampling, as planned during this project, will allow a temporal monitoring of some key geochemical parameters to provide information on possible magma recharges at depth. These are: i) the flux of CO2 emitted from the crater vents, which is known to increase days to weeks before volcanic unrest, for example as observed at Stromboli volcano (Inguaggiato et al., 2011). A higher degassing of CO2 would also induce a lowering of the pH in hydrothermal waters and in the water column above the vents; ii) the 3He/4He ratio is known to increase months before an eruption starts, due to the intrusion from the mantle into the crustal plumbing system of a volcano of more primitive and 3He-rich magma batches. This behavior has been observed in many volcanoes on Earth (e.g., Etna, Rizzo et al., 2006; Stromboli, Rizzo et al., 2015; Turrialba, Rizzo et al., 2016b; Ontake, Sano et al., 2015) and would be expected also for Kolumbo in case of its reactivation; iii) temperatures and pressures within the hydrothermal system are expected to increase weeks before an unrest phase starts, as observed in 2002 before and during the submarine degassing crisis of Panarea volcano (e.g., Caliro et al., 2004).
Furthermore, in concert with abrupt changes in the physical-chemical properties of seawater caused by volcanic discharge, we need to sample the hydrothermal plumes above and in the near-field of the KHV in order to understand the potential toxicity-producing modification processes of fine SMS-bearing vent−detritus, which may be released and dispersed in the seawater column via episodic plumes, which may be caused by eruptive or landslide disturbances, i.e., submarine eruptions, steam-blast eruptions, failure of hydrothermally weakened volcanic edifices etc. (e.g., El Hierro) (Fraile-Nuez et al., 2012). Hydrothermal plume sampling protocols and analytical methods are detailed in Kleint et al. (2022).
Detailed study of actively forming to mature extinct SMS, and episodic plumes of fine SMS-bearing vent−detritus, is therefore needed to give us new and temporally constrained insights into these processes on the seafloor and in the water column, particularly the fate of environmentally hazardous SMS-derived potentially toxic, metal(loid)s.
Shore-based studies will employ next-generation sequencing technologies to study the benthic communities in relation to possible changes in chemosynthetic energy sources from hydrothermal venting. Until the SANTORY observatory, sampling for microbial diversity was performed sporadically. Despite the vital role of microorganisms in hydrothermal vent ecosystems, only recently have bio-geochemical (Kilias et al., 2013; Christakis et al., 2018) and metagenomics and genomic investigations (Oulas et al., 2016; Mandalakis et al., 2019; Bravakos et al., 2021) been performed at Kolumbo volcano. These investigations revealed that both Kolumbo crater and Santorini caldera harbor highly complex prokaryotic communities (Oulas et al., 2016; Christakis et al., 2018) and microbes with an enhanced co-tolerance to acidity and antibiotics (Mandalakis et al., 2019; Bravakos et al., 2021). The observatory will enable microbiologists to locate and revisit specific microbiological features of the vent field, to study the microbial communities’ composition, structure and response to changes in the volcanic/hydrothermal system and to understand the physicochemical factors that shape the antibiotic resistome. These results will help to identify and forecast ecological changes of active submarine volcanic systems and will establish baselines and protocols for fast assessment of volcanic ecosystem diversity and structure.
Thus, a higher frequency of sampling for microbial communities within the framework of SANTORY observatory would allow a better understanding of a) how biodiversity and microbial communities’ stability are linked to chemosynthetic energy sources of HVs b) the extent to which extreme ecosystems may serve as reservoirs of resistance mechanisms, and c) the effect of bioleaching of sulfide minerals during oxidative weathering.
Scientific Impact
We expect to comprehend the links between deep-seated geological processes that have associated risks and their expression in the hydrothermal activity we monitor at the surface. In particular, our goal is to document the temporal variability of a dynamic system and identify significant events that cause changes in the behavior of the system. Hence monitoring, as proposed here, becomes a key component of risk assessment as already happened for the island of Panarea which is a tourist place similar to Santorini, but after the 2002 submarine explosion is monitored due to the large seasonal hazard variability.
At Panarea, coupling the results of periodical geochemical investigations with acoustic data recorded by the sea-floor observatory, it was possible to detect how the deep magma chamber of Stromboli volcano is responsible for the activity changes of the hydrothermal system (Heinicke et al., 2009; Longo et al., 2021a). With this background the data provided by SANTORY observatory will gain a better insight into the dynamics of the hydrothermal system and their relationships with changes of the deep magmatic activity with a positive impact on the risk mitigation for the Santorini area.
There will be a strong exchange of information between SANTORY and the upcoming IODP Expedition 398: Hellenic Arc Volcanic Field on-board the JOIDES Resolution from December 5 2022 to February 6 2023 (Druitt et al., 2022). The Expedition 398 will help SANTORY as:
1. Drilling on the flanks of Kolumbo will provide samples of quenched magma from the different Kolumbo eruptions. The phenocrysts will contain melt inclusions that can be analysed for volatile elements and trace metals. The samples will thus give a database of the contents and compositions of magmatic volatiles, metal and metalloids in the magmas that are the source of the fluids emitted in the crater. The samples will include the magmas of intermediate to silicic composition that are erupted in quantity, but it is possible that some basalts feeding the Kolumbo magmatic system are also sampled, either as lava, scoria or mafic inclusions in silicic magmas.
2. Drilling will also tell us about the nature of the Kolumbo eruptions and the hazards from them. As such, fusion of the drill data with the observatory data will give us a full picture of the state of the volcano in different regimes (Plinian, inter-plinian) to input into hazard and risk assessments.
3. Drilling at Kameni will allow us to compare and contrast the two systems.
● Why is there this large heat and fluid flow at Kolumbo but not at Kameni. Are fluids somehow channeled NE-wards towards Kolumbo?
● How do the fluid compositions and hydrothermal processes compare and contrast?
● How do the biospheres at Kameni and Kolumbo compare and contrast, and why?
Moreover, project outcomes will benefit scientific research at an international level by complementing and enriching monitoring practices developed in other world regions with different depths e.g. the shallower (<100 m b.s.l.) hydrothermal system of the Panarea volcano (Aeolian volcanic arc, Italy) (Heinicke et al., 2009; Longo et al., 2021b and references therein), where in the last decade a multi-parametric system of sensors has been deployed to monitor seafloor activities. In the mainframe of the EMSO-ERIC initiatives, a Panarea-like cabled observatory, would be the next step for the research team of SANTORY. Foreseen synergies will be established or strengthened in the framework of SANTORY with the ones with top–rank, internationally leading institutes that already have established scientific activity and/or have executed cruise expeditions successfully relating to marine geohazards in Mediterranean. SANTORY will additionally linked with complementary projects, such as the EU H2020 Pathfinder RAMONES which aims at investing on novel robotics capabilities for the exploration of the marine ecosystems (Mertzimekis et al., 2021).
Social Impact
SANTORY plans to: i) Establish a monitoring protocol and advise policy makers on scenario planning and possible strategies for hazard mitigation in underwater volcanic systems; ii) Invest on open–access data by creating an Open Data Hub for keeping local citizens, visitors and scientists informed about potential hazards related to submarine volcanoes and associated shallow hydrothermal vents; iii) Educate the general public and disseminate scientific information via outreach activities; iv) Train early–career researchers and students; v) Develop and integrate innovative monitoring technologies to promote surveillance of submarine arc volcanic areas located close to Mediterranean touristic islands.
The SANTORY Open Data Hub will implement a user-friendly, cross-platform and open-source toolkit (with algorithms and applications) that is integrated closely with the acquired datasets and exploits emerging visualization tools. The SANTORY Open Data Hub will be linked to major initiatives, such as the EMSO, the U.S. National Science Foundation’s Ocean Observatory Initiative (OOI) and the Ocean Networks Canada observatory to encourage collaborations in infrastructure, architecture, and interoperability. Moreover, it will develop different strategies and protocols for underwater hazard monitoring targeting specialist and non–specialist audiences. Finally, SANTORY will provide scientists, policymakers and stakeholders at all levels (local, national and EU/International) with data for interregional monitoring protocols, hazard warning codes, services to the local authorities and the public and guidance for mitigating societal impacts (e.g. timely evacuation) of natural hazards for populated areas. SANTORY will be a novel communication platform, using virtual and augmented reality and mobile platforms to promote the fascinating world of active underwater volcanic ecosystems on the EU’s shores.
The Future
SANTORY will be a reliable source of novel new data regarding the links between deep-seated geological processes that have associated hazard risks and their expression in the hydrothermal activity we monitor at the surface. More in particular, our goal is to establish the expected temporal variability of a dynamic system vs significant events that indicate changes in the fundamental behavior of the system. Hence monitoring, as proposed here, becomes a key component of risk assessment. In addition, reference monitoring protocols in the sense of combining active volcano measurements with Santorini’s on-land data (e.g., seismic, geodetic, geochemical), will provide the necessary impetus for understanding the long–term threat and developing novel risk assessment mechanisms.
The cataclysmic eruption of Hunga Tonga–Hunga Ha’apai submarine volcano, unlike anything seen in the modern scientific era, destroyed Tonga on 15 January 2022, and has shown that submarine volcanoes will continue to pose a hazard (Witze, 2022). Submarine volcanoes are understudied, therefore SANTORY is a largely hoped for addition to an emerging technological tendency, demonstrated by the installation of submarine cabled observatories in Mozambique, Japan, Taiwan Norway, China, and Canada, as well as the Mediterranean (Delaney and Kelley, 2015; Trowbridge et al., 2019; Feuillet et al., 2021). A cabled volcanic observatory, such as the NSF-funded observation OOI at Axial seamount, at Santorini, would be the next step for the research team of SANTORY.
We envision the future development of a network of similar seafloor observatories in the Mediterranean offering a new perspective for oceanography and ocean management in the region.
Data Availability Statement
The raw data supporting the conclusions of this article will be made available by the authors, without undue reservation.
Author Contributions
PN, PP, AR, SP, MH, SK, DP, JE, KK, TM, VA, MK, LG, and FI conceived the study. DL helped in figures preparation. All of the authors contributed to the preparation and editing of the final manuscript. All authors contributed to the article and approved the submitted version.
Conflict of Interest
The authors declare that the research was conducted in the absence of any commercial or financial relationships that could be construed as a potential conflict of interest.
Publisher’s Note
All claims expressed in this article are solely those of the authors and do not necessarily represent those of their affiliated organizations, or those of the publisher, the editors and the reviewers. Any product that may be evaluated in this article, or claim that may be made by its manufacturer, is not guaranteed or endorsed by the publisher.
Acknowledgments
The SANTORY program is funded by the Hellenic Foundation for Research and Innovation (HFRI) (Grant Number 1850) in the framework of the “1st Announcement of Research Projects HFRI for Faculty Members and researchers and the supply of high-value research equipment” with a duration of three years. TM acknowledges support by RAMONES (EU H2020 Pathfinder Grant No 101017808). We thank William W. Chadwick and Tim Druitt for their comments and feedback on an earlier version of the paper. We thank the Chief Editor PF for handling and revising the manuscript, PM and a reviewer for suggestions that greatly improved the manuscript.
References
Barreyre T., Escartín J., Garcia R., Cannat M., Mittelstaedt E. (2012). Structure, Temporal Evolution, and Heat Flux Estimates From the Lucky Strike Deep-Sea Hydrothermal Field Derived From Seafloor Image Mosaics. Geochem. Geophys. Geosyst. 13, Q04007. doi: 10.1029/2011GC003990
Barreyre T., Escartín J., Sohn R. A., Cannat M., Ballu V., Crawford W. C. (2014). Temporal Variability and Tidal Modulation of Hydrothermal Exit-Fluid Temperatures at the Lucky Strike Deep-Sea Vent Field, Mid-Atlantic Ridge. J. Geophys. Res.: Solid Earth 119 (4), 2543–2566. doi: 10.1002/2013JB010478
Barreyre T., Olive J.-A., Crone T. J., Sohn R. A. (2018). Depth-Dependent Permeability and Heat Output at Basalt-Hosted Hydrothermal Systems Across Mid-Ocean Ridge Spreading Rates. Geochem. Geophysics Geosystems 19, 1259– 1281. doi: 10.1002/2017GC007152
Barreyre T., Parnell-Turner R., Wu J.-N., Fornari D. J. (2022). Tracking Crustal Permeability and Hydrothermal Response During Seafloor Eruptions at the East Pacific Rise, 9°50’n. Geophys. Res. Lett. 49 (3). doi: 10.1029/2021GL095459
Beaulieu S. E., , Graedel T. E., , Hannington M. D. (2017). Should We Mine the Deep Seafloor? Earth’s Future 5, 655–658. doi: 10.1002/2017EF000605
Best M., Favali P., Beranzoli L. (2014). EMSO: A Distributed Infrastructure for Addressing Geohazards and Global Ocean Change. Oceanography 27 no. 2, 167–169. doi: 10.5670/oceanog.2014.52
Bravakos P., Mandalakis M., Nomikou P., Anastasiou T. I., Kristofferen J. B., Stavroulaki M., et al. (2021). Genomic Adaptation of Pseudomonas Strains to Acidity and Antibiotics in Hydrothermal Vents at Kolumbo Submarine Volcano, Greece. Nat. Sci. Rep. 11, 1336. doi: 10.1038/s41598-020-79359-y
Butterfield D. A., Jonasson I. R., Massoth G. J., Feely R. A., Roe K. K., Embley R. W., et al. (1997). Seafloor Eruptions and Evolution of Hydrothermal Fluid Chemistry, Phil. Trans. R. Soc Lond. A 355, 369–386. doi: 10.1098/rsta.1997.0013
Butterfield D. A., Massoth G. J., McDuff R. E., Lupton J. E., Lilley M. D. (1990). Geochemistry of Hydrothermal Fluids From Axial Seamount Hydrothermal Emissions Study Vent Field, Juan De Fuca Ridge: Subseafloor Boiling and Subsequent Fluid-Rock Interaction. J. Geophys. Res. 95, 12,895 – 12,921. doi: 10.1029/JB095iB08p12895
Cabaniss H. E., Gregg P. M., Nooner S. L., Chadwick W. W. (2020). Triggering of Eruptions at Axial Seamount, Juan De Fuca Ridge. Sci. Rep. 10 no. 1, 10219. doi: 10.1038/s41598-020-67043-0
Caliro S., Caracausi A., Chiodini G., Ditta M., Italiano F., Longo M., et al. (2004). Evidence of a Recent Input of Magmatic Gases Into the Quiescent Volcanic Edifice of Panarea, Aeolian Islands, Italy, Geophys. Res. Lett. 31, L07619. doi: 10.1029/2003GL019359
Cantner K., Carey S., Nomikou P. (2014a). Integrated Volcanologic and Petrologic Analysis of the 1650AD Eruption of Kolumbo Submarine Volcano, Greece. J. Volcanol. Geothermal Res. 269, 28–43. doi: 10.1016/j.jvolgeores.2013.10.004
Capaccioni B., Tassi F., Vaselli O., Tedesco D., Poreda R. (2007). Submarine Gas Burst at Panarea Island (Southern Italy) on 3 November 2002: A Magmatic Versus Hydrothermal Episode. J. Geophys. Res. 112, B05201. doi: 10.1029/2006JB004359
Caracausi A., Ditta M., Italiano F., Longo M., Nuccio P. M., Paonita A. (2005b). Massive Submarine Gas Output During the Volcanic Unrest Off Panarea Island (Aeolian Arc, Italy): Inferences for Explosive Conditions. Geochemical J. 39 (5), 449–467. doi: 10.2343/geochemj.39.459
Caracausi A., Ditta M., Italiano F., Longo M., Nuccio P. M., Paonita A., et al. (2005a). Changes in Fluid Geochemistry and Physico-Chemical Conditions of Geothermal Systems Caused by Magmatic Input: The Recent Abrupt Outgassing Off the Island of Panarea, Aeolian Islands, Italy. Geochim. Cosmochim. Acta 69, 3045–3059. doi: 10.1016/j.gca.2005.02.011
Carey S., Bell K. L. C., Nomikou P., Vougioukalakis G., Roman C. N., Cantner K., et al. (2011). “Exploration of the Kolumbo Volcanic Rift Zone,” in New Frontiers in Ocean Exploration: The E/V Nautilus 2010 Field Season, vol. 24 . Eds. Bell K. L. C., Fuller S. A. (Rockville, MD, USA: Oceanography), 24–25. supplement. doi: 10.5670/oceanog.24.1.supplement
Carey S., Nomikou P., Bell K. C., Lilley M., Lupton J., Roman C., et al. (2013). CO2 Degassing From Hydrothermal Vents at Kolumbo Submarine Volcano, Greece, and the Accumulation of Acidic Crater Water. Geology 41, 1035–1038. doi: 10.1130/G34286.1
Chadwick W. W. Jr., Wilcock W. S. D., Nooner S. L., Beeson J. W., Sawyer A. M., Lau T.-K. (2022). Geodetic Monitoring at Axial Seamount Since its 2015 Eruption Reveals a Waning Magma Supply and Tightly Linked Rates of Deformation and Seismicity, Geochem. Geophys. Geosyst. 22, e2021GC010153. doi: 10.1029/2021GC010153
Chiodini G., Caliro S., Caramanna G., Granieri D., Minopoli C., Moretti R., et al. (2006). Geochemistry of the Submarine Gaseous Emissions of Panarea (Aeolian Islands, Southern Italy): Magmatic vs. Hydrothermal Origin and Implications for Volcanic Surveillance. Pure Appl. Geophys. 163, 759–780. doi: 10.1007/s00024-006-0037-y
Christakis C. A., Polymenakou P. N., Mandalakis M., Nomikou P., Kristoffersen J. B., Lampridou D., et al. (2018). Microbial Community Differentiation Between Active and Inactive Sulfide Chimneys of the Kolumbo Submarine Volcano, Hellenic Volcanic Arc. Extremophiles: Life Under Extreme Conditions 22 (1), 13–27. doi: 10.1007/s00792-017-0971-x
Christopoulou M. E., Mertzimekis T. J., Nomikou P., Papanikolaou D., Carey S., Mandalakis M. (2016). Influence of Hydrothermal Venting on Water Column Properties in the Crater of the Kolumbo Submarine Volcano, Santorini Volcanic Field (Greece). Geo-Marine Lett. 36 (1), 15–24. doi: 10.1007/s00367-015-0429-z
Chrousos G. P., Mentis A. F. A., Dardiotis E. (2020). Biomedical Research: Lessons From the Last Decade’s Crisis and Austerity-Stricken Small Countries for the Current COVID-19-Related Crisis. Nat. Med. 26 (5), 644–646. doi: 10.1038/s41591-020-0859-7
Colaço A., Blandin J., Cannat M., Carval T., Chavagnac V., Connelly D., et al. (2011). Momar-D: A Technological Challenge to Monitor the Dynamics of the Lucky Strike Vent Ecosystem. ICES J. Marine Sci. 68 no. 2, 416– 424. doi: 10.1093/icesjms/fsq075
Crisp J. A. (1984). Rates of Magma Emplacement and Volcanic Output. J. Volcanol. Geotherm. Res. 20, 177–211. doi: 10.1016/0377-0273(84)90039-8
Crone T. J., Wilcock S. D. (2005). Modeling the Effects of Tidal Loading on Mid-Ocean Ridge Hydrothermal Systems. Geochem. Geophysics Geosystems 6 no. 7, Q07001. doi: 10.1029/2004GC000905
Dando P. R., Aliani S., Arabj H., Bianchi C. N., Brehmeg M., Cocito S., et al. (2000). Hydrothermal Studies in the Aegean Sea. Phys. Chem. Earth 25, 1–8. doi: 10.1016/S1464-1909(99)00112-4
Dando P. R., Hughes J. A., Leahy Y., Niven S. J., Taylor L. J., Smith C. (1995). Gas Venting Rates From Submarine Hydrothermal Areas Around the Island of Milos, Hellenic Volcanic Arc. Continental Shelf Res. 15 (8), 913–929. doi: 10.1016/0278-4343(95)80002-U
Daskalopoulou K., D’Alessandro W., Longo M., Pecoraino G., Calabrese S.. (2005). Shallow Sea Gas Manifestations in the Aegean Sea (Greece) as Natural Analogues to Study Ocean Acidification: First Catalogue and Geochemical Characterization. Front. Marine Sci. doi: 10.3389/fmars.2021.775247
Delaney J. R., Kelley D. (2015). “Next Generation Science in the Ocean Basins: Expanding the Oceanographer’s Toolbox Utilizing Electro-Optical Submarine Networks,” in Seafloor Observatories: A New Vision of the Earth From the Abyss. Eds. Favali P., Beranzoli L., De Santis A. (Berlin: Springer-Praxis Publishing).
de Ronde C. E. J., Hannington M. D., Stoffers P., Wright I. C., Ditchburn R. G., Reyes A. G., et al. (2005). Evolution of a Submarine Magmatic-Hydrothermal System: Brothers Volcano, Southern Kermadec Arc, New Zealand. Economic Geol. 100, 1097–1133. doi: 10.2113/gsecongeo.100.6.1097
de Ronde C. E. J., Humphris S. E., Höfig T. W., Brandl P. A., Cai L., Cai Y., et al. (2019). “Expedition 376 Summary,” in Proceedings of the International Ocean Discovery Program, vol. 376 . Eds. de Ronde C. E. J., Humphris S. E., Höfig T. W. (College Station, TX: International Ocean Discovery Program). the Expedition 376 Scientists, (2019) Brothers Arc Flux. doi: 10.14379/iodp.proc.376.101.2019
Dimitriadis I., Karagianni E., Panagiotopoulos D., Papazachos C., Hatzidimitriou P., Bohnhoff M., et al. (2009). Seismicity and Active Tectonics at Coloumbo Reef (Aegean Sea, Greece): Monitoring an Active Volcano at Santorini Volcanic Center Using a Temporary Seismic Network. Tectonophysics 465, 136–149. doi: 10.1016/j.tecto.2008.11.005
Druitt T. H., Edwards L., Mellors R. M., Pyle D. M., Sparks R. S. J., Lanphere M., et al. (1999). Santorini Volcano Vol. 19165 (London, UK: Geological Society of London, Memoirs).
Druitt T., Kutterolf S., Höfig T. W. (2022). Expedition 398 Scientific Prospectus: Hellenic Arc Volcanic Field. Int. Ocean Discovery Program. doi: 10.14379/iodp.sp.398.2022
Escartin J., Barreyre T., Cannat M., Garcia R., Gracias N., Deschamps A. (2015). Hydrothermal Activity Along the Slow-Spreading Lucky Strike Ridge Segment (Mid-Atlantic Ridge): Distribution, Heatflux, and Geological Controls. Earth Planetary Sci. Lett. 431, 173–185. doi: 10.1016/j.epsl.2015.09.025
Esposito M., Tse T., Soufani K. (2018). Introducing a Circular Economy: New Thinking With New Managerial and Policy Implications. California Manage. Rev. 60 (3), 5–19. doi: 10.1177/0008125618764691
Evans G. N., Tivey M. K., Monteleone B., Shimizu N., Seewald J. S., Rouxel O. J. (2020). Trace Element Proxies of Seafloor Hydrothermal Fluids Based on Secondary Ion Mass Spectrometry (SIMS) of Black Smoker Chimney Linings. Geochimica Cosmochimica Acta 269, 346–375. doi: 10.1016/j.gca.2019.09.038
Fabian M., Villinger H. (2008). The Bremen Ocean Bottom Tiltmeter (OBT) - a Technical Article on a New Instrument to Monitor Deep Sea Floor Deformation and Seismicity Level. Mar. Geophys. Res. 28, 13–26. doi: 10.1007/s11001-006-9011-4
Fallon E. K., Frische M., Petersen S., Brooker R. A., Scott T. B. (2019). Geological, Mineralogical and Textural Impacts on the Distribution of Environmentally Toxic Trace Elements in Seafloor Massive Sulfide Occurrences. Minerals 9, 162. doi: 10.3390/min9030162
Fallon E. K., Niehorster E., Brooker R. A., Scott T. B. (2018). Experimental Leaching of Massive Sulphide From TAG Active Hydrothermal Mound and Implications for Seafloor Mining. Marine Pollution Bull. 126, 501–515. doi: 10.1016/j.marpolbul.2017.10.079
Fallon E. K., Petersen S., Brooker R. A., Scott T. B. (2017). Oxidative Dissolution of Hydrothermal Mixed-Sulphide Ore: An Assessment of Current Knowledge in Relation to Seafloor Massive Sulphide Mining. Ore Geol. Rev. 86, 309–337. doi: 10.1016/j.oregeorev.2017.02.028
Feuillet N., Jorry S., Crawford W. C., Deplus C., Thinon I., Jacques E., et al. (2021). Birth of a Large Volcanic Edifice Offshore Mayotte via Lithosphere-Scale Dyke Intrusion. Nat. Geosci. 14, 787–795. doi: 10.1038/s41561-021-00809-x
Fraile-Nuez E., González-Dávila M., Santana-Casiano J. M., Arístegui J., Alonso-González I. J., Hernández-León S., et al. (2012). The Submarine Volcano Eruption at the Island of El Hierro: Physical-Chemical Perturbation and Biological Response. Sci. Rep. 2, 486. doi: 10.1038/srep00486
Fuchida S., Yokoyama A., Fukuchi R., Ishibashi J., Kawagucci S., Kawachi M., et al. (2017). Leaching of Metals and Metalloids From Hydrothermal Ore Particulates and Their Effects on Marine Phytoplankton. ACS Omega 7, 3175–3182. doi: 10.1021/acsomega.7b00081
Fuller S., Carey S., Nomikou P. (2018). Distribution of Fine-Grained Tephra From the 1650 CE Submarine Eruption of Kolumbo Volcano, Greece. J. Volcanol. Geothermal Res. 352, 10. doi: 10.1016/j.jvolgeores.2018.01.004
Gartman A., Findlay A. J., Hannington M., Garbe-Schönberg D., Jamieson J. W., Kwasnitschka T. (2019). The Role of Nanoparticles in Mediating Element Deposition and Transport at Hydrothermal Vents. Geochimica Cosmochimica Acta 261, 113–131. doi: 10.1016/j.gca.2019.06.045
Gartman A., Hannington M., Jamieson J. W., Peterkin B., Garbe-Schönberg D., Findlay A. J., et al. (2018). Boiling-Induced Formation of Colloidal Gold in Black Smoker Hydrothermal Fluids. Geology 46 (1), 39–42. doi: 10.1130/G39492.1
Hannington M. D. (Ed.) (2018). “RV POSEIDON Fahrtbericht/Cruise Report POS510 - ANYDROS: Rifting and Hydrothermal Activity in the Cyclades Back-Arc Basin, Catania (Italy) – Heraklion (Greece) 06.03.-29.03.2017,” in Geomar Report, N. Ser. 043 (Kiel, Germany: GEOMAR Helmholtz-Zentrum für Ozeanforschung). 56 + Appendix. doi: 10.3289/geomar_rep_ns_43_2018
Hannington M. D., de Ronde C. E. J., Petersen S. (2005). Sea-Floor Tectonics and Submarine Hydrothermal Systems: Economic Geology 100th Anniversary (Washington DC: Society of Economic Geologists). 111–142, Volume.
Hannington M., Garbe-Schönberg D. (2019). Detection of Gold Nanoparticles in Hydrothermal Fluids. Economic Geol. 114 (2), 397–400. doi: 10.5382/econgeo.2019.4636
Hannington M., Jamieson J., Monecke T., Petersen S., Beaulieu S. (2011). The Abundance of Seafloor Massive Sulfide Deposits. Geology 39 (12), 1155–1158. doi: 10.1130/G32468.1
Hauton C., Brown A., Thatje S., Mestre N. C., Bebianno M. J., Martins I., et al. (2017). Identifying Toxic Impacts of Metals Potentially Released During Deep-Sea Mining—a Synthesis of the Challenges to Quantifying Risk. Front. Mar. Sci. 4. doi: 10.3389/fmars.2017.00368
Hawkes J., Rossel P., Stubbins A., Butterfield D., Connelly D. P., Achterberg E. P., et al. (2015). Efficient Removal of Recalcitrant Deep-Ocean Dissolved Organic Matter During Hydrothermal Circulation. Nat. Geosci 8, 856–860. doi: 10.1038/ngeo2543
Heinicke J., Fischer T., Gaupp R., Götze J., Koch U., Konietzky H., et al. (2009). Hydrothermal Alteration as a Trigger Mechanism for Earthquake Swarms: The Vogtland/NW Bohemia Region as a Case Study. Geophys. J. Int. 1782009 (1), 1–13. doi: 10.1111/j.1365-246X.2009.04138.x
Hooft E. E. E., Nomikou P., Toomey D. R., Lampridou D., Getz C., Christopoulou M.-E., et al. (2017). Backarc Tectonism, Volcanism, and Mass Wasting Shape Seafloor Morphology in the Santorini-Christiana-Amorgos Region of the Hellenic Volcanic Arc. Tectonophysics 712-713, 396. doi: 10.1016/j.tecto.2017.06.005
Hwa Oh Y., Kim G. (2015). A Radon-Thoron Isotope Pair as a Reliable Earthquake Precursor. Sci. Rep. 5, 13084. doi: 10.1038/srep13084
Inguaggiato S., Vita F., Rouwet D., Bobrowski N., Morici S., Sollami A. (2011). Geochemical Evidence of the Renewal of Volcanic Activity Inferred From CO2 Soil and SO2 Plume Fluxes: The 2007 Stromboli Eruption (Italy) Bullettin of Volcanology. International Association of Volcanology and Chemistry of the Earth’s Interior (IAVCEI) 2007 , Vol. 73 Number 4. 443–456.
Jamieson J. W., Hannington M. D., Clague D. A., Kelley D. S., Delaney J. R., Holden J. F., et al. (2013). Sulfide Geochronology Along the Endeavour Segment of the Juan De Fuca Ridge. Geochem. Geophys. Geosyst 14, 2084– 2099. doi: 10.1002/ggge.20133
Kandylakis Z., Karantzalos K., Doulamis A., Doulamis N. (2015). “Multiple Object Tracking With Background Estimation in Hyperspectral Video Sequences,” in IEEE Workshop on Hyperspectral Image and Signal Processing: Evolution in Remote Sensing (WHISPERS).
Kelley D., Delaney J. R., Juniper S. K. (2014). Establishing a New Era of Submarine Volcanic Observatories: Cabling Axial Seamount and the Endeavour Segment of the Juan De Fuca Ridge. Marine Geol. 352, 426–450. doi: 10.1016/j.margeo.2014.03.010
Kilias S. P., Gousgouni M., Godelitsas A., Gamaletsos P., Mertzimekis T. J., Nomikou P., et al. (2016). Antimony Fixation in Solid Phases at the Hydrothermal Field of Kolumbo Submarine Arc-Volcano (Santorini): Deposition Model and Environmental Implications. Bull. Geol. Soc Greece 50 (4), 2200. doi: 10.12681/bgsg.14276
Kilias S. P., Nomikou P., Papanikolaou D., Polymenakou P. N., Godelitsas A., Argyraki A., et al. (2013). New Insights Into Hydrothermal Vent Processes in the Unique Shallowsubmarine Arc-Volcano, Kolumbo (Santorini), Greece. Sci. Rep. 3, 2421. doi: 10.1038/srep02421
Kilias S. P., Zygouri E., Nomikou P., Chrysafeni M., Ivarsson M., Chi Fru E., et al. (2017). Metal Resource Potential of Modern Sea-Floor Massive Sulfides at Kolumbo Shallow-Submarine Arc Volcano (Santorini), Greece. Goldschmidt2017 Abstracts, Paris 2017, August 13-18.
Kilias S. P., Zygouri E., Zegkinoglou N., Nomikou P., Keith M., Zack T., et al. (2022). “Fluid-Mediated Coupled Dissolution-Reprecipitation (CDR) Reaction Drives Gold Remobilization in Shallow-Water Massive Sulfides at the Kolumbo Arc-Volcano, Greece,” in Proceedings of the 16th SGA Biennial Meeting, 28-31 March (Rotorua, New Zealand: Society for Geology Applied to Mineral Deposits). Eds.
Klaver M., Carey S., Nomikou P., Smet I., Godelitsas A., Vroon P. (2016). A Distinct Source and Differentiation History for Kolumbo Submarine Volcano, Santorini Volcanic Field, Aegean Arc. Geochem. Geophysics Geosystems 17 (8), 3254. doi: 10.1002/2016GC006398
Kleint C., Zitoun R., Neuholz R., Walter M., Schnetger B., Klose L., et al. (2022). Trace Metal Dynamics in Shallow Hydrothermal Plumes at the Kermadec Arc. Front. Marine Sci. Volume 8. doi: 10.3389/fmars.2021.782734
Klose L., Keith M., Hafermaas D., Kleint C., Bach W., Diehl A., et al. (2021). Trace Element and Isotope Systematics in Vent Fluids and Sulphides From Maka Volcano, North Eastern Lau Spreading Centre: Insights Into Three-Component Fluid Mixing. Front. Earth Sci. 9. doi: 10.3389/feart.2021.776925
Lan T. F., Sano Y., Yang T. F., Takahata N., Shirai K., Pinti D. (2010). Evaluating Earth Degassing in Subduction Zones by Measuring Helium Fluxes From the Ocean Floor. Earth Planet. Sci. Lett. 289, 317–322. doi: 10.1016/j.epsl.2010.07.049
Lilley M. D., Butterfield D. A., Olson E. J., Lupton J. E., Macko S. A., McDuff R. E. (1993). Anomalous CH4 and NH4+ Concentrations at an Unsedimented Mid-Ocean-Ridge Hydrothermal System. Nature 364, 45–47. doi: 10.1038/364045a0
Longo M., Lazzaro G. C., Caruso G., Corbo A., Sciré Scappuzzo S., Italiano F. (2021a). Hydro-Acoustic Signals From the Panarea Shallow Hydrothermal Field: New Inferences of a Direct Link With Stromboli. Geol. Soc. Spec. Publ. (2021) SP519-2020-184. doi: 10.1144/SP519-2020-184
Longo M., Lazzaro G., Caruso G. C., Radulescu V., Radulescu R., SciréScappuzzo S. S., et al. (2021b). Black Sea Methane Flares From the Seafloor: Tracking Outgassing by Using Passive Acoustics. Front. Earth Sci. 9, 678834. doi: 10.3389/feart.2021.678834
Lupton J. E. (1990). Water Column Hydrothermal Plumes on the Juan De Fuca Ridge. J. Geophys. Res. 95 (b8), 12,829–12,842. doi: 10.1029/JB095iB08p12829
Lupton J. E., Arculus R. J., Greene R. R., Evans L. J., Goddard C. I. (2009). Helium Isotope Variations in Seafloor Basalts From the Northwest Lau Backarc Basin: Mapping the Influence of the Samoan Hotspot. Geophys. Res. Lett. 36, L17313. doi: 10.1029/2009GL039468
Lupton J. E., Baker E. T., Massoth G. J. (1999). Helium, Heat, and the Generation of Hydrothermal Event Plumes at Mid-Ocean Ridges. Earth Planetary Sci. Lett. 171 (3), 343–350. doi: 10.1016/S0012-821X(99)00149-1
Lupton J., Butterfield D., Lilley M., Evans L., Ko-ichi N., Chadwick W., et al. (2006). Submarine Venting of Liquid Carbon Dioxide on a Mariana Arc Volcano. Geochem. Geophys. Geosyst 7, Q08007. doi: 10.1029/2005GC001152
Lupton J., Lilley M., Butterfield D., Evans L., Embley R., Massoth G., et al. (2008). Venting of a Separate CO2-Rich Gas Phase From Submarine Arc Volcanoes: Examples From the Mariana and Tonga-Kermadec Arcs. J. Geophys. Res. 113, B08S12. doi: 10.1029/2007JB005467
Mandalakis M., Gavriilidou A., Polymenakou P., Christakis C., Nomikou P., Medvecky M., et al. (2019). Microbial Strains Isolated From CO2-Venting Kolumbo Submarine Volcano Show Enhanced Co-Tolerance to Acidity and Antibiotics. Marine Environ. Res. 144, 102–110. doi: 10.1016/j.marenvres.2019.01.002
Mei K., Wang D., Jiang Y., Shi M., Chen C.-T. A., Zhang Y., et al. (2022). Transformation, Fluxes and Impacts of Dissolved Metals From Shallow Water Hydrothermal Vents on Nearby Ecosystem Offshore of Kueishantao (NE Taiwan). Sustainability 14, 1754. doi: 10.3390/su14031754 2022.
Mertzimekis T. J., Nomikou P., Petra E., Batista P., Cabecinhas D., Pascoal A., et al. (2021). “Radioactivity Monitoring in Ocean Ecosystems (RAMONES),” in Proceedings of the Conference on Information Technology for Social Good (Goodit ‘21) (New York, NY, USA: Association for Computing Machinery), 216–220. doi: 10.1145/3462203.3475906
Monecke T., Petersen S., Hannington M. D. (2014). Constraints on Water Depth of Massive Sulfide Formation: Evidence From Modern Seafloor Hydrothermal Systems in Arc-Related Settings. Economic Geol. 109 (8), 2079–2101. doi: 10.2113/econgeo.109.8.2079
Neuholz R., Kleint C., Schnetger B., Koschinsky A., Laan P., Middag R., et al. (2020). Submarine Hydrothermal Discharge and Fluxes of Dissolved Fe and Mn, and He Isotopes at Brothers Volcano Based on Radium Isotopes. Minerals 10, 969. doi: 10.3390/min10110969
Nomikou P., Carey S., Papanikolaou D., Croff Bell K., Sakellariou D., Alexandri M., et al. (2012). Submarine Volcanoes of the Kolumbo Volcanic Zone NE of Santorini Caldera Greece. Glob. Planet. Change 9, 135–151. doi: 10.1016/j.gloplacha.2012.01.001
Nomikou P., Druitt T. H., Hübscher C., Mather T. A., Paulatto M., Kalnins L. M., et al. (2016). Post-Eruptive Flooding of Santorini Caldera and Implications for Tsunami Generation. Nat. Commun. 7, 13332. doi: 10.1038/ncomms13332
Nomikou P., Hannington M., Petersen S., Wind S., Heinath V., Lange S., et al. (2019b). “Advanced Mapping of Kolumbo Submarine Volcano (Santorini) Using AUV “Abyss,” in RCG2019 – Regional Conference of Geomorphology. (Athens Greece: International Association of Geomorphologists).
Nomikou P., Hübscher C., Carey S. (2019). The Christiana–Santorini–Kolumbo Volcanic Field. Elements; 15 (3), 171–176. doi: 10.2138/gselements.15.3.171
Nomikou P., Hübscher C., Papanikolaou D., Farangitakis P. G., Ruhnau M., Lampridou D. (2018). Expanding Extension, Subsidence and Lateral Segmentation Within the Santorini - Amorgos Basins During Quaternary: Implications for the 1956 Amorgos Events, Central - South Aegean Sea. Greece. Tectonophysics 722, 138–153. doi: 10.1016/j.tecto.2017.10.016
Nomikou P., Hübscher C., Ruhnau M., Bejelou K. (2016). Tectonophysics Tectono-Stratigraphic Evolution Through Successive Extensional Events of the Anydros Basin, Hosting Kolumbo Volcanic Field at the Aegean. Tectonophysics 671, 202–217. doi: 10.1016/j.tecto.2016.01.021
Nomikou P., Papanikolaou D., Alexandri M., Sakellariou D., Rousakis G. (2013). Submarine Volcanoes Along the Aegean Volcanic Arc. Tectonophysics 59, 123–146. doi: 10.1128/AEM.01835-08
Nooner S. L., Chadwick W. W. Jr. (2016). Inflation-Predictable Behavior and Co-Eruption Deformation at Axial Seamount. Science 354 (6318), 1399–1403. doi: 10.1126/science.aah4666
Oulas A., Polymenakou P. N., Seshadri R., Tripp H. J., Mandalakis M., Paez-Espino A. D., et al. (2016). Metagenomic Investigation of the Geologically Unique Hellenic Volcanic Arc Reveals a Distinctive Ecosystem With Unexpected Physiology. Environ. Microbiol. 18 (4), 1122. doi: 10.1111/1462-2920.13095
Petersen S., Monecke T., Westhues A., Hannington M. D., J. Gemmell J. B., Sharpe R., et al. (2014). Drilling Shallow-Water Massive Sulfides at the Palinuro Volcanic Complex, Aeolian Island Arc, Italy. Economic Geol 109 (8), 2129–2158. doi: 10.2113/econgeo.109.8.2129
Price R., Giovannelli D. (2017). A Review of the Geochemistry and Microbiology of Marine Shallow-Water Hydrothermal Vents. Reference Module in Earth Systems and Environmental Sciences (Elsevier) 1–29 doi: 10.1016/B978-0-12-409548-9.09523-3
Puzenat V., Escartín J., Martelat J.-E., Barreyre T., Moine Bauer S. L., Nomikou P., et al. (2021). Shallow-Water Hydrothermalism at Milos (Greece): Nature, Distribution, Heat Fluxes and Impact on Ecosystems. Marine Geol. 438, 106521. doi: 10.1016/j.margeo.2021.106521
Rizzo A. L., Caracausi A., Chavagnac V., Nomikou P., Polymenakou P. N., Mandalakis M., et al. (2016a). Kolumbo Submarine Volcano (Greece): An Active Window Into the Aegean Subduction System. Sci. Rep. 6, 28013. doi: 10.1038/srep28013
Rizzo A. L., Caracausi A., Chavagnac V., Nomikou P., Polymenakou P. N., Mandalakis M., et al. (2019). Geochemistry of CO2-Rich Gases Venting From Submarine Volcanism: The Case of Kolumbo (Hellenic Volcanic Arc, Greece). Front. Earth Sci. 7. doi: 10.3389/feart.2019.00060
Rizzo A., Caracausi A., Favara R., Martelli M., Nuccio P. M., Paonita A., et al. (2006). New Insights Into Magma Dynamics During Last Two Eruptions of Mount Etna as Inferred by Geochemical Monitoring From 2002 to 2005. Geochem. Geophys. Geosyst. 7, Q06008. doi: 10.1029/2005GC001175
Rizzo A. L., Di Piazza A., de Moor J. M., Alvarado G. E., Avard G., Carapezza M., et al. (2016b). Eruptive Activity at Turrialba Volcano (Costa Rica): Inferences From 3He/4He in Fumarole Gases and Chemistry of the Products Ejected During 2014 and 2015, Geochem. Geophys. Geosyst. 17, 4478–4494. doi: 10.1002/2016GC006525
Rizzo A. L., Federico C., Inguaggiato S., Sollami A., Tantillo M., Vita F., et al. (2015). The 2014 Effusive Eruption at Stromboli Volcano (Italy): Inferences From Soil CO2 Flux and 3He/4He Ratio in Thermal Waters. Geophys. Res. Lett. 42, 2235–2243. doi: 10.1002/2014GL062955
Sander S., Koschinsky A. (2011). Metal Flux From Hydrothermal Vents Increased by Organic Complexation. Nat. Geosci 4, 145–150. doi: 10.1038/ngeo1088
Sano Y., Kagoshima T., Takahata N., Nishio Y., Roulleau E., Pinti D., et al. (2015). Ten-Year Helium Anomaly Prior to the 2014 Mt Ontake Eruption. Sci. Rep. 5, 13069. doi: 10.1038/srep13069
Sigurdsson H., Carey S., Alexandri M., Vougioukalakis G., Croff K., Roman C., et al. (2006). Marine Investigations of Greece’s Santorini Volcanic Field. EOS Trans. Am. Geophys. Union 87, 337. doi: 10.1029/2006EO340001
Sohn R. A., Thomson R. E., Rabinovich A. B., Mihali S. F. (2009). Bottom Pressure Signals at the TAG Deep-Sea Hydrothermal Field: Evidence for Short-Period, Flow-Induced Ground Deformation. Geophys. Res. Lett. 36, L19301. doi: 10.1029/2009GL040006
Taran Y., Torokhov P., Pokrovski G., Shabayeva I. (1992). Isotopic Composition of Mineral Precipitates and Free Gas Associated With Hydrothermal Vents of Piip Submarine Volcano, Bering Sea. Geochem. J. 26, 291–297. doi: 10.2343/geochemj.26.291
Tassi F., Capaccioni B., Caramanna G., Cinti D., Montegrossi G., Pizzino L., et al. (2009). Low-Ph Waters Discharging From Submarine Vents at Panarea Island (Aeolian Islands, Southern Italy) After the 2002 Gas Blast: Origin of Hydrothermal Fluids and Implications for Volcanic Surveillance. Appl. Geochem. 24, 246–254. doi: 10.1016/j.apgeochem.2008.11.015
Tassi F., Capaccioni B., Vaselli O. (2014). Compositional Spatial Zonation and 2005–2013 Temporal Evolution of the Hydrothermal-Magmatic Fluids From the Submarine Fumarolic Field at Panarea Island. J. Volcanol. Geothermal Res. 277, 41–50. doi: 10.1016/j.jvolgeores.2014.03.010
Tassi F., Venturi S., Cabassi J., Capecchiacci F., Nisi B., Vaselli O. (2015). Volatile Organic Compounds (Vocs) in Soil Gases From Solfatara Crater (Campi Flegrei, Southern Italy): Geogenic Source(s) vs. Biogeochemical Processes. Appl. Geochem. 56, 37–49. doi: 10.1016/j.apgeochem.2015.02.005
Trowbridge J., Weller R., Kelley D., Dever E., Plueddemann A., Barth J. A., Kawka O.. (2019). The Ocean Observatories Initiative. Front. Marine Sci. 6, 74. doi: 10.3389/fmars.2019.00074
Tsunogai U., Ishibashia J., Wakitaa H., Gamob T., Watanabec K., Kajimurac T., et al. (1994). Peculiar Features of Suiyo Seamount Hydrothermal Fluids, Izubonin Arc: Differences From Subaerial Volcanism. Earth Planet. Sci. Lett. 126, 289–301. doi: 10.1016/0012-821X(94)90113-9
Ulvrova M., Paris R., Nomikou P., Kelfoun K., Leibrandt S., Tappin D. R., et al. (2016). Source of the Tsunami Generated by the 1650 AD Eruption of Kolumbo Submarine Volcano (Aegean Sea, Greece). J. Volcanol. Geothermal Res. 321, 125. doi: 10.1016/j.jvolgeores.2016.04.034
Vakalopoulou M., Karantzalos K. (2014). Automatic Descriptor-Based Co-Registration of Frame Hyperspectral Data. Remote Sens. 6 no.4, 3409–3426. doi: 10.3390/rs6043409
Van Dover C. L., German C. R., Speer K. G., Parson L. M., Vrijenhoek R. C. (2002). Evolution and Biogeography of Deep-Sea Vent and Seep Invertebrates. Science 295 (5558), 1253–1257. doi: 10.1126/science.1067361
Vera M., Schippers A., Sand W. (2013). Progress in Bioleaching: Fundamentals and Mechanisms of Bacterial Metal Sulfide Oxidation-Part a. Appl. Microbiol. Biotechnol. 97, 7529–7541. doi: 10.1007/s00253-013-4954-2
Vilaseca G., Deplus C., Escartín J., Ballu V., Nomikou P., Mével C., et al. (2016). Oceanographic Signatures and Pressure Monitoring of Seafloor Vertical Deformation in Near-Coastal, Shallow Water Areas: A Case Study From Santorini Caldera. Marine Geodesy 39 no. 6, 401–421. doi: 10.1080/01490419.2016.1226222
Von Damm K. L. (1995). Controls on the Chemistry and Temporal Variability of Seafloor Hydrothermal Fluids,” in Seafloor Hydrothermal Systems. Physical Chemical Biological Geological Interact. (Washington, DC) 91, 222–247. doi: 10.1029/GM091p0222
Von Damm K. L., Oosting S. E., Kozlowski R., Buttermore L. G., Colodner D. C., Edmonds H. N., et al. (1995). Evolution of East Pacific Rise Hydrothermal Vent Fluids Following a Volcanic Eruption. Nature 375, 47–50. doi: 10.1038/375047a0
Wilcock W. S. D., Tolstoy M., Waldhauser F., Garcia C., Tan Y. J., Bohnenstiehl D. R., et al. (2016). Seismic Constraints on Caldera Dynamics From the 2015 Axial Seamount Eruption. Science 354 (6318), 1395–1399. doi: 10.1126/science.aah5563
Witze A. (2022). Why the Tongan Eruption Will Go Down in the History of Volcanology. Nature. 602, 376–378. doi: 10.1038/d41586-022-00394-y NEWS FEATURE-09 February 2022.
Zegkinoglou N., Kilias S. P., Keith M., Smith D. J., Nomikou P., Polymenakou P. (2019a). Decoupled Geochemical Behaviour of Tl and, Au and as, in Modern Sea-Floor Massive Sulfides, Kolumbo Arc-Volcano: Evidence From LA-ICP-MS Elemental Mapping of (Au, Tl, Sb)-Rich Arsenian Pyrite. Bull. Geological Soc. Greece. Sp. Pub. 7 Ext. Abs. GSG2019-339, p. 450–451.
Zegkinoglou N., Kilias S. P., Keith M., Smith D. J., Nomikou P., Polymenakou P. (2019b). Goldschmidt2019 Abstracts, Barcelona 2019, August 18-23.
Zimanowski B., Büttner R. (2003). “Phreatomagmatic Explosions in Subaqueous Volcanism, in Explosive Subaqueous Volcanism,”. Ed. White J. D. L., Smellie J. L., Clague D. A. (Eds). (Washington, D.C: American Geophysical Union), 51–60.
Keywords: Kolumbo, hydrothermal vents, monitoring, submarine volcano, Santorini, marine technological innovation
Citation: Nomikou P, Polymenakou PN, Rizzo AL, Petersen S, Hannington M, Kilias SP, Papanikolaou D, Escartin J, Karantzalos K, Mertzimekis TJ, Antoniou V, Krokos M, Grammatikopoulos L, Italiano F, Caruso CG, Lazzaro G, Longo M, Sciré Scappuzzo S, D’Alessandro W, Grassa F, Bejelou K, Lampridou D, Katsigera A and Dura A (2022) SANTORY: SANTORini’s Seafloor Volcanic ObservatorY. Front. Mar. Sci. 9:796376. doi: 10.3389/fmars.2022.796376
Received: 16 October 2021; Accepted: 07 March 2022;
Published: 31 March 2022.
Edited by:
Paolo Favali, ERIC Foundation, ItalyReviewed by:
Pavlos Megalovasilis, University of Patras, GreeceKaren Bemis, Rutgers, The State University of New Jersey, United States
Copyright © 2022 Nomikou, Polymenakou, Rizzo, Petersen, Hannington, Kilias, Papanikolaou, Escartin, Karantzalos, Mertzimekis, Antoniou, Krokos, Grammatikopoulos, Italiano, Caruso, Lazzaro, Longo, Sciré Scappuzzo, D’Alessandro, Grassa, Bejelou, Lampridou, Katsigera and Dura. This is an open-access article distributed under the terms of the Creative Commons Attribution License (CC BY). The use, distribution or reproduction in other forums is permitted, provided the original author(s) and the copyright owner(s) are credited and that the original publication in this journal is cited, in accordance with accepted academic practice. No use, distribution or reproduction is permitted which does not comply with these terms.
*Correspondence: Paraskevi Nomikou, ZXZpbm9tQGdlb2wudW9hLmdy