- 1Departamento de Medio Ambiente, CIIDIR Sinaloa – Instituto Politécnico Nacional, Sinaloa, Mexico
- 2Laboratorio de Biología, Universidad Autónoma de Occidente, Sinaloa, Mexico
- 3Laboratorio: Biopolímeros, ENCB – Instituto Politécnico Nacional, Ciudad de México, Mexico
The production of total phenolic content (TPC) and total flavonoid content (TFC), antioxidant (AA), and copper chelating (CACu+) activities of Rhizophora mangle from three coastal lagoons under different grades of anthropogenic pollution and pollutant presence were analyzed. R. mangle is a mangrove plant that inhabits tropical coastal lagoons and shows the ability to accumulate pollutants in their tissues and respond to environmental stressors via the production of secondary metabolites AA and CACu+ activities. In total, 108 samples (June 2010 to August 2011) of leaves, barks, and the roots of R. mangle from Magdalena Bay (MBAY), Navachiste Lagoon (NLAG), and Terminos Lagoon (TLAG) (Mexico) were collected. Folin-Ciocalteu colorimetric, aluminum chloride colorimetric, ABTS∙+ discoloration, and pyrocatechol-violet methods calculated TPC, TFC, AA, and, CACu+, respectively. Atomic absorption spectrometry, gas chromatography, and Walkley–Black methods were used to calculate the metal content, organochlorine pesticides (OCPs), and organic matter (OM) in sediments. One-way ANOVA and post hoc Tukey’s, Scheffé’s, and Bonferroni and Holm multiple comparisons were used to determine significance, and Pearson’s test was applied to determine the coefficient of correlations. Significant differences among the lagoons of TPC, TFC, AA, and Cu+CA activities of Rhizophora mangle are explained by their different grades and type of anthropogenic pollution. A significant increase in the TPC and TFC in bark implies a response to the metal and OCPs pollution in surrounding sediments. Significant AA and Cu+CA activities responded to combat environmental stress conditions caused by the OCP and trace metal concentrations in surrounding sediments. Strong and positive correlations among OCP and trace metal were found and related to the constant pesticide residues input. However, OCP concentrations in the surrounding sediments of R. mangle trees did not influence the phenolic production of AA or the Cu+ CA. R. mangle is affected by the type of anthropogenic pollutants and was reflected in the bio-compounds production, antioxidant, and chelating activities related to the concentration of the metals in sediments.
Introduction
In the last decades, the pollution caused by anthropogenic sources has been increasing. Most organic pollutants residues persist in the environment and eventually are discharged, such as the coastal lagoons. The constant input of anthropogenic pollutants, such as oils, trace metals, and pesticides residues maintain mangrove ecosystems constantly stressed (Lewis et al., 2011). In these areas, the pollutant residues usually attach to the organic matter (OM) found in the sediments and become bioavailable to the marine biota that inhabits these coastal lagoons. The mangrove ecosystems present in these zones are very important because of their role in ecology services, such as primarily the coastal line protection, breeding or reproduction areas, and sediment retention.
Mangroves trees to deal with this pollution stress secrete different molecules, such as catalase, peroxidases, polyphenol oxidase, indoleacetic acid oxidase, carotenoids, and phenolic compounds (Lattanzio et al., 2012). These compounds are reactive oxygen scavenging species (ROS) that are produced to avoid ROS undesirable potential adverse effects (Rico et al., 2015), protection against ultraviolet radiation, herbivores, pathogens, salinity, and show antioxidant and chelating properties (Bayen, 2012; Sivanadanam et al., 2012; Agati et al., 2013; Glasenapp et al., 2019). The antioxidant activity prevents damage from ROS through radical scavenging or as prevention by binding metal ions (Brunetti et al., 2013), by scavenging of free radicals, or chelating metal traces (Lattanzio et al., 2012).
The Rhizophora mangle is a mangrove species that is distributed on the tropical coast of the world, and Mexico occupies the first line of coastal lagoons in these zones, such as the Navachiste, Terminos, and Bahia Magdalena lagoons. This species is known to produce ROS. Among them are the phenolic compounds, such as procyanidins, catechin, epicatechin, epigallocatechin, epicatechin gallate, quercetin, epicatechin, catechin, hydroxybenzoic acid, kaempferol–3–β–glucopyranoside, quercetin 3–O–β–glucopyranoside, quercetin 3–O–6″-trans-coumaroyl–β-glucoside, kaempferol 3–O–β–rutinoside, and quercetin 3–O–β–rutinoside (Costa et al., 2014). The chelating capacity of phenolic compounds gives R. mangle trees the ability to accumulate metal concentrations of the iron plaque deposits in their roots (MacFarlane et al., 2007). Due that R. mangle seems to vary the production of secondary metabolites according to the pollution conditions in the environment, such as the phenolic compounds and their antioxidant or chelating capabilities (Vasavilbazo-Saucedo et al., 2018; Martínez-Álvarez et al., 2019; Diaz et al., 2021), it is essential to know if under different pollutant or grade of pollution affect this production.
The hypothesis in this study was to determine that even some environmental conditions affect the concentration of polyphenols in mangroves. It is possible to evaluate how the source and grade of trace metal and organochlorine pesticides pollutants presented in the sediments in different coastal lagoons influence the secretion of phenolic compounds and antioxidant or chelating activities of R. mangle against these pollutants.
Materials and Methods
Study Sites
The mangrove trees were identified by taxon keys (Rzedowski, 1981; Agraz-Hernández et al., 2006). All samples were taxonomically identified by Prof. José Adrian Beltran Magallanes, who is in charge of the Biology School Herbarium of the University of Sinaloa, Mexico. No voucher code is given. Tissues samples were collected from the three coastal lagoons: Terminos Lagoon (TLAG) is located in the Gulf of Mexico (18° 35′ N and 91° 32′ W) and is impacted by petroleum-related activities, pesticides, and metals (Benítez et al., 2012); Navachiste Lagoon Complex (NLAG) is located in the south-eastern part of the Gulf of California (25° 28′ N and 108° 48′ W) and neighbored by the most prominent agricultural area of Mexico (Reyes-Montiel et al., 2013; Granados-Galván et al., 2015) that generates pollution from the use of organochlorine pesticides (OCPs) whose residues are disposed into this lagoon; and Magdalena Bay (MBAY) is located in the western part (Pacific) of the Baja California Peninsula (26° 44′ N and 113° 09′ W), and it is a low anthropogenic-polluted lagoon free of small or large industries or large-scale agriculture which most of its environmental degradation is due to artisanal fisheries (Sujitha et al., 2019; Figure 1).
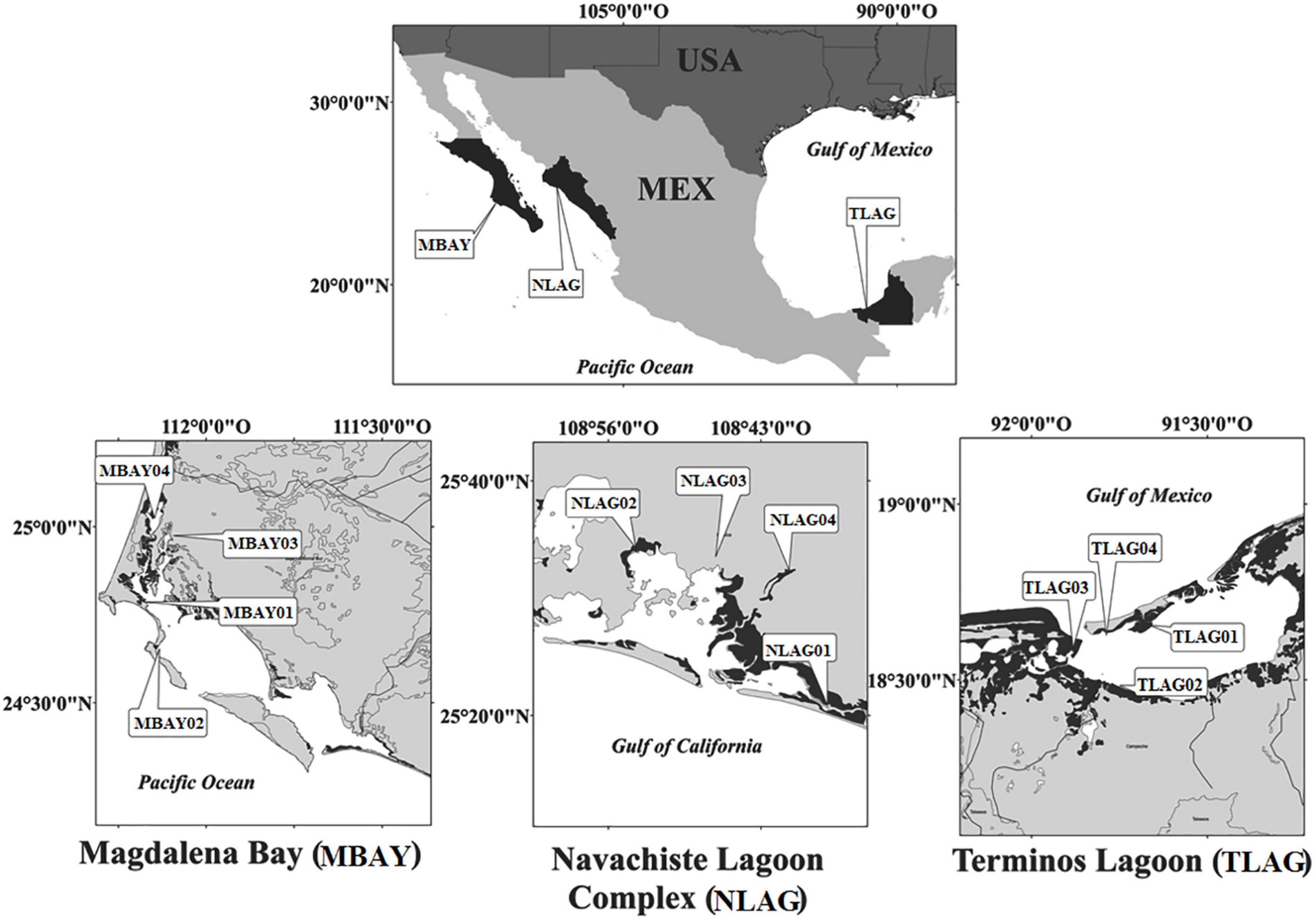
Figure 1. Geographical location of the study area and location of sampling sites in Magdalena Bay (MBAY) (A), Navachiste Lagoon complex (NLAG) (B), and Terminos Lagoon (TLAG) (C).
Experimental Design
Mangrove Sample Collection
Three samples of leaves, bark, and roots of Rhizophora mangle trees (between 1.5 and 4 m height) were sampled from four sampling sites inside each lagoon during the period of June to August 2011 (Figure 1). To avoid differences between sampled trees, individuals within sampled regions, sites adjoined to effluents drainages from aquaculture or agriculture, or without the apparent influence of these effluents were selected. Tissue samples were analyzed in triplicate. The selection criteria of trees within the regions were focused on individuals above 5 m height, healthy, and close to each other in the same sampling site. Bark and roots samples were collected by scraping the mangrove’s tissues with a jack-knife (Naranjo-Sánchez and Troncoso-Olivo, 2008). Three leaves per tree were randomly removed and were selected without yellowish coloration or signals of broken edges and physical or parasitic damage. Each leaf was cleaned with distilled water and packed in an aluminum foil in a cold dark recipient and transported to be stored in a freezer at the CICIMAR Environmental Laboratory (MBAY), CIIDIR SINALOA Research Center (NLAG), or the University of Campeche in Ciudad del Carmen (TLAG). All tissues samples were individually wrapped with newspaper and were dehydrated slowly at 40°C in a botanical incubator for 7 days (Haq et al., 2011). After dehydration, the samples were separated and individually milled in a grain grinder (Krups® GX 4100, Type F408, Mexico). The powders were stored in black plastic bags under darkness and dry room-temperature conditions.
Ethanolic Extracts
All samples were transported to the CIIDIR-IPN Research Center in Durango City, Mexico. Three aliquots per sample were used. Since phenolic compounds have many unsubstituted hydroxyl or sugar groups, they are considered polar and soluble in polar solvents, such as ethanol and water. Briefly, 10 ml of ethanol at 5°C (80%, v/v) was added to each milled dry aliquot (0.5 g) and were shaken on a heating plate (Cimarec®, United States) in darkness and room temperature (30 min) and then filtered (Whatman® No. 1). Solutions were evaporated to dryness using a water evaporator at 40°C, and a second extraction was done with 10 ml of ethanol at 5°C (80%, v/v). Then, 20 ml of petroleum ether was added to each solution after extraction to remove chlorophyll. All supernatant extracts were kept in darkness and refrigerated until further analysis. The leaf (100 μl), bark (10 μl), and root (20 μl) extracts were placed in a vial and diluted with ethanol (800 g L–1) at 900, 990, and 980 μl, respectively, at room temperature, to calculate TPC, TFC, ABTS∙ + radical scavenging capacity, and Cu+ CA (Saiga et al., 2003).
Absorbance readings (ABS) of TPC (750 nm), TFC (510 nm), and AC (695 nm) were compared with their standard curves with Gallic acid (TPC (Equations 1 and 3), and catechin (TFC) (Equation 2), AC (ABTS∙ +) (Equation 3), and Cu+ CA chelating rate (Equation 4) equivalent concentrations, respectively.
Total Phenolic Content Evaluation
Each extract (100 μl) was mixed with Folin-Ciocalteu reagent (750 μl) diluted ten times with distilled water, and kept in darkness (5 min); then, a 60 g L–1 solution of Na2CO3 (750 μl) was added to neutralize the reaction and incubated for 90 min to read the absorbance (750 nm) to estimate the TPC expressed as Gallic acid equivalents (GAE) per 100 g dry basis (db) (mg GAE 100 g–1 db). A standard curve of Gallic acid (SIGMA® Cat. G7384) was read at 750 nm in a Thermo Scientific® Spectrophotometer UV-VIS GENESYS® 10 s (r2 = 0.9968) (Adom and Liu, 2002) (Eq. 1).
Total Flavonoid Content Evaluation
Based on the aluminum chloride colorimetric method (Sultana et al., 2009), 100 μl of each extract was mixed with 75 μl of NaNO2 (50 g L–1). After 5 min, 75 μl of AlCl3 (100 g L–1) was added, and 6 min later, 500 μl of NaOH (40 g L–1) and 600 μl of distilled water were added. The solution was mixed and the absorbance (510 nm) was read within 30 min in the Thermo Scientific® and expressed in milligrams of catechin per gram dry basis (mg CE 100 g–1 db). The standard curve was performed with a standard solution (0.1 mg ml–1, catechin hydrate, SIGMA®, Cat. QO125) (Eq. 2).
Antioxidant Activity Assay
ABTS∙ + Radical-Scavenging Capacity Evaluation
The discoloration method (Arnao et al., 2001) was carried out with some modifications. ABTS∙+ (0.0384 g) and K2S2O8 (0.0066 g) solutions were diluted with 10 ml of distilled water and incubated for 12 h to obtain radical ABTS+ solution, which was diluted in 550 μl (working solution) to 50 ml in ethanol. The ABTS+ solution was diluted with distilled water to a 0.70 absorbance (SD = 0.02) at 734 nm at room temperature (Pawlak et al., 2010) in the Thermo Scientific® Spectrophotometer. An aliquot (990 μl) of ABTS+ standard solution (SIGMA®, Cat. A1888) supplemented with ethanol (800 g L–1, 10 μl) and an aliquot sample from the previously prepared dilutions (triplicate) were read every minute for 7 min, calculating the scavenging activity (Equation 3):
Where: Abs sample = sample absorbance; Abs control = control absorbance; T0 = minute zero; T5 = 5 min. The absorbance decrease was expressed as the inhibition percentage of ABTS+ expressed as Trolox equivalent per gram of dry basis (20–200 μmol L–1).
Determination of Cu+ CA
The method described by Saiga et al. (2003) was used. A mixed solution of 290 μl of 50 mM acetate buffer (pH 6.0), 6 μl of 4 mM pyrocatechol violet, copper (20 μl), and 1 μg (10 μl) (CuSO4 5H20) was added to each aliquot sample (by triplicate). The Abs of change of color from blue to yellow was measured at 632 nm in a Thermo Scientific® Spectrophotometer. The standard was an aliquot of Kupfer (II)—sulfate standard solution (Merck®, Cat. 202790) (Eq. 4):
Where: A0 = absorbance of the control (blank, without extract); A1 = absorbance in the presence of the extract; and; Cu = chelating activity assay.
Textural Class of Sediments
The particle size and clay type of all sediment samples were analyzed in the Environmental Pollution Laboratory of the CIIDIR-IPN Research Centre in Sinaloa (CIIDIR-SINALOA), Mexico. Four sediments samples were collected from each collecting point in the three lagoons. The textural class of the soil was determined with the Bouyoucos method (Bouyoucos, 1962). Three replicas for a given sample (50 g) of soil (dry and 2-mm sieved) per sample were analyzed. Each replica was placed in a cup filled with distilled water up to 5∼6 cm above the sediment supplied with 35 ml of 1 N sodium hexametaphosphate. Each replica was thoroughly mixed (for high clay soils: allow to slake (soak) for 15 min; for sandy soils: mix for 5 min, and for finely textured soils: mix for 15 min). The soil suspensions were transferred to a sedimentation cylinder and diluted in 1 L of water. A hydrometer was placed inside the cylinder, filling with water to the 1,150 ml mark. The hydrometer was removed and was sealed each time at the top of the cylinder with parafilm. The cylinder was tilted back and forth for thorough mixing several times and set on a stable surface. The hydrometer was placed into it, and the 40 s timing was immediately recorded when it was read. The hydrometer was removed from the cylinder. The time and temperature were recorded and started a 2 h setting period. A control blank cylinder (water; 5 ml of l N sodium hexametaphosphate) was prepared, and both were read as follows:
Temperature correction factor (T°C) = (observed temperature – 20°C) × 0.03.
Corrected 40 s reading: 40 s (T°C): 40 s reading – Blank reading + T°C.
Corrected 2 h reading: 2 h (T°C) = 2 h reading – blank reading + T°C.
Particle percentages: Sand (%) = (2 - 0.05 mm): [Oven dry soil weight – 40 s reading (c)] + [oven dry soil weight] × 100%; Clay (%) = (< 0.002 mm): [2 h reading (c)] × [oven dry soil weight]–1 × 100%; Silt (%) = (0.05 - 0.002 mm): 100 - (% sand + % clay).
We determined the textural classes of the soil sample using the textural triangle.
Trace Metal Elements in Sediment
Eight sediment samples per coastal lagoon, two samples per sampling point (n = 72), were collected with a Teflon spoon and immediately placed into polyethylene bags, stored in an icebox, and then kept in a freezer for preservation until analysis in the Environmental Pollution Laboratory in the CIIDIR-SINALOA.
Acidic digestion was used to determine Fe, Cu, Pb, Ni, Cd, and Mn contents (Breder, 1982). Each sediment sample was dried (60°C) for 48–96 h and homogenized by grinding. Then, 0.5 g of each sample was placed on the heating plates with 5 ml aqua regia (1:3 HCl: HNO3) and kept until dryness was achieved again. After cooling, the solution was adjusted to 50 ml with deionized water and stored in plastic vials until analysis. The total content of metals was determined by flame atomic absorption spectroscopy (GBC® Avanta) (air-acetylene flame), recovering over 90%. Reference materials (PACS-2, Marine Sediment Reference Materials for Trace Metals and other Constituents, National Research Council, Canada) were used to ensure standardization.
Organochlorine Pesticides
Eight sediment samples per coastal lagoon, two samples per sampling point, were collected with a Teflon® spoon, wrapped carefully in aluminum foils, and stored in polyethylene bags. Samples were kept in a cooler with ice for preservation and transported to the Environmental Pollution Laboratory of the CIIDIR-SINALOA.
The USEPA 8270, 508, 8081b, 3660, 3500, and 3600 extraction technics were conducted (Usepa, 1995, 1996a, 1996b, 1998, 2007a, 2007b, 2007c). In general, 5 g of dried sediment was added to an Erlenmeyer flask adding enough dichloromethane to cover the sediment and immediately was sonicated for 15 min (repeated three times). The dichloromethane was removed at ∼150 ml each time (method 3550). After that, a sodium sulfate tap was placed in a fiberglass funnel, removing the rest of the dichloromethane particles and placing the flask in a rotavapor until dryness to recover 2 ml of hexane.
Gas chromatograph Perkin Elmer® AutoSystem XL, with an electron capture detector (ECD), coupled to the Navigator software © V. 6.3.1., provided with a J&W® DB 5–30 m (0.25 mm internal diameter capillary column, 5% diphenyl-95% dimethylpolysiloxane), was used to determine the OCPs. Briefly, 1 μl of the hexane-diluted OCP sample was injected into the chromatograph. OCPs were identified by their retention times, and quantitation was calculated on peak height/area and by comparison with the results of the reference standard (SUPELCO® 48858-U Sigma-Aldrich) that included the OCP analytes: α–BHC, β–BHC, lindane, δ–BHC, aldrin, 6–hydroxy-2–naphthyl disulfide, 1,1–dichloro–2,2–bis (4–chlorophenyl) ethane, 4,4′–DDT, dieldrin, α–endosulfan, β–endosulfan, endosulfan sulfate, endrin, endrin aldehyde, heptachlor, heptachlor exo-epoxide, and methoxychlor.
Organic Matter in Sediments
In the Environmental Pollution Laboratory of the CIIDIR-SINALOA, the chromic and sulfuric acid methods (Walkley and Black, 1934) were used. A 100 g sample of soil was weighed and placed into a 500 ml Erlenmeyer flask containing 10 ml of 1 N potassium dichromate solution. Then, 20 ml of sulfuric acid was added and mixed by gently rotating for 1 min. Samples were left for 30 min and then diluted with deionized water (200 ml). After dilution, 10 ml of phosphoric acid, 0.2 g ammonium fluoride, and 10 drops of diphenylamine indicator were added. The mixture (drop by drop) was titrated with 0.5 N ferrous ammonium sulfate solution until the endpoint from dull green to a brilliant green. Three replicas per sample and one quality control sample was analyzed for each set of samples with the following equation:
% Organic Matter = 10[1(S÷B)] × 0.67
Where, S = sample titration; B = blank titration.
Statistical Analysis
One-way ANOVA to analyze the differences in TPC, TFC, ABTS∙ +, and Cu+ CA, OCP, metal, OM, and phosphate concentrations in sediments was applied, and if significant differences were found (p < 0.05), a post hoc honestly comparison tests (HSD) Tukey’s, Scheffé’s, and Bonferroni and Holm multiple were used (Vasavada, 2014). Pearson’s analysis was performed using SIGMA PLOT® software to obtain the coefficient of correlations (r) of TPC, TFC, AA, and Cu+ CA per tissue among sites and tissues within sites, among bio compounds, and AA and Cu+ CA activities with trace metal concentrations.
The mean concentration and standard deviation (SD) were calculated for trace metals and OCPs and compared with the average continental crust metal concentration (Taylor, 1964) to determine the enrichment rate. A regression analysis was carried out to determine the coefficient of determination (r2) among trace metals.
Results
Total Phenolic Content
Among lagoons, ANOVA and Tukey’s HSD test showed higher TPC in the leaves extracts from MBAY (p < 0.01) and bark extracts in TLAG (p < 0.01) (Figure 2A), while within lagoons, significant higher TFC in the bark was found in the three lagoons (p ≤ 0.05) (Figure 2B).
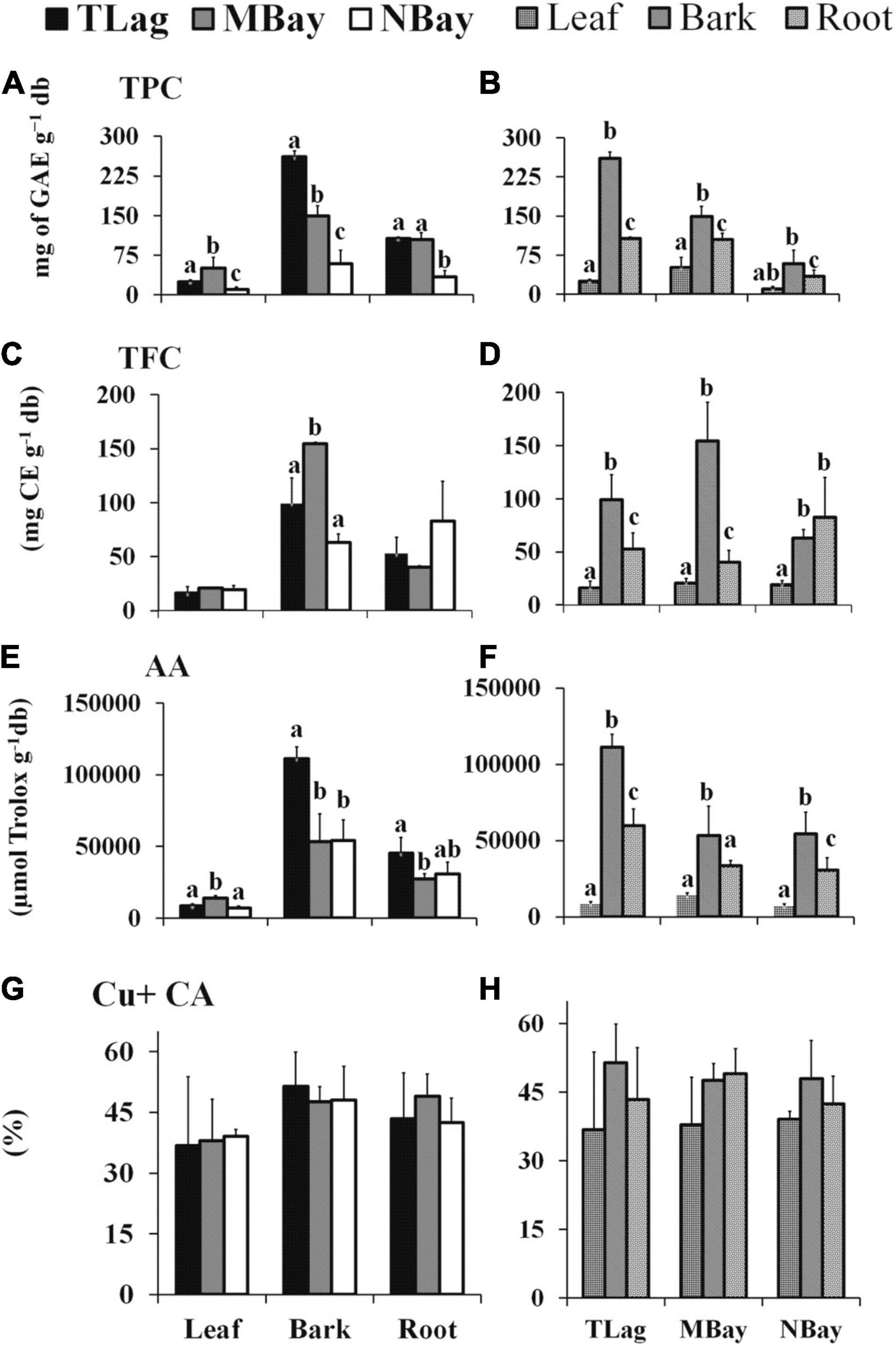
Figure 2. Biocompounds concentrations (A–D) (average ± SD), AA (E,F), and chelating activities (G,H) of leaves, bark, and roots of R. mangle among and within MBAY, NLAG, and TLAG. Different letters show significant statistical differences (p ≤ 0.05).
Total Flavonoid Content
One-way ANOVA and Tukey’s HSD test among lagoons showed the highest significant TFC (p = 0.002) in the bark extracts from MBAY (p < 0.05) (Figure 2C). Within lagoons, higher significant TFC was found in the bark extracts from TLAG and MBAY (p ≤ 0.01) (Figure 2D).
Antioxidant ABTS∙ + Radical-Scavenging Capacity
One-way ANOVA results showed significant differences (p < 0.0005) in leaf extracts in MBAY (p < 0.0001), and bark and root extracts (p < 0.05) from TLAG (Figure 2E). Within lagoons, antioxidant activities were found in the bark extracts in MBAY, NBay, and Tag (p ≤ 0.01) (Figure 2F).
Copper Chelating Activity
Among and within lagoons, one-way ANOVA and post hoc HSD showed no significant differences in the Cu+ CA chelating activity (Figures 2G,H).
Organic Matter in Sediments
One-way ANOVA and post hoc HSD showed no significant differences within-sample collecting sites and among lagoons (Figures 3A,B).
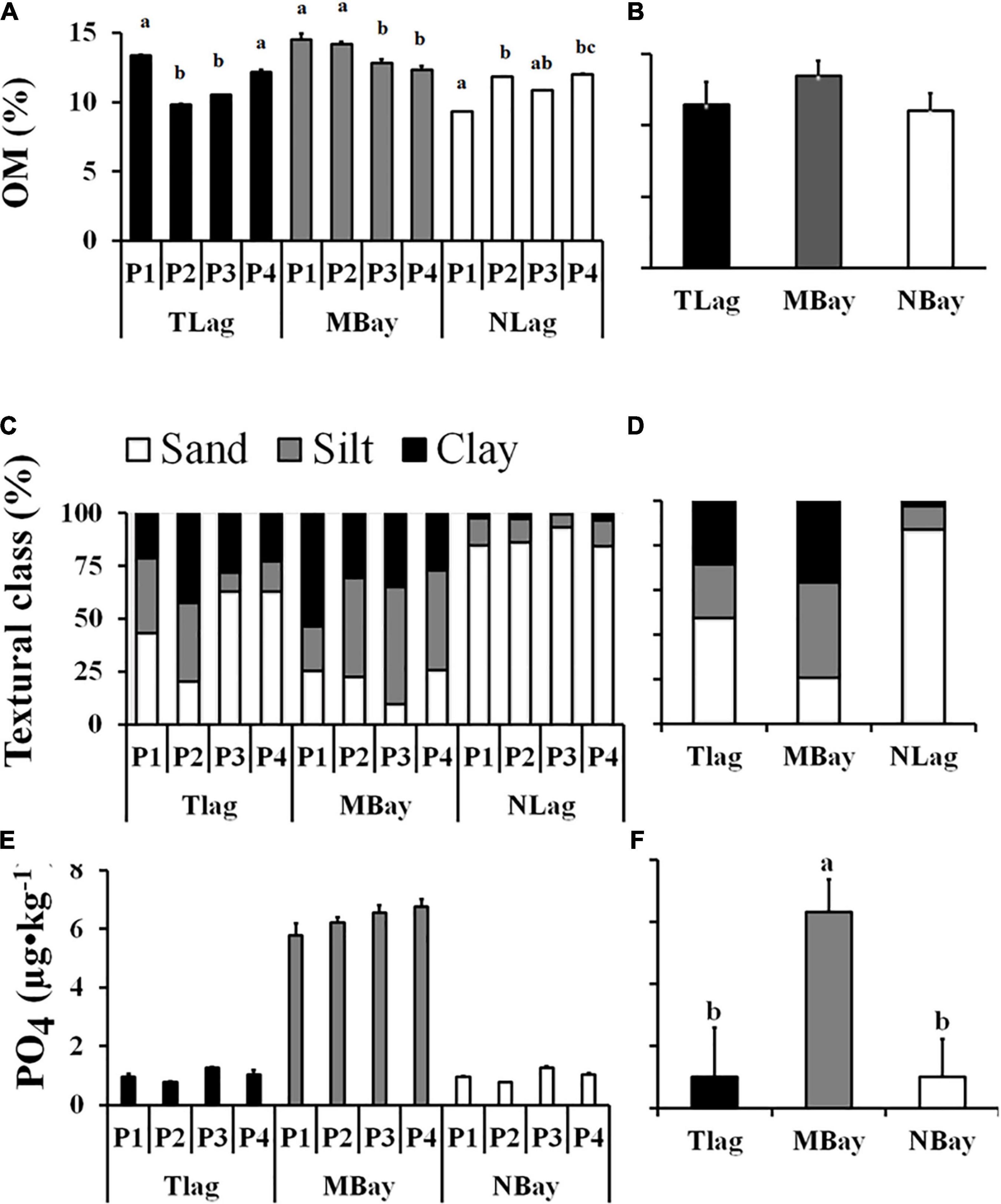
Figure 3. Organic matter (OM) concentration (A,B), PO4 (C,D), and textural classes (E,F) (average ± SD) in sediments from MBAY, NLAG, and TLAG. Different letters show significant statistical differences (p ≤ 0.05).
Organic matter correlation was strong and significant with Cu (−0.79), Pb (−0.85), Ni (−0.87), Zi (0.82), and Cd (−0.15) in NLAG. In TLAG, OM showed significant (p < 0.05) strong positive linear correlations with Cu (0.87), Pb (0.79), and Cd (0.78). In MBAY, OM showed no significant correlations with the variables analyzed (Table 1).
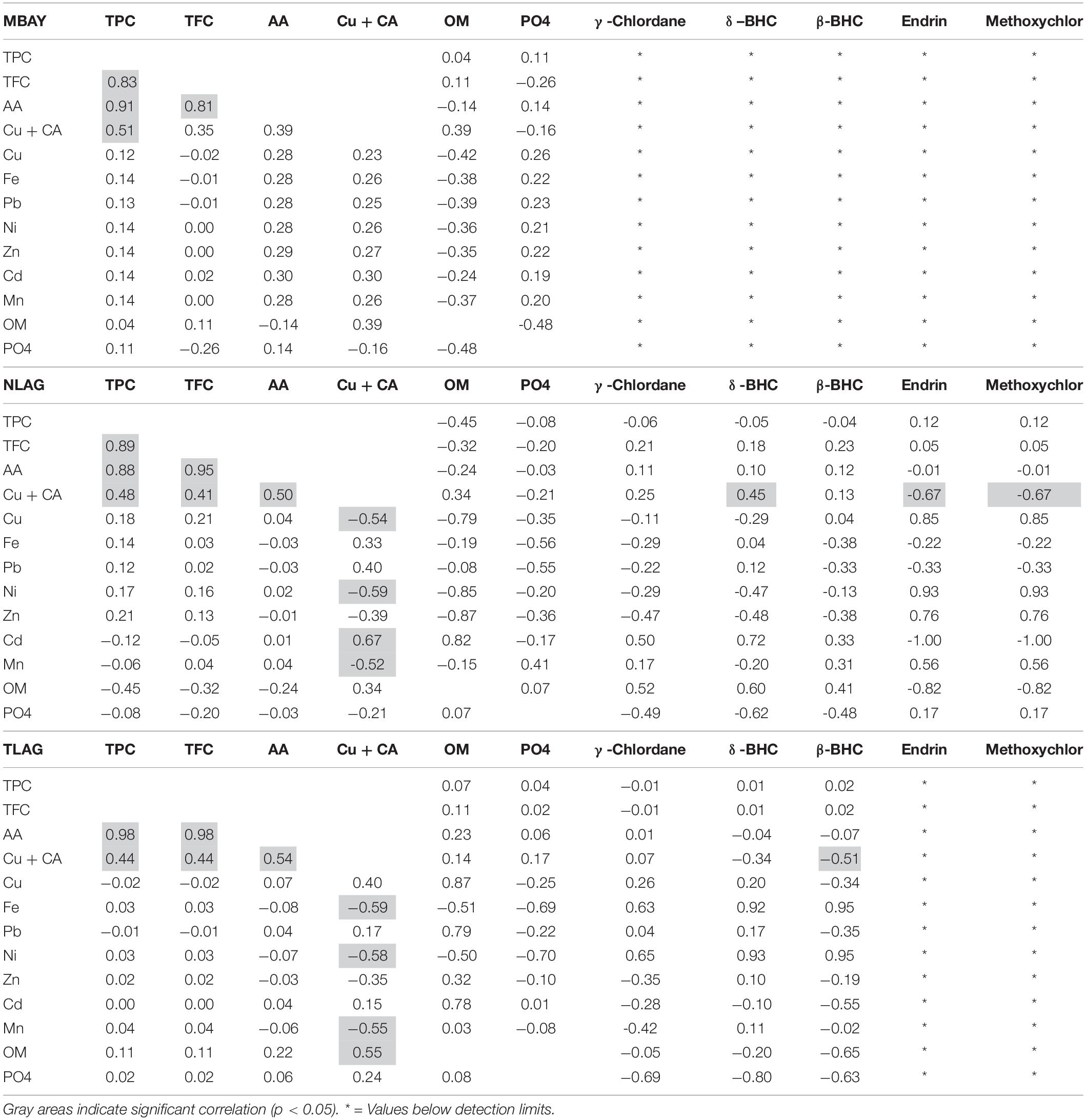
Table 1. Coefficient of correlation among bioactive compounds (TPC, TFC), antioxidant and chelating activities, organic matter (OM), and PO4 of R. mangle and with trace metals and OCPs in sediment.
Textural Class in Sediments
The soil texture structure of sediments among lagoons was similar in the sand, silt, and clay in TLAG (47, 24, and 29%, respectively) and MBAY (87, 11, and 2%, respectively). NLAG was different in silt, clay, and sand (42, 37, and 21 silt%, respectively) (Figure 3C). Within the lagoons, the textural NLAG was different compared with the other two lagoons (Figure 3D).
Phosphates
Within lagoons, one-way ANOVA showed no significant differences (p < 0.01) in the PO4 concentrations (Figure 3E) and showed significant higher PO4 concentrations (p < 0.05) in MBAY (Figure 3F).
Metal Content in Sediments
The trace metals above continental crust average concentrations were in MBAY, the Pb; in NLAG were Pb and Zn; and in TLAG were Cu, Pb, Ni, and Cd (Table 2). One-way ANOVA showed significantly higher concentrations (p < 0.05) of Mn and Fe in NLAG among lagoons (Figure 4).
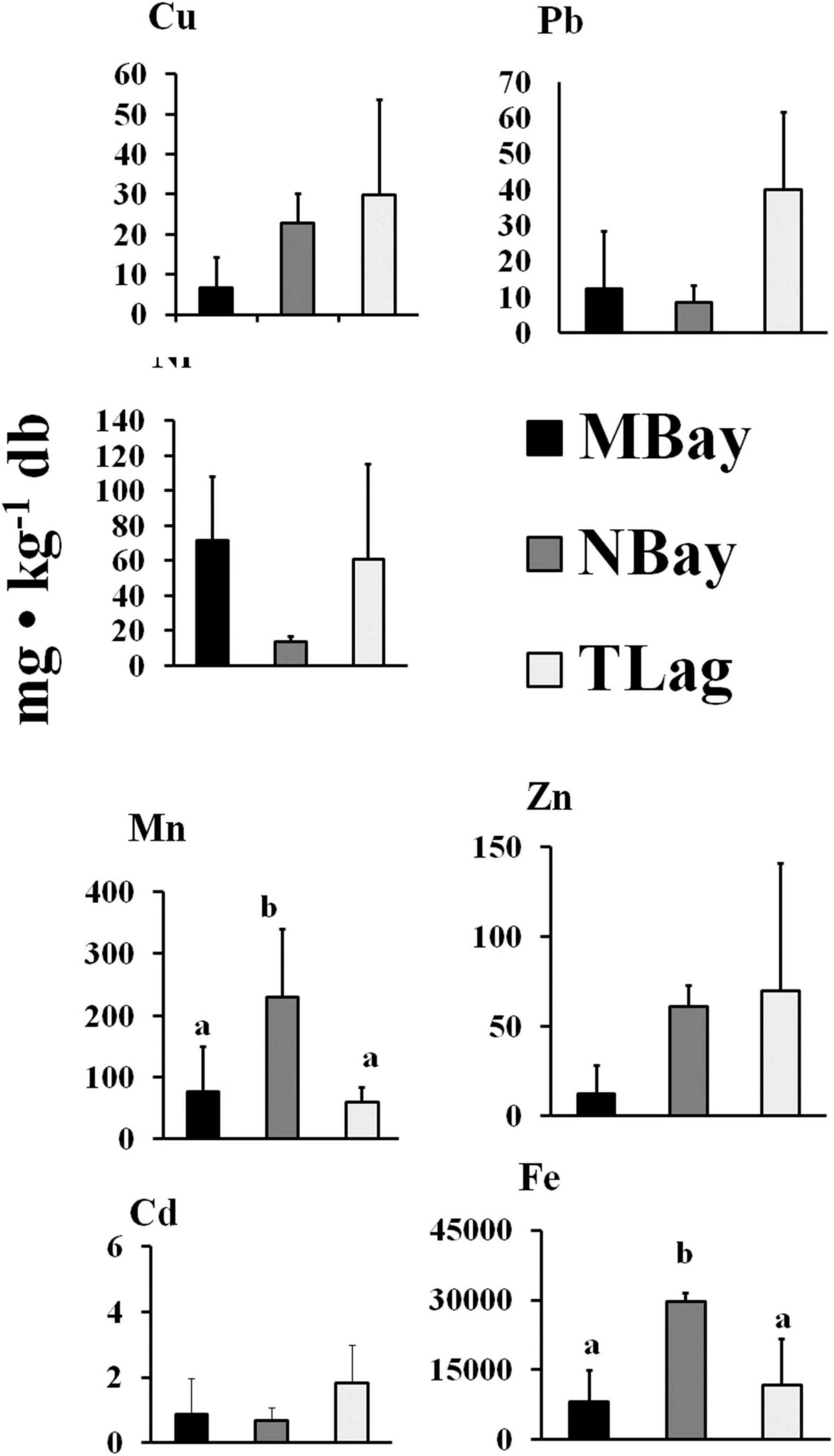
Figure 4. Trace metal concentrations (average ± SD) in surrounding sediments of R. mangle from MBAY, NLAG, and TLAG. Different letters show significant statistical differences (p ≤ 0.05).
The trace metal concentrations (higher to lower) in MBAY were Fe > Mn > Ni > Zn > Pb > Cu > Cd; in NLAG were Fe > Mn > Zn > Cu > Ni > Pb > Cd; and in TLAG were Fe > Mn > Zn > Ni > Pb > Cu > Cd.
In TLAG, strong and significant (p < 0.05) positive correlations were found between OM and Cu, Pb, and Cd (0.87, 0.79, and 0.78, respectively), and significant (p < 0.05) and negative correlations were found between PO4 with Fe and Ni (−0.70). In NLAG, OM showed negative and significant (p < 0.05) correlations with Cu, Ni, and Mn (−0.79, −0.85, and −0.15, respectively), and a strong positive and significant correlation (p < 0.05) with Cd (0.82). In MBAY, no significant correlations (p > 0.05) were found (Table 1).
Organochlorine Pesticides in Sediments
Organochlorine pesticide concentrations were detected in TLAG and NLAG. The highest OCPs proportions in TLAG were methoxychlor, β–BHC, δ–BHC, and γ–chlordane. In NLAG, the highest OCP proportions were β–BHC, δ–BHC, and γ–chlordane. In TLAG, were β–BHC, δ–BHC, and δ–chlordane (Figure 5). No significant correlations (p > 0.05) were found among OCP concentrations with bio compounds, AA, and Cu+ CA.
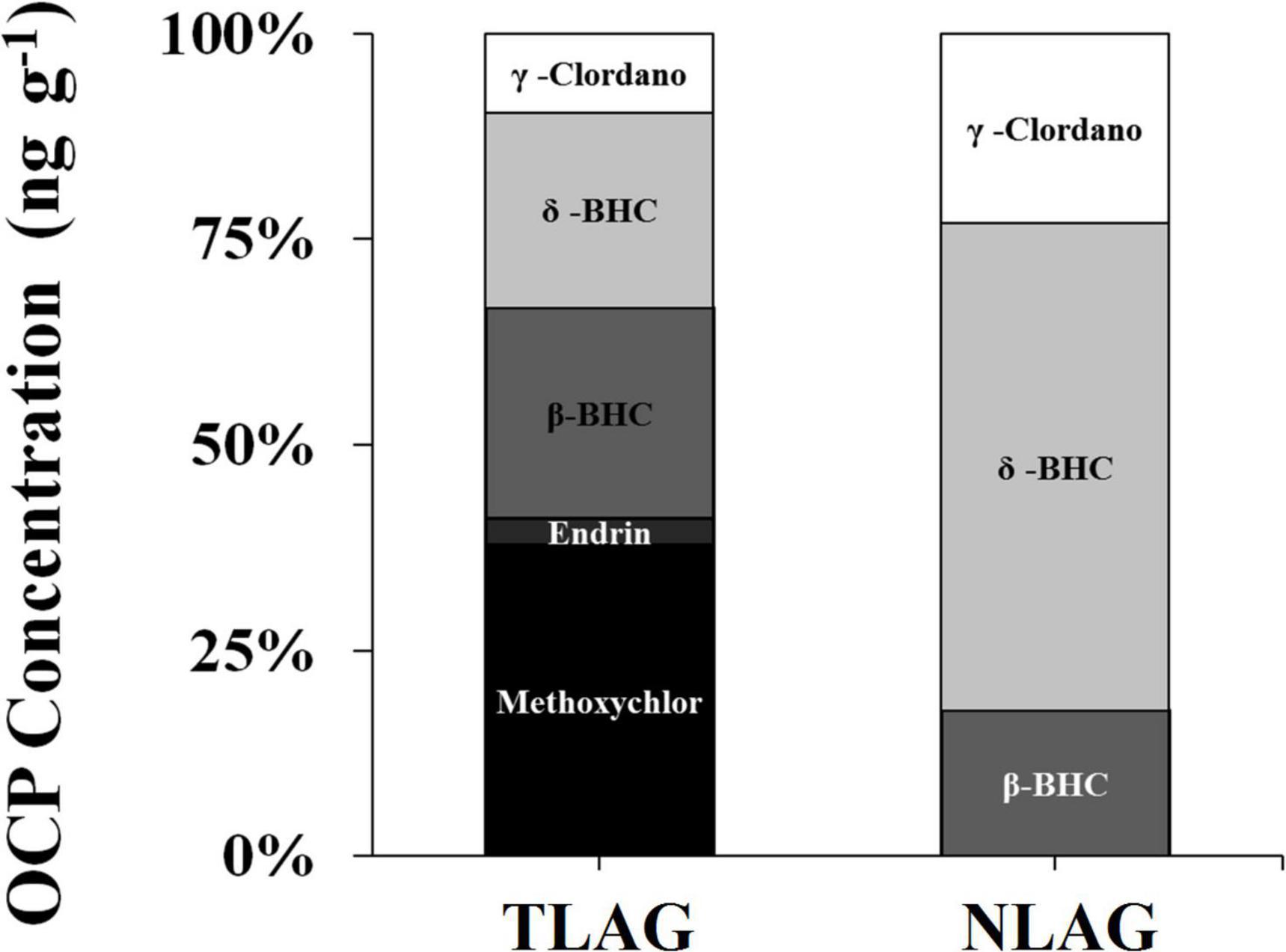
Figure 5. Organochlorine pesticides (OCP) concentrations (average ± SD) in sediments from MBAY, NLAG, and TLAG. Different letters show significant statistical differences (p ≤ 0.05).
Bioactive Compounds, AA, Cu+ CA, Trace Metal, and OCP Correlations
Among TFC, TPC, and AA within lagoons revealed significant correlations (p < 0.05), but no significant correlations were detected among trace metals with TFC, TPC, AA, and Cu+ CA.
In MBAY, TPC showed significant positive (p < 0.05) and robust correlations with TFC and AA and weak positive correlation with Cu+ CA, while TFC showed significant positive (p < 0.05) and robust correlations with AA. In NLAG, Cu+ CA showed significant positive (p < 0.05), and weak correlation with AA, a significant (p < 0.05) negative correlations with Cu and Ni, a significant positive (p < 0.05), medium correlation with Cd, moderate correlation with endrin but a significant negative (p < 0.05) correlation with methoxychlor. The OM showed a significant positive and weak correlation (p < 0.05) with δ–BHC and significant negative and high correlations with endrin and methoxychlor in this lagoon. The PO4 showed a significant (p < 0.05) and moderate correlation with δ–BHC. In TLAG, AA showed a significant positive and robust correlation with TPC and TFC, and the Cu+ CA showed significant negative and medium correlations with Cu, Zn, and Mn. The OM in this lagoon showed significant positive and robust correlations with Cu, Pb, and Cd and significant negative and medium correlations with Fe and Ni. The PO4 showed a significant negative and moderate correlation with Fe. In TLAG, γ–chlordane, δ–BHC, and β–BHC showed significant (p < 0.05) and positive correlations with Fe (0.63, 0.92, and 0.95, respectively). β–BHC had a significant (p ≤ 0.05) and strong positive correlation with Ni (0.95) and PO4 (0.63); negative correlation with Cd and OM (−0.56 and −0.65, respectively) (Table 1).
Discussion
The significant differences among lagoons of TPC, TFC, AA, and Cu+ CA activities of R. mangle are explained by their different grades and the type of anthropogenic pollution. Specifically to TPC, in TLAG where the oil extraction of the Campeche sound and the agriculture residues highly impact this lagoon and the significant increase in the TPC in bark extracts could be due to the stress response to the metal and OCPs pollution, previously reported in mangrove species (Jithesh et al., 2006; Krishnamoorthy et al., 2011). MBAY, less anthropogenically impacted than the other two, presented a significantly higher TFC in bark extracts. MBAY is constantly affected by the enrichment of trace metals due to natural causes, such as currents, upwelling, primary productivity, volcanism, and tectonic activity (Shumilin et al., 2005; Rodríguez-Meza et al., 2007). The TPC increase in the species R. mangle in MBAY could be due to the high concentrations of Cu, Pb, Ni, Zn, and Cd present in sediments as previously reported in other mangrove zones (Souza et al., 2014). The significant higher TFC in the bark of MBAY could be related to the vacuolar flavonoids, which constitute a secondary antioxidant system activated under severe stress conditions or to the regulation of different protein kinases activities responsible for mediating ROS-induced signaling cascades (Agati et al., 2012; Brunetti et al., 2013). Even in this study, leaves without visible damages or yellowish color or order to the apical bud were selected; no significant TPC or TFC differences in leaf extracts were found among and within lagoons. Nevertheless, since the composition of phenolic compounds is influenced by the position in the tree branches or on the shoot (Wongsen et al., 2013; Vagiri et al., 2015), studies are recommended regarding the TPC and TFC and leaf’s position in this species.
The adaptation mechanisms to combat the environmental stress conditions of mangroves increased the AA significantly in the bark of R. mangle in TLAG. The positive and strong correlation of AA with the concentrations of TPC (r2 = 0.88) and TFC (r2 = 0.95) has been previously reported in Rhizophora species (Asha et al., 2012) and is partly due to phenol compounds (Zhang et al., 2010). In R. mangle, the significantly higher AA in the bark from TLAG was correlated with the higher flavonoids and its production has been related as a defense mechanism to the abiotic stress (Brunetti et al., 2013; Shivashankara et al., 2016), possibly due to the high trace metal concentrations in sediments. Limiting nutrients in mangrove plants is caused by the deficiencies of P, N2, Fe, or Mg (Almahasheer et al., 2016; Alhassan and Aljahdali, 2021), and significant higher phosphorus values (p < 0.005) were found in MBAY, but no significant correlation with bio compounds, antioxidant, or chelating activities was determined. It implies that requirements in this nutrient in R. mangle were covered, and the production of phenolic compounds was not stimulated. The significant trace metal concentrations in the bark extract from TLAG and NLAG highlight the importance of this tissue against trace metals pollution in surrounding sediments, that after specific concentrations, generate stress (Bayen, 2012). The stored polyphenolic compounds in the bark of R mangle species have shown chelating properties (Sanchez et al., 2006), and the significant higher Cu+ CA (p < 0.05) could be related to the higher concentrations of Cu, Pb, Zn, and Cd in TLAG, or Mn in NLAG. In MBAY, the metal bioaccumulation has been occurring due to lithological characteristics and the hydrothermal processes of the lagoon (Sujitha et al., 2019), for which the concentrations of Cd, Cu, and Zn in R. mangle have been previously described in root > bark > leaf (Naranjo-Sánchez and Troncoso-Olivo, 2008). Nevertheless, it is necessary to analyze whether the bio-disposition of metals in the sediments in both lagoons was affected by the pH, salinity, or redox potential (Parra and Espinosa, 2008).
A correlation between high organic carbon content with high OCP concentrations in sediments has been reported (Eqani et al., 2011). In contrast, previous reports in the area have not found a correlation between OCPs concentration in edible tissues and dry-rainy seasons (Reyes-Montiel et al., 2013; Granados-Galván et al., 2015). Instead of the seasons or rain periods, Cl–1, total P, and electrical conductivity (EC) have been reported to be essential for causing the variations in OCP concentrations in sediments (Eqani et al., 2011), even it is possible that OCP concentrations in the surrounding sediments of R. mangle trees in the present study could not influence the phenolic production, the AA, or the Cu+ CA. Nevertheless, correlations among OCP and trace metal in mangrove sediments have been reported to be related to pesticide pollution (Chen et al., 2020; Ji et al., 2021), which is corroborated in the present study, that in NLAG and TLAG where strong positive correlations between OCPs and some trace metal concentrations (Cd, Ni, Zn, Mn, and Fe) were found. These lagoons are constantly being polluted by anthropogenic sources, such as the oil extraction industry as in TLAG (Celis-Hernandez et al., 2022) or intensive agriculture in NLAG (Ponce-Vélez and Botello, 2018). In the present study, the detected POCs have been banned internationally, according to the Stockholm Convention (Templeton, 2020). However, it is remarkable that the recent uses of OCPs are banned, such as γ –Chlordane, δ–BHC, β–BHC, Endrin, and Methoxychlor, probably due to illegal buying and selling through countries which production is still occurring (Ortíz et al., 2014) and illegally introduced by Port or Border Customs and clandestine applications.
The species R. mangle is well adapted to environmental stress and is capable of responding to different pollution scenarios (Souza et al., 2014). However, in the present study, it was evident that metal concentrations influenced the production of the phenolic compounds, AA, and chelating activities more than PO4, Nitrogen, OCP, OM, or textural classes in surrounding sediments. The three lagoons studied here are impacted under the different grades of pollution and pollutants, but the conspicuous production of TPC, TFC, and the AA and chelating activities was higher in the NLAG y TLAG where higher anthropogenic pollutants were determined. These lagoons are constantly impacted by anthropogenic activities, especially the intensive agriculture and oil extraction industry (Ramírez-Ayala et al., 2020). Regarding the response to environmental stress, a transcriptomic gene expression study in R. mangle will determine which gene is turned on or off in R. mangle under the extreme variable environment, such as the anthropogenic pollution grade or pollutants to understand the mechanisms that are activated during immune responses in plants. Finally, the present study results showed that R. mangle could be modulating their phenolic compounds production, antioxidant and chelating activities in tissues according to the pollution grade and pollutants presence in MBAY, TLAG, and NLAG lagoons.
Data Availability Statement
The raw data supporting the conclusions of this article will be made available by the authors, without undue reservation.
Author Contributions
HG-O has contributed equally to this work and share senior authorship. All authors contributed to the article and approved the submitted version.
Conflict of Interest
The authors declare that the research was conducted in the absence of any commercial or financial relationships that could be construed as a potential conflict of interest.
Publisher’s Note
All claims expressed in this article are solely those of the authors and do not necessarily represent those of their affiliated organizations, or those of the publisher, the editors and the reviewers. Any product that may be evaluated in this article, or claim that may be made by its manufacturer, is not guaranteed or endorsed by the publisher.
Acknowledgments
We are thankful to the IPN (SIP-20120079 and SIP-20130398) and FOMIX CONACYT-CAMPECHE (FOMIX-CAMP-2010-144280) for the financial support and Mrs. Ingrid Mascher for the English edition.
References
Adom, K. K., and Liu, R. H. (2002). Antioxidant Activity of Grains. J. Agri. Food Chem. 50, 6182–6187. doi: 10.1021/jf0205099
Agati, G., Azzarello, E., Pollastri, S., and Tattini, M. (2012). Flavonoids as antioxidants in plants: location and functional significance. Plant Sci. 196, 67–76. doi: 10.1016/j.plantsci.2012.07.014
Agati, G., Brunetti, C., Di Ferdinando, M., Ferrini, F., Pollastri, S., and Tattini, M. (2013). Functional roles of flavonoids in photoprotection: new evidence, lessons from the past. Plant Physiol. Biochem. 72, 35–45. doi: 10.1016/j.plaphy.2013.03.014
Agraz-Hernández, C. M., Noriega, R., López-Portillo, J., Flores-Verdugo, F., and Jiménez–Zacarías, J. (2006). Mangrove Key Identification in Mexico [Identificación de Los Manglares en México]. Mexico: Universidad Autónoma de Campeche.
Alhassan, A. B., and Aljahdali, M. O. (2021). Nutrient and physicochemical properties as potential causes of stress in mangroves of the central Red Sea. PLoS One 16:e0261620 doi: 10.1371/journal.pone.0261620
Almahasheer, H., Duarte, C. M., and Irigoien, X. (2016). Nutrient Limitation in Central Red Sea Mangroves. Front. Mar. Sci. 3:271 doi: 10.3389/fmars.2016.00271
Arnao, M. B., Cano, A., and Acosta, M. (2001). The hydrophilic and lipophilic contribution to total antioxidant activity. Food Chem. 73, 239–244. doi: 10.1016/S0308-8146(00)00324-1
Asha, K. K., Mathew, S., and Lakshmanan, P. T. (2012). Flavonoids and phenolic compounds in two mangrove species and their antioxidant property. Ind. J. Mar. Sci. 41, 259–264.
Bayen, S. (2012). Occurrence, bioavailability and toxic effects of trace metals and organic contaminants in mangrove ecosystems: A review. Environ. Int. 48, 84–101. doi: 10.1016/j.envint.2012.07.008
Benítez, J. A., Vidal, J., Brichieri-Colombi, T., and Delgado-Estrella, A. (2012). Monitoring ecosystem health of the Terminos Lagoon region using heavy metals as environmental indicators. Environ. Impact 1, 349–358. doi: 10.2495/eid120311
Bouyoucos, G. J. (1962). Hydrometer method improved for making particle size analyses of soils. Agro. J. 54, 464–465.
Breder, R. (1982). Optimization studies for reliable trace metal analysis in sediments by atomic absorption spectrometric methods. Fresenius’. Zeitschrift für analytische Chemie 313, 395–402. doi: 10.1007/bf00495841
Brunetti, C., Di Ferdinando, M., Fini, A., Pollastri, S., and Tattini, M. (2013). Flavonoids as antioxidants and developmental regulators: relative significance in plants and humans. Int. J. of Mol. Sci. 14, 3540–3555. doi: 10.3390/ijms14023540
Celis-Hernandez, O., Villoslada-Peciña, M., Ward, R. D., Bergamo, T. F., Perez-Ceballos, R., and Girón-García, M. P. (2022). Impacts of environmental pollution on mangrove phenology: Combining remotely sensed data and generalized additive models. Sci. Total Environ. 810:152309 doi: 10.1016/j.scitotenv.2021.152309
Costa, F. D. N., da Silva, M. D., Borges, R. M., and Leitao, G. G. (2014). Isolation of phenolics from Rhizophora mangle by combined counter-current chromatography and gel-filtration. Nat. Prod. Commun. 9, 1729–1731.
Chen, K., Cai, M., Wang, Y., Chen, B., Li, X., Qiu, C., et al. (2020). Organochlorine pesticides in sediment of zhang river estuary mangrove national natural reserve: The implication of its source change in China’s mangroves. Sustain. 12:3016 doi: 10.3390/su12073016
Diaz, J. S., Flores-Miranda, M. C., Almaraz-Abarca, N., Fierro-Coronado, A., Luna-Gonzalez, A., Garcia-Ulloa, M., et al. (2021). Effect of microencapsulated phenolic compound extracts of maclura tinctoria (L.) steud on growth performance and humoral immunity markers of white leg shrimp (litopenaeus vannamei, boone, 1931) juveniles. Spanish J. Agri. Res. 19:e0604 doi: 10.5424/sjar/2021191-16505
Eqani, S. A.-M.-A.-S., Malik, R. N., and Mohammad, A. (2011). The level and distribution of selected organochlorine pesticides in sediments from River Chenab, Pakistan. Environ. Geochem. Health 33, 33–47. doi: 10.1007/s10653-010-9312-z
Glasenapp, Y., Korth, I., Nguyen, X. V., and Papenbrock, J. (2019). Sustainable use of mangroves as sources of valuable medicinal compounds: Species identification, propagation and secondary metabolite composition. South Afr. J. Bot. 121, 317–328. doi: 10.1016/j.sajb.2018.11.020
Granados-Galván, I. A., Rodríguez-Meza, D. G., Luna-González, A., and González-Ocampo, H. A. (2015). Human health risk assessment of pesticide residues in snappers (Lutjanus) fish from the Navachiste Lagoon complex. Mexico. Mar. Poll. Bull. 97, 178–187. doi: 10.1016/j.marpolbul.2015.06.018
Haq, M., Sani, W., Hossain, A. B. M. S., Taha, R. M., and Monneruzzaman, K. M. (2011). Total phenolic contents, antioxidant and antimicrobial activities of Bruguiera gymnorrhiza. J. Med. Plants Res. 5, 4112–4118.
Ji, Z., Long, Z., Zhang, Y., Wang, Y., Qi, X., Xia, X., et al. (2021). Enrichment differences and source apportionment of nutrients, stable isotopes, and trace metal elements in sediments of complex and fragmented wetland systems. Environ. Poll. 289:117852. doi: 10.1016/j.envpol.2021.117852
Jithesh, M. N., Prashanth, S. R., Sivaprakash, K. R., and Parida, A. (2006). Monitoring expression profiles of antioxidant genes to salinity, iron, oxidative, light and hyperosmotic stresses in the highly salt tolerant grey mangrove, Avicennia marina (Forsk.) Vierh. by mRNA analysis. Plant Cell Rep. 25, 865–876. doi: 10.1007/s00299-006-0127-4
Krishnamoorthy, M., Sasikumar, J. M., Shamna, R., Pandiarajan, C., Sofia, P., and Nagarajan, B. (2011). Antioxidant activities of bark extract from mangroves, Bruguiera cylindrica (L.) Blume and Ceriops decandra Perr. Ind. J. Pharmacol. 43, 557–562. doi: 10.4103/0253-7613.84972
Lattanzio, V., Cardinali, A., and Linsalata, V. (2012). “Plant phenolics: a biochemical and physiological perspective,” in Recent Advances in Polyphenol Research, eds V. Cheynier, P. Sarni-Manchado, and S. Quideau (London, UK: John Wiley & Sons), 364.
Lewis, M., Pryor, R., and Wilking, L. (2011). Fate and effects of anthropogenic chemicals in mangrove ecosystems: a review. Environ. Poll. 159, 2328–2346. doi: 10.1016/j.envpol.2011.04.027
MacFarlane, G. R., Koller, C. E., and Blomberg, S. P. (2007). Accumulation and partitioning of heavy metals in mangroves: a synthesis of field-based studies. Chemosphere 69, 1454–1464. doi: 10.1016/j.chemosphere.2007.04.059
Martínez-Álvarez, I., Leyva-Madrigal, K. Y., Maldonado-Mendoza, I., Jaramillo-Flores, M. E., and González-Ocampo, H. (2019). In vitro Antifungal Effect of Mangrove extracts on Fusarium verticillioides Isolates. Ind. J. Pharm. Sci. 81, 181–187.
Naranjo-Sánchez, Y. A., and Troncoso-Olivo, W. (2008). Contents of cadmium, copper, zinc, and lead in organs of Rhizophora mangle in river Sevilla mouth-Ciénaga Grande de Santa Marta, Colombian Caribbean [Contenidos de cadmio, cobre, zinc y plomo en órganos de Rhizophora mangle de la ciénaga grande de Santa Marta, Caribe Colombiano]. Boletín Investigaciones Marinas y Costeras 37, 107–129.
Ortíz, I., Avila-Chávez, M. A., and Torres, L. G. (2014). Pesticides in Mexico: uses, risks and regulatory framework [Plaguicidas en México: usos, riesgos y marco regulatorio]. Revista Latinoamericana de Biotecnología Ambiental y Algal 5:3. doi: 10.7603/s40682-014-0003-9
Parra, J. P., and Espinosa, L. F. (2008). Distribution of heavy metals (Pb, Cd, and Zn) in sediment profiles associated to Rhizophora mangle in Sevilla River - Ciénaga Grande de Santa Marta, Colombia [Distribución de metales pesados (Pb, Cd y Zn) en perfiles de sedimento asociado a Rhizophora mangle en el Río Sevilla - ciénaga grande de Santa Marta, Colombia]. Boletín de Investigaciones Marinas y Costeras - INVEMAR 37, 95–110.
Pawlak, K., Bylka, W., Jazurek, B., Matlawska, I., Sikorska, M., Manikowski, H., et al. (2010). Antioxidant activity of flavonoids of different polarity, assayed by modified ABTS cation radical decolorization and EPR technique. Acta Biologica Cracoviensia Series Botanica 52, 97–104.
Ponce-Vélez, G., and Botello, A. V. (2018). Organochlorine pesticides in coastal and marine organisms of the Mexican coastlines: a review [Plaguicidas organoclorados en organismos costeros y marinos de los litorales mexicanos: una revisión]. Revista Internacional de Contaminación Ambiental 34, 81–98. doi: 10.20937/rica.2018.34.esp02.07
Ramírez-Ayala, E., Arguello-Pérez, M. A., Tintos-Gómez, A., Pérez-Rodríguez, R. Y., Díaz-Gómez, J. A., Borja-Gómez, I., et al. (2020). Review of the biomonitoring of persistent, bioaccumulative, and toxic substances in aquatic ecosystems of Mexico: 2001-2016. Lat. Am. J. Aquat. Res. 48, 705–738. doi: 10.3856/vol48-issue5-fulltext-2461
Reyes-Montiel, N. J., Santamaría-Miranda, A., Rodríguez-Meza, G. D., Galindo-Reyes, J. G., and González-Ocampo, H. A. (2013). Concentrations of organochlorine pesticides in fish (Mugil cephalus) from a coastal ecosystem in the southwestern gulf of California. Biol. Environ. Proc. R. Irish Acad. 113B, 1–11. doi: 10.3318/BIOE.2013.25
Rico, C., Peralta-Videa, J., and Gardea-Torresdey, J. (2015). “Chemistry, Biochemistry of Nanoparticles, and Their Role in Antioxidant Defense System in Plants,” in Nanotechnology and Plant Sciences M. H. Siddiqui, M. H. A. Whaibi, and F. Mohammad (Berlin: Springer), 1–17. doi: 10.1007/978-3-319-14502-0_1
Rodríguez-Meza, D., Choumiline, E. Méndez-Rodríguez, L. Acosta-Vargas, B., Sapozhnikov, D. Funes-Rodríguez, R., et al. (2007). “Chemical composition of sediments and macroalgae of the lagoon complex “Magdalena-Almejas” [Composición química de los sedimentos y macroalgas del complejo lagunar Magdalena-Almejas],” in Ecological Studies in Magdalena Bay [Estudios Ecológicos en Bahía Magdalena], eds R. Funes Rodríguez, J. Gómez Gutiérrez, and R. Palomares García (Ciudad de México: CIBNOR-CICIMAR, IPN), 61–82.
Saiga, A., Tanabe, S., and Nishimura, T. (2003). Antioxidant Activity of Peptides Obtained from Porcine Myofibrillar Proteins by Protease Treatment. J. Agri. Food Chem. 51, 3661–3667. doi: 10.1021/jf021156g
Sanchez, J., Melchor, G., Martinez, G., Escobar, A., and Faure, R. (2006). Antioxidant activity of Rhizophora mangle bark. Fitoterapia 77, 141–143. doi: 10.1016/j.fitote.2005.11.011
Shivashankara, K., Pavithra, K., and Geetha, G. (2016). “Antioxidant Protection Mechanism During Abiotic Stresses,” in Abiotic Stress Physiology of Horticultural Crops N.K. Srinivasa, K.S. Rao, and R.H. Shivashankara Laxman (Berlin: Springer), 47–69.
Shumilin, E., Rodríguez Meza, G. D., Sapozhnikov, D., Lutsarev, S., and Murrillo de Nava, J. (2005). Arsenic Concentrations in the Surface Sediments of the Magdalena–Almejas Lagoon Complex, Baja California Peninsula, Mexico. Bull.Environ. Contam. Toxicol. 74, 493–500. doi: 10.1007/s00128-005-0612-4
Sivanadanam, V., Neelamegam, R., and Chellappan, K. (2012). Salinity effect: IX on catalase, polyphenol oxidase and peroxidase enzyme activity in Rhizophora apiculata blume and Acanthus ilicifoliuslinn seedlings. Plant Arch. 12, 195–199.
Souza, D. C., Morozesk, M., Duarte, I. D., Bonomo, M. M., Rocha, L. D., Furlan, L. M., et al. (2014). Matching pollution with adaptive changes in mangrove plants by multivariate statistics. A case study, Rhizophora mangle from four neotropical mangroves in Brazil. Chemosphere 108, 115–124. doi: 10.1016/j.chemosphere.2014.02.066
Sujitha, S. B., Jonathan, M. P., Aurioles-Gamboa, D., Campos Villegas, L. E., Bohórquez-Herrera, J., and Hernández-Camacho, C. J. (2019). Trace elements in marine organisms of Magdalena Bay. Pacific Coast of Mexico: Bioaccumulation in a pristine environment. Environ. Geochem. Health 41, 1075–1089. doi: 10.1007/s10653-018-0198-5
Sultana, B., Anwar, F., and Ashraf, M. (2009). Effect of extraction solvent/technique on the antioxidant activity of selected medicinal plant extracts. Molecules 14, 2167–2180. doi: 10.3390/molecules14062167
Taylor, S. R. (1964). Abundance of chemical elements in the continental crust: a new table. Geochimica et Cosmochimica Acta 28, 1273–1285. doi: 10.1016/0016-7037(64)90129-2
Templeton, J. (2020). “Stockholm Convention on Persistent Organic Pollutants,” in Essential Concepts of Global Environmental Governance J. F. Morin and A. Orsini (London: Routledge), 242–243. doi: 10.1016/j.chemosphere.2018.12.098
Usepa (1995). Method 508, Revision 3.1. Determination of chlorinated pesticides in water by gas chromatography with an electron capture detector. Washington, D.C: United States Environmental Protecction Agency
Usepa (1996a). Method 3600C, Revsion 3: Cleanup, part of Test Methods for Evaluating Solid Waste, Physical/Chemical Methods. Washington, D.C: United States Environmental Protecction Agency
Usepa (1996b). in Method 3660B, Revision 2: Sulfur Cleanup, part of Test Methods for Evaluating Solid Waste, Physical/Chemical Methods, Washington, D.C: United States Environmental Protecction Agency
Usepa (1998). Method 8270d, Revision 4. Semivolatile organic compounds by gas chromatography/mass spectrometry. Washington, D.C: United States Environmental Protecction Agency
Usepa (2007a). Method 3500C, Revision 3: Organic Extraction and Sample Preparation, part of Test Methods for Evaluating Solid Waste, Physical/Chemical Methods. Washington, D.C: United States Environmental Protecction Agency
Usepa (2007b). Method 3550C, Revision 3: Ultrasonic Extraction, part of Test Methods for Evaluating Solid Waste, Physical/Chemical Methods. Washington, D.C: United States Environmental Protecction Agency
Usepa (2007c). Method 8081B, Revision 2: Organochlorine Pesticides by Gas Chromatography, part of Test Methods for Evaluating Solid Waste, Physical/Chemical Methods. Washington, D.C: United States Environmental Protecction Agency.
Vagiri, M., Conner, S., Stewart, D., Andersson, S. C., Verrall, S., Johansson, E., et al. (2015). Phenolic compounds in blackcurrant (Ribes nigrum L.) leaves relative to leaf position and harvest date. Food Chem. 172, 135–142. doi: 10.1016/j.foodchem.2014.09.041
Vasavada, N. (2014). One-way ANOVA (Analysis of variance) with post-hoc Tukey HSD (Honestly Significant Difference). Test Calculator for comparing multiple treatments.. Available online at: http://astatsa.com/OneWay_Anova_with_TukeyHSD/ (Accessed on APR 04 2016)
Vasavilbazo-Saucedo, A., Almaraz-Abarca, N., González-Ocampo, H. A., Ávila-Reyes, J. A., González-Valdez, L. S., Luna-González, A., et al. (2018). Phytochemical characterization and antioxidant properties of the wild edible acerola Malpighia umbellata Rose. CyTA J. Food 16, 698–706. doi: 10.1080/19476337.2018.1475424
Walkley, A., and Black, I. (1934). An examination of Degtjareff method for determining soil organic matter and a proposed modification of the chromic acid in soil analysis. 1. Exper. Soil Sci. 79, 459–465.
Wongsen, W., Bodhipadma, K., Noichinda, S., and Leung, D. (2013). Relationship between leaf position and antioxidant properties in three basil species. Int. Food Res. J. 20, 1113–1117. 5p
Keywords: phenolic compound, antioxidant, chelating activity, Navachiste, Terminos Lagoon, Bahía Magdalena
Citation: González-Ocampo HA, Martínez-Álvarez IG, Jaramillo-Flores ME and Luna-González A (2022) Comparison of Phenolic and Flavonoid Content and Antioxidant and Chelating Activities of Rhizophora mangle in Different Anthropogenically-Polluted Coastal Lagoons. Front. Mar. Sci. 9:791748. doi: 10.3389/fmars.2022.791748
Received: 11 October 2021; Accepted: 21 February 2022;
Published: 07 April 2022.
Edited by:
Naser A. Anjum, Aligarh Muslim University, IndiaReviewed by:
Wilson Thau Lym Yong, Universiti Malaysia Sabah, MalaysiaNidal Jaradat, An-Najah National University, Palestine
Copyright © 2022 González-Ocampo, Martínez-Álvarez, Jaramillo-Flores and Luna-González. This is an open-access article distributed under the terms of the Creative Commons Attribution License (CC BY). The use, distribution or reproduction in other forums is permitted, provided the original author(s) and the copyright owner(s) are credited and that the original publication in this journal is cited, in accordance with accepted academic practice. No use, distribution or reproduction is permitted which does not comply with these terms.
*Correspondence: Héctor Abelardo González-Ocampo, aGdvY2FtcG9AeWFob28uY29t; Iván Guadalupe Martínez-Álvarez, aWdtYV9iaW9sb2dpQGhvdG1haWwuY29t
†These authors have contributed equally to this work