- 1ArcticNet, Québec Océan, Département de Biologie, Université Laval, Quebec City, QC, Canada
- 2Benthic Communities Group, Institute of Marine Research, His, Norway
- 3Indian Ocean Marine Research Centre, School of Biological Sciences, The University of Western Australia, Perth, WA, Australia
- 4Demersal and Benthic Sciences Division, Maurice Lamontagne Institute, Fisheries and Oceans Canada, Mont-Joli, QC, Canada
- 5Centro FONDAP de Investigación Dinámica de Ecosistemas Marinos de Altas Latitudes (IDEAL), Instituto de Ciencias Marinas y Limnológicas (ICML), Facultad de Ciencias, Universidad Austral de Chile, Valdivia, Chile
- 6Laboratorio Costero de Recursos Acuáticos de Calfuco (LCRAC), Facultad de Ciencias, Universidad Austral de Chile, Valdivia, Chile
- 7Arctic and Aquatic Research Division, Fisheries and Oceans Canada, Winnipeg, MB, Canada
- 8Centre for Earth Observation Science, Clayton H. Riddell Faculty of Environment, Earth, and Resources, University of Manitoba, Winnipeg, MB, Canada
- 9College of Fisheries and Ocean Sciences, University of Alaska Fairbanks, Fairbanks, AK, United States
- 10Department of Oceanography, Dalhousie University, Halifax, NS, Canada
The coastal zone of the Canadian Arctic represents 10% of the world’s coastline and is one of the most rapidly changing marine regions on the planet. To predict the consequences of these environmental changes, a better understanding of how environmental gradients shape coastal habitat structure in this area is required. We quantified the abundance and diversity of canopy forming seaweeds throughout the nearshore zone (5–15 m) of the Eastern Canadian Arctic using diving surveys and benthic collections at 55 sites distributed over 3,000 km of coastline. Kelp forests were found throughout, covering on average 40.4% (±29.9 SD) of the seafloor across all sites and depths, despite thick sea ice and scarce hard substrata in some areas. Total standing macroalgal biomass ranged from 0 to 32 kg m–2 wet weight and averaged 3.7 kg m–2 (±0.6 SD) across all sites and depths. Kelps were less abundant at depths of 5 m compared to 10 or 15 m and distinct regional assemblages were related to sea ice cover, substratum type, and nutrient availability. The most common community configuration was a mixed assemblage of four species: Agarum clathratum (14.9% benthic cover ± 12.0 SD), Saccharina latissima (13% ± 14.7 SD), Alaria esculenta (5.4% ± 1.2 SD), and Laminaria solidungula (3.7% ± 4.9 SD). A. clathratum dominated northernmost regions and S. latissima and L. solidungula occurred at high abundance in regions with more open water days. In southeastern areas along the coast of northern Labrador, the coastal zone was mainly sea urchin barrens, with little vegetation. We found positive relationships between open water days (days without sea ice) and kelp biomass and seaweed diversity, suggesting kelp biomass could increase, and the species composition of kelp forests could shift, as sea ice diminishes in some areas of the Eastern Canadian Arctic. Our findings demonstrate the high potential productivity of this extensive coastal zone and highlight the need to better understand the ecology of this system and the services it provides.
Introduction
Kelp forests are created by canopy-forming seaweeds of the order Laminariales and dominate cool-temperate and subarctic coasts (Steneck et al., 2002; Wernberg et al., 2019). These marine habitats play an important role in coastal ecosystem functioning and are declining in many regions globally (Krause-Jensen et al., 2018; Wernberg et al., 2019) due to a variety of drivers, including increased temperatures, overgrazing by sea urchins and overharvesting (Filbee-Dexter and Wernberg, 2018; Rogers-Bennett and Catton, 2019; Smale, 2020). Kelp forests in the Arctic are frequently underrepresented in overviews of these ecosystems and are not always considered in assessments of global change (Krause-Jensen et al., 2020; Starko et al., 2021). This is a remarkable omission considering that Arctic coasts make up a third of the world’s coastlines (Lantuit et al., 2012) and have extensive benthic areas where light levels can sustain benthic primary production (20% of the continental shelves <20 m) (Gattuso et al., 2006). Despite sea ice, cold temperatures and long periods with little to no daylight, these shallow areas can support lush marine vegetation such as kelps and other seaweeds when they receive enough light and suitable substrate occurs (Wiencke and Amsler, 2012; Filbee-Dexter et al., 2019).
The Arctic is experiencing dramatic environmental changes, which are driving shifts in marine ecosystems (Bryndum-Buchholz et al., 2020; Pecuchet et al., 2020). The entire region is an ocean warming hotspot with temperatures increasing 2–4 times faster than the global average IPCC, 2019; Meredith et al., 2019). Sea ice cover is becoming thinner and starting to break up earlier, reaching historic lows in the last decade (Cavalieri et al., 2020). The coastal zone is also the main recipient of increasing sediment fluxes from thawing permafrost and eroding continental shelves (Lantuit et al., 2012; Fritz et al., 2017), as well as increasing freshwater inputs from strong river discharge (Rood et al., 2017) and glacier melt (Van Wychen et al., 2020). Overall, these environmental changes are predicted to drive borealization (i.e., the northward shift of temperate species with warming), which includes “Arctic greening” as plants expand northward with land ice and sea ice retreat (Krause-Jensen and Duarte, 2014; Myers-Smith et al., 2020).
In the Arctic nearshore, sea ice cover and extreme environmental conditions can restrict both the abundance and productivity of seaweeds (Lee, 1980; Krause-Jensen et al., 2012; Filbee-Dexter et al., 2019). Sea ice restricts the upper limits of seaweeds by mechanical abrasion and restricts the lower depth limits by light shading (Wilce, 2016; Filbee-Dexter et al., 2019). A warmer Arctic with less sea ice is thus predicted to support larger, more productive kelp forests and extend the northern distributions of many kelp species (Krause-Jensen et al., 2012; Krause-Jensen and Duarte, 2014; Bartsch et al., 2016). Ocean warming is also predicted to make Arctic coasts better suited for kelps, because many species originate from the North Atlantic or Pacific oceans, and in the Arctic occur below their thermal optima (Wiencke and Clayton, 2011; Wulff et al., 2011). However, northern refugia exist, and some kelp species appear to have adapted to Arctic environments (Bringloe et al., 2020). Despite these predictions, the degree to which these changes will positively affect kelps will likely vary regionally and depends on both the kelp species in question and the extent that melting sea ice, glacial melt, and permafrost erosion increase turbidity and freshening in coastal areas, all of which limit seaweeds (Bartsch et al., 2016; Bonsell and Dunton, 2018; Traiger and Konar, 2018). Currently, a pan-Arctic review of existing long-term monitoring and field studies on vegetative marine ecosystems shows a trend of increasing abundance in response to climate change, but limited direct evidence of range expansions (Krause-Jensen et al., 2020). Knowledge of the abundance and distribution of kelp forests across a range of environmental conditions found in Arctic regions can provide insights on the future of these important ecosystems.
The current knowledge of kelp forests in the Eastern Canadian Arctic is based on diving research at a few sites (e.g., Chapman and Lindley, 1980; Cross et al., 1987; Sharp et al., 2008), occurrence data (Starko et al., 2021), and historical records of algal diversity (Lee, 1980). The region is biodiverse with 210 species of macroalgae reported (Archambault et al., 2010). However, most of the vast coastal zone is inaccessible because shore-based infrastructure and access (roads) are rare and use of large research vessels is limited to deeper waters. The Canadian Arctic is also colder than most other Arctic regions, largely due to the outflow of Arctic Ocean waters along the Canadian Arctic Archipelago and eastern shelf and into the North Atlantic (Michel et al., 2015). The shallow nearshore (depths of 0–5 m) in the Canadian High Arctic is often described as barren, scoured heavily by sea ice with few sessile invertebrates and little to no subtidal vegetation (Wilce, 2016; Renaud et al., 2021). Early phycologists and polar explorers reported some deeper subtidal areas with lush seaweeds, which typically became smaller and more fragmented from the east to the west and did not form extensive forests due to limited rocky substrata (Lee, 1973). Broader characterizations of patterns of the abundance, extent and structure of coastal vegetated habitats in this region are lacking (Wilce, 2016; Filbee-Dexter et al., 2019).
The objective of this study was to expand substantially our knowledge of kelp distribution, abundance, and biodiversity in the Eastern Canadian Arctic. To do so, we surveyed 55 sites, distributed across 3,000 km of linear coastline and nine distinct regions throughout the Eastern Canadian Arctic between 2011 and 2020, to quantify the abundance and diversity of kelp and other seaweeds. We asked the following research questions: (1) What is the extent and species composition of kelp forests in the Eastern Canadian Arctic? (2) Do kelp forests differ across distinct ecological regions in the study area? (3) How is the abundance of kelp influenced by environmental conditions, particularly gradients in sea ice and ocean temperature?
Materials and Methods
Study Area
The 55 sites fell within nine distinct geographic regions in the Eastern Canadian Arctic (Figure 1). We defined these regional groupings a priori based on Spalding et al.’s (2007) ecoregions, but further divided Eclipse Sound (North Baffin Island) from Baffin Bay, and separated Hudson Bay into four regions (Roes Welcome Sound, Hudson Strait, Foxe Basin, and Hudson Bay) using previously published biogeographical and oceanographic information for the area (Adey and Hayek, 2011; Bell and Brown, 2018; Brown et al., 2018). We made these further subdivisions to ensure regions captured differences between mid-Baffin Island and northern Eclipse Sound and across the complex range of environmental conditions and mixing water bodies within Hudson Bay. These known geographic and environmental boundaries (e.g., range discontinuities, dominant habitats, geomorphological features, currents, and sea temperatures) provide a solid basis against which to explore regional trends and test the impacts of targeted environmental variables known to be important for kelp species. Sites were selected with little or no prior knowledge of the local benthic community and many were sampled opportunistically during research campaigns that did not target kelp (Supplementary Table 1).
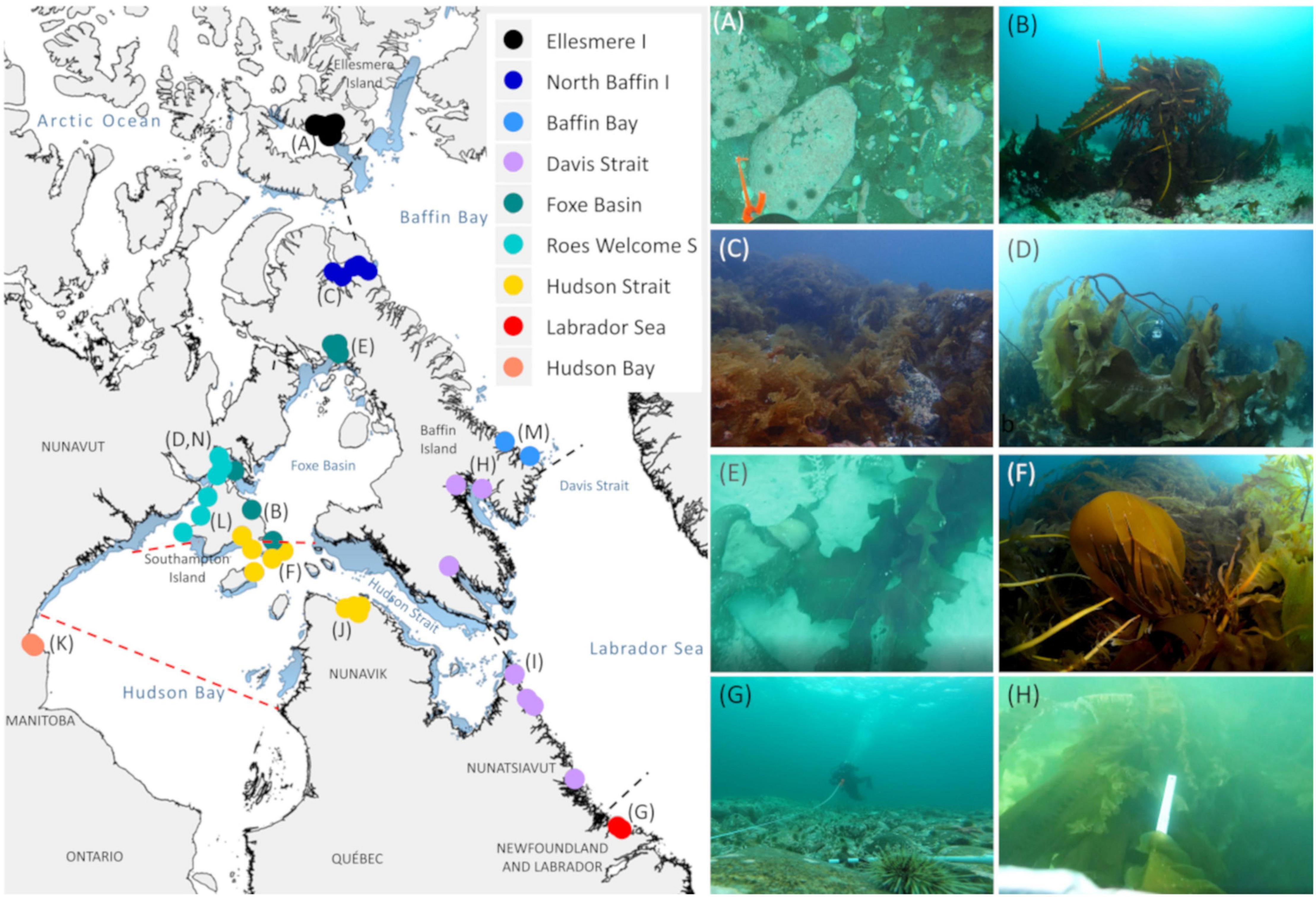
Figure 1. Location of study sites within the Eastern Canadian Arctic. Colors show distinct regions divided by Spalding et al. (2007) classification (dashed black lines) and known oceanographic features (Adey and Hayek, 2011; Brown et al., 2018) (red lines). Light blue shading shows polynya zones (WWF Global Arctic Program). Study site names and coordinates provided in Supplementary Table 1. Note some points are overlapping. Letters correspond to photographs showing diverse configurations of assemblages across regions. (A) Coralline algae with sea urchins and sparse Agarum clathratum 10 m depth in Ellesmere Island (Starnes Fiord; credit Eric Brossier); (B) Alaria esculenta on sand and pebbles in Foxe Basin (Southampton Island, credit Ignacio Garrido); (C) A. clathratum-dominated bedrock in north Baffin Island (Mittimatalik; credit Karen Filbee-Dexter); (D) “Floating” S. latissima forest rising over diver in Rose Welcome Sound (Southampton Island; credit Ignacio Garrido); (E) Sand with Laminaria solidungula and prostrate Saccharina latissima in Igloolik (Foxe Basin; credit: CAISN); (F) Hedophyllum nigripes and A. esculenta in Hudson Strait (Southampton Island; credit Ignacio Garrido); (G) Sea urchin barren devoid of erect algae at 10 m depth in Labrador (Makkovik; credit Kathleen MacGregor); and (H) S. latissima and A. esculenta forests in Davis Strait (Pangnirtung; Jonathan Fisher) and (I–N) in Supplementary Figure 1.
Extent and Species Composition of Kelp Forests
At all sites (Figure 1, Supplementary Figure 2, and Supplementary Table 1), we obtained kelp cover estimates from videos or photographs of the seafloor and canopy. At a subset of study sites, we collected additional measures of the biomass of seaweeds. Data obtained for all sites included percent cover, but sampling methods varied slightly across campaigns, depending on access and equipment (Supplementary Table 1). Specifically, Ellesmere Island was sampled by divers and video transects from the sailboat Vagabond in 2020. Northern Baffin Island was sampled by divers from a small fishing vessel in 2019. Southampton Island (Foxe Basin, Roes Welcome Sound, and Hudson Bay) was sampled by divers using an inflatable boat deployed off the R.V. William Kennedy in 2019. Sites in Baffin Bay and northern Labrador were sampled by divers using an inflatable boat deployed off the M.S. Cape Race in 2014. Pangnirtung (Davis Strait) was sampled by researchers from a fishing vessel in 2019 (Cumberland Sound Ecosystem Survey). Steensby Inlet (Foxe Basin), Deception Bay (Hudson Strait), Iqaluit (Davis Strait), and Churchill (Hudson Bay) sites were sampled by divers from small locally sourced vessels in 2011 and 2012 (Fisheries and Oceans Canada – DFO, Canadian Aquatic Invasive Species Network – CAISN) (Supplementary Table 1).
At the four sites in Ellesmere Island, the six sites in northern Baffin Island and the 13 sites in Southampton Island, divers laid one 30 m transect along 5, 10, and (at some sites) the 15 m depth contours (Supplementary Table 1). Along these transects, eight 1 m × 1 m quadrats were haphazardly placed and photographed. At each of the sites in Baffin Bay, Davis Strait and northern Labrador, divers laid one 30 m transect along the 5, 10, and if rock was still present below this, the 15 m depth contours. This was the only campaign that targeted sampling at 15 m based on the possible presence of kelp. The transect in these regions consisted of divers swimming ∼1 m above the canopy videoing the seafloor. A similar protocol was followed for the DFO-CAISN sites (in Steensby Inlet, Deception Bay, Iqaluit, and Churchill), except transects were 50-m long and covered a range of depths which were assigned to 5, 10, or 15 m depth in post processing (Supplementary Table 1). For Pangnirtung, photographs from 10 to 15 m were taken using a drop camera deployed multiple times from a small vessel at each depth. Photographs were analyzed for percent cover of all kelp species and other macroalgae using ImageJ (Schindelin et al., 2012). Video transects were analyzed by taking frame grabs (10–12 per transect) at regularly spaced intervals along the video (every ∼20–30 s depending on total video time). Only high-quality images with a clear view of the canopy or substratum were used. In ImageJ, we overlaid 48–50 points over each image and identified the seaweeds (or substratum) under each point and calculated percent covers (point count method). Seaweeds were separated into kelp species (Agarum clathratum, Alaria esculenta, Hedophyllum nigripes, Laminaria digitata, Laminaria solidungula, Saccharina latissima), Saccorhiza dermatodea, Desmarestia spp., Palmaria palmata, fucoids, fleshy red algae and other non-canopy forming brown fleshy and filamentous algae.
Seaweed biomass was sampled at 31 of the 55 sites. At these sites, we collected all macroalgae in four 0.25-m2 quadrats at each depth, haphazardly placed approximately 5 m apart, placed them in mesh bags and brought them to the ship or onshore to be processed. Plants were only collected if the holdfast fell within the quadrat. Small turf algae (e.g., Pessarrodona et al., 2021a) that could not be collected using a scraper and mesh bag were not collected. All collected seaweeds were identified to species or coarse macroalgal groups (red fleshy, non-canopy forming brown fleshy, filamentous) and weighed wet. Excess water was removed from small filamentous seaweeds with a paper towel. Total biomass of each macroalgal group was recorded for each quadrat, but in addition each kelp individual was weighed to the nearest gram. For sites from Cape Race, we estimated biomass from species density and individual sizes, using species-specific relationships between total length and biomass that we calculated from our other sites. To obtain estimates of kelp biomass at each site, we calculated the average kelp biomass for all sampling depths. For regional comparisons, means were calculated by averaging across sampled depths and then across all sites within a region.
To estimate the total standing stock of kelp (Tg C) in the Eastern Canadian Arctic we applied average kelp biomass per area in our study region to the total area of kelp in distribution models for this region by Goldsmit et al. (2021). We used wet weight biomass to carbon conversion ratios of 0.21 WW:DW and 0.3 DW:C (Pedersen et al., 2020; Pessarrodona et al., 2021b). We explored uncertainties in the modeled areal estimates by calculating total standing stock using different lower depth limits, from 10 to 50 m depth, which represent a range of possible areal kelp extents giving uncertainties in lower depth limits of kelp and the poor quality of available bathymetric data for much of the region.
For species identifications, we grouped Laminaria digitata and Hedophyllum nigripes because we were not confident of specific identifications determined in the field. Recent research indicates these two species cannot be distinguished using morphology alone (Dankworth et al., 2020). We classified Alaria species as A. esculenta in this study. However, genetic analyses on Alaria collected from Pond Inlet during this sampling campaign subsequently revealed this species to be a unique Arctic lineage of Alaria (Bringloe et al., 2021), which was also difficult to distinguish using morphology, so this A. esculenta grouping may be two species (A. esculenta and possibly a distinct species of Alaria).
Patterns of Seaweed Assemblages Across Regions
We examined patterns of kelp diversity using community characteristics (species richness, Shannon diversity, Pielou evenness) calculated for each region from biomass data, averaged for each of the 31 sites. Seaweed assemblage structure for all 55 percent cover sites and 31 biomass sites was also examined with multivariate analyses. First, species-level percent cover data and biomass data were analyzed with a three-factor hierarchical design (region, site, and depth) using permutational multivariate analysis of variance (PERMANOVA; Anderson et al., 2008), based on a Bray–Curtis similarity matrix generated from square-root transformed data. The transformation was chosen to down weight the influence of the most dominant species because we were working with abundance data. We used type III sums of squares to account for unbalanced design. Depth (5, 10, and 15 m) was a fixed factor nested in the site, a random factor. To examine differences in assemblages between regions (which were of specific interest and therefore a fixed factor), we examined multivariate variability within and between regions (estimated from the mean squares of the hierarchical PERMANOVA). A PERMDISP test was performed for each factor in the model to examine heterogeneity in multivariate dispersion between groups. The statistical significance of multivariate variance components was tested using a maximum of 9,999 permutations.
Metric multidimensional scaling (mMDS) ordinations, based on the Bray–Curtis similarity matrix, were generated to visualize multivariate patterns in seaweed assemblage composition among regions and sites (averaged across depth). Because decisions to sample transects at 5- vs. 15-m depth were based on the substratum and presence of kelp in some regions, we also analyzed regional averages from only the 10-m depth, which was consistently sampled, to see whether any depth sampling bias affected overall interpretations (e.g., the decision to sample 15 m depth during the Cape Race campaign targeted rock areas due to the greater chance of kelp). We further examined variation in assemblages between sites and regions for all data from 10-m depth using mMDS ordinations to explore effects on overall structure from the different sampling resolution at the three depths. All multivariate procedures reported here were performed using Primer 7 with the PERMANOVA add-on (Anderson et al., 2008).
Environmental Drivers of Seaweed Assemblages
Substrata were classified into bedrock, boulders, cobbles, pebbles, shells, and sand, using a simplified version of the Wentworth scale. For sites sampled by divers, substratum type was recorded in situ by visually estimating the percent of total substratum that was bedrock, boulders, cobbles, pebbles, shell, and sand. For sites with only video measures, substratum composition was estimated using the amount of visible seafloor in each frame. For frames with 100% canopy cover and no visible sea floor (7.45% of all frames), substratum type was estimated from nearest sections of video. This method could, however, underestimate rock cover at these sites, because it would be more likely to be covered by seaweed than sand. This concern was unlikely to matter in regions with sparse kelp cover where the seafloor was visible and we found high kelp cover on sand and pebbles substrata, as well as on rock. The percentage of red encrusting coralline algae on rock was also quantified by either divers or from underwater videos using the point count method. All estimates from sites with videos were verified using dive logs describing substratum types from each transect. For site level measures of substratum types, percent cover data were pooled across depths and averaged.
Other environmental layers with known relationships with seaweeds (Wiencke and Clayton, 2011; Assis et al., 2018a) were obtained from Bio-ORACLE v2.1 (Assis et al., 2018b). These included average sea temperature, light intensity (photosynthetically active radiation; PAR), salinity, open water days (percent of year with <10% sea ice cover), sea ice thickness, and phosphate and nitrate concentrations. We used estimates for the seafloor (minimum depth of the cell surrounding each study site apart from ice cover, thickness, and light intensity) using data from the nearest neighboring points to each study site and from present day conditions (2006–2018).
Spearman’s rank correlations were used to examine relationships of ordination scores for kelp species percent cover and biomass with abiotic variables (BIO-ENV analyses, PRIMER). The abiotic variables tested were sea ice cover, sea ice thickness, salinity, light, open water days, nitrate, % sand, and % rock (summed bedrock, boulder, cobble, pebble). All percent cover and biomass variables were averaged over depth and then over site to match the resolution of the abiotic variables. Variables were examined with Draftsman plots to check for skewness and multi-collinearity. If collinearity was present, we used only one of the variables in the analysis. All abiotic variables were normalized before the algorithm was run.
We also examined relationships between abiotic variables and seaweed communities using distance-based linear models (DistLM). To define the best fitted model, we used AICc and R2 values. DistLM models were coupled to a distance-based redundancy analysis (dbRDA; 999 permutations) to explore these relationships. For DistLM models we partitioned bedrock, boulders, cobble and pebbles into a single set of variables (“rock”). This analysis was also performed for data only from 10-m depth.
The relationships between specific abiotic variables of importance identified in BIO-ENV and DistLM analyses, and the abundance of the most dominant kelp species, were further explored using generalized additive models (GAM) with a Gaussian error distribution. Specifically, we examined the relationships between cover of A. clathratum and % cover of rock substrata, cover of S. latissima and sea ice thickness (m), and total kelp cover and salinity, which were identified as predictor variables of interest by the DistLM and dbRDA analyses. A GAM was fit to data from all study sites, averaged across depths at each site to match the resolution of the abiotic data. Models were fit using the gam function from the “mgcv” package (Wood, 2017) using a cubic spline smoother with k = 4 and 5, respectively. To verify k selection we fit models with increasing values of k (3, 4, 5, and 6), and extracted the deviance residuals to see if increasing k could better explain the patterns in our data. We finally explored spatial autocorrelation in our data using Moran’s I (test statistic 0.17, p = 0.063) using the weighted matrix method in (package “ape”). We performed these statistical analyses using R 4.1.0 (R Core Team, 2021).
Results
Widespread and Abundant: Extent and Species Composition of Arctic Kelp Forests
Kelp forests were found throughout the Eastern Canadian Arctic, covering on average 40.4% (±29.9 SD) of the seafloor across all sites and depths and ranging from 5 to 61.7% average cover across regions (Figure 2). The most common community configuration in each region was a mixed assemblage composed of four dominant kelp species: A. clathratum (14.9% ± 12.0 SD), S. latissima (13.0% ± 14.7 SD), A. esculenta (5.4% ± 1.2 SD), and L. solidungula (3.7% ± 4.9 SD) (Figure 2 and Supplementary Figure 3). The regions Hudson Strait, Roes Welcome Sound and Davis Strait had the highest average kelp cover (mean across sites and depths, 57.4, 46.0, and 44.1%, respectively). Other seaweeds also occurred in the regions, mainly filamentous brown algae, Desmarestia sp. and Fucus sp., which made up an average cover of 9.4% ± 19.6 SD, 1.3% ± 4.1 SD, and 1.8% ± 6.0 SD, respectively (Supplementary Figure 3). S. latissima in these regions had upright morphologies with floating hollow stipes [identified as Saccharina longicruris in Greenland (Krause-Jensen et al., 2012)]. Ellesmere Island and Hudson Bay had the lowest average kelp cover (7.9 and 5.0%, respectively) (Figure 2). Palmaria palmata and other red algae were mostly present in the Hudson Complex, including Foxe Basin, Hudson Strait, and Hudson Bay.
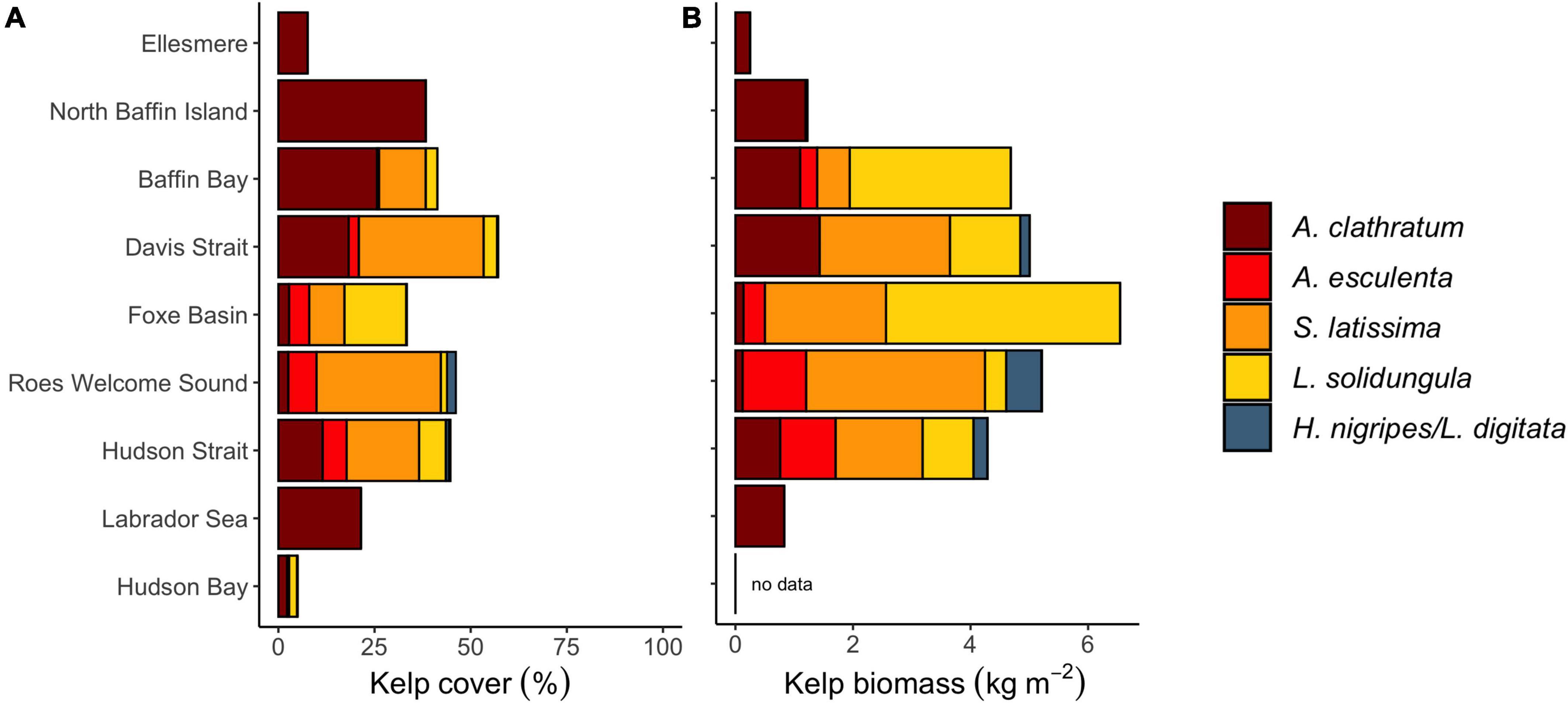
Figure 2. Average percent canopy cover (A) and biomass (B) of kelp species in different regions, averaged across depth and then site. Total bar length is total kelp cover or total biomass. Note biomass was only sampled at a subset of sites with percent cover data.
Total standing macroalgal biomass ranged from 0 to 32 kg m–2 and averaged 3.7 kg m–2 (±0.6 SD) across all 31 sites. S. latissima and L. solidungula occurred at an average biomass (±SD) of 1.2 ± 0.3 kg m–2 and 1.0 ± 0.4 kg m–2, respectively. H. nigripes averaged 0.2 ± 0.1 kg m–2 and A. esculenta 0.4 ± 0.2 kg m–2. A. clathratum occurred at a biomass averaging 0.7 ± 0.1 kg m–2, reached a maximum of 2.5 kg m–2 in the Labrador Sea, and tended to form lower-lying canopies. The highest average regional kelp biomass occurred in Foxe Basin, Roes Welcome Sound, and Davis Strait, while the lowest regional biomass was in the Labrador Sea and Ellesmere Island (Figure 2 and Supplementary Figure 3). Sites with the largest kelp biomass were dominated by S. latissima and L. solidungula, which often formed dense 2–5 m high canopies. The maximum site-level biomass for S. latissima was 7.8 kg m–2 at a site in Roes Welcome Sound and the maximum site-level biomass for L. solidungula was 10.5 kg m–2 in Foxe Basin. At the quadrat scale, maximum biomass was 34.4 kg m–2 for S. latissima, 23.9 kg m–2 for L. solidungula, 13.8 kg m–2 for H. nigripes, 18.5 kg m–2 for A. esculenta, and 5.6 kg m–2 for A. clathratum. The biomass of other seaweeds averaged 0.3 ± 0.1 kg m–2 across all sampled regions and reached a maximum of 3.6 kg m–2 in Roes Welcome Sound. Although Desmarestia sp. and other filamentous algae made up substantial cover at some sites, especially in Roes Welcome Sound and Foxe Basin, their biomass never exceeded 0.4 kg m–2 and 0.1 kg m–2, respectively.
Based the average biomass per area measured in our study (3.7 kg m–2) and predicted areal extent of kelp forests in waters 30 m or shallower (312,000 km2), the total standing stock for the Eastern Canadian Arctic is 72.7 (±12.3 SE) Tg C. However, this estimate changes substantially based on the lower depth limit of kelps, which if limited to 15 m depth resulted in a total areal extent of 158,577 km2 and standing stock of 50.2 (±8.5 SE) Tg C and if extended to 50 m depth increased to 490,126 km2 and a standing stock of 114 (±19.3 SE) Tg C (Supplementary Table 4).
Patterns of Seaweed Abundance and Diversity Across Regions
We found support for the hypothesis that kelps were restricted in cover at shallow depths of 5 m compared to 10 or 15 m, despite site-level variation in dominant species and abundance among depths in some areas (Supplementary Figure 3). Total kelp cover and biomass increased from 5 to 15 m (Table 1, Figure 3, and Supplementary Figures 3–5), often due to larger sized individual kelps at deeper depths. The average biomass per area (based on mean for each depth at each site) of S. latissima was 2.9× higher at 10–15 m compared to 5 m and that of L. solidungula was 1.7× higher at 10–15 m compared to 5 m. The largest S. latissima plants (3.2 kg ind–1) were collected from 15 m in Foxe Basin and Hudson Strait. The three largest A. esculenta plants (1.0, 1.0, and 2.2 kg ind–1) were collected from 15 m at three sites in Roes Welcome Sound and Hudson Strait and the largest L. solidungula plants (1.4, 1.3, and 1.6 kg) were found at 15 m in Roes Welcome Sound and 15 and 10 m in Foxe Basin. The largest A. clathratum plants (0.6, 0.6, and 0.7 kg ind–1) were collected from North Baffin Island at 10 m (the lowest depth sampled in that region).

Table 1. Permutational multivariate analyses of variance, based on Bray–Curtis similarity matrices of square-root transformed percent cover and average biomass (WW) data, at depth level and sites in each region.
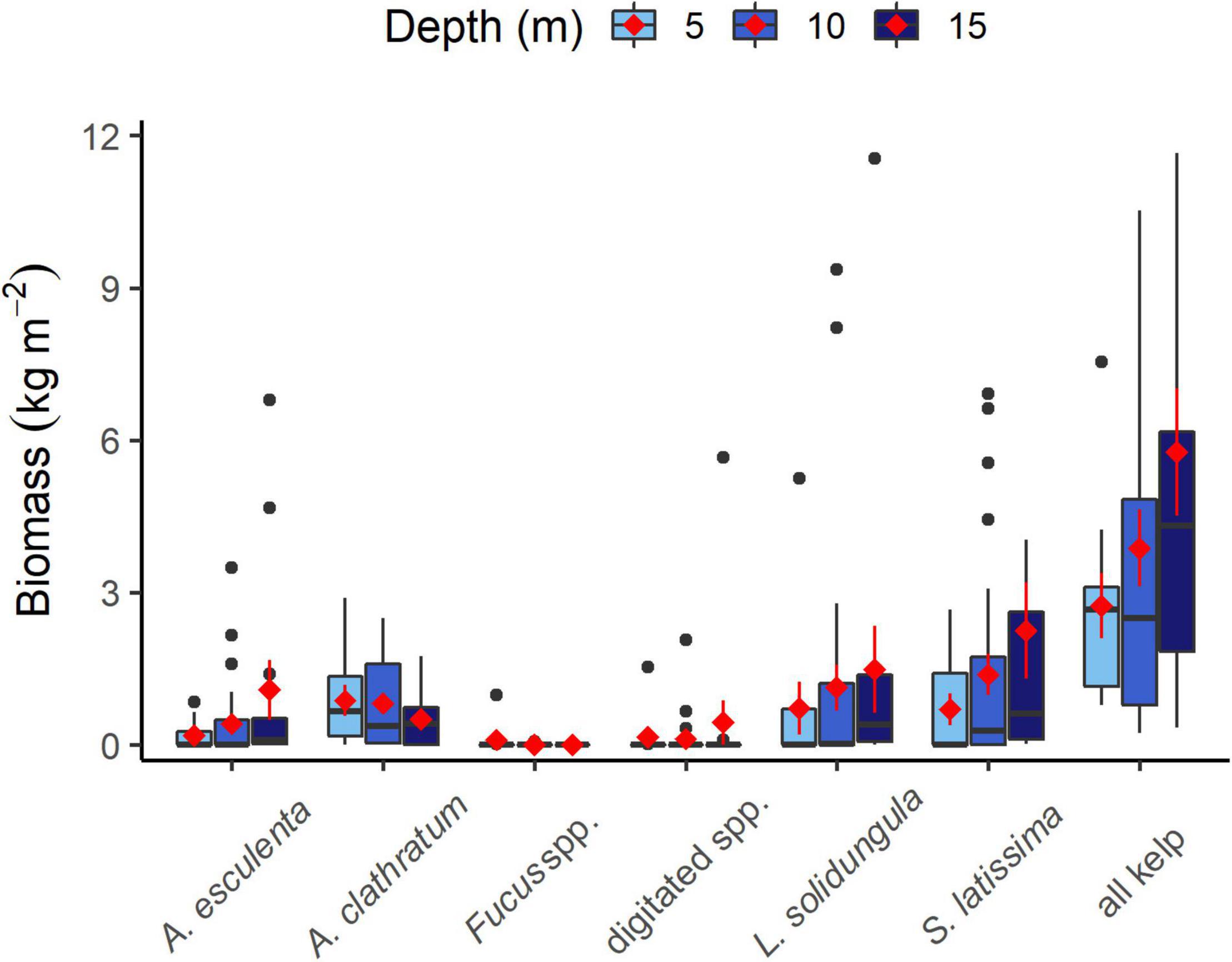
Figure 3. Biomass for kelp species at 5, 10, and 15 m depths, at all sites. Final column shows total kelp biomass. Red diamonds are average over all sites ± SE, black line is median. Upper and lower bars of boxplot show first and third quartiles, upper whiskers show 1.5 IQR. Digitated category includes Laminaria digitata and Hedophyllum nigripes.
Community assemblages differed significantly among regions and depths with respect to both cover and biomass (Table 1). A breakdown of the variance components for percent cover in the PERMANOVA indicated that variability at the smallest scales of the residual (e.g., among quadrats) (34.2%) and depth level (30.0%) were the major contributors to overall variability, compared to regions (24.0%). Variance components for biomass (square-root transformed) indicated that variability at the smallest scales of the residual (27.3%) contributed most to overall variability, compared to regions (17.5%) and depths (17.0%). Significant differences in multivariate dispersion for percent cover of kelp species were observed at the scale of depth (F2,823 = 4.2, P = 0.042), site (F51,771 = 13.87, P = 0.001), and region (F8,814 = 97.7, P = 0.001) and for biomass of kelp species at the scale of depth (F2,186 = 4.6, P = 0.026), site (F30,158 = 5.11, P = 0.001), and region (F7,181 = 24.2, P = 0.001). This variability in multivariate dispersion at various spatial scales suggests that the significant PERMANOVA tests may be either due to differences in species assemblages or to variation of species abundances at each of these spatial scales. Although depth significantly influenced kelp assemblages, mMDS using site-level percent cover and biomass data averaged across all depths, and site level data for just 10-m depth (which was consistently sampled at each site), showed similar overall patterns in cover and biomass, suggesting pooling across depths was appropriate to visualize similarities in kelp assemblages across the Eastern Canadian Arctic (Supplementary Figure 6).
A variety of kelp assemblages was found throughout the Eastern Canadian Arctic (Figure 1 and Supplementary Figures 1, 3, 4). The highest species richness and diversity were found in Roes Welcome Sound and Foxe Basin. The lowest values were found in Ellesmere Island and the Labrador Sea, where only A. clathratum was present. Roes Welcome Sound, Foxe Basin, Hudson Strait, and Davis Strait had the most species per site (Table 2). A. clathratum was dominant in the Ellesmere, North Baffin Island, Baffin Bay, and the Labrador Sea regions and was often the sole kelp species at a site. S. latissima and L. solidungula tended to co-exist and occurred at high biomass and cover at sites in Davis Strait, Foxe Basin, and Roes Welcome Sound. Prostrate morphologies were observed in North Baffin Island and the Labrador Sea. A. esculenta occurred across most regions but was rarely the dominant canopy former (apart from 1 site in Pangniqtuuq, Davis Strait) and instead was usually associated with other kelp species, particularly S. latissima (Figure 2 and Supplementary Figures 1, 4). S. latissima reached highest abundances in Davis Strait, Roes Welcome Sound and Foxe Basin. Two digitated kelp species, H. nigripes and L. digitata, occurred at low abundances and were often associated with S. latissima and L. solidungula, but at several sites in Roes Welcome Sound and Hudson Strait these digitated species formed significant biomass. These species have similar morphologies and were difficult to differentiate consistently in the field by multiple researchers across the five field campaigns and without genetic tools. However, based on what is known about their distribution (Bringloe et al., 2021; Savoie pers. comm.), H. nigripes was most likely present in Hudson Strait and Roes Welcome Sound, and L. digitata in Davis Strait. Fucus spp. were present at minor abundances at some sites (site average 0.013 ± 0.06 kg m–2), as were red algae (including Palmaria palmata; site average 0.08 ± 0.23 kg m–2), both of which tended to be at shallow depths (5 m). The Hudson Bay region had sites around Churchill, in a region with sparse rock substrate and high cover of filamentous algae. Labrador Sea sites were rocky reefs dominated by the sea urchin Strongylocentrotus droebachiensis with sparse A. clathratum except for an area located just south of the Torngats (Davis Strait), where kelp cover and biomass were high. These southern reefs supported similar communities to patchy A. clathratum kelp forests in north Baffin Island and Ellesmere Island, which both had high cover of red encrusting coralline algae.
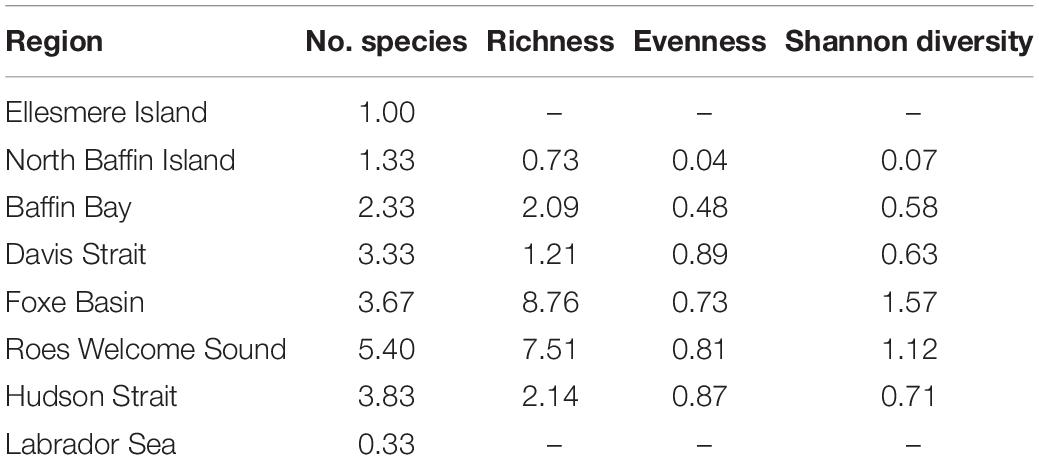
Table 2. Mean diversity indices – species number, richness, evenness, and Shannon diversity for regions from biomass collections (site measures are averaged across depths).
Environmental Associations With Seaweed Assemblages
Coastal zones across our study area were characterized by a diverse range of abiotic conditions, from subarctic to extreme high Arctic conditions. Average temperatures on the seafloor ranged from −1.17 to 1.45°C. Sea ice cover ranged from 50 days (13.6%) to 194 days (53.2%) of each year, with average sea ice thicknesses of 0.18–0.89 m and maximum thicknesses of 3.2 m in Ellesmere Island (Supplementary Figure 7). Nutrients were consistently low, ranging from 0.38 to 1.04 mol m–3 (phosphate) and 0.57 to 11.8 mol m–3 (nitrate). Salinity ranged from 27.2 to 34.2 and tended to decrease from east to west (Supplementary Figure 7), likely as a result of salinity differences between Atlantic and Arctic water masses, as well as freshwater inputs from rivers or land ice melt.
Results from BIO-ENV showed moderate correlations between the kelp assemblage similarity matrix and abiotic variables. The model selected sea ice thickness for the best environmental distance matrix related to the percent cover similarity matrix and salinity and % sand for the matrix best related to biomass. The DistLM model showed that the presence of rock substrata best predicted cover-based seaweed assemblages (explaining 25.0% of the total variation), and sea ice thickness as the second-best parameter (12.6%), followed by salinity (9.8%) as the third. Using a Bonferroni-corrected significance threshold of p = 0.0177 to account for multiple tests, every variable but temperature (p = 0.364) and nitrate concentration (p = 0.019) were significantly correlated with seaweed assemblages in marginal tests. The most parsimonious model consisted of sea ice thickness, salinity and rock and explained 40.5% of the total variation in species assemblages. The first two axis in the dbRCA analysis explained 87.8% of the fitted variation and 57.4% of the cumulative variation. The first axis was driven by gradients in rock and salinity, the second by sea ice thickness.
For sites with biomass data, seaweed assemblages were best explained by nitrate (31.6%) and salinity (28.1%) in marginal DistLM tests. The most parsimonious model consisted of nitrate, % sand and light, and explained 44.5% of the total variation in species assemblages. The first two axis in dbRCA analyses explained 70.7% of the fitted variation and 46.4% of the cumulative variation. The first axis was driven by gradients in nitrate and salinity, and the second by the cover of rock. Overall, dbRCAs show that sites with the least kelp cover and biomass were found in areas of thick sea ice and high nutrients. More A. clathratum tended to occur at sites with higher salinity and there was a weak relationship between kelp cover and sea temperature for some species, with S. latissima occurring at high biomasses at sites with higher sea temperatures compared to A. clathratum and to some extent L. solidungula (Figure 4). L. solidungula showed a weak, positive relationship with low light. These relationships changed slightly with kelp biomass compared to kelp cover, which was likely partially due to the reduced number of study sites with biomass compared to cover data.
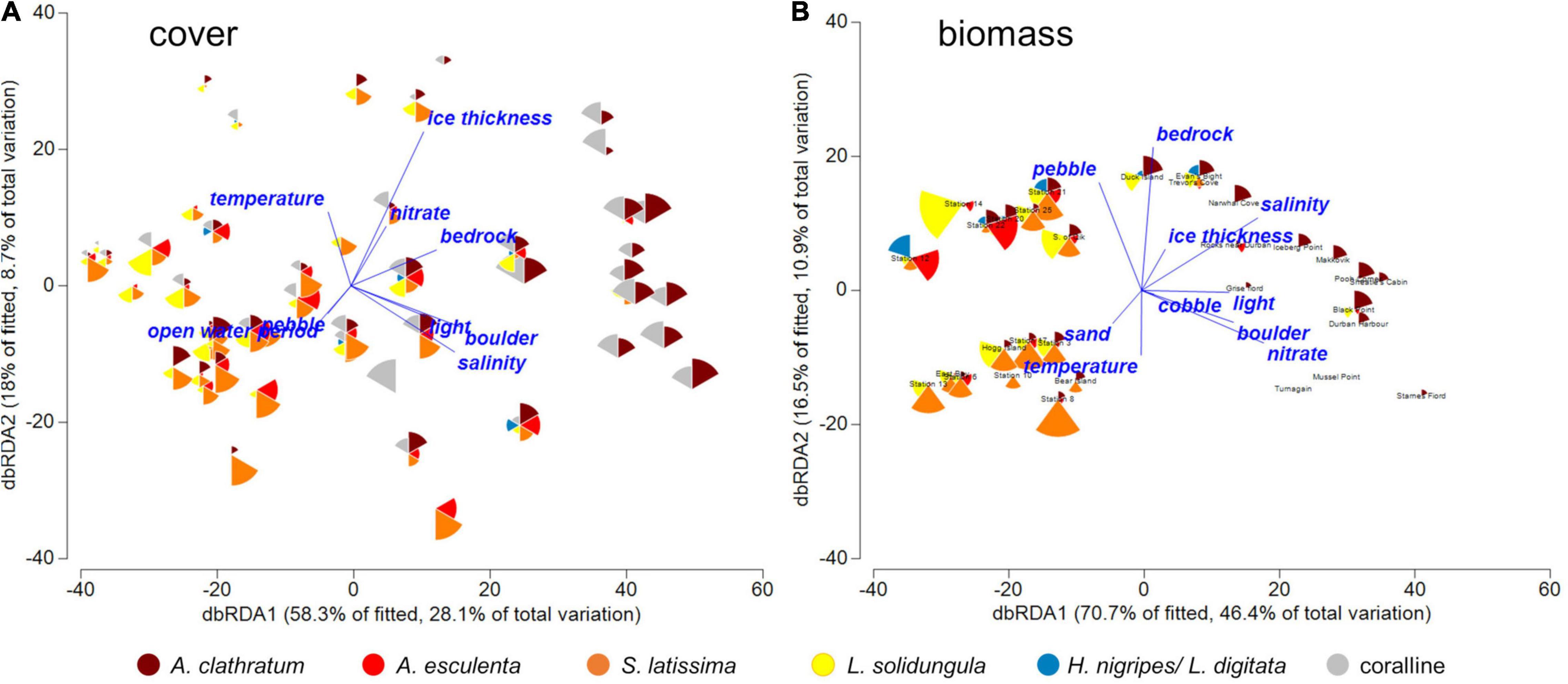
Figure 4. Seaweed assemblage relationship with abiotic variables across the Eastern Canadian Arctic. Data are presented for cover (A) and biomass (B), pooled over 5, 10, and 15 m depths. Points are based on dbRDA using Bray Curtis dissimilarity matrix of square-root transformed data. Vectors (blue) show correlations between ordinations for macroalgal assemblages and average environmental conditions and substrata at each site. Color and size of the pie sections shows the relative abundance of the five most dominant kelp species. The size of each piece indicates the percent cover and biomass of different species, from 0 to 100% or 0 to 10 kg m– 2. Note “sand” substrata is not shown for clarity in (A) because it occurs directly under “open water period.” For site key see Supplementary Table 1.
Many of the largest and most abundant kelp forests were found in areas with little sea ice. These occurred at lower latitudes along the northern Labrador coast (Torngats, Davis Strait) and at higher latitudes in polynya zones (Figure 1). The results of the GAM analysis further supported this relationship, with increasing S. latissima cover at sites with thinner sea ice and more open water days, but a sharp decrease in areas with the least amount of sea ice along the coast of Labrador, where sea urchin barrens were abundant (Figure 5 and Supplementary Figure 7). The northernmost sites in Grise Fiord supported little kelp and had high sea ice thicknesses (>0.8 m thick on average, Bio-ORACLE) and cover (>53%). At some sites in north Baffin Island, we observed evidence of iceberg groundings that created ∼0.5-m deep furrows along the seafloor and removed all upright macroalgae and appeared to bury coralline algae as well. The dbRCA also shows a negative correlation between sites with thick sea ice and sites with high kelp cover (Figure 4). However, this negative relationship with sea ice thickness did not hold for sites in northern Labrador, which had the most open water days and were often overgrazed by sea urchins with little to no macroalgal cover or biomass except for encrusting coralline algae.
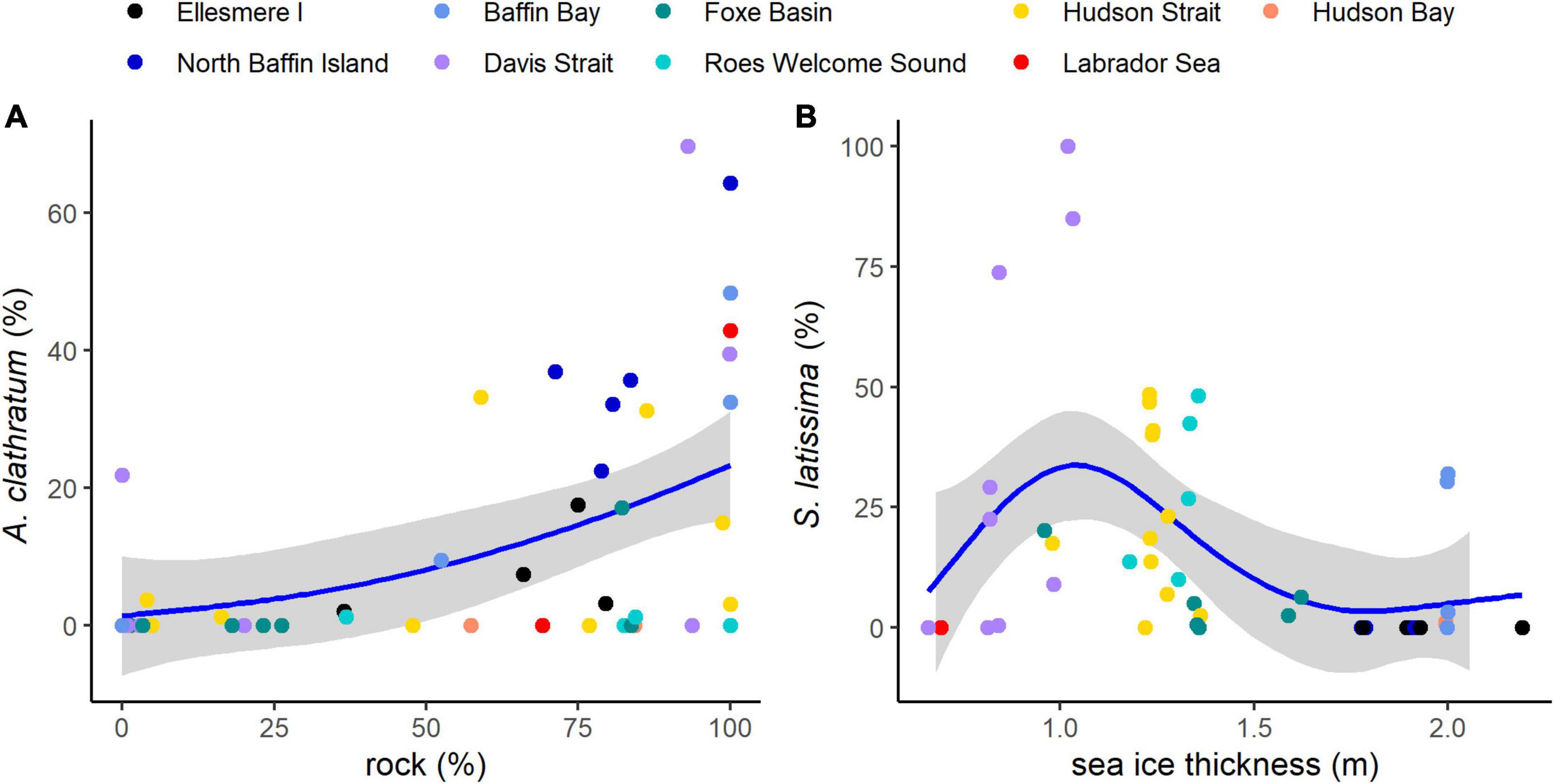
Figure 5. Relationships between (A) percent cover of Agarum clathratum and substrate (% rock), for each site, and (B) percent cover of Saccharina latissima and sea ice thickness. Colors of points show regions. Blue lines are single predictor Generalized Additive Models (GAMs) with 95% CI in gray shading.
Interestingly, kelp forests in the Eastern Canadian Arctic did not appear to require substantial rock substrata to form high cover and biomass habitats. Sand and pebbles covered an average of 41.6% (±3.9 SE) and 6.2% (±1.5 SE) of the seafloor across all sites and depths sampled, respectively, with a smaller percentage of the substratum composed of boulders 14.4% (±2.2 SE) and bedrock 16.4% (±3.0 SE) (Supplementary Figure 8). High cover of S. latissima, L. solidungula, and A. esculenta occurred in regions with sand or pebble substrata (Figure 4). These species showed a remarkable ability to attach to the seafloor in areas with minimal rock cover, and it was common to see a 5–10-m long plant attached by a small holdfast to several ∼2 cm pebbles. In contrast to Laminariaceae, A. clathratum occurred more frequently on predominantly rock substrata, often regardless of geographic region, which is supported by the GAM (Figure 5).
Discussion
Arctic kelp forests are relatively understudied compared to their temperate counterparts; yet they represent a substantial portion of the global distribution of these habitats (Filbee-Dexter et al., 2019; Starko et al., 2021). As Arctic coastal zones continue to change rapidly with climate shifts, the consequences for benthic coastal habitats are largely unknown, with little baseline data and sparse records in many regions. However, in some regions Arctic kelp forests are predicted to become increasingly abundant and productive in the future (Krause-Jensen et al., 2020), and this change will likely have important impacts on coastal ecosystem function and provision of services to humans (Wernberg et al., 2019). This study found that large stretches of the coastlines of the Eastern Canadian Arctic already support kelp forests, suggesting these productive seaweed habitats could currently dominate much of this extensive underwater region. Broad-scale field surveys like reported in this study, which allow the comparison of ecological conditions across different environmental conditions, can provide strong insight into how future climates might affect species and communities (Wernberg et al., 2012).
Inferring the underlying drivers of observed differences in kelp forests in the Eastern Canadian Arctic can be challenging because many potentially important factors covary in space and time. Kelp forests did not consistently decrease in biomass and extent from south to north, as expected from latitudinal patterns demonstrated in western Greenland and northern Europe (Krause-Jensen et al., 2012; Pessarrodona et al., 2018). Instead, biomass and cover varied regionally, with some sites at similar latitudes supporting either large canopies of S. latissima and L. solidungula forests, smaller canopies of A. clathratum, or sparse macroalgal assemblages intermixed with bare substrate. These differences suggest that latitudinal gradients in photoperiod are not the main driver of kelp forests in the region. This variation could be a result of the convoluted coastline, differences in substrata, local mixing of freshwater runoff and suspended sediments (McClelland et al., 2012) and the complex mixing of three water bodies in this area, originating from the Arctic Ocean, the North Atlantic, and the North Pacific (Michel et al., 2006). These three water bodies have different nutrient, temperature and salinity profiles, and their convergence creates gradients of environmental conditions that may drive localized patterns of kelp abundance within regions. Available rock substrata and the presence of grazers may also alter broader predictions of the poleward expansion of kelp and may limit the extent that sea ice loss and warming sea temperature will increase kelp abundance in some areas.
Kelp Community Composition and Substratum
It is widely believed that kelp forests rely on rock substrata to thrive, yet sand-dominated substratum with little rock (e.g., gravel or scattered cobbles) was enough to support some of the largest kelp forests in the Eastern Canadian Arctic. This observation aligns with a growing recognition that macroalgae can dominate sedimentary habitats, which has consequences for macroalgal burial and potential long-term carbon storage (Ortega et al., 2019). There was evidence of detachment in sedimentary areas, with numerous free-floating S. latissima observed on the sea surface in waters surrounding these forests in Roes Welcome Sound and Foxe Basin. It is possible that kelps attached to sand or pebbles could become unstable and more prone to dislodgement when they lose the protective cover of sea ice and are increasingly exposed to ocean storms and waves (Filbee-Dexter and Scheibling, 2012; Bonsell and Dunton, 2018).
The northern dominance of A. clathratum in the study area was unexpected. This species appears to be the proverbial “last one standing” at the most extreme limits of the Eastern Canadian Arctic, found farther north than even the endemic Arctic species L. solidungula. It is not clear what is driving dominance of this rather understudied species in this region. Along more temperate coasts in the north Atlantic, A. clathratum is typically restricted to deep waters and outcompeted by faster-growing kelps such as L. digitata, S. latissima, and A. esculenta (Adey and Hayek, 2011; Simonson et al., 2015). Perhaps its tolerance of greater depths and ability to develop during cool temperatures (Vadas, 1968; Witman and Lamb, 2018) allows A. clathratum to better survive in cool regions with high cover of thick sea ice because it has lower requirements for light and warm temperatures. It’s abundance was also strongly related to the amount of rock, and patterns of abundance may be partially due to differences in dominant substrata. High densities of sea urchins could also favor the survival of A. clathratum, which has defenses against grazing Dubois and Iken, 2012), enabling it to persist while less defended kelp species are grazed. This may explain the dominance of A. clathratum in the Labrador Sea, where sea urchins were abundant.
Unlike A. clathratum, patterns of abundance and extent of A. esculenta – which was found at almost all sites throughout the Arctic – were similar to those observed in temperate regions, and this species never dominated the community, but rather tended to be part of mixed canopies. A. esculenta is an opportunistic kelp species with high dispersal ability, that is often the first kelp to colonize during macroalgal succession (Hawkins and Harkin, 1985; Campana et al., 2009). It can eventually be outcompeted by other kelp species, such as S. latissima or L. digitata, or experience higher rates of grazing. Although its high dispersal strategy could explain its high prevalence across the Arctic, this species may represent multiple subspecies or distinct populations throughout the region (Bringloe et al., 2021), suggesting local adaptation.
Kelp Communities and Environmental Conditions
Despite the complicated relationship of kelp cover and biomass with latitude, sea ice cover and thickness appeared to shape kelp forests in the Eastern Canadian Arctic. This lack of a continuous latitudinal gradient in sea ice was partly due to polynyas, where currents and winds prevent consolidation of a contiguous sea ice cover, resulting in more open water days compared to nearby areas (Hannah et al., 2009; Melling et al., 2015). This created a mosaic of light availability across our study area. We saw this at mid-latitudes in Roes Welcome Sound, Hudson Strait and Davis Strait, where sites in polynyas or in areas with thin sea ice supported larger and more continuous kelp forests than nearby areas with thicker sea ice. There was also evidence of less kelp at shallower depths, which could be consistent with effects of intense sea ice scour or freshwater inputs. The trend of higher kelp biomass and cover under longer open-water conditions, shown by the positive relationship between abundance of high biomass S. latissima forests and open-water days, is consistent with expectations that sea ice limits the abundance of more temperate kelp species through shading and/or scour (Krause-Jensen and Duarte, 2014; Bartsch et al., 2017; Scherrer et al., 2019). This relationship has also been confirmed by species distribution models that found that sea ice thickness was one of the most important variables for predicting habitat suitability and percent cover of kelp forests in the Eastern Canadian Arctic (Goldsmit et al., 2021). Yet, individual kelp species appeared to be influenced more or less strongly by sea ice, suggesting that kelp forests in this region could be more likely to shift in species composition and cover compared to shifting in overall distribution as sea ice diminishes. Ocean temperature was not strongly correlated with seaweed cover, despite being a key predictor in global models of kelp distribution (Assis et al., 2016; Jayathilake and Costello, 2020). This finding is again consistent with regional models for the Eastern Canadian Arctic, which show that the percent cover of L. solidungula, S. latissima and A. clathratum was not well explained by temperature (Goldsmit et al., 2021). Minimum sea temperatures across our study sites were highly similar, bordering on freezing (−1.8°C) in most regions, with average sea temperatures less than 1.5°C, which could explain this pattern. Warm temperatures, and in particular marine heatwaves, are important stressors for kelp forests globally, but these losses frequently occur at warmer range edges (Wernberg et al., 2016; Rogers-Bennett and Catton, 2019; Filbee-Dexter et al., 2020b). In their more northern range limits, Arctic kelps typically experience temperatures well below thermal limits for mortality (Filbee-Dexter et al., 2019). Instead, sea urchin abundances and substratum type, which are variables that are not typically available for habitat suitability models, seem to influence percent cover, biomass and species composition in this region.
Although the surface waters in the Canadian Arctic are known to be nutrient poor and seaweeds thrive in nutrient-rich waters (Wernberg et al., 2019), nutrients did not appear to be good predictors of kelp. Nitrate and phosphate concentrations showed a negative relationship with overall kelp abundance. This relationship could be explained by the well-known nutrient storage capacity of Arctic kelp species, which may receive adequate nutrients from seasonal mixing or upwelling processes that bring nutrient-rich water to the surface in some areas for short periods of time (e.g., Chapman and Lindley, 1980) or seasonally (e.g., Henley and Dunton, 1997). The timing and intensity of such sporadic to periodic nutrient inputs may not have been properly captured by environmental layers sourced from Bio-Oracle annual means. Alternatively, nutrient-rich areas could support greater phytoplankton production and decrease light availability on the seafloor during the important spring/summer growth period. It is also difficult to make any strong inferences regarding the relationships between nutrients and kelp abundance, because nitrate and phosphate concentrations covary in space with other important environmental variables. Nutrients are highest in Lancaster Sound, the northern-most region around Ellesmere Island, where the shoal along Barrow Strait acts to mix waters of Pacific origin to the surface (Michel et al., 2006), and that also has high sea ice cover and strong seasonality in light, which both influence seaweed performance (Wiencke et al., 2007). Regions with high nutrient concentrations could also reflect higher turbidity (i.e., from terrestrial runoff), which leads to reduced light penetration and could contribute to the negative association with seaweed abundance (Aumack et al., 2007; Fritz et al., 2017; Traiger and Konar, 2018).
Variation in species composition of kelp forests between regions and across smaller-scale gradients of abiotic conditions within the study area also provides insights into what drives these communities and how these habitats could change in the future. Kelp forests with high biomass of S. latissima, L. solidungula, and A. esculenta at sites in polynya zones, where strong currents and/or winds keep water ice-free for most of the year (e.g., Hudson Strait, Roes Welcome Sound, and Davis Strait) (Hannah et al., 2009) could represent the productive kelp habitats that will become more widespread when sea ice retreats. These high-biomass forests may then develop in neighboring regions that have similar substrata (sediment and pebbles) but currently experience sea ice conditions that limit kelp growth. However, these zones are also associated with high water flow and vertical mixing that likely have a positive influence on the growth and abundance of seaweeds, limiting how well these areas may represent future ice-free regions.
In contrast to regional gradients of sea ice created by polynya zones, the broader latitudinal gradient of increased sea ice and sparse A. clathratum across the Eastern Canadian Arctic, moving northward along the rocky coast from Baffin Bay to north Baffin Island and Ellesmere Island, suggests that A. clathratum forests could increase along this northeastern coastline as sea ice retreats. This prediction is supported by models for the region under the extreme emission scenario (8.5 RCP) for years 2050 and 2100 (Goldsmit et al., 2021). Moreover, the more abundant rocky substrata found to the east in these regions appeared to favor A. clathratum, particularly at higher latitude sites, where it dominates almost all depths. Similarly, the trend of decreasing S. latissima (and to some extent L. solidungula) abundance from the relatively ice-free Davis Strait northward into Baffin Bay, suggests that these larger canopy-forming forests may be limited by high Arctic conditions. Yet, despite the close association of these species within our study sites, models based on their broader distributions predict that S. latissima will encounter more suitable northern habitat under future climate change scenarios than will L. solidungula, which is expected to decrease in the region (Goldsmit et al., 2021). If conditions in more northern regions converge with those currently found in subarctic locations, it is possible that these species could become more dominant in northern Baffin Bay, with S. latissima outcompeting A. clathratum at shallow depths. Replacement of A. clathratum with S. latissima would increase total kelp biomass and habitat structure (i.e., canopy height) in these areas. However, although our study region included southern Ellesmere Island, our sites did not capture the northern limit of kelp distribution, which could be located closer to areas covered by multi-year sea ice further north. The above environmentally driven predictions may differ if biological variables such as presence of grazers are taken into account; for example, increases in sea urchins can favor A. clathratum over other kelp species (Gagnon et al., 2003) as we observed in Labrador.
The relative importance of sea ice at the northernmost sites compared to sea urchin grazing pressure at the southernmost sites in the study region along the Labrador coast, aligns with ecological theory, where biological interactions become more important as you move toward the equator and environmental drivers are more critical toward the poles (Moles et al., 2011; Poore et al., 2012; Steneck et al., 2017). Sea urchin barrens in northern Labrador occurred at a known transition between temperate and subarctic conditions (Adey and Hayek, 2011; Merzouk and Johnson, 2011). If environmental conditions are creating this boundary change, sea urchins may overgraze erect macroalgae northward, as they have in other regions when oceans have warmed (Ling et al., 2009; Christie et al., 2019). A similar subarctic band of sea urchin barrens also occurs in Norway, Alaska, and eastern Russia (Konar and Estes, 2003; Norderhaug and Christie, 2009; Filbee-Dexter and Scheibling, 2014), but not in more high Arctic places like Greenland, Svalbard, the White Sea, and the Beaufort Sea (Krause-Jensen et al., 2020). This lack of extensive barrens in the northern Arctic is not because sea urchins were absent in these regions (they were common around northern Baffin Island and Ellesmere Island) but could instead be due to environmental conditions limiting destructive grazing behavior. For example, if cooler temperatures are suboptimal for S. droebachiensis, it may lead to smaller sea urchin sizes, reduced reproductive success and slower grazing rates (Scheibling et al., 2020). Alternatively, the presence of barrens in subarctic regions could reflect a legacy of high fishing pressure on the Newfoundland-Labrador shelves, which may have removed sea urchin predators such as large groundfish (e.g., Norderhaug et al., 2020b), although, no evidence of this association has been found for Labrador. Accurate predictions of how this boundary could shift will require ecophysiological tests of the relationship between sea urchins and environmental conditions, as well as a much better knowledge of predator abundance, bathymetry, dispersal ability and projections of future coastal conditions.
Arctic Kelp and Ecosystem Function
Kelp forests in the Eastern Canadian Arctic are estimated to currently cover 312,000 km2 of the coastal zone, in waters 30 m or shallower (Goldsmit et al., 2021), which is 9% of the estimated global distribution of kelp (1,500,000–2,500,000 km2) (Assis et al., 2020; Jayathilake and Costello, 2020). There are uncertainties around this estimate, yet the relatively high abundance and areal extent of these primary producers compared to phytoplankton and sea ice algal production along these coasts suggests that Arctic kelp forests could be an important source of primary production for marine communities (Krumhansl and Scheibling, 2012; Vilas et al., 2020; Pessarrodona et al., 2021b) and a standing stock of carbon. The total standing stock for the Eastern Canadian Arctic of 72.7 Tg C is 4.4× more than the standing stock of kelp forests in Australia (16.6 Tg C; Filbee-Dexter and Wernberg, 2020) and 10.2× the standing stock of kelp forests in Norway (158 million tonnes wet weight or 7.1 Tg C; Frigstad et al., 2021) (using FW:C ratios for Laminaria hyperborea from Pedersen et al., 2020). Annual productivity rates measured for S. latissima and L. solidungula at sites around Southampton Island in 2019 ranged from 23.1 to 67.8 g C m–2 y–1 (Filbee-Dexter, unpublished data) and for L. solidungula from Igloolik (Foxe Basin) in 1977 were 19.6 (±12.1 SD) g C m–2 y–1 (Chapman and Lindley, 1980). These measures of NPP are comparable to NPP for phytoplankton in Hudson Bay, Baffin Bay, and Labrador Sea (44–58 g C m–2 y–1) (Frey et al., 2020), 3.5× lower than estimates of combined ice algae and phytoplankton productivity for Hudson Bay (72 C m–2 y–1) (Matthes et al., 2021) and an order of magnitude lower than the productivity of most kelp forests (global average 516 ± 30 SE g C m–2 y–1; Pessarrodona et al., 2021b), yet the extensive area of kelp in the Eastern Canadian Arctic suggests these habitats are still cycling large quantities of carbon in the coastal zone, ranging from 2.2 to 6.4 Tg C y–1 and 10.4 to 30.6 Tg C y–1, based on a lower depth limit of 10 and 40 m, respectively (Supplementary Table 4).
The presence of kelp forests is likely significant for coastal productivity in the Eastern Canadian Arctic, as kelps provide food for coastal food webs through direct consumption by grazers (Franco et al., 2015; O’Brien and Scheibling, 2016; Wernberg et al., 2016; Filbee-Dexter et al., 2020a) or through detrital pathways (Duggins et al., 1989; Vanderklift and Wernberg, 2008; Vilas et al., 2020). Kelp forests also act as important nutrient filters, focal points for high biodiversity and carbon sinks (Krause-Jensen and Duarte, 2016; Teagle et al., 2017; Wernberg et al., 2019; Filbee-Dexter, 2020). In fact, the slower decomposition of kelp carbon at high latitudes could mean that kelp detritus in these regions has a greater chance to reach long-term sinks and be sequestered (i.e., long-term storage in the deep ocean) (Filbee-Dexter et al., 2021).
The spatial gradients in kelp biomass, cover and dominant species shown in this study likely have follow-on consequences for coastal ecosystem functioning in these regions. Benthic primary production is most likely higher in areas with higher kelp cover and biomass, as there is generally a positive relationship between total standing biomass and areal net primary production (NPP) for many kelp species (Pessarrodona et al., 2018; Pedersen et al., 2020). In regions characterized by a higher biomass and cover, more kelp carbon should be available for uptake by coastal food webs or carbon sequestration (Pessarrodona et al., 2018). The canopy coverage and height of kelp forests will also affect how these species “engineer” the local environment, altering the overall habitat structure. Kelp forests with high cover and biomass (“marine forests”) (Wernberg and Filbee-Dexter, 2019), often have more three-dimensional space for associated species to use for shelter and habitat and can support distinct understory communities through shading (Teagle et al., 2017; Norderhaug et al., 2020a). Thus, the sparse A. clathratum kelps in high latitude regions that do not form continuous canopies likely provide limited vertical habitat structure compared to larger S. latissima and L. solidungula forests farther south [although A. clathratum holdfasts in these regions do provide habitat for numerous species (Kimberly Howland, pers. obs.)]. Shifts in dominant kelp species in the Eastern Canadian Arctic, as well as overall increases in kelp abundance and geographic extent, could therefore alter coastal carbon cycles, coastal biodiversity and overall primary and secondary productivity of this extensive coastal zone.
Conclusion
Our results suggest that kelp forests are prevalent throughout the Eastern Canadian Arctic and form one of the dominant coastal ecosystems along this extensive coastline. Both the small-scale environmental gradients within regions of the Eastern Canadian Arctic and the broad range of abiotic and biotic conditions across the entire study area provide an initial yet nuanced understanding of what drives kelp community structure in this relatively unexplored region of the planet, highlighting the importance of sea ice, substratum type, and herbivory in shaping kelp assemblages. As sea ice becomes less prominent in some regions, Arctic kelp forests could experience a shift from abiotic drivers to more biotic drivers of community structure, such as herbivory and competition with other seaweeds that characterize more temperate kelp forests. Our findings are consistent with the hypothesis that kelp forests will expand in the Arctic under climate change but suggest that this expansion will largely be due to a change in dominant species or shifts in overall cover and biomass within existing ranges. This could represent a negative feedback mechanism on climate change, whereby less sea ice means more subsurface carbon capture from underwater seaweeds. Our findings also demonstrate the high potential productivity of this extensive coastal zone and highlight the need to better understand the ecology of these systems and the services they provide, particularly in the context of global change.
Data Availability Statement
The datasets presented in this study can be found in online repositories. The names of the repository/repositories and accession number(s) can be found below: https://github.com/robwschlegel/ArcticKelp/tree/master/Filbee-Dexter_et_al_FMARS.
Author Contributions
KF-D led the writing. KF-D, KAM, IG, LCdG, KLH, LEJ, CWM, CJM, and JG collected the data. KF-D, CL, RWS, PA, and KAM analyzed the data. PA, KF-D, LEJ, KLH, CJM, CWM, and BK acquired funding and conceived the study. CL, KAM, and KF-D created the graphics. All authors contributed to writing and editing the manuscript.
Funding
This work was supported by the ArcticNet through the ArcticKelp project (P101 ArcticKelp), Fisheries and Oceans Canada Arctic Climate Change Adaptation Strategy, Arctic Science and Aquatic Invasive Species Monitoring and Research Funds, Natural Sciences and Engineering Research Council (NSERC), NRCan Polar Continental Shelf Program Support, Canadian Aquatic Invasive Species Network (CAISN), Nunavut Marine Region Wildlife Management Board (NWMB), Nunavut Fisheries Association, Cumberland Sound Fisheries Ltd., Centre for Fisheries Ecosystems Research, Memorial University of Newfoundland, Oceans North, Institute of Marine Research, Norway. KF-D was supported by the Natural Sciences and Engineering Research Council of Canada (NSERC-PDF 516938-2018) and Australian Research Council (DE1901006192). BK was supported by National Science Foundation (NSF #1906726). Sampling around Southampton Island was funded by Marine Environmental Observation, Prediction and Response Network, Network of Centres of Excellence, Polar Knowledge Canada, and a NSERC ship time grant for the Southampton Island Marine Ecosystem Project (SIMEP) and by the Belmont Forum/BiodivERsA for the De-icing of Arctic Coasts: Critical or new opportunities for marine biodiversity and Ecosystem Services (ACCES) project.
Conflict of Interest
The authors declare that the research was conducted in the absence of any commercial or financial relationships that could be construed as a potential conflict of interest.
Publisher’s Note
All claims expressed in this article are solely those of the authors and do not necessarily represent those of their affiliated organizations, or those of the publisher, the editors and the reviewers. Any product that may be evaluated in this article, or claim that may be made by its manufacturer, is not guaranteed or endorsed by the publisher.
Acknowledgments
We are grateful to local people and organizations for providing sampling support: C. Basler, J. Batstone, and L. Fishback (community of Churchill); D. Kaludjak, K. Lindell, A. Williams, and J. Williams (community of Iqaluit); C. Alaku, C. Kadjulik, K. Ningiurluut, K. Okituk, C. Okituk, L. Yuliusie, and A. Keatainak (community of Salluit); A. Allianaq, T. R. Avingaq, G. Illupaalik, J. Kukkik, A. Kutiq, and T. A. Taqqaugaq (communities of Igloolik and Hall Beach); Leo Mucktar, Cara Killiktee, Trevor Arreak (community of Mittimatalik); Mittimatalik, Igloolik, Hall Beach, and Amaruq Hunters and Trappers Organization (HTO); Municipality of Coral Harbour and the Aiviit HTO; Municipality of Naujaat and the Arviq HTO; the Qaqqalik Landholding Corporation; Churchill Northern Studies Centre; as well as the DFO and university field team members: P. Bruning, G. Martineau, C. Binet, S. Bourgeois, C. Grant, F. Hartog, R. Felix, K. Jansen, Z. Martin, F. McCaan, S. Paterson, C. Pokiak, P. Robichaud, D. Stewart, J. Stewart, B. Townsend, M. Wetton, S. Wiley, G. Williams, R. Young, and the staff and crew of the William Kennedy, and Eric Brossier and the Vagabond crew. Erin Keenan from WWF canada provided the polynya maps. Katrin Iken provided feedback and edits to the manuscript. Brian Burke assisted with logistics. We recognize the Traditional Inhabitants of both ceded and unceded territory on which this research was conducted.
Supplementary Material
The Supplementary Material for this article can be found online at: https://www.frontiersin.org/articles/10.3389/fmars.2022.754074/full#supplementary-material
References
Adey, W. H., and Hayek, L.-A. C. (2011). Elucidating marine biogeography with macrophytes: quantitative analysis of the North Atlantic supports the thermogeographic model and demonstrates a distinct subarctic Region in the Northwestern Atlantic. Northeast. Nat. 18, 1–128. doi: 10.1656/045.018.m801
Anderson, M., Clark, M. R., and Gorley, R. (2008). PERMANOVA+ for Primer: Guide to Software and Statistical Methods. Plymouth: PRIMER-E.
Archambault, P., Snelgrove, P. V. R., Fisher, J. A. D., Gagnon, J. M., Garbary, D. J., Harvey, M., et al. (2010). From sea to sea: Canada’s three oceans of biodiversity. PLoS One 5:e12182. doi: 10.1371/journal.pone.0012182
Assis, J., Araújo, M. B., and Serrão, E. A. (2018a). Projected climate changes threaten ancient refugia of kelp forests in the North Atlantic. Glob. Chang. Biol. 24, e55–e66. doi: 10.1111/gcb.13818
Assis, J., Tyberghein, L., Bosch, S., Verbruggen, H., Serrão, E. A., and De Clerck, O. (2018b). Bio-ORACLE v2.0: extending marine data layers for bioclimatic modelling. Glob. Ecol. Biogeogr. 27, 277–284. doi: 10.1111/geb.12693
Assis, J., Fragkopoulou, E., Frade, D., Neiva, J., Oliveira, A., Abecasis, D., et al. (2020). A fine-tuned global distribution dataset of marine forests. Sci. Data 7:119. doi: 10.1038/s41597-020-0459-x
Assis, J., Lucas, A. V., Bárbara, I., and Serrão, E. Á (2016). Future climate change is predicted to shift long-term persistence zones in the cold-temperate kelp Laminaria hyperborea. Mar. Environ. Res. 113, 174–182. doi: 10.1016/j.marenvres.2015.11.005
Aumack, C. F., Dunton, K. H., Burd, A. B., Funk, D. W., and Maffione, R. A. (2007). Linking light attenuation and suspended sediment loading to benthic productivity within an arctic kelp-bed community. J. Phycol. 43, 853–863. doi: 10.1111/j.1529-8817.2007.00383.x
Bartsch, I., Paar, M., Fredriksen, S., Hop, H., Asmus, R., Asmus, H., et al. (2017). Arctic Warming Affects Kelp Forest with Associated Fauna in Kongsfjorden, Svalbard. Available online at: http://epic.awi.de/43693/ [Accessed February 5, 2018]
Bartsch, I., Paar, M., Fredriksen, S., Schwanitz, M., Daniel, C., Hop, H., et al. (2016). Changes in kelp forest biomass and depth distribution in Kongsfjorden, Svalbard, between 1996–1998 and 2012–2014 reflect Arctic warming. Polar Biol. 39, 2021–2036. doi: 10.1007/s00300-015-1870-1
Bell, T., and Brown, T. (2018). From Science to Policy in the Eastern Canadian Arctic: An Integrated Regional Impact Study (IRIS) of Climate Change and Modernization. Quebec, QC: ArcticNet.
Bonsell, C., and Dunton, K. H. (2018). Long-term patterns of benthic irradiance and kelp production in the central Beaufort Sea reveal implications of warming for Arctic inner shelves. Prog. Oceanogr. 162, 160–170. doi: 10.1016/j.pocean.2018.02.016
Bringloe, T. T., Zaparenkov, D., Starko, S., Vieira, C., Kawai, H., Hanyuda, T., et al. (2021). Whole genome sequencing reveals forgotten lineages and recurrent hybridizations within the kelp genus Alaria (Phaeophyceae) whole genome sequencing reveals forgotten lineages and (Phaeophyceae). J. Phycol. 57:1721–1738. doi: 10.1111/jpy.13212
Bringloe, T., Verbruggen, H., and Saunders, G. (2020). Population structure in Arctic marine forests is shaped by diverse recolonisation pathways and far northern glacial refugia. bioRxiv [Preprint] doi: 10.1101/2020.03.19.999466
Brown, T., Bell, T., and Forbes, D. (2018). “Regional geography of the Eastern Canadian Arctic,” in An Integrated Regional Impact Study (IRIS) of Climate Change and Modernization: From Science to Policy in the Eastern Canadian ARCTIC, eds T. Bell and T. Brown (Quebec, QC: ArcticNet), 27–51.
Bryndum-Buchholz, A., Prentice, F., Tittensor, D. P., Blanchard, J. L., Cheung, W. W. L., Christensen, V., et al. (2020). Differing marine animal biomass shifts under 21st century climate change between Canada’s three oceans. FACETS 5, 105–122. doi: 10.1139/facets-2019-0035
Campana, G. L., Zacher, K., Fricke, A., Molis, M., Wulff, A., Liliana Quartino, M., et al. (2009). Drivers of colonization and succession in polar benthic macro- and microalgal communities. Bot. Mar. 52, 655–667. doi: 10.1515/BOT.2009.076
Cavalieri, D. J., Parkinson, C. L., Gloersen, P., and Zwally, H. J. (2020). Sea Ice Concentrations from Nimbus-7 SMMR and DMSP SSM/ISSMIS Passive Microwave Data. Boulder: National Snow and Ice Data Center. doi: 10.5067/8GQ8LZQVL0VL
Chapman, A. R. O., and Lindley, J. E. (1980). Seasonal growth of Laminaria solidungula in the Canadian High Arctic in relation to irradiance and dissolved nutrient concentrations. Mar. Biol. 57, 1–5. doi: 10.1007/BF00420961
Christie, H., Gundersen, H., Rinde, E., Filbee-Dexter, K., Norderhaug, K. M., Pedersen, T., et al. (2019). Can multitrophic interactions and ocean warming influence large-scale kelp recovery? Ecol. Evol. 9, 2847–2862. doi: 10.1002/ece3.4963
Cross, W. E., Wilce, R. T., and Fabijan, M. F. (1987). Effects of experimental releases of oil and dispersed oil on Arctic nearshore macrobenthos. III. Macroalgae. Arctic 40, 211–219.
Dankworth, M., Heinrich, S., Fredriksen, S., and Bartsch, I. (2020). DNA barcoding and mucilage ducts in the stipe reveal the presence of Hedophyllum nigripes (Laminariales. Phaeophyceae) in Kongsfjorden (Spitsbergen). J. Phycol. 56, 1245–1254. doi: 10.1111/JPY.13012
Dubois, A., and Iken, K. (2012). Seasonal variation in kelp phlorotannins in relation to grazer abundance and environmental variables in the Alaskan sublittoral zone. ALGAE 27, 9–19. doi: 10.4490/ALGAE.2012.27.1.009
Duggins, D. O., Simenstad, C. A., and Estes, J. A. (1989). Magnification of secondary production by kelp detritus in coastal marine ecosystems. Science 245, 170–173. doi: 10.1126/SCIENCE.245.4914.170
Filbee-Dexter, K. (2020). Ocean forests hold unique solutions to our current environmental crisis. One Earth 2, 398–401. doi: 10.1016/j.oneear.2020.05.004
Filbee-Dexter, K., and Scheibling, R. E. (2012). Hurricane-mediated defoliation of kelp beds and pulsed delivery of kelp detritus to offshore sedimentary habitats. Mar. Ecol. Prog. Ser. 455, 51–64. doi: 10.3354/meps09667
Filbee-Dexter, K., and Scheibling, R. E. (2014). Sea urchin barrens as alternative stable states of collapsed kelp ecosystems. Mar. Ecol. Prog. Ser. 495, 1–25. doi: 10.3354/meps10573
Filbee-Dexter, K., and Wernberg, T. (2018). Rise of Turfs: a new battlefront for globally declining kelp forests. Bioscience 68, 64–76. doi: 10.1093/biosci/bix147
Filbee-Dexter, K., and Wernberg, T. (2020). Substantial blue carbon in overlooked Australian kelp forests. Sci. Rep. 101:12341. doi: 10.1038/s41598-020-69258-7
Filbee-Dexter, K., Feehan, C., Smale D., Krumhansl, K., Augustine, S., de Bettignies, F., et al. (2021). Ocean temperature controls kelp decomposition and carbon sink potential. Res. Square [Preprint]. doi: 10.21203/rs.3.rs-38503/v1
Filbee-Dexter, K., Wernberg, T., Fredriksen, S., Norderhaug, K. M., and Pedersen, M. F. (2019). Arctic kelp forests: diversity, resilience and future. Glob. Planet. Change 172, 1–14. doi: 10.1016/j.gloplacha.2018.09.005
Filbee-Dexter, K., Wernberg, T., Grace, S. P., Thormar, J., Fredriksen, S., Narvaez, C. N., et al. (2020b). Marine heatwaves and the collapse of marginal North Atlantic kelp forests. Sci. Rep. 10:13388. doi: 10.1038/s41598-020-70273-x
Filbee-Dexter, K., Pedersen, M. F., Fredriksen, S., Norderhaug, K. M., Rinde, E., Kristiansen, T., et al. (2020a). Carbon export is facilitated by sea urchins transforming kelp detritus. Oecologia 192, 213–225. doi: 10.1007/s00442-019-04571-1
Franco, J., Wernberg, T., Bertocci, I., Duarte, P., Jacinto, D., Vasco-Rodrigues, N., et al. (2015). Herbivory drives kelp recruits into ‘hiding’ in a warm ocean climate. Mar. Ecol. Prog. Ser. 536, 1–9. doi: 10.3354/meps11445
Frey, K. E., Comiso, J. C., Cooper, L. W., Grebmeier, J. M., and Stock, L. V. (2020). Arctic Ocean Primary Productivity: The Response of Marine Algae to Climate Warming and Sea Ice Decline. Available online at: https://arctic.noaa.gov/Report-Card/Report-Card-2020/ArtMID/7975/ArticleID/900/Arctic-Ocean-Primary-Productivity-The-Response-of-Marine-Algae-to-Climate-Warming-and-Sea-Ice-Decline [Accessed November 2, 2021].
Frigstad, H., Gundersen, H., Andersen, G. S., Borgersen, G., Kvile, K. O., Krause-Jensen, D., et al. (2021). Blue Carbon – Climate Adaptation, CO2 Uptake and Sequestration of Carbon in Nordic Blue Forests. Copenhagen: Nordic Council of Ministers. doi: 10.6027/temanord2020-541
Fritz, M., Vonk, J. E., and Lantuit, H. (2017). Collapsing Arctic coastlines. Nat. Clim. Chang. 7, 6–7. doi: 10.1038/nclimate3188
Gagnon, P., Himmelman, J. H., and Johnson, L. E. (2003). Algal colonization in urchin barrens: defense by association during recruitment of the brown alga Agarum cribrosum. J. Exp. Mar. Bio. Ecol. 290, 179–196. doi: 10.1016/S0022-0981(03)00077-7
Gattuso, J.-P., Gentili, B., Duarte, C. M., Kleypas, J. A., Middelburg, J. J., and Antoine, D. (2006). Light availability in the coastal ocean: impact on the distribution of benthic photosynthetic organisms and contribution to primary production. Biogeosciences 3, 489–513. doi: 10.5194/bg-3-489-2006
Goldsmit, J., Schlegel, R., Filbee-Dexter, K., MacGregor, K. A., Johnson, L. E., Mundy, C. J., et al. (2021). Kelp in the Eastern Canadian Arctic: current and future predictions of habitat suitability and cover. Front. Mar. Sci. 18:1453. doi: 10.3389/FMARS.2021.742209/BIBTEX
Hannah, C., Dupont, F., and Dunphy, M. (2009). Polynyas and tidal currents in the Canadian Arctic Archipelago. Arctic 62, 83–95.
Hawkins, S. J., and Harkin, E. (1985). Preliminary canopy removal experiments in algal dominated communities low on the shore and in the shallow subtidal on the Isle of Man. Bot. Mar. 28, 223–230. doi: 10.1515/botm.1985.28.6.223
Henley, W. J., and Dunton, K. H. (1997). Effects of nitrogen supply and continuous darkness on growth and photosynthesis of the arctic kelp Laminaria solidungula. Limnol. Oceanogr. 42, 209–216. doi: 10.4319/lo.1997.42.2.0209
IPCC (2019). “Summary for policymakers,” in IPCC Special Report on the Ocean and Cryosphere in a Changing Climate, eds H.-O. Pörtner, D. C. Roberts, V. Masson-Delmotte, P. Zhai, M. Tignor, E. Poloczanska, et al. (Geneva: IPCC).
Jayathilake, D. R. M., and Costello, M. J. (2020). A modelled global distribution of the kelp biome. Biol. Conserv. 252:108815. doi: 10.1016/J.BIOCON.2020.108815
Konar, B., and Estes, J. A. (2003). The stability of boundary regions between kelp forests and deforested areas. Ecology 84, 174–185. doi: 10.1890/0012-9658(2003)084[0174:tsobrb]2.0.co;2
Krause-Jensen, D., and Duarte, C. M. (2014). Expansion of vegetated coastal ecosystems in the future Arctic. Front. Mar. Sci. 1:77. doi: 10.3389/fmars.2014.00077
Krause-Jensen, D., and Duarte, C. M. (2016). Substantial role of macroalgae in marine carbon sequestration. Nat. Geosci. 9, 737–742. doi: 10.1038/ngeo2790
Krause-Jensen, D., Archambault, P., Assis, J., Bartsch, I., Bischof, K., Filbee-Dexter, K., et al. (2020). Imprint of climate change on pan-Arctic marine vegetation. Front. Mar. Sci. 7:617324. doi: 10.3389/fmars.2020.617324
Krause-Jensen, D., Lavery, P., Serrano, O., Marbà, N., Masque, P., and Duarte, C. M. (2018). Sequestration of macroalgal carbon: the elephant in the Blue Carbon room. Biol. Lett. 14:20180236. doi: 10.1098/rsbl.2018.0236
Krause-Jensen, D., Marbà, N., Olesen, B., Sejr, M. K., Christensen, P. B., Rodrigues, J., et al. (2012). Seasonal sea ice cover as principal driver of spatial and temporal variation in depth extension and annual production of kelp in Greenland. Glob. Chang. Biol. 18, 2981–2994. doi: 10.1111/j.1365-2486.2012.02765.x
Krumhansl, K., and Scheibling, R. (2012). Production and fate of kelp detritus. Mar. Ecol. Prog. Ser. 467, 281–302. doi: 10.3354/meps09940
Lantuit, H., Overduin, P. P., Couture, N., Wetterich, S., Aré, F., Atkinson, D., et al. (2012). The Arctic coastal dynamics database: a new classification scheme and statistics on arctic permafrost coastlines. Estuaries Coast. 35, 383–400. doi: 10.1007/s12237-010-9362-6
Lee, R. K. S. (1980). A Catalogue of the Marine Algae of the Canadian Arctic. Ottawa: National Museums of Canada.
Ling, S. D., Johnson, C. R., Ridgeway, K., Hobday, A. J., and Haddon, M. (2009). Climate-driven range extension of a sea urchin: inferring future trends by analysis of recent population dynamics. Glob. Chang. Biol. 15, 719–731. doi: 10.1111/j.1365-2486.2008.01734.x
Matthes, L. C., Ehn, J. K., Dalman, L. A., Babb, D. G., Peeken, I., Harasyn, M., et al. (2021). Environmental drivers of spring primary production in Hudson Bay. Elementa 9:00160. doi: 10.1525/ELEMENTA.2020.00160/117238
McClelland, J. W., Holmes, R. M., Dunton, K. H., and Macdonald, R. W. (2012). The Arctic ocean estuary. Estuaries Coast. 35, 353–368. doi: 10.1007/s12237-010-9357-3
Melling, H., Haas, C., and Brossier, E. (2015). Invisible polynyas: modulation of fast ice thickness by ocean heat flux on the Canadian polar shelf. J. Geophys. Res. Ocean. 120, 777–795. doi: 10.1002/2014JC010404
Meredith, M., Sommerkorn, M., Cassotta, S., Derksen, C., Ekaykin, A., Hollowed, A., et al. (2019). “Chapter 3 – Polar regions,” in IPCC Special Report on the Ocean and Cryosphere in a Changing Climate, eds H.-O. Pörtner, D. C. Roberts, V. Masson-Delmotte, P. Zhai, M. Tignor, E. Poloczanska, et al. (Geneva: IPCC).
Merzouk, A., and Johnson, L. E. (2011). Kelp distribution in the northwest Atlantic Ocean under a changing climate. J. Exp. Mar. Bio. Ecol. 400, 90–98. doi: 10.1016/j.jembe.2011.02.020
Michel, C., Hamilton, J., Hansen, E., Barber, D., Reigstad, M., Iacozza, J., et al. (2015). Arctic Ocean outflow shelves in the changing Arctic: a review and perspectives. Prog. Oceanogr. 139, 66–88. doi: 10.1016/j.pocean.2015.08.007
Michel, C., Ingram, R. G., and Harris, L. R. (2006). Variability in oceanographic and ecological processes in the Canadian Arctic Archipelago. Prog. Oceanogr. 71, 379–401. doi: 10.1016/j.pocean.2006.09.006
Moles, A. T., Bonser, S. P., Poore, A. G. B., Wallis, I. R., and Foley, W. J. (2011). Assessing the evidence for latitudinal gradients in plant defence and herbivory. Funct. Ecol. 25, 380–388. doi: 10.1111/j.1365-2435.2010.01814.x
Myers-Smith, I. H., Kerby, J. T., Phoenix, G. K., Bjerke, J. W., Epstein, H. E., Assmann, J. J., et al. (2020). Complexity revealed in the greening of the Arctic. Nat. Clim. Chang. 10, 106–117. doi: 10.1038/s41558-019-0688-1
Norderhaug, K. M., and Christie, H. C. (2009). Sea urchin grazing and kelp re-vegetation in the NE Atlantic. Mar. Biol. Res. 5, 515–528. doi: 10.1080/17451000902932985
Norderhaug, K. M., Nedreaas, K., Huserbråten, M., and Moland, E. (2020b). Depletion of coastal predatory fish sub-stocks coincided with the largest sea urchin grazing event observed in the NE Atlantic. Ambio 501, 163–173. doi: 10.1007/S13280-020-01362-4
Norderhaug, K. M., Filbee-Dexter, K., Freitas, C., Birkely, S.-R., Christensen, L., Mellerud, I., et al. (2020a). Ecosystem-level effects of large-scale disturbance in kelp forests. Mar. Ecol. Prog. Ser. 656, 163–180. doi: 10.3354/MEPS13426
O’Brien, J. M., and Scheibling, R. E. (2016). Nipped in the bud: mesograzer feeding preference contributes to kelp decline. Ecology 97, 1873–1886. doi: 10.1890/15-1728.1
Ortega, A., Geraldi, N. R., Alam, I., Kamau, A. A., Acinas, S. G., Logares, R., et al. (2019). Important contribution of macroalgae to oceanic carbon sequestration. Nat. Geosci. 12, 748–754. doi: 10.1038/s41561-019-0421-8
Pecuchet, L., Blanchet, M. A., Frainer, A., Husson, B., Jørgensen, L. L., Kortsch, S., et al. (2020). Novel feeding interactions amplify the impact of species redistribution on an Arctic food web. Glob. Chang. Biol. 26, 4894–4906. doi: 10.1111/gcb.15196
Pedersen, M. F., Filbee-Dexter, K., Fagerli, C. W., Fredriksen, S., Norderhaug, K., and Wernberg, T. (2020). Detrital carbon production and export in high latitude kelp forests. Oecologia 1–33. doi: 10.1007/s00442-019-04573-z
Pessarrodona, A., Filbee-Dexter, K., Alcoverro, T., Boada, J., Feehan, C. J., Fredriksen, S., et al. (2021a). Homogenization and miniaturization of habitat structure in temperate marine forests. Glob. Chang. Biol. 27, 5262–5275. doi: 10.1111/GCB.15759
Pessarrodona, A., Filbee-Dexter, K., Krumhansl, K. A., Moore, P. J., and Wernberg, T. (2021b). A global dataset of seaweed net primary productivity. bioRxiv [Preprint] doi: 10.1101/2021.07.12.452112
Pessarrodona, A., Moore, P. J., Sayer, M. D. J., and Smale, D. A. (2018). Carbon assimilation and transfer through kelp forests in the NE Atlantic is diminished under a warmer ocean climate. Glob. Chang. Biol. 24, 4386–4398. doi: 10.1111/gcb.14303
Poore, A. G. B., Campbell, A. H., Coleman, R. A., Edgar, G. J., Jormalainen, V., Reynolds, P. L., et al. (2012). Global patterns in the impact of marine herbivores on benthic primary producers. Ecol. Lett. 15, 912–922. doi: 10.1111/j.1461-0248.2012.01804.x
R Core Team (2021). R: A Language and Environment for Statistical Computing. Vienna: R Foundation for Statistical Computing. Available online at: https://www.R-project.org/
Renaud, P. E., Wêsławski, J. M., and Conlan, K. (2021). “Ecology of Arctic shallow subtidal and intertidal benthos,” in Arctic Ecology, ed. D.N. Thomas (Hoboken, NJ: Wiley), 289–324. doi: 10.1002/9781118846582.ch11
Rogers-Bennett, L., and Catton, C. A. (2019). Marine heat wave and multiple stressors tip bull kelp forest to sea urchin barrens. Sci. Rep. 9, 1–9. doi: 10.1038/s41598-019-51114-y
Rood, S. B., Kaluthota, S., Philipsen, L. J., Rood, N. J., and Zanewich, K. P. (2017). Increasing discharge from the Mackenzie River system to the Arctic Ocean. Hydrol. Process. 31, 150–160. doi: 10.1002/hyp.10986
Scheibling, R. E., Feehan, C. J., and Hatcher, B. G. (2020). Strongylocentrotus droebachiensis. Dev. Aquac. Fish. Sci. 43, 553–591. doi: 10.1016/B978-0-12-819570-3.00031-7
Scherrer, K. J. N., Kortsch, S., Varpe, Ø, Weyhenmeyer, G. A., Gulliksen, B., and Primicerio, R. (2019). Mechanistic model identifies increasing light availability due to sea ice reductions as cause for increasing macroalgae cover in the Arctic. Limnol. Oceanogr 64, 330–341. doi: 10.1002/lno.11043
Schindelin, J., Arganda-Carreras, I., Frise, E., Kaynig, V., Longair, M., Pietzsch, T., et al. (2012). Fiji: an open-source platform for biological-image analysis. Nat. Methods 9, 676–682. doi: 10.1038/nmeth.2019
Sharp, G., Allard, M., Lewis, A., Semple, R., and Rochefort, G. (2008). The potential for seaweed resource development in subarctic Canada; Nunavik, Ungava Bay. J. Appl. Phycol. 20, 491–498. doi: 10.1007/s10811-008-9323-7
Simonson, E., Scheibling, R., and Metaxas, A. (2015). Kelp in hot water: I. Warming seawater temperature induces weakening and loss of kelp tissue. Mar. Ecol. Prog. Ser. 537, 89–104. doi: 10.3354/meps11438
Smale, D. A. (2020). Impacts of ocean warming on kelp forest ecosystems. New Phytol. 225, 1447–1454. doi: 10.1111/nph.16107
Spalding, M. D., Fox, H. E., Allen, G. R., Davidson, N., Ferdaña, Z. A., Finlayson, M., et al. (2007). Marine ecoregions of the world: a bioregionalization of coastal and shelf areas. Bioscience 57, 573–583. doi: 10.1641/B570707
Starko, S., Wilkinson, D. P., and Bringloe, T. T. (2021). Recent global model underestimates the true extent of Arctic kelp habitat. Biol. Conserv. 257:109082. doi: 10.1016/J.BIOCON.2021.109082
Steneck, R. S., Bellwood, D. R., and Hay, M. E. (2017). Herbivory in the marine realm. Curr. Biol. 27, R484–R489. doi: 10.1016/j.cub.2017.04.021
Steneck, R. S., Graham, M. H., Bourque, B. J., Corbett, D., Erlandson, J. M., Estes, J. A., et al. (2002). Kelp forest ecosystems: biodiversity, stability, resilience and future. Environ. Conserv. 29, 436–459. doi: 10.1017/S0376892902000322
Teagle, H. A., Hawkins, S. J., Moore, P., and Smale, D. A. (2017). The role of kelp species as biogenic habitat formers in coastal marine ecosystems. J. Exp. Mar. Bio. Ecol. 492, 81–98. doi: 10.1016/J.JEMBE.2017.01.017
Traiger, S. B., and Konar, B. (2018). Mature and developing kelp bed community composition in a glacial estuary. J. Exp. Mar. Bio. Ecol. 501, 26–35. doi: 10.1016/J.JEMBE.2017.12.016
Vadas, R. (1968). The Ecology of Agarum and the Kelp Bed Community. Ph.D. dissertation. Settle: University of Washington.
Van Wychen, W., Copland, L., and Burgess, D. (2020). “Ice masses of the Eastern Canadian Arctic archipelago,” in Landscapes and Landforms of Eastern Canada. World Geomorphological Landscapes, eds O. Slaymaker and N. Catto (Cham: Springer), 297–314. doi: 10.1007/978-3-030-35137-3_13
Vanderklift, M. A., and Wernberg, T. (2008). Detached kelps from distant sources are a food subsidy for sea urchins. Oecologia 157, 327–335. doi: 10.1007/s00442-008-1061-7
Vilas, D., Coll, M., Pedersen, T., Corrales, X., Filbee-Dexter, K., Pedersen, M. F., et al. (2020). Kelp-carbon uptake by Arctic deep-sea food webs plays a noticeable role in maintaining ecosystem structural and functional traits. J. Mar. Syst 203:103268. doi: 10.1016/j.jmarsys.2019.103268
Wernberg, T., and Filbee-Dexter, K. (2019). Missing the marine forest for the trees. Mar. Ecol. Prog. Ser. 612, 209–215. doi: 10.3354/meps12867
Wernberg, T., Bennett, S., Babcock, R. C., de Bettignies, T., Cure, K., Depczynski, M., et al. (2016). Climate-driven regime shift of a temperate marine ecosystem. Science 353, 169–172. doi: 10.1126/science.aad8745
Wernberg, T., Krumhansl, K. A., Filbee-Dexter, K., and Pedersen, M. F. (2019). “Status and trends for the world’s kelp forests,” in World Seas: An Environmental Evaluation: Ecological Issues and Environmental Impacts, ed. C. Sheppard (Cambridge, MA: Academic Press), 57–78. doi: 10.1016/b978-0-12-805052-1.00003-6
Wernberg, T., Smale, D. A., and Thomsen, M. S. (2012). A decade of climate change experiments on marine organisms: procedures, patterns and problems. Glob. Chang. Biol. 18, 1491–1498. doi: 10.1111/j.1365-2486.2012.02656.x
Wiencke, C., and Amsler, C. D. (2012). Seaweeds and Their Communities in Polar Regions. Berlin: Springer, doi: 10.1007/978-3-642-28451-9_13
Wiencke, C., and Clayton, M. N. (2011). “Introduction: biology of polar benthic algae,” in Biology of Polar Benthic Algae, ed. C. Wiencke (Berlin: De Gruyter), 337.
Wiencke, C., Clayton, M. N., Gómez, I., Iken, K., Lüder, U. H., Amsler, C. D., et al. (2007). Life strategy, ecophysiology and ecology of seaweeds in polar waters. Life Extrem. Environ. 6, 213–244. doi: 10.1007/978-1-4020-6285-8-13
Wilce, R. T. (2016). The “Arctic Stamp”, its imprint on an endangered marine flora. Perspect. Phycol. 3, 155–180. doi: 10.1127/pip/2016/0046
Witman, J. D., and Lamb, R. W. (2018). Persistent differences between coastal and offshore kelp forest communities in a warming Gulf of Maine. PLoS One 13:e0189388. doi: 10.1371/journal.pone.0189388
Wood, S. N. (2017). Generalized Additive Models: An Introduction with R. 2nd Edn. London: Chapman and Hall. doi: 10.1201/9781315370279/GENERALIZED-ADDITIVE-MODELS-SIMON-WOOD
Wulff, A., Iken, K., Quartino, M. L., Al-Handal, A., Wiencke, C., and Clayton, M. N. (2011). “Biodiversity, biogeography and zonation of marine benthic micro- and macroalgae in the Arctic and Antarctic,” in Biology of Polar Benthic Algae, ed. C. Wiencke (New York, NY: De Gruyter), 337. doi: 10.1515/BOT.2009.072
Keywords: macroalgae, polar, borealization, marine forests, seaweed, sea ice
Citation: Filbee-Dexter K, MacGregor KA, Lavoie C, Garrido I, Goldsmit J, Castro de la Guardia L, Howland KL, Johnson LE, Konar B, McKindsey CW, Mundy CJ, Schlegel RW and Archambault P (2022) Sea Ice and Substratum Shape Extensive Kelp Forests in the Canadian Arctic. Front. Mar. Sci. 9:754074. doi: 10.3389/fmars.2022.754074
Received: 05 August 2021; Accepted: 10 January 2022;
Published: 31 March 2022.
Edited by:
Mariana Mayer Pinto, University of New South Wales, AustraliaReviewed by:
Hanzhi Lin, Science Systems and Applications, Inc., United StatesAlejandra Mora-Soto, University of Victoria, Canada
Christina Bonsell, Bureau of Ocean Energy Management, United States
Copyright © 2022 Filbee-Dexter, MacGregor, Lavoie, Garrido, Goldsmit, Castro de la Guardia, Howland, Johnson, Konar, McKindsey, Mundy, Schlegel and Archambault. This is an open-access article distributed under the terms of the Creative Commons Attribution License (CC BY). The use, distribution or reproduction in other forums is permitted, provided the original author(s) and the copyright owner(s) are credited and that the original publication in this journal is cited, in accordance with accepted academic practice. No use, distribution or reproduction is permitted which does not comply with these terms.
*Correspondence: Karen Filbee-Dexter, a2ZpbGJlZWRleHRlckBnbWFpbC5jb20=