- 1Mote Marine Laboratory, Sarasota, FL, United States
- 2The University of Texas at Arlington, Arlington, TX, United States
Insecticide use is common in developed tropical regions where it may enter coastal reef ecosystems through land-based sources. This local introduction of contaminants could affect ecosystem health as corals can better withstand global stressors more readily if local pressures are reduced. The present study investigated the microbial community and photochemical efficiency of the reef building coral, Montastraea cavernosa, when exposed to the commercially applied insecticide, permethrin. Montastraea cavernosa was exposed to an acute concentration of permethrin for 24 h in a controlled laboratory setting. Fourteen fragments were integrated across four treatments (2 or 0.325 μg/l of permethrin, acetone control, and saltwater control) with three to four replicates per treatment. Photosynthetic efficiency was measured by quantifying the maximum photochemical yield and maximum electron transport rate (ETR), which were recorded for each fragment before exposure and 24 h after exposure to permethrin. The microbial communities of M. cavernosa tissue was measured using 16S rRNA sequencing for each fragment. Permethrin exposure at the tested concentrations suggested no significant effect on the M. cavernosa’s photochemical parameters measured during the 24-h permethrin exposure. Microbial communities were significantly different between permethrin treated (2 and 0.325 μg/l) and non-treated conditions (acetone and saltwater controls). In permethrin treated coral, this study documented a significant increase in Burkholderia pyroccinnia and Bacillus sp., bacteria groups known to bioremediate insecticides. Exposure of permethrin also decreased the relative abundance of Mesorhizobium sp., Sediminibacterium sp., Sphingorhabdus sp., and Chondromyces sp., which are known to inhibit pathogen colonization and provide essential macromolecules. Therefore, although the symbiotic relationship between the host and the intracellular algae remained intact after the corals were exposed to permethrin, the significant shift in the microbiome indicate permethrin may destabilize the microbial composition of the holobiont.
Introduction
Corals are frequently threatened by both global and local stressors that are causing significant mortality worldwide (Bruno et al., 2007; Eakin et al., 2010; Kennedy et al., 2013). Considerable research has focused on global issues such as high water temperatures from climate change, ocean acidification, and disease (Hoegh-Guldberg et al., 2007; Hughes et al., 2016). However, it is imperative to investigate other existing anthropogenic and natural influences affecting reefs on the local scale, especially because those threats could be alleviated through local management action. Previous research suggest that corals may be able to withstand some global pressures more readily if local threats are reduced (Carilli et al., 2009; Kennedy et al., 2013; Donovan et al., 2021). One potential local threat that has had little attention includes the applications of pesticides within nearshore environments. Permethrin [(3-phenoxyphenyl)methyl(+)cis,trans-3-(2,2-dichloroethenyl)-2,2-dimethylcyclopropane-carboxylate] is a mix of synthetically produced cis and trans isomer pyrethroids, which functions to paralyze the nervous system of insects (Pierce, 1998). Often, permethrin is sprayed from a mounted sprayer attached to the back of a moving vehicle, typically applied in the summer rainy season (May–October) to target when adult mosquitos hatch after a rainfall (Monroe County Mosquito Control) (Ross et al., 2015). Permethrin has been detected in surface water canal systems throughout the Florida Keys (5.1–9.4 μg/l) and within the Florida Keys National Marine Sanctuary ranging from 0.5 to 50.1 μg/m2 due to aerial drift and runoff (Pierce, 2005). Permethrin is highly toxic to aquatic invertebrates, with reported 96-h LC-50 to be 0.02 μg/l for mysid shrimp, 0.2 μg/l for penaeid shrimp, and 2.2 μg/l for silverside minnows (Clark et al., 1989; Tietze et al., 1995). Permethrin typically has a half-life of 14 days in water and sediment (Lee et al., 2004).
Very few investigations have studied the effects of insecticides on corals. However, one study showed that the mosquito pesticide naled, which is typically sprayed intermittently with permethrin, has a negative effect to larval settlement in Porites astreoides by reducing survivorship (Ross et al., 2015). Permethrin, however, did not affect larval settlement success under ambient conditions nor was there a negative response when corals were exposed to permethrin in concert with increased water temperatures. Low concentrations of permethrin (0.3–1.0 μg/l), however, showed reduced settlement and metamorphosis on the larvae of Acropora millepora (Markey et al., 2007), suggesting species and life-stage specific responses. Acropora cervicornis corals also showed specific transcription of stress-associated genes after being exposed to low doses of permethrin (Morgan et al., 2001). To date, studies have focused on the effects of insecticides on the coral host and its associated algal symbiont although a suite of microbes exist to create the coral holobiont (Morgan et al., 2001; Ritchie, 2006). The effects of the widely commercialized insecticides on the microbiome of corals are generally unknown.
A healthy coral microbiome can exhibit antibiotic properties to defend the coral from invasive bacteria (Ritchie, 2006). However, as the health of reef ecosystems have shifted from fully functioning to disturbed, microbial shifts within corals often occur with these environmental stressors that result in the introduction of opportunistic bacteria (Sunagawa et al., 2009). Known stressors that elicit a bacterial shift include high water temperatures, excessive nutrients, coastal development, and overfishing, which all influence coral health (Bruno et al., 2003, 2007; Brandt and Mcmanus, 2009) as well as others. The present study aimed to further investigate the effects of anthropogenic effects on corals by characterizing the microbial community within corals as well as a suite of photochemical efficiency parameters after exposure to two different concentrations of permethrin within a controlled setting. We hypothesized that exposure to permethrin would reduce photochemical efficiency within the coral holobiont, which would suggest a disruption in the coral-algal symbiosis. Additionally, we hypothesized that permethrin exposure would result in a dysbiosis of the microbial community through identifiable shifts in bacterial members. Quantifying the response of the holobiont physiology and the microbiome community characterized the effects of this short-term contaminant exposure on a major reef building coral of Florida’s Coral Reef.
Materials and Methods
Coral Collection
Montastraea cavernosa, the great star coral, was originally collected from the National Oceanic and Atmospheric Administration (NOAA) Rescue Nursery located off of Key West, Florida using permit FKNMS-2015-082 and transferred to Mote Marine Laboratory’s research facility in Sarasota, Florida. Ten parent colonies of M. cavernosa were cut into fourteen 1.5″ × 1.5″ fragments and allowed to recover for 14 days within a flow-through raceway prior to random fragment assignment in the subsequent exposure experiment. The raceway was equipped with a scheduled 12-h activated T5 light (Wave Point). The temperature-regulated raceway provided consistent water parameters by circulating water with additional water jets. To conduct the exposure experiment, 14 glass beakers (1 L) were distributed within the water bath of the raceway. A randomly selected single fragment of coral was placed within each beaker and allowed to further acclimate to the controlled system for 7 days. Treatments were then assigned to beakers as controls or permethrin treatments (Supplementary Figure 1). Each glass beaker was assigned a water hose for flow through water exchange during acclimation and an air stone to provide additional aeration and to eventually aid in the mixing of permethrin. Water quality parameters, including temperature, pH, dissolved oxygen, and salinity were recorded daily. Temperatures were maintained at 27°C (±0.006), pH averaged 7.94 [±0.001; National Bureau of Standards (NBS)], salinity averaged 36.35 ppt (±0.005), and dissolved oxygen averaged 5.98 mg/L (±0.003).
Permethrin Exposure
Permethrin (Chem Service Inc., West Chester, PA, United States, 99.25% purity) concentrations for exposure to M. cavernosa were 2 (high) and 0.325 ug/L (low), plus acetone and seawater controls, for a total of four treatments. Acetone (5 μl/L) was utilized to increase the solubility of the permethrin treatments. The target doses of permethrin were selected due to the limited solubility of permethrin in acetone, which precipitates in excess of 10 μg/L (Ross et al., 2015). Low permethrin and acetone treatments had four replicate aquaria each and high and saltwater controls both had three replicate aquaria. The high permethrin treatment and saltwater control had one less replicate due to sample processing error at the end of the exposure and subsequent contamination, which warranted their removal from the analysis.
The corals were dosed with treatment conditions on October 12, 2016, a week after the fragments were placed into the 1-L beakers for acclimation. Water samples (500 ml) were collected for analysis of permethrin concentration 30 mins and 24-h after dosing occurred. Permethrin was recovered from water samples by liquid-liquid extraction into dichloromethane (DCM). The DCM was replaced with MeOH by rotary evaporation for DCM solvent recovery. The MeOH containing permethrin extract was reduced to 1 ml volume for HPLC-MS/MS analysis of both the cis- and trans-permethrin isomers.
Permethrin analysis was conducted using a Thermo Electron Quantum Access HPLC system, interfaced with a Quantum Access triple quadrupole MS/MS. A Phenomenex Kinetex 2.6 μm particle size, 100 mm × 2.1 mm analytical column was used with mobile phase gradient of 10 mM Ammonium Formate in Methanol, 10 mM Ammonium Formate in HPLC water, initial ratio 20:80 for 5 min, then to 99:1 for 8 min, back to 20:80 for 2 min, total sample run time 15 min at a flow rate of 200 μl/min. Analytical standards, Trans- and Cis-Permethrin solutions were purchased from Chem Service.
Pulse Amplitude Modulation Fluorometer
To determine the state of the coral-algal symbiosis the photochemical efficiency on each colony was measured using a Walz Imaging Pulse Amplitude Modulator (PAM) Fluorometer. The imaging PAM measures the spatial distribution of chlorophyll fluorescence of photosystem II. Montastraea cavernosa colonies were dark acclimated (30 min) prior to conducting light curves. Light curves provided information on photochemical yield (Fv/Fm) and electron transport rate (ETR). The photochemical yield (Fv/Fm) is a normalizing ratio created by dividing the variable fluorescence (Fv) by the maximum fluorescence (Fm). By dark acclimating the colony, this relaxes the photosystem II reaction centers. When the colony was exposed to a pulse of light, the calculated yield represented the maximum potential quantum efficiency of photosystem II if all capable reaction centers were open. The maximum ETR represents the photosynthetic capacity of an organism after which photoinhibition occurs where the organism cannot process additional photosynthetic active radiation (PAR) that is being emitted by the PAM. The ETR represents photosynthetic capacity because it is informative of the number of electrons passing through photosystem II where four electrons pass through photosystem II for every O2 produced during photosynthesis (Ritchie and Bunthawin, 2010). The PAM measurements were taken prior to permethrin exposure and 24 h after exposure. After passing the assumptions for normality using the Shapiro-Wilk test and homoscedasticity, tested using the Levene’s test, a paired t-test, and the multiple comparison’s false discovery rate (FDR) test compared replicates within treatments before exposure and 24 h after exposure to permethrin in R v1.2.5033 (R Core Team, 2019).
Microbial Community
After 24 h of exposure, tissue samples were collected from each of the M. cavernosa fragments for DNA isolation by scraping off a single polyp using a sterile scalpel. DNA extraction was performed on the coral tissue using a Powersoil DNA isolation kit (Qiagen, Germantown, MD, United States). The extracted DNA was sent to MR DNA for sequencing of the 16S rRNA gene V1–V3 region using PCR primers 27 F and 519 R. Polymerase chain reaction protocol required a 28 cycle PCR using HotStarTaq Plus Master Mix Kit (Qiagen, United States) under the following conditions: 94°C for 3 min, followed by 28 cycles of 94°C for 30 s, 53°C for 40 s, and 72°C for 1 min, after which a final elongation step at 72°C for 5 min. Samples were purified using Ampure XP beads and then sequenced at MR DNA1 (Shallowater, TX, United States) on a Miseq following the manufacturer’s standard guidelines. Sequence data was processed using MR DNA analysis pipeline. In summary, sequences were stitched together to assemble contigs, and sequences <150 bp or with ambiguous nucleotide identification were removed. Operational taxonomic units (OTUs) were categorized by clustering at 97% sequence similarity (Supplementary Table 1). Using the 16S barcoding region, 3,311 of the final OTUs were taxonomically classified using the nucleotide basic local alignment search tool (BLASTn) against a database derived from the Ribosomal Database Project (RDPII)2 and the National Center for Biotechnology Information (NCBI)3 and organized into each taxonomic level.
A permutational analysis of variance (PERMANOVA) tested for differences in the bacterial community between water samples and coral tissue samples. A PERMANOVA was also used to test for differences in the bacterial community within the coral fragments among treatments. Dominant bacterial classes were identified as those with greater than 3% average relative abundance in any treatment condition. Classes were compared among treatments using a Kruskal Wallis test. A similarity percentage (SIMPER) analysis was used to determine the dissimilarity between treatments and provided the percent dissimilarity caused by each bacterial OTUs. The top six OTUs that contributed to dissimilarity were tested for differences among treatments using the Kruskal Wallis test and post-hoc analysis was performed using a Dunnett’s test. Bacterial OTUs for each sample were processed through a non-metric multidimensional scaling (NMDS) plot, which provided a visual representation of the bacterial community in multidimensional space in a two-dimension visual plot. The relative abundance of bacterial classes and genera of coral tissue and water samples were also graphed for visualization.
Simpson diversity, Pielou’s richness, and beta diversity of the bacterial community was calculated at the bacterial species level for each sample (Supplementary Figure 2 and Supplementary Table 2). Using an analysis of variance (ANOVA) test, the differences in diversity and richness among treatments for each sample was applied after passing assumptions of normality using the Shapiro-Wilk test and homoscedasticity using the Levene Test.
Results
Permethrin Adsorption
The high treatment dose of permethrin (n = 3) ranged between 1.60 and 2.20 μg/L with an average of 2.0 μg/l (±0.122 μg/l SE) (Supplementary Figure 3). The low permethrin treatments (n = 4) received a dosing concentration between 0.20 and 0.40 μg/L with an average of 0.325 μg/l (±0.046 μg/l SE). No permethrin was found in the saltwater control (n = 3) or acetone controls (n = 4). Measurements after 24 h showed an absence of permethrin for all but one sample within low concentration treatment, which generated an average of 0.0125 μg/l (±0.0125 μg/l SE). The loss of permethrin from exposure solutions was most likely a result of the hydrophobic nature of permethrin causing it to adsorb to surfaces when dispersed in seawater or potentially the biological accumulation of permethrin within the coral tissue itself. However, identifying where the permethrin adsorbed to during the depuration process was outside of the scope of the present project.
Photochemical Parameters of the Algal Symbiont
Within each treatment, there were no significant differences between the initial and post photochemical yields (p > 0.1 for all comparisons; Supplementary Figure 4). The photochemical yield decreased for corals exposed to permethrin in high and low treatments, but this was not significant (t = 1.0182, df = 6, p-value = 0.3479, FDR padj = 0.80; Supplementary Figure 4). The ETR had a significant increase in post permethrin exposure relative to before permethrin exposure in only coral exposed to permethrin, however the post-hoc FDR test showed no significant differences (t = −3.2609, df = 6, p-value = 0.01723, FDR padj = 0.075; Supplementary Figure 5).
Microbial Community
The 16S rDNA sequencing resulted in an average of 47,993 (±5,633 SE) reads per coral tissue sample and the water samples had an average of 108,899 (±7,453 SE) reads. There were a total number of 1,350 distinct OTUs within the coral tissue samples, whereas there was a total of 2,771 distinct OTUs within the water samples. A permutational ANOVA showed a significant difference between the bacterial community within the water compared to the bacterial community of the M. cavernosa tissue, suggesting the tissue samples indeed represent the community associated with the living coral tissue (F = 9.5354, df = 1, p-value < 0.001; Supplementary Table 3 and Supplementary Figures 6, 7). There was also a significant difference in the bacterial community of M. cavernosa among treatments (F = 5.272, df = 3, p-value = 0.0028; Figure 1). However, pairwise PERMANOVAs detected no significance between treatment pairs (Supplementary Table 4) due to the conservative approach of the test.
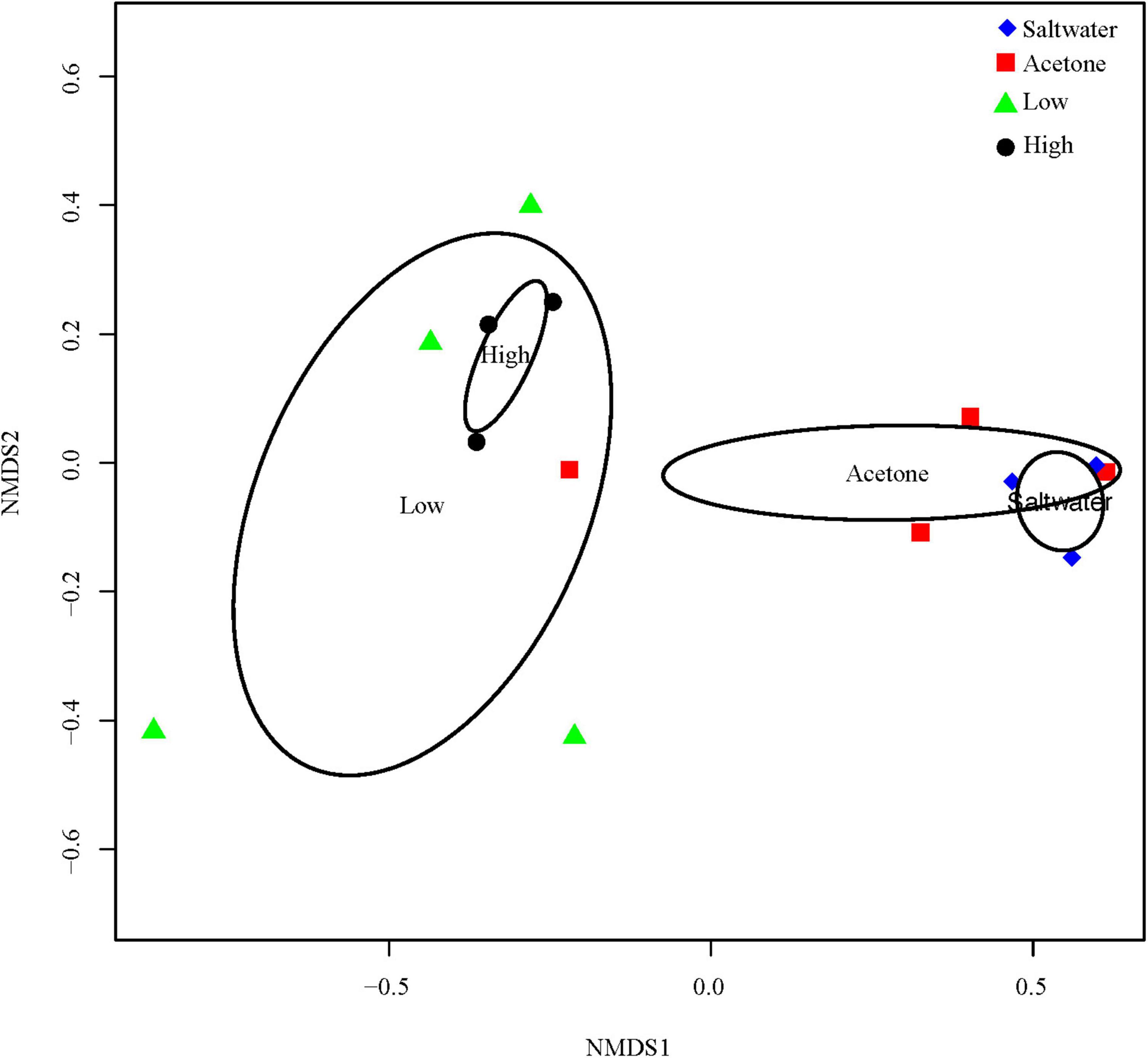
Figure 1. Nonmetric dimensional scaling (NMDS) of microbiome reads from M. cavernosa tissue samples. “High” indicates samples that were dosed with a concentration of 1.93 mg/l of permethrin 24 h prior to sampling. “Low” indicates samples that were dosed with a concentration of 0.33 mg/l of permethrin. “Acetone” represents the coral that was dosed with 5 ml/l of acetone to match the acetone concentration used to dilute the permethrin in permethrin-treated aquaria. “Seawater” controls were dosed with seawater from the controlled flow-through system to account for a control treatment.
The coral tissue bacterial community of the seawater control treatment was dominated by two classes: Alphaproteobacteria (41.7%) and Sphingobacteria (33.9%). The acetone control tissue samples were dominated by three bacterial classes, Alphaproteobacteria (24%), Betaproteobacteria (34.5%), and Sphingobacteria (22.5%). In seawater controls, Alphaproteobacteria (41.7%) was primarily composed of Sphingopyxis sp. (16%), Mesorhizobium sp. (11.4%), and Bradyrhizobium sp. (6.8%) (Figure 2C). Alphaproteobacteria (24%) abundance was lower in acetone control corals but was still primarily composed of Sphingopyxis sp. (3.6%), Mesorhizobium sp. (8.6%), and Bradyrhizobium sp. (5%) (Figures 2B,C). Betaproteobacteria was dominated by Burkholderia pyrrocinia (Figure 2D), and Sphingobacteria was dominated by Sediminibacterium sp. (Figure 2E) within the acetone and saltwater control colonies.
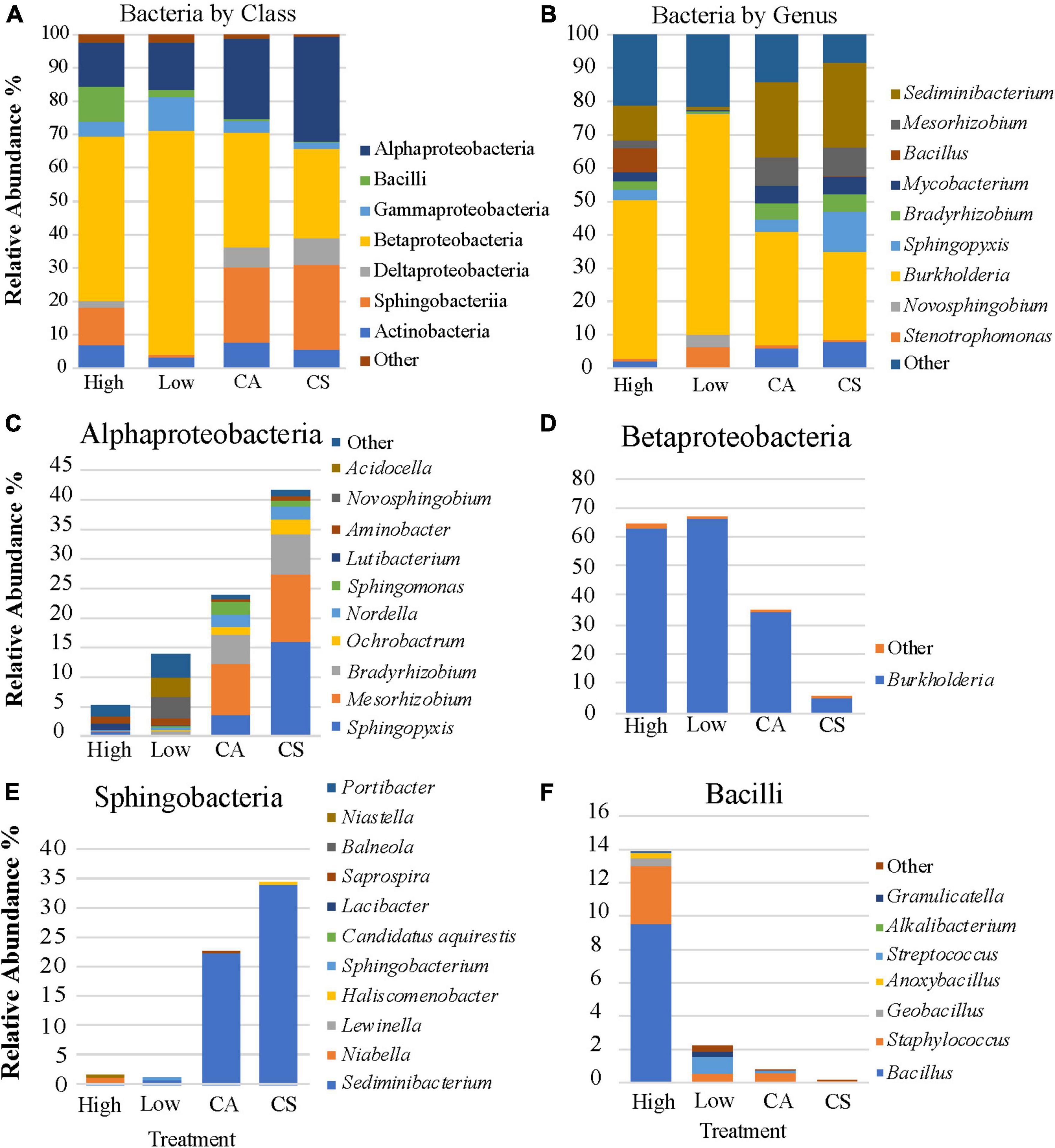
Figure 2. The average relative abundance of the bacterial community members by class (A), genus (B), and the genera that comprise the four dominant classes (C–F).
In low permethrin treatment coral, Betaproteobacteria contributed to 67% of the coral’s microbiome, nearly exclusively composed of B. pyrrocinia (66.4%) (Figure 2D). Betaproteobacteria was higher in abundance in low-dosed coral compared with the other treatments and the bacterial community of this treatment also showed significantly lower (p = 0.006) abundances of the bacterial classes that were dominant in the control coral tissue. Alphaproteobacteria (14%) and Sphingobacteria (0.9%) were lower in the low dose treated corals compared with controls (Figure 2A). Alphaproteobacteria was dominated by Sphingopyxis sp., Mesorhizobium sp., and Bradyrhizobium sp. in the controls, but these genera were nearly absent in the low-dosed tissue. Rather, this class was composed primarily of Novosphingobium sp. (3.6%) and Acidocella sp. (3.3%) (Figure 2C). The relative abundance of Sphingobacteria (0.9%) remained dominated by Sediminibacterium sp. (0.9%) in low-dosed coral (Figure 2E).
In the high-dosed coral bacterial community, Betaproteobacteria (64.8%), dominated by B. pyrrocinia (62.7%), comprised most of the microbiome. Alphaproteobacteria (5.6%) abundance in the high-dosed treatment was less than in the controls and low-dosed coral. Sphingopyxis sp. and Mesorhizobium sp. were significantly reduced (df = 3, p-value < 0.05; df = 3, p-value < 0.05; Figure 3) in permethrin treated microbiomes and the Alphaproteobacteria was represented by different bacterial genera and primarily composed of Acidocella sp. and Novosphingobium sp. (Figure 2C). Sphingobacteria was relatively less abundant in high-dosed tissue (1.2%) than in the controls (24%) and was similar in abundance compared with the low-dosed tissue (0.9%) (Figure 2E). Bacilli (13.8%), dominated by Bacillus sp., increased in abundance in high-dosed tissue (Figure 2F). In comparison, Bacilli was observed at decreased abundance in low-dosed (2.3%), acetone control (0.8%), and seawater controls (0.14%).
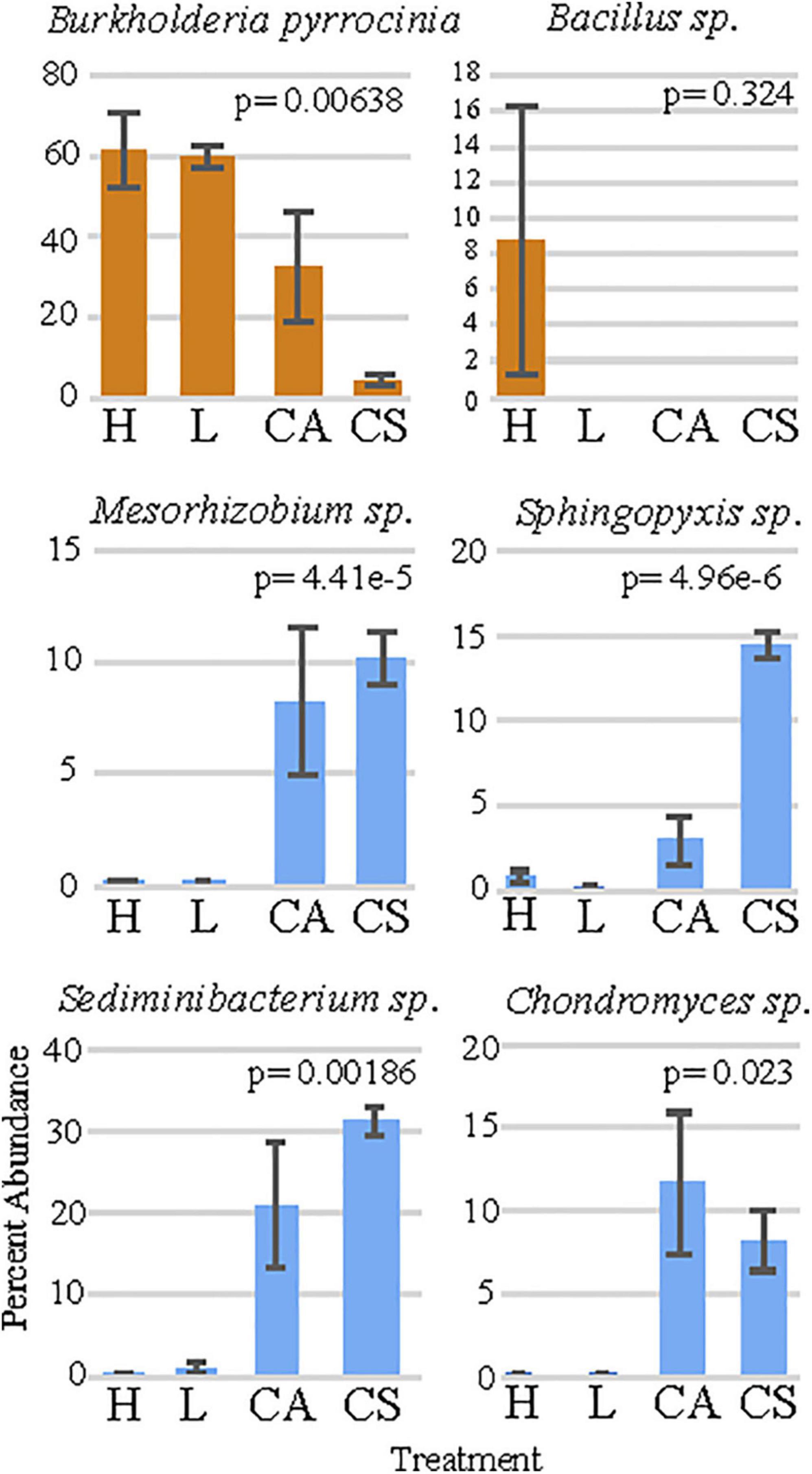
Figure 3. The percent abundance of the six bacteria that are responsible for the largest dissimilarity between treatments. “H” = high permethrin concentration treatment, “L” = low permethrin concentration treatment, “CA” = acetone control, and “CS” = seawater control. Error bars represent standard error.
Dissimilarity Among Treatments
The SIMPER analysis showed that the six OTU’s were responsible for the greatest dissimilarity among treatments included: (1) B. pyrrocinia (homology identified at 99%; Figure 3), (2) Sediminibacterium sp. (homology identified at 96%; Figure 3), (3) Sphingopyxis sp. (homology identified at 99%; Figure 3), (4) Chondromyces sp. (homology identified at 97%; Figure 3), (5) Bacillus sp. (homology identified as 99%; Figure 3), and (6) Mesorhizobium sp. (homology identified as 98%; Figure 3). Comparisons of these genera among treatments using non-parametric Kruskal-Wallis tests presented significant differences in the relative abundance (Supplementary Table 5) for B. pyrrocinia (x2 = 8.3476, df = 3, p = 0.039; Figure 3), Sediminibacterium sp. (x2 = 8.7238, df = 3, p = 0.033; Figure 3), Mesorhizobium sp. (x2 = 10.799, df = 3, p = 0.012; Figure 3), Sphyngopyxis sp. (x2 = 9.8762, df = 3, p = 0.02; Figure 3), and Chondromyces sp. (x2 = 10.933, df = 3, p = 0.012; Figure 3). The multiple comparison post-hoc Dunnett’s test showed that B. pyrrocinia was significantly higher in the high permethrin treatment compared to the saltwater control (t = 3.957, p = 0.007). This conservative post-hoc test did not detect any difference in Bacillus sp. among treatments. Sediminibacterium sp., Mesorhizobium sp., Sphingopyxis sp., and Chondromyces sp. were significantly more abundant in seawater control corals compared to low permethrin exposure conditions (p < 0.037 for all comparisons). Sediminibacterium sp., Mesorhizbium sp., and Sphingopyxis sp., were also significantly more abundant in seawater control corals compared with the high permethrin exposure conditions (p < 0.005 for all comparisons).
Microbial Diversity and Richness
Statistical analyses showed no significant differences in the alpha diversity of the bacterial community among treatments (Supplementary Figures 2A–C and Supplementary Table 2). Interestingly, both the Simpson’s diversity and the Pielou’s Evenness metrics showed a trending linear decrease among treatments from controls to the high treatment exposure (Supplementary Figure 2C). Beta diversity of the microbial community was not significantly different among treatments (Supplementary Figure 2B) with no apparent trends visible.
Discussion
Stable Photochemical Efficiency
Previous investigations on the effects of pesticide exposure on coral have documented a decline in settlement, survivorship, stunted metamorphosis, and transcriptional stress response in the larvae of Acropora sp. (Morgan et al., 2001; Markey et al., 2007; Ross et al., 2015). However, Ross et al. (2015) showed no significant effects of permethrin exposure on Porites astreoides larvae settlement and survival. The present study measured the photochemical and microbial community response in adult M. cavernosa to an acute permethrin exposure. The maintenance of similar maximum ETR and maximum photochemical yield suggests the algal-coral symbiosis of adult M. cavernosa exposed to an acute permethrin dose over 24 h was not negatively affected. The lack of an effect on the coral-algal symbiosis suggests that the dose may have been too low to elicit a response, the exposure may have been too short, or the corals tested may be resistant to permethrin exposure. Further studies that incorporate repeated or long-term exposure, increased levels of dosing, and additional species are needed to quantify the level of resistance corals may have to this commonly used insecticide.
Microbiome Shifts May Mediate Insecticide Resistance
Burkholderia pyrrocinia, a nitrogen fixing bacteria, was the most abundant bacteria within the bacterial community of the fragments exposed to permethrin, consisting of approximately 60% of the bacterial community. On the other hand, the presence of Burkholderia contributed to less than 10% of the bacterial community within the control corals. Burkholderia has been identified as an insecticide resistant bacterium found in the soybean bug Riptortus pedestris, which allowed the bean bug to survive fenitrothion spraying (Kikuchi et al., 2012). Burkholderia hydrolyzes fenitrothion, an organophosphorous insecticide, into 3-methyl 4-nitrophenol and metabolizes the degradation product as a carbon source for Burkholderia’s growth. Burkholderia has also been identified with insecticide degradation properties toward bifenthrin, a pyrethroid that includes permethrin (Lee et al., 2004). The increased relative abundance of this particular bacteria within corals exposed to permethrin suggests it may function in a similar way within corals. However, further study is needed to target the function of the bacterial group that becomes dominant within the microbiome of M. cavernosa exposed to acute levels of permethrin.
Bacillus sp., which was also abundant within permethrin exposed corals, has the ability to hydrolyze pyrethroids, particularly even permethrin into non-insecticidal compounds (Maloney et al., 1992, 1993; Chen et al., 2014). Bacillus shifted to an 8.76% abundance in the high dosage of permethrin treatment compared to the near 0% of Bacillus in other treatments. These results may suggest bioremediation, or a breakdown of permethrin is occurring by the microbiome to mitigate the insecticide presence. However, Bacillus is a large genus with hundreds of species with different functional roles. Further exploring how the increase of this bacterial genera may affect the coral holobiont could be an important research topic for follow-up studies. Alternatively, the exposure could have simply resulted in a selection for insecticide resistant bacteria, thus changing the community to have more representation of bacteria that can survive and even break down the compounds within insecticides.
Permethrin Reduced Potentially Stabilizing Bacteria
The exposure of corals to permethrin caused a significant shift in the bacterial community, perhaps reducing beneficial bacterial found within healthy corals. For example, Sediminibacterium sp. was highest in abundance in control corals (up to 32%) and completely lost in permethrin exposed coral. This bacteria has been identified in healthy coral tissues in previous studies (Lesser and Jarett, 2014). Similarly, Chondromyces sp. was approximately 10% of the bacterial community in acetone and seawater control coral tissues. However, permethrin treated coral had close to 0% relative abundance of Chondromyces sp. This bacteria species has been identified in sponges and produce the bacterial metabolite Apicularen A isolates, which have strong inhibition of angiogenesis, or blood vessel development associated with tumor growth (Kwon, 2002). While Sediminibacterium sp.’s function in coral is unknown in corals, it is a well-recognized plant growth promoting rhizobacteria and reported to be responsible for subduing soil borne pathogens, suggesting at least some species within this genus may have probiotic properties (Han et al., 2019). Additionally, the reduced abundance of Chondromyces sp.’s to nearly absent as a consequence of permethrin exposure implicates a functional loss in opportunity for this bacteria species when corals interact with this contaminant.
The loss of Sphingopyxis sp. bacterium in the permethrin exposed fragments may also have negative effects to the host. Sphingorhabdus litoris (previously Sphingopyxis litoris) is the closest phylogenetically related bacterium within Sphingopyxis sp. that has been observed in coral and isolated from the gorgonian Eunicella labiate. Genome annotation of S. litoris presented genes coding for polyphosphate kinase and exopolyphosphatase, which mitigates the synthesis of energetic polyphoshatases (Silva et al., 2018). This likely provides phosphorous for the host, an important requirement for stony corals, soft corals, and sponges. Further annotation of the S. litoris genome presents genes that are associated with homeostasis with metals, and even resistance to antibiotics. Some strains (EL 138) of S. litoris contain genes with homology to astaxanthin, which has anti-inflammatory properties (Silva et al., 2018). Finally, Sphingopyxis sp. in general can extrude porphyrin-like growth factors that promote the growth of other bacteria that otherwise grew poorly (Bhuiyan et al., 2015). The beneficial properties of this bacteria within other similar marine organisms suggest that the loss of this genus within corals exposed to permethrin could have larger implications on coral health by the insecticide exposure resulting in the loss of beneficial microbes within the holobiont.
Finally, Mesorhizobium sp. showed a similar result as Sediminibacterium sp., Chrondromyces sp., and Sphingopyxis sp. Mesorhizobium composed approximately 8.6% of the relative abundance in controls only to decline to 2.25% in high permethrin treatment and 0.1% in low permethrin treated coral tissues. The nitrogen fixing Mesorhizobium sp. have been identified in previous coral studies (Kaneko et al., 2000; Carlos et al., 2013), but little is known about this bacterial group. However, a bacterial isolate completed Koch’s postulate to induce white plague type II had the highest homology to Mesorhizobium mediterraneum with 92% similarity, and so this isolate was given a new genus and species name to become the more well-known, Aurantimonas coralicida (Denner et al., 2003). While Aurantimonas coralicida and Mesorhizobium sp. possess phylogenic proximity, their homologous functional contributions to the host are unknown. They are still both Rhizobiales bacteria, and likely have a meaningful prokaryotic-eukaryotic relationship suggesting that the reduced abundance of Mesorhizobium sp. functional proclivity to fix nitrogen in permethrin exposed coral may have negative impacts to the health of the coral holobiont.
Conclusion
The present 24-h study, designed to mimic an acute permethrin exposure that a coral may experience under natural circumstances, suggests the algal-coral symbiosis and particularly the photochemical parameters of the algal symbionts within the coral host are not significantly impaired under this scenario. Rather, it appears the microbiome of the host significantly shifts after just a single acute exposure to permethrin. While the observed shift in the microbiome toward the potential for insecticide bioremediating bacteria may be beneficial for the coral to mitigate the immediate permethrin exposure, it is unclear if these bacteria indeed bioremediate and if this microbial shift is optimal for the stability of the microbiome to mitigate other stressors. Shifts in the microbiome may be detrimental to the health of the coral as opportunistic bacteria colonize the host during times of microbial dysbiosis. The increased abundance of Burkholderia and Bacillus along with the reduced abundance in Sediminibacterium sp., Chondromyces, Sphingopyxis sp., and Mesorhizobium sp., in permethrin exposed coral suggest significant shifts in the bacterial community are occurring, which could leave the host more vulnerable to secondary threats such as infectious disease. However, the functional changes in the coral holobiont associated with this microbiome shift are largely unknown. Further investigations would benefit from determining the functional role of these shifting bacteria within the coral holobiont and conducting chronic insecticide exposures to determine if these microbial shifts return to pre-exposure communities or settle into a community dominated by opportunistic bacteria.
Data Availability Statement
The datasets presented in this study can be found in online repositories. The names of the repository/repositories and accession number(s) can be found below: NCBI (accession: PRJNA747979).
Author Contributions
EM and RP were responsible for compiling the intellectual merit behind the research objectives, design, and acquiring funding for this original research. NM, LA, and AC recorded pulse amplitude modulator measurements and extracted DNA. MH prepared permethrin concentrations and recorded actual permethrin results. EM designed the statistical analysis and implemented by NM created all the figures. NM wrote the manuscript with considerable editorial input from EM with additional revisions from all authors. All authors contributed to the experimental permethrin exposure and submitted the approved version.
Funding
This work was supported by the Environmental Protection Agency award number 00D51817 to RP.
Conflict of Interest
The authors declare that the research was conducted in the absence of any commercial or financial relationships that could be construed as a potential conflict of interest.
Publisher’s Note
All claims expressed in this article are solely those of the authors and do not necessarily represent those of their affiliated organizations, or those of the publisher, the editors and the reviewers. Any product that may be evaluated in this article, or claim that may be made by its manufacturer, is not guaranteed or endorsed by the publisher.
Acknowledgments
We thank the members of the ecotoxicology and coral nursery staff at Mote Marine laboratory.
Supplementary Material
The Supplementary Material for this article can be found online at: https://www.frontiersin.org/articles/10.3389/fmars.2022.748308/full#supplementary-material
Footnotes
References
Bhuiyan, T., Mitsuhashi, S., Shigetomi, K., Tanaka, Y., Kamagata, Y., and Ubukata, M. (2015). Zincmethylphyrins and Coproporphyrins, Novel Growth Factors Released by Sphingopyxis Sp., Enable Laboratory Cultivation of Previously Uncultured Leucobacter Sp. Through Interspecies Mutualism. Tokyo: The Journal of Antibiotics.
Brandt, M. E., and Mcmanus, J. W. (2009). Disease incidence is related to bleaching extent in reef-building corals. Ecology 90, 2859–2867. doi: 10.1890/08-0445.1
Bruno, J. F., Selig, E. R., Casey, K. S., Page, C. A., Willis, B. L., Drew Harvell, C., et al. (2007). Thermal stress and coral cover as drivers of coral disease outbreaks. PLoS Biol. 5:e124. doi: 10.1371/journal.pbio.0050124
Bruno, J. F., Stachowicz, J. J., and Bertness, M. D. (2003). Inclusion of facilitation into ecological theory. Trends Ecol. Evol. 18, 119–125. doi: 10.1016/S0169-5347(02)00045-9
Carilli, J. E., Norris, R. D., Black, B. A., Walsh, S. M., and McField, M. (2009). Local stressors reduce coral resilience to bleaching. PLoS One 4:e6324. doi: 10.1371/journal.pone.0006324
Carlos, C., Torres, T. T., and Ottoboni, L. M. M. (2013). Bacterial communities and species-specific associations with the mucus of Brazilian coral species. Sci. Rep. 3:1634. doi: 10.1038/srep01624
Chen, S., Chang, C., Deng, Y., An, S., Dong, Y. H., Zhou, J., et al. (2014). Fenpropathrin biodegradation pathway in Bacillus Sp. DG-02 and its potential for bioremediation of pyrethroid-contaminated soils. J. Agric. Food Chem. 62, 2147–2157. doi: 10.1021/jf404908j
Clark, L. R. Jr., Goodman, P. W., Borthwick, J. M., Patrick, G. M., Cripe, P. M., Moody, J. C., et al. (1989). Toxicity of pyrethroids to marine invertebrates and fish: a literature review and test results with sediment-sorbed chemicals. Environ. Toxicol. Chem. 8, 393–401. doi: 10.1002/etc.5620080505
Denner, E. B. M., Smith, G. W., Busse, H. J., Schumann, P., Narzt, T., Polson, S. W., et al. (2003). Aurantimonas coralicida gen. nov., sp. nov., the causative agent of white plague type II on Caribbean scleractinian corals. Int. J. Syst. Evol. Microbiol. 53, 1115–1122. doi: 10.1099/ijs.0.02359-0
Donovan, M. K., Burkepile, D. E., Kratochwill, C., Shlesinger, T., Sully, S., Oliver, T. A., et al. (2021). Local conditions magnify coral loss following marine heatwaves. Science 980, 977–980. doi: 10.1126/science.abd9464
Eakin, C. M., Morgan, J. A., Heron, S. F., Smith, T. B., Liu, G., Alvarez-Filip, L., et al. (2010). Caribbean corals in crisis: record thermal stress, bleaching, and mortality in 2005. PLoS One 5:e13969. doi: 10.1371/journal.pone.0013969
Han, L., Wang, Z., Li, N., Wang, Y., Feng, J., and Zhang, X. (2019). Bacillus amyloliquefaciens B1408 suppresses Fusarium wilt in cucumber by regulating the rhizosphere microbial community. Appl. Soil Ecol. 136, 55–66. doi: 10.1016/j.apsoil.2018.12.011
Hoegh-Guldberg, O., Mumby, P. J., Hooten, A. J., Steneck, R. S., Greenfield, P., Gomez, E., et al. (2007). Coral reefs under rapid climate change and ocean acidification. Science 318, 1737–1742. doi: 10.1126/science.1152509
Hughes, T. P., Baird, A. H., Bellwood, D. R., Card, M., Connolly, S. R., Folke, C., et al. (2016). Climate change, human impacts, and the resilience of coral reefs. Science 301, 929–933. doi: 10.1126/science.1085046
Kaneko, T., Nakamura, Y., Sato, S., Asamizu, E., Kato, T., Sasamoto, S., et al. (2000). Complete genome structure of the nitrogen-fixing symbiotic bacterium Mesorhizobium loti. DNA Res. 7, 331–338. doi: 10.1093/dnares/7.6.331
Kennedy, E. V., Perry, C. T., Halloran, P. R., Iglesias-Prieto, R., Schönberg, C. H. L., Wisshak, M., et al. (2013). Avoiding coral reef functional collapse requires local and global action. Curr. Biol. 23, 912–918. doi: 10.1016/j.cub.2013.04.020
Kikuchi, Y., Hayatsu, M., Hosokawa, T., Nagayama, A., and Tago, K. (2012). Symbiont-mediated insecticide resistance. Proc. Natl. Acad. Sci. U.S.A. 109, 8618–8622. doi: 10.1073/pnas.1200231109
Kwon, H. J. (2002). Apicularen A, a macrolide from Chondromyces Sp., inhibits growth factor induced in vitro angiogenesis. J. Microbiol. Biotechnol. 12, 702–705.
Lee, S., Gan, J., Kim, J. S., Kabashima, J. N., and Crowley, D. E. (2004). Microbial transformation of pyrethroid insecticides in aqueous and sediment phases. Environ. Toxicol. Chem. 23, 1–6. doi: 10.1897/03-114
Lesser, M. P., and Jarett, J. K. (2014). Culture-dependent and culture-independent analyses reveal no prokaryotic community shifts or recovery of Serratia Marcescens in Acropora palmata with White pox disease. FEMS Microbiol. Ecol. 88, 457–467. doi: 10.1111/1574-6941.12311
Maloney, S. E., Maule, A., and Smith, A. R. (1993). Purification and preliminary characterization of permethrinase from a pyrethroid-transforming strain of Bacillus cereus. Appl. Environ. Microbiol. 59, 2007–2013. doi: 10.1128/aem.59.7.2007-2013.1993
Maloney, S. E., Maule, A., and Smith, A. R. W. (1992). Transformation of synthetic pyrethroid insecticides by a thermophilic Bacillus Sp. Arch. Microbiol. 158, 282–286. doi: 10.1007/BF00245246
Markey, K. L., Baird, A. H., Humphrey, C., and Negri, A. P. (2007). Insecticides and a fungicide affect multiple coral life stages. Mar. Ecol. Prog. Ser. 330, 127–137. doi: 10.3354/meps330127
Morgan, M. B., Vogelien, D. L., and Snell, T. W. (2001). Assessing coral stress responses using molecular biomarkers of gene transcription. Environ. Toxicol. Chem. 20, 537–543. doi: 10.1002/etc.5620200312
Pierce, R. H. (1998). Effects of Mosquito Control Measures on Non-Targeted Organisms in the Florida Keys National Marine Sanctuary Draft Final, No. 609. Sarasota, FL: Mote Marine Laboratory.
Pierce, R. H. (2005). Aerial and tidal transport of mosquito control pesticides into the Florida keys national marine sanctuary. Rev. Biol. Trop. 53, 117–125.
R Core Team (2019). R: A Language and Environment for Statistical Computing. Vienna: R Foundation for Statistical Computing.
Ritchie, K. B. (2006). Regulation of microbial populations by coral surface mucus and mucus-associated bacteria. Mar. Ecol. Prog. Ser. 322, 1–14. doi: 10.3354/meps322001
Ritchie, R. J., and Bunthawin, S. (2010). The use of pulse amplitude modulation (PAM) fluorometry to measure photosynthesis in a CAM orchid, Dendrobium Spp. (D. CV. Viravuth Pink). Int. J. Plant Sci. 171, 575–585. doi: 10.1086/653131
Ross, C., Olsen, K., Henry, M., and Pierce, R. (2015). Mosquito control pesticides and sea surface temperatures have differential effects on the survival and oxidative stress response of coral larvae. Ecotoxicology 24, 540–552. doi: 10.1007/s10646-014-1402-8
Silva, S. G., Lago-lestón, A., Costa, R., and Keller-Costa, T. (2018). Crossm. Tokyo: The Journal of Antibiotics, 1–2.
Sunagawa, S., Desantis, T. Z., Piceno, Y. M., Brodie, E. L., Desalvo, M. K., Voolstra, C. R., et al. (2009). Bacterial diversity and White Plague disease-associated community changes in the Caribbean coral Montastraea faveolata. ISME J. 3, 512–521. doi: 10.1038/ismej.2008.131
Keywords: permethrin, Montastraea cavernosa, PAM, microbiome, coral, dysbiosis, bioremediation, microbial shift
Citation: MacKnight NJ, Arick L, Crawford AC, Henry M, Pierce R and Muller EM (2022) An Acute Permethrin Exposure Causes Significant Microbial Shifts in Montastraea cavernosa. Front. Mar. Sci. 9:748308. doi: 10.3389/fmars.2022.748308
Received: 27 July 2021; Accepted: 28 January 2022;
Published: 04 March 2022.
Edited by:
Ranjeet Bhagooli, University of Mauritius, MauritiusReviewed by:
Josef Velíšek, University of South Bohemia, CzechiaNeviaty Zamani, Bogor Agricultural University, Indonesia
Copyright © 2022 MacKnight, Arick, Crawford, Henry, Pierce and Muller. This is an open-access article distributed under the terms of the Creative Commons Attribution License (CC BY). The use, distribution or reproduction in other forums is permitted, provided the original author(s) and the copyright owner(s) are credited and that the original publication in this journal is cited, in accordance with accepted academic practice. No use, distribution or reproduction is permitted which does not comply with these terms.
*Correspondence: Erinn M. Muller, ZW11bGxlckBtb3RlLm9yZw==