- 1Fisheries College, Jimei University, Xiamen, China
- 2Key Laboratory of Healthy Mariculture for the East China Sea, Ministry of Agriculture, Xiamen, China
- 3Fujian Engineering Research Center of Aquatic Breeding and Healthy Aquaculture, Fujian Development and Reform Commission, Xiamen, China
Macroalgae are important primary producers in coastal waters; they have high carbon sink potential and are often subjected to hyposaline stress in their natural habitats. The effect of hyposaline stress on dissolved organic carbon (DOC) release from macroalgae remains to be studied in depth. In this study, five common intertidal macroalgae in coastal waters of Fujian Province, China—Pyropia haitaneisis, Gracilaria lemaneiformis, Sargassum thunbergii, Enteromorpha prolifera, and Ulva lactuca—were used as research materials to investigate the effects of 6-h hyposaline treatments (5 PSU, 0 PSU) on the growth, DOC release rate, photosynthesis, respiration, and contents of carbon (C), nitrogen (N), and phosphorus (P). Our results showed that, although there were significant interspecific differences in the tolerance of the five species of macroalgae to low salinity, the DOC release rate of macroalgae increased overall with decreasing salinity, while the photosynthetic rate showed the opposite trend. Hyposaline treatments reduced the net photosynthetic rate of macroalgae, as the net photosynthetic rate of all five species decreased by more than 50% and 75% under the 5 PSU and 0 PSU treatments, respectively. The tissue C contents of P. haitaneisis, G. lemaneiformis, and E. prolifera increased significantly with decreasing salinity, by 6.90%, 40.15%, and 43.80% at 0 PSU, respectively. However, the tissue C contents of S. thunbergii and U. lactuca were not influenced or were slightly decreased by low salinity. These results suggest that short-term hyposaline treatment has a dual effect on organic carbon accumulation of macroalgae by inhibiting photosynthetic carbon fixation and increasing DOC release, and this in turn may have a large impact on the carbon cycle in macroalgae enrichment areas.
1. Introduction
Owing to increasing global warming, the topics of greenhouse gas emission reduction and carbon sequestration have attracted worldwide attention. Our planet includes two main categories of primary producers: marine phytoplankton and terrestrial plants, each accounting for about 50% of global net primary productivity (Field et al., 1998; Schlesinger and Bernhardt, 2020). The carbon fixed by marine photoautotrophs (including macroalgae, phytoplankton, and seagrasses) is generally referred to as “Blue Carbon” (Nellemann et al. 2009; Krause-Jensen et al., 2018; Raven, 2018; Gao et al., 2022). During the growth process, macroalgae convert inorganic carbon into organic carbon through photosynthesis, and part of this organic carbon is involved in the formation of algal tissues, while the other part is released into the environment as dissolved organic carbon (DOC) and particulate organic carbon (POC). Part of the POC and DOC are eventually exported to the deep sea to be sequestered; this is the carbon sink formation mechanism of macroalgae (Krause-Jensen and Duarte, 2016; Krause-Jensen et al., 2018). Some economically important macroalgae have already been established in complete industrial chains and therefore have greater potential for application in carbon sequestration and sink enhancement (Raven, 2018; Chen et al., 2020a; Gao et al., 2021; Gao et al., 2022; Tian et al., 2023). However, the current understanding of macroalgal-mediated carbon sink processes is not sufficiently advanced to accurately assess the carbon sink potential of macroalgae (Paine et al., 2021; Hurd et al., 2022).
The mechanism of organic matter release from algae is generally considered to include two types: active release and passive leakage (Thornton, 2014; Paine et al., 2021). Overflow is a typical example of active release as it results in the leaching of DOC from cells when the production capacity of algal photosynthesis exceeds consumption (Hatcher et al., 1977; Fogg, 1983; Livanou et al., 2019). This DOC active release mechanism can avoid the excessive accumulation of photosynthetic products that leads to algal damage and thus can maximize the utility of ribulose-1,5-bisphosphate carboxylase (Rubisco). Since the released organic carbon and the release processes are closely related to photosynthesis and respiration, it is thought that those factors that could influence these two metabolic pathways may alter the release of organic carbon (Thornton, 2014; Paine et al., 2021). In this manner, stressful growth conditions due to changes in CO2 concentration, temperature, light, salinity, and photoperiod can indirectly but strongly increase the release of organic carbon (Paine et al., 2021). However, the effects of environmental stresses on organic carbon release from macroalgae lack in-depth study.
Fujian Province ranks first in China in terms of production of macroalgae, and it has abundant wild macroalgae resources (Chen et al., 2020a; Fishery-Department-of-China, 2021). The tides in Fujian Province are semi-diurnal, and the macroalgae at the high tide zone can experience a maximum of 6 h of desiccation. Moreover, Fujian is one of the provinces of China with the highest average annual rainfall, up to 2,432.6 mm (Fujian-Meteorological-Bureau, 2021). As a result, intertidal macroalgae in Fujian coastal waters are often under pressure from hyposaline stress. In addition, many macroalgae are found in estuaries where the salinity of seawater depends on the mixing ratio of seawater and freshwater and thus is highly variable; for example, the salinity falls in the range of 0.81–50 PSU (Hurd et al., 2014; Ma, 2021). This is another cause of hyposaline stress. It has been shown that hyposaline stress due to rainfall is an important driver of DOC release from the macroalgae Enteromorpha prolifera, Chondrus crispus, and Fucus vesiculosus (Sieburth, 1969; Pregnall, 1983). However, such studies are few, and it is uncertain whether the conclusions are widely representative.
Based on the above analyzation, we hypothesized that hyposaline stress may increase DOC release from macroalgae, resulting in an underestimation of the carbon sink potential of macroalgae. Therefore, this study analyzed the effects of hyposaline stress on the growth, organic matter release rate, and tissue C, N, and P contents of five common intertidal macroalgae in the coastal areas of Fujian Province, China, and preliminarily analyzed the biogeochemical effects of hyposaline stress on the carbon sink process mediated by macroalgae.
2. Experimental materials and methods
The W28 strain of P. haitaneisis was obtained from the Laboratory of Germplasm Improvements and Applications of Pyropia in Jimei University of Fujian Province. Gracilaria lemaneiformis was obtained from the culture raft. The collection time and locations of wild Sargassum thunbergii, Enteromorpha prolifera, and Ulva lactuca are shown in Table 1. The collected algae were quickly transported back to the laboratory, rinsed at least three times with sterilized seawater to remove impurities, and placed in five liter glass bottles for temporary incubation for 3–5 days. The photoperiod was 12 L:12 D, and the light intensity was set at 100 μmol m-2 s-1.
The healthy algae were selected and rinsed again with sterilized seawater three times and placed in 500 mL glass bottles. The algae were treated with three salinities (0 PSU, 5 PSU, and 30 PSU) for 6 h. During the incubation period, the cultures were aerated and irradiated with a light intensity of 100 μmol m-2 s-1. The incubation temperatures were the same as the temperature at the time of algal collection (Table 1). Different salinities of seawater were taken from natural seawater and ultrapure water.
2.1. DOC release rate
A seawater sample of 20 mL was collected from each bottle and filtered using pre-combusted (450°C, 4 h) GF/F filters (with a pore size of 0.70 μm, Whatman) at low vacuum pressure. The filtered water samples were stored at −20°C until analysis. All glassware was combusted at 450°C for 4 h in a muffle furnace followed by immersion in 0.1 mol/L HCl solution for more than 24 h, and finally rinsed with ultrapure water. Dissolved organic carbon (DOC) in the filtered water samples was determined with a total organic carbon analyzer (TOC-VCPH, Shimadzu). The DOC release rate (mg g-1 FW h-1) was calculated as follows:
where DOC0 and DOCt are DOC concentrations (mg L-1) before and after 6 h of incubation, respectively; V is the volume of seawater (L); FW is the fresh weight of algal tissue (g).
2.2. Tissue C, N, and P contents
After 6 h of incubation, the algal samples were quickly rinsed with ultrapure water, and then the surface water was gently removed with gauze. Algal samples were dried at 60°C and ground into powder. The contents of C and N in algal tissues were analyzed using a Costech ECS CHNSO elemental analyzer (Costech Analytical Technologies, USA). P contents were determined by an automatic chemical analyzer (CleverChem380, Germany) according to the method of Solórzano (1980).
2.3. Photosynthesis and respiration
The algal samples were dark-treated in trays for 15 min, and then the maximum quantum yield of photosystem II, fv/fm, was measured using an underwater modulated fluorometer (Walz DivingPAM, Germany) or a chlorophyll fluorometer (Qubit Systems FluorPen, Czech Republic). The equation for fv/fm is as follows:
where f0 and fm are the minimum and maximum fluorescence of a dark-adapted sample, respectively; f0 is the fluorescence value obtained when all reaction centers of photosystem II are in the open state; fm is the fluorescence value obtained when all reaction centers are in the closed state.
The rates of photosynthesis and respiration were measured by the light-dark bottle technique. The algal tissues were transported into dissolved oxygen bottles filled with seawater and then incubated under light or dark for 6 h at different salinity treatments. The bottles without algae were set at blanks. The light intensity for the light bottles was 100 μmol m-2 s-1. After incubation, the oxygen concentration of seawater was measured based on Winkler’s method using an automatic titrator (Mettler-Toledo G20, Greifensee, Switzerland) equipped with a DMi140-SC platinum electrode (Shriwastav et al., 2017). The net photosynthetic rate, respiration rate, and total photosynthetic rate were calculated from the difference in dissolved oxygen concentrations in light, dark, and blank bottles. The calculation formulas were as follows:
where Od, Ol and Ob are dissolved oxygen concentrations in dark, light, and blank bottles (mg·L-1), respectively; V is the culture volume (L); FW is the fresh weight of algal tissues (g); Pn is the net photosynthetic rate (mg·g-1·h-1); Rd is the dark respiration rate (mg·g-1·h-1), and Pt is the total photosynthetic rate (mg·g-1·h-1).
2.4. Data statistics and analysis
The experimental data were processed and statistically analyzed using the software SPSS 26.0 and Excel 2010. One-way ANOVA was used to compare the differences between different data groups, with p< 0.05 indicating significant differences and p< 0.01 indicating highly significant differences.
3. Results
3.1. Photosynthesis and respiration
As shown in Figure 1, a salinity of 5 PSU did not significantly influence the fv/fm of P. haitaneisis or S. thunbergii, while this level significantly decreased the fv/fm of the other three species G. lemaneiformis, E. prolifera, and U. lactuca (Figure 1). In addition, 0 PSU strongly decreased fv/fm values of all five macroalgal species compared with 30 PSU.
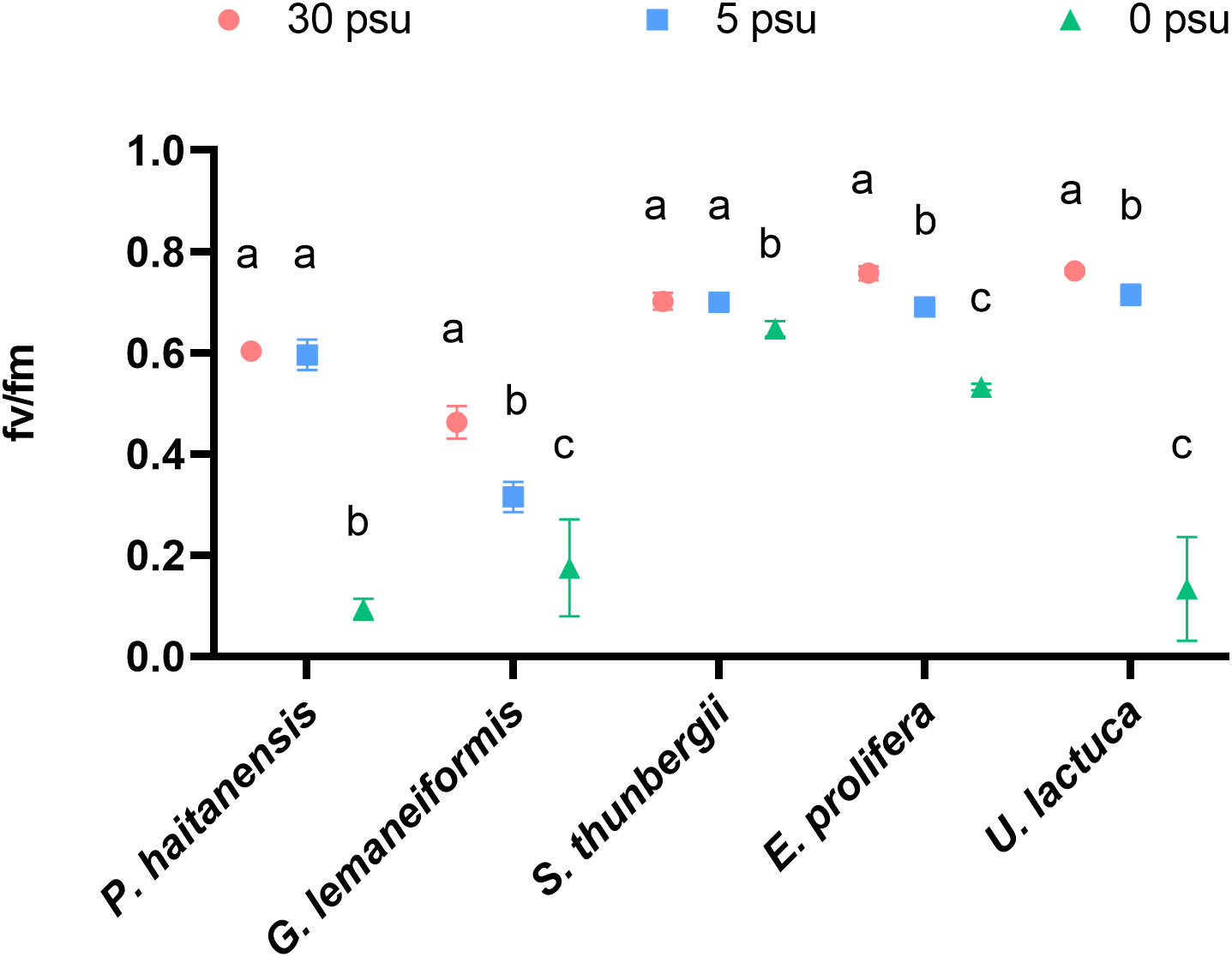
Figure 1 Effects of hyposaline stress on fv/fm of five macroalgal species. Different lowercase letters only indicate significant differences between the three salinity treatments for each macroalgal species. Data are presented as mean ± SD, n = 4. p< 0.05 was considered significant.
The net (Pn) and total (Pt) photosynthetic rates of all five macroalgal species decreased significantly with decreasing salinity (Figures 2A–E, p< 0.05). Compared with 30 PSU, A low salinity of 5 PSU decreased Pn of P. haitaneisis, G. lemaneiformis, S. thunbergii, E. prolifera, and U. lactuca by 77.6%, 84.26%, 81.36%, 56.18%, and 58.97%, respectively. Except for G. lemaneiformis, 0 PSU further decreased Pn of the other four macroalgal species (p< 0.01); in particular, Pn of S. thunbergia decreased to a negative value (p< 0.01).
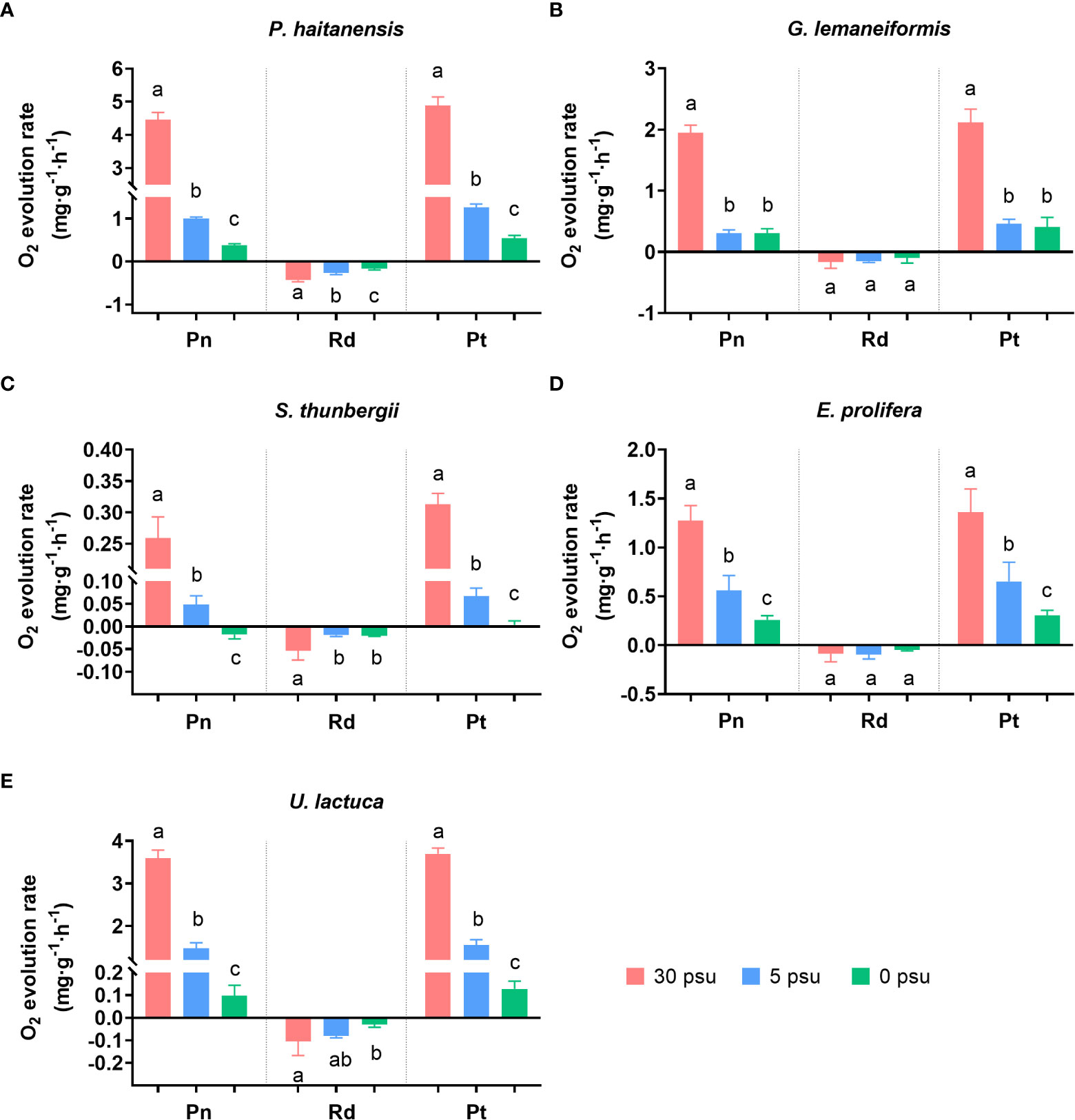
Figure 2 Effects of hyposaline stress on net photosynthetic rate (Pn, three left bars), dark respiration rate (Rd, three middle bars) and total photosynthetic rate (Pt, three right bars) of five macroalgal species P. haitaneisis (A), G. lemaneiformis (B), S. thunbergii (C), E. prolifera (D), and U. lactuca (E). Different lowercase letters only indicate significant differences between the three salinity treatments for each parameter. Data are presented as mean ± SD, n = 4. p< 0.05 was considered significant.
The dark respiration rates (Rd) of G. lemaneiformis and E. prolifera did not change significantly with the decrease in salinity (p > 0.05), while Rd of the other three species decreased with decreasing salinity (p< 0.05). Compared to 30 PSU, 0 PSU decreased the Rd of P. haitaneisis, S. thunbergii, and U. lactuca by 61.35%, 42.18%, and 71.55%, respectively (p< 0.05). The Rd of U. lactuca was unchanged by the 5 PSU treatment (p > 0.05). A further decrease in salinity from 5 PSU to 0 PSU significantly decreased the Rd of P. haitaneisis (p< 0.01) but did not decrease the Rd of S. thunbergii or U. lactuca (p > 0.05).
The response trends of total photosynthetic rates (Pt) of all five macroalgal species were almost the same as net photosynthetic rates (Pn). A low salinity of 5 PSU decreased Pt of P. haitaneisis, G. lemaneiformis, S. thunbergii, E. prolifera, and U. lactuca by 74.19%, 78.29%, 78.47%, 52.01%, and 57.97% (p< 0.01), respectively. Except for G. lemaneiformis, a further decrease to 0 PSU further reduced Pt of the other four macroalgal species (p< 0.05).
3.2. Release of dissolved organic carbon
As shown in Figure 3, the DOC release rates of five macroalgal species increased with decreasing salinity. Compared with the 30 PSU treatment, 5 PSU did not significantly increase the DOC release rates of P. haitaneisis or E. prolifera (p > 0.05), while those of the other three seaweeds were significantly enhanced (p< 0.05). Compared with the 5 and 30 PSU treatments, 0 PSU significantly increased the DOC release rate of all five macroalgal species. At 0 PSU, the DOC release rate of G. lemaneiformis was the highest, more than 3.5 times that of the other four macroalgal species.
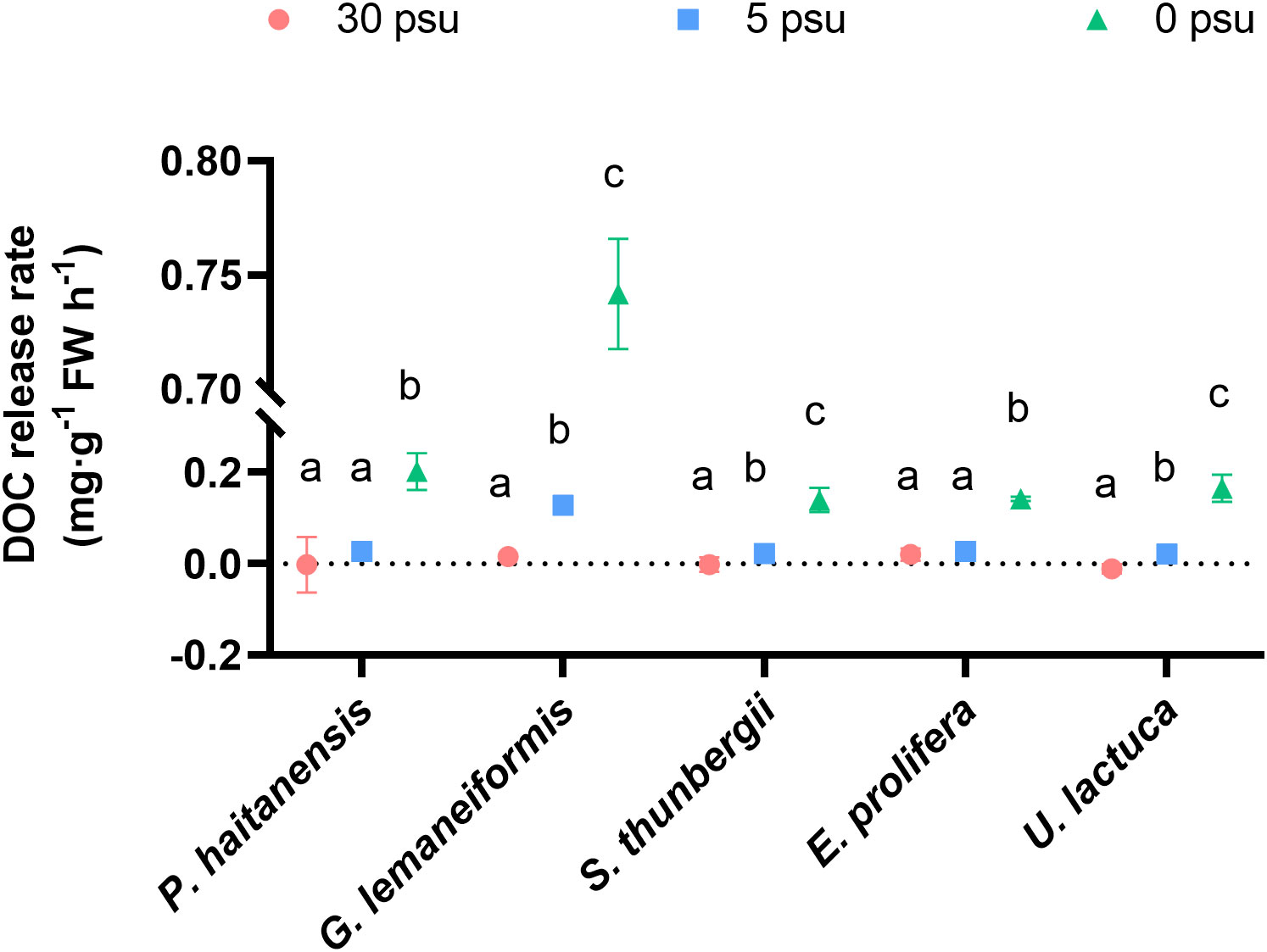
Figure 3 Effects of hyposaline stress on the DOC release rate of five macroalgal species. Different lowercase letters only indicate significant differences between the three salinity treatments for each macroalgal species. Data are presented as mean ± SD, n = 4. p< 0.05 was considered significant.
3.3. Tissue C, N, and P contents and ratios
With decreasing salinity, the tissue C contents of P. haitaneisis, G. lemaneiformis, and E. prolifera were elevated significantly (p< 0.01), while the C contents of S. thunbergii did not change significantly (p > 0.05) and that of U. lactuca decreased significantly (Figure 4A, p< 0.01). Compared with 30 PSU, the 0 PSU treatment resulted in 6.90%, 40.15%, and 43.79% increases in the C contents of P. haitaneisis, G. lemaneiformis, and E. prolifera, respectively. The C contents of U. lactuca decreased (p< 0.01) by 2.54% and 2.45% under 5 PSU and 0 PSU, respectively, compared to the 30 PSU treatment, but there was no significant difference between two low salinity treatments (p > 0.05). The N and P contents of P. haitaneisis did not differ significantly at different salinities (p > 0.05), and the N and P contents of G. lemaneiformis showed an increase followed by a decrease with decreasing salinity (p< 0.01). The N contents of S. thunbergii showed a significant increase (p< 0.01) only at 0 PSU, while the N contents of E. prolifera showed a significant decrease (p< 0.05) only at 0 PSU. In addition, the P contents of S. thunbergii and E. prolifera did not change significantly (p > 0.05), and the N and P contents of U. lactuca increased and decreased, respectively, with decreasing salinity (Figures 4B, C, p< 0.01).
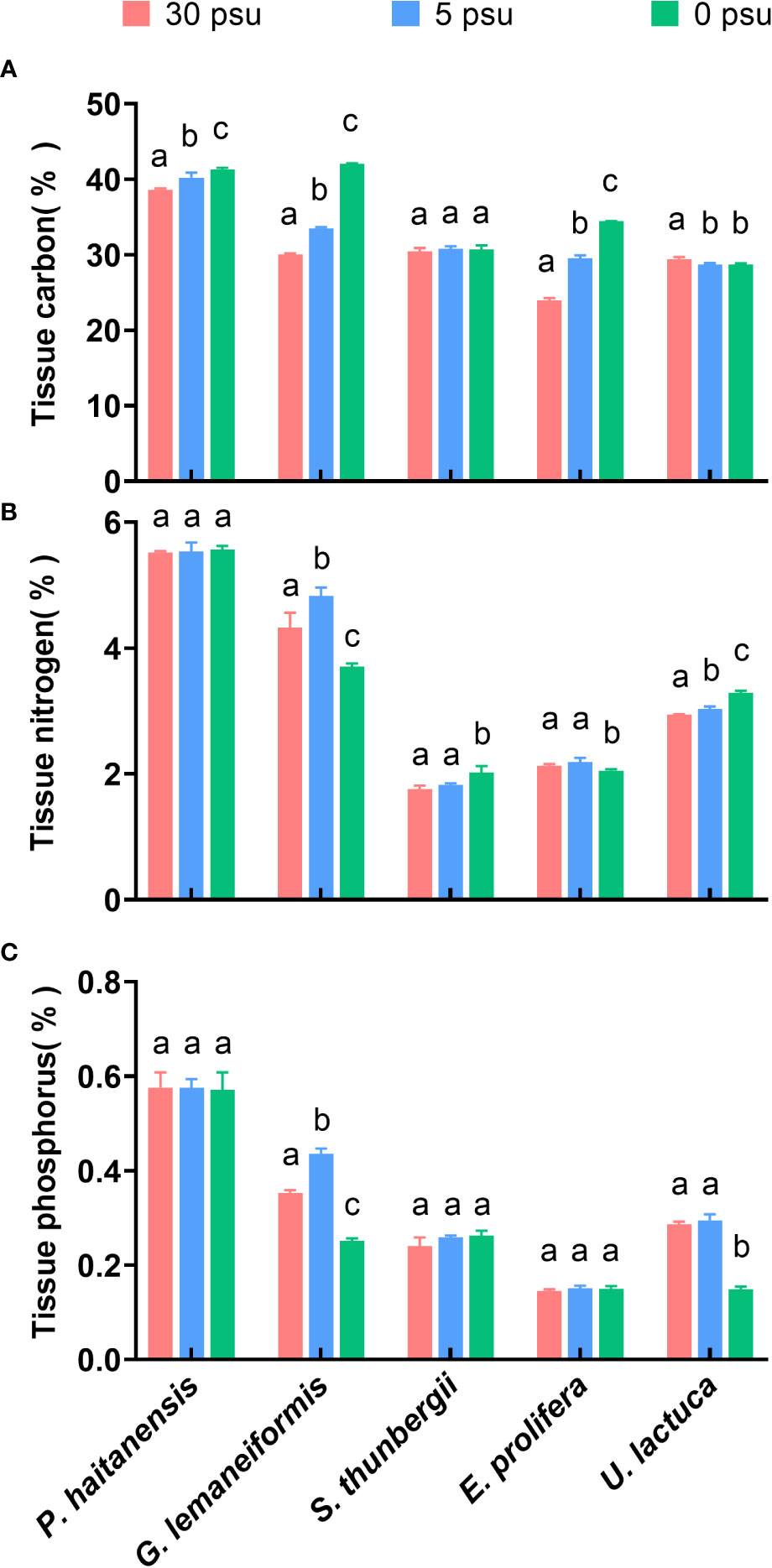
Figure 4 Effects of hyposaline stress on tissue carbon (A), nitrogen (B), and phosphorus (C) contents (%) of five macroalgal species. Different lowercase letters only indicate significant differences between the three salinity treatments for each macroalgal species. Data are presented as mean ± SD, n = 4. p< 0.05 was considered significant.
The C:N ratios of P. haitaneisis, E. prolifera and G. lemaneiformis decreased with salinity (Figure 5A, p< 0.05), while those of U. lactuca and S. thunbergii showed a significant increase (p< 0.05). The C:P ratios and N:P ratios of all five macroalgal species either slightly changed (p > 0.05) or significantly increased (Figures 5B, C, p< 0.05) by hyposaline stress. A low salinity of 0 PSU significantly increased the C:P ratios of G. lemaneiformis, E. prolifera, and U. lactuca by 97.56%, 39.33%, and 88.06%, respectively (p< 0.01), while increasing the N:P ratios of G. lemaneiformis and U. lactuca by 20.68% and 115.79%, respectively (p< 0.01).
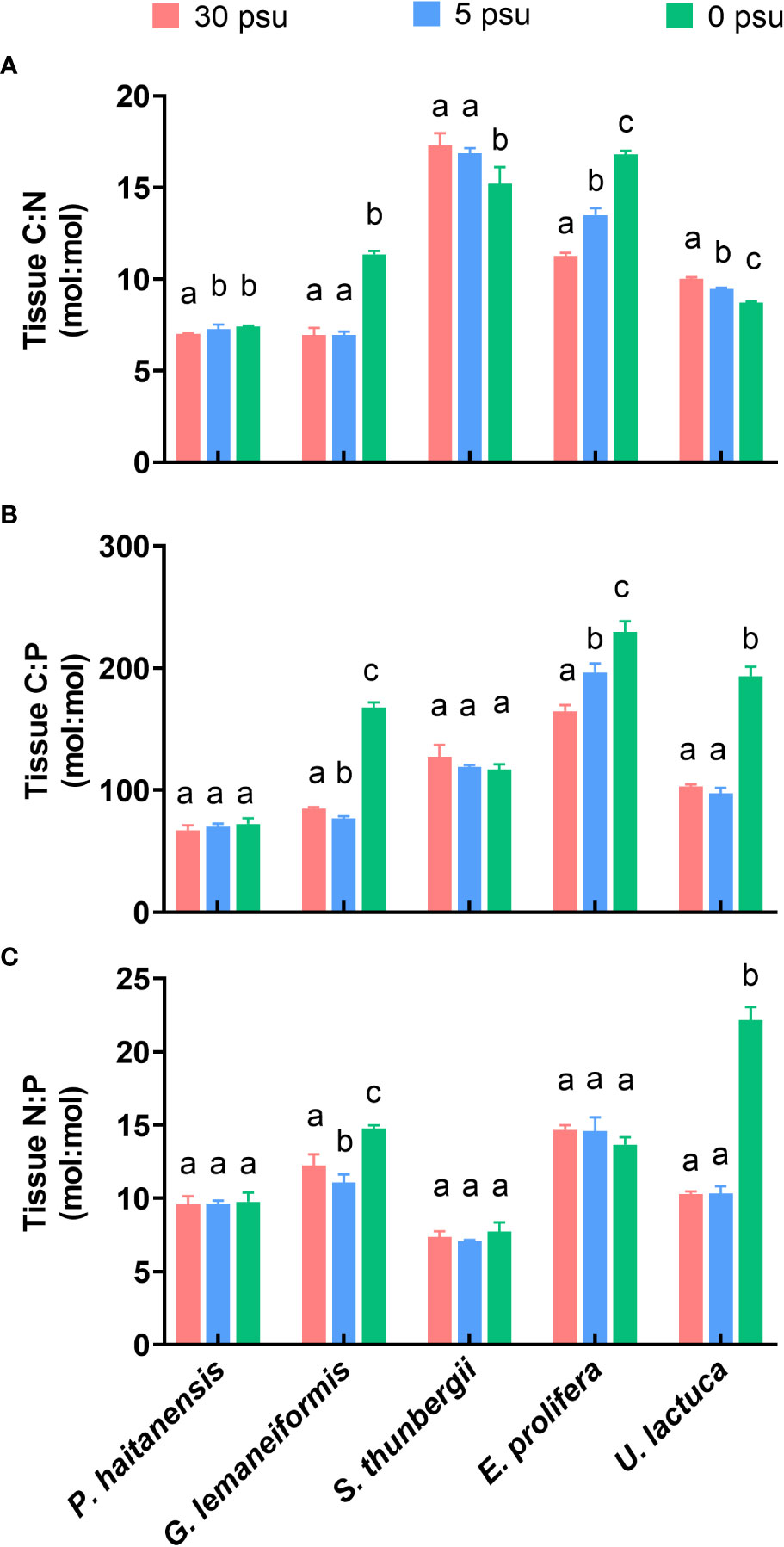
Figure 5 Effects of hyposaline stresses on tissue C:N (A), C:P (B), and N:P (C) molar ratios of five macroalgal species. Different lowercase letters only indicate significant differences between three salinity treatments for each macroalgal species. Data are presented as mean ± SD, n = 4. p< 0.05 was considered significant.
This study also provides a comprehensive analysis of the relationship between elemental ratios and elemental contents by combining data from all five macroalgal species. The C:N ratios of the algal tissues can be expressed as an exponential function of tissue N contents (R2 = 0.8755, Figure 6A), while C:N ratios decreased linearly with increasing P contents (R2 = 0.4100, p< 0.001, Figure 6B). The C:P ratios not only decreased linearly with increasing N contents (R2 = 0.4241, p< 0.001, Figure 6C) but also can be expressed as an exponential function of P contents (R2 = 0.8624, Figure 6D).
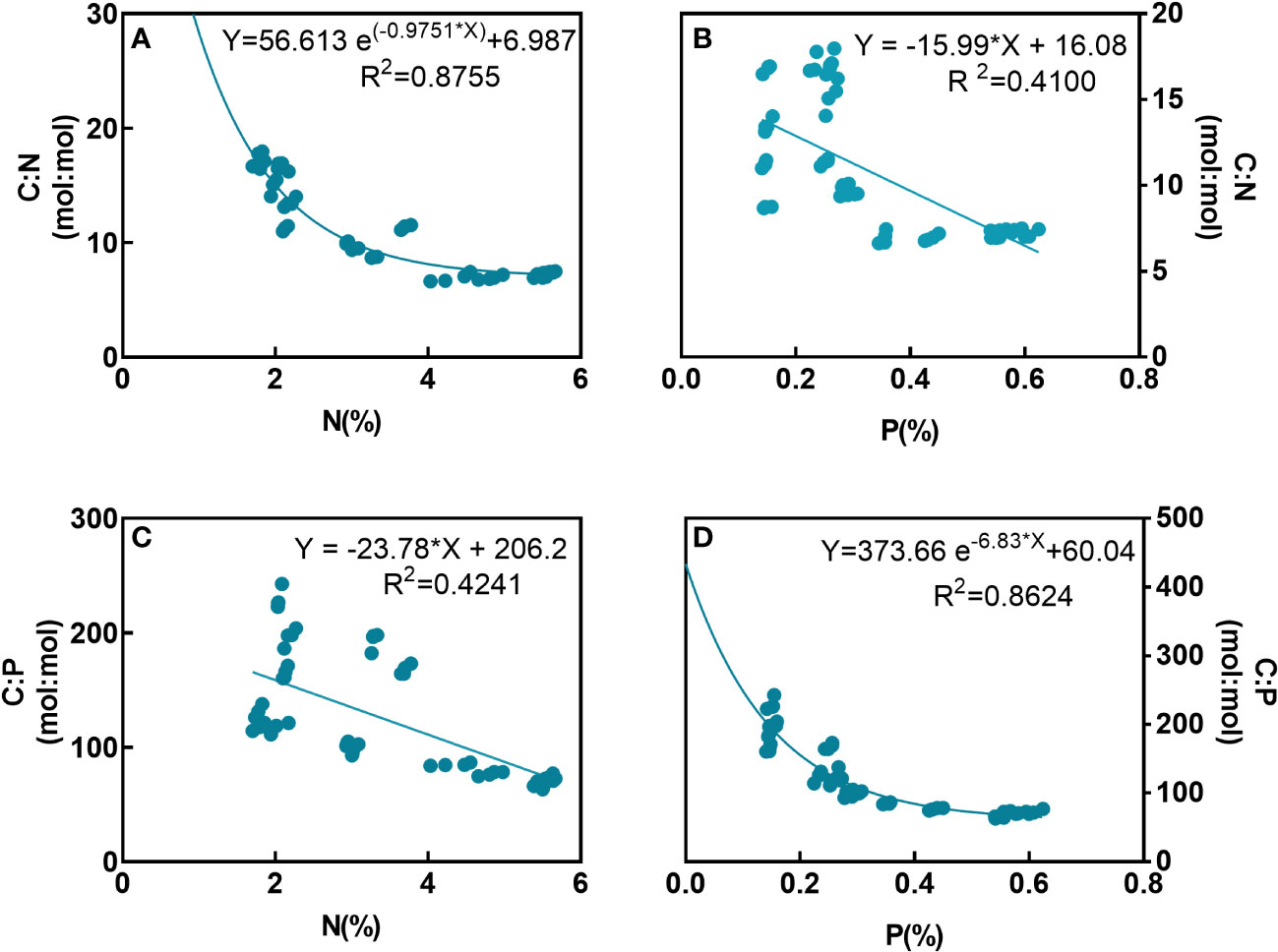
Figure 6 Correlations between N and C:N (A), P and C:N (B), N and C:P (C), and P and C:P (D) of macroalgal tissues.
The correlation analysis (Figure 7) revealed that salinity was negatively correlated with DOC release rate (p< 0.01), tissue C contents (p< 0.01), and C:P ratios (p< 0.05). In contrast, salinity was positively correlated with Pn (p< 0.01), Pt (p< 0.01), fv/fm (p< 0.01), and Rd (p< 0.05). The DOC release rate was negatively correlated with Pn (p< 0.01), Pt, fv/fm (p< 0.05), and positively correlated with tissue C content (p< 0.01), N:P (p< 0.01) and C:P (p< 0.05) ratios.
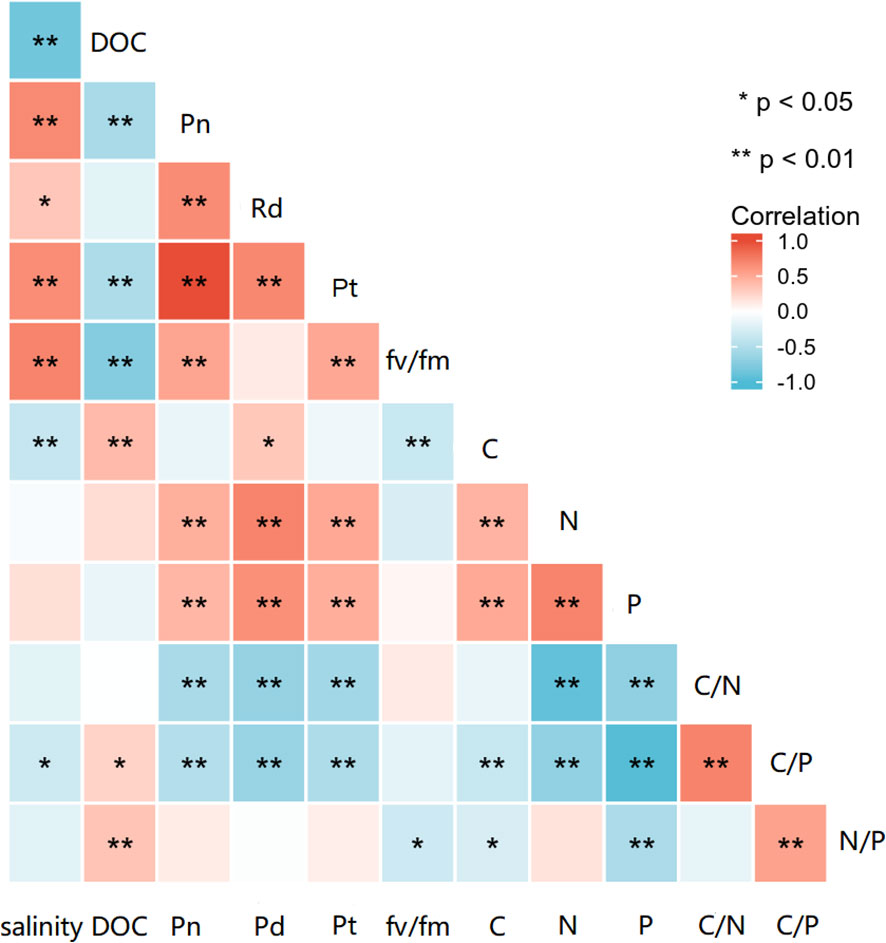
Figure 7 Correlation analysis of salinity with DOC, photosynthetic parameters, tissue C, N, and P contents, and elemental ratios. “*” indicates a significant difference (p< 0.05), “**” indicates an extremely significant difference (p< 0.01).
4. Discussion
Macroalgae can release a large amount of DOC to the environment during growth, and this makes macroalgae have a high carbon sink potential (Krause-Jensen and Duarte, 2016; Ortega et al., 2019; Watanabe et al., 2020; Li et al., 2022). However, a variety of environmental stressors have significant effects on DOC release from macroalgae (Paine et al., 2021; Hurd et al., 2022). This makes it difficult to assess the carbon sink potential of macroalgae. For example, intertidal macroalgae often face low salinity stress caused by rainfall or estuarine flushing of freshwater, and there is a lack of research on the effects of low salinity stress on DOC release from macroalgae. To this end, this study analyzed the effects of hyposaline stress on common macroalgal species in coastal waters of Fujian Province, China, and found that hyposaline treatment significantly inhibited photosynthesis but significantly increased the DOC release rate (Figure 2). Fujian is one of the province of China with the highest average annual rainfall, up to 2,432.6 mm (Fujian-Meteorological-Bureau, 2021). This suggests that the assessment of the carbon sink contribution of macroalgae in Fujian coastal waters must take into account the effects of hyposaline stress.
The tolerance level of different macroalgal species to salinity stress differs significantly (Hurd et al., 2014). This conclusion is consistent with the results of present study. For example, a low salinity of 5 PSU had no significant effect on the fv/fm of P. haitaneisis or S. thunbergii (Figure 1), but significantly inhibited the fv/fm of the other three macroalgal species. In addition, 0 PSU strongly reduced the fv/fm of all five species of macroalgae. This study also found that compared to 30 PSU, hyposaline stress significantly reduced the net photosynthetic rate and total photosynthetic rate of all five macroalgal species (Figure 3). A previous study also showed that the fv/fm of P. haitaneisis and Sargassum fusiforme decreased significantly with the increase of hyposaline treatment time (Chen et al., 2019; Yonemori et al., 2022). Recent studies have shown that hyposaline treatment causes osmotic damage to the cells of P. haitaneisis, resulting in down-regulated expression of relevant genes and proteins involved in photosynthesis, reduced light energy capture and transfer efficiency, and reduced photosynthetic pigment content, thus inhibiting photosynthesis (Wu et al., 2015; Feng et al., 2016; Chen et al., 2019; Wen et al., 2022). These results suggest that 6 h of hyposaline treatment inhibited the photosynthetic rate of macroalgae and negatively affected PSII function.
Under normal growth conditions, about 14% of the carbon fixed by photosynthesis is released by macroalgae to the environment in the form of DOC (Chen et al., 2020a). The present study found that hyposaline treatment significantly increased the release rate of DOC from macroalgae (Figure 2) while strongly decreasing the photosynthetic rate (Figure 3). A previous study by Pregnall (1983) found that osmotic stress caused by salinity fluctuations led to an increase in DOC release from E. prolifera. The DOC release rate of E. prolifera increased from 457.95 μmol C g DW-1 h-1 to 1290.59 μmol C g DW-1 h-1 when the salinity decreased from 30 PSU to 5 PSU (Pregnall, 1983). Similar results were reported by Sieburth (1969) in the macroalgae Chondrus crispus and Fucus vesiculosus. These studies and our results suggest that hyposaline stress causes a double inhibition of organic carbon accumulation in macroalgal tissue (Figure S1).
By analyzing the effect of hyposaline stress on the C contents of macroalgal tissues, we found that hyposaline treatment substantially increased (> 40%) the C contents of G. lemaneiformis and E. prolifera but had a smaller effect (< 7%) on the C contents of P. haitaneisis, S. thunbergii, and U. lactuca. Thus, there were significant interspecific differences in the effects of hyposaline stress on the elemental composition of macroalgae (Figure 4). A previous study found that P enrichment caused a significant increase in tissue P concentrations of the three macroalgal species Caulerpa serrulata, Laurencia intricate, and Sargassum polyphyllum, but the increases were significantly different among the three species (Reef et al., 2012). In conclusion, the physiological responses of marine algae to environmental factors such as salinity, CO2, light, temperature, and nutrients are generally species-specific (Koch et al., 2013; Ji et al., 2016; Ji and Gao, 2021).
Macroalgae need to absorb large amounts of N and P from the environment during growth, and thus large-scale cultivation of macroalgae can alleviate the offshore eutrophication problem and thereby play an important role in the offshore N and P cycles (Xu et al., 2008; Hurd et al., 2014; Mao et al., 2018). In this study, we found significant interspecies differences in the effects of hyposaline treatment on the N and P contents of macroalgae as well as the C:N:P ratios (Figures 4, 5). This is further evidence that different species have different levels of tolerance to low salinity (Figure 1). Many studies have shown that C:N and C:P ratios are inversely correlated with N and P contents (Chen et al., 2020b; Xu et al., 2021; Wang et al., 2022). The results of these studies are consistent with our findings (Figure 6). Therefore, short-term hypersaline stress affects the N and P metabolism of macroalgae, changing their N and P contents and C:N:P ratios, and the resulting biogeochemical effects need to be further investigated.
It is believed that large-scale macroalgae cultivation is a low-cost, high-efficiency blue carbon strategy, which is why this method has attracted a lot of attention (Gao et al., 2021; Yang et al., 2021; Gao et al., 2022). China has abundant macroalgal resources (including wild seaweeds and artificially cultivated seaweeds) and 12.5 million square kilometers of coastal aquaculture area, laying a sufficient foundation for planning the cultivation of large seaweed (Xiao et al., 2017). Therefore, macroalgal cultivation will help China to achieve its carbon neutrality plan. However, the major uncertainty of this method is the difficulty of quantifying the role of macroalgae in the coastal carbon cycle (Paine et al., 2021; Hurd et al., 2022). The present study suggests hyposaline stress needs to be considered when quantifying DOC release from macroalgae. Besides, a recent study found that macroalgal cultivation shaped the microbial community structure by increasing dissolved oxygen, decreasing inorganic nutrients, and releasing of DOC (Xu et al., 2022). Thus, hyposaline stress may indirectly influence the microbial community structure of the tidal zone. Further studies are needed to comprehensively explore the ecological and biogeochemical influence of hyposaline stress on macroalgae.
5. Conclusions
In this study, we found that the DOC release rates of five common macroalgal species in the offshore waters of Fujian Province, namely P. haitaneisis, G. lemaneiformis, S. thunbergii, E. prolifera, and U. lactuca, all increased with decreasing salinity, while photosynthetic rates decreased with decreasing salinity. Both responses could seriously affect the accumulation of organic carbon in tissues of macroalgae. There were significant interspecific differences in the effects of low salinity stress on the carbon, nitrogen, and phosphorus contents and their ratios in the five macroalgal species, which may alter the competitive advantage and ecological functions of macroalgae, thus affecting the offshore carbon, nitrogen, and phosphorus cycles. This study showed that hyposaline stress substantially altered the carbon metabolism of macroalgae, especially increasing the DOC release rate from macroalgae, and therefore the effects of salinity stress should be considered in assessing the carbon sink potential of macroalgae.
Data availability statement
The original contributions presented in the study are included in the article/Supplementary Material. Further inquiries can be directed to the corresponding authors.
Author contributions
JC and KX conducted the data analysis and wrote the first draft of the manuscript. KX, DJ, and YX participated in data collection and sample processing. WW, CX, and CC contributed to the design and interpretation of results. All authors contributed to writing, revising, and approving the submitted version of the manuscript.
Funding
The authors gratefully acknowledge funding from the Science and Technology Program of Education Department of Fujian Province (2022J01801), the National Natural Science Foundation of China (U21A20265), and the China Agriculture Research System (CARS-50-2021), and the Foundation of Jimei University (ZP2020026).
Acknowledgments
We thank LetPub (www.letpub.com) for its linguistic assistance during the preparation of this manuscript.
Conflict of interest
The authors declare that the research was conducted in the absence of any commercial or financial relationships that could be construed as a potential conflict of interest.
Publisher’s note
All claims expressed in this article are solely those of the authors and do not necessarily represent those of their affiliated organizations, or those of the publisher, the editors and the reviewers. Any product that may be evaluated in this article, or claim that may be made by its manufacturer, is not guaranteed or endorsed by the publisher.
Supplementary material
The Supplementary Material for this article can be found online at: https://www.frontiersin.org/articles/10.3389/fmars.2022.1106703/full#supplementary-material
Supplementary Figure 1 | Effects of hyposaline stress on DOC release, photosynthesis, and respiration of macroalgae.
References
Chen T., Wang W., Xu K., Xu Y., Ji D., Chen C., et al. (2019). K+ and na+ transport contribute to K+/Na+ homeostasis in Pyropia haitanensis under hypersaline stress. Algal Res. 40, 101526. doi: 10.1016/j.algal.2019.101526
Chen S., Xu K., Ji D., Wang W., Xu Y., Chen C., et al. (2020a). Release of dissolved and particulate organic matter by marine macroalgae and its biogeochemical implications. Algal Res. 52, 102096. doi: 10.1016/j.algal.2020.102096
Chen S., Xu K., Wang W., Xu Y., Chen C., Xie C., et al. (2020b). Growth and contents of c, n, and p of kelp (Saccharina japonica) cultured in nanri island, China and its effects on particulate and dissolved organic matter of seawater. J. Fisheries China 44, 1–11. doi: 10.11964/jfc.20190811917
Feng C., Ding H., Yan X. (2016). Transcriptomic profiling of Pyropia haitanensis blade in responding to low-salinity stress. J. Fisheries China 40, 1842–1849. doi: 10.11964/jfc.20160410374
Field C. B., Behrenfeld M. J., Randerson J. T., Falkowski P. (1998). Primary production of the biosphere: integrating terrestrial and oceanic components. Science 281, 237–240. doi: 10.1126/science.281.5374.237
Fogg G. E. (1983). The ecological significance of extracellular products of phytoplankton photosynthesis. Botanica Marina 26, 3–14. doi: 10.1515/botm.1983.26.1.3
Fujian-Meteorological-Bureau (2021). Fujian climate bulletin. (Fujian, China). Available at: http://fj.weather.com.cn/zxfw/qhgb/03/3527254.shtml.
Gao G., Beardall J., Jin P., Gao L., Xie S., Gao K. (2022). A review of existing and potential blue carbon contributions to climate change mitigation in the anthropocene. J. Appl. Ecol. 59, 1686–1699. doi: 10.1111/1365-2664.14173
Gao G., Gao L., Jiang M., Jian A., He L. (2021). The potential of seaweed cultivation to achieve carbon neutrality and mitigate deoxygenation and eutrophication. Environ. Res. Lett. 17, 014018. doi: 10.1088/1748-9326/ac3fd9
Hatcher B. G., Chapman A. R. O., Mann K. H. (1977). An annual carbon budget for the kelp Laminaria iongicruris. Mar. Biol. 44, 85–96. doi: 10.1007/BF00386909
Hurd C. L., Harrison P. J., Bischof K., Lobban C. S. (2014). Seaweed ecology and physiology (Cambridge: Cambridge University Press).
Hurd C. L., Law C. S., Bach L. T., Britton D., Hovenden M., Paine E. R., et al. (2022). Forensic carbon accounting: Assessing the role of seaweeds for carbon sequestration. J. Phycology. 58, 347–363. doi: 10.1111/jpy.13249
Ji Y., Gao K. (2021). Effects of climate change factors on marine macroalgae: A review. Adv. Mar. Biol. 88, 91–136. doi: 10.1016/bs.amb.2020.11.001
Ji Y., Xu Z., Zou D., Gao K. (2016). Ecophysiological responses of marine macroalgae to climate change factors. J. Appl. Phycology 28, 2953–2967. doi: 10.1007/s10811-016-0840-5
Koch M., Bowes G., Ross C., Zhang X. H. (2013). Climate change and ocean acidification effects on seagrasses and marine macroalgae. Glob Chang Biol. 19, 103–132. doi: 10.1111/j.1365-2486.2012.02791.x
Krause-Jensen D., Duarte C. M. (2016). Substantial role of macroalgae in marine carbon sequestration. Nat. Geosci. 9, 737–742. doi: 10.1038/ngeo2790
Krause-Jensen D., Lavery P., Serrano O., Marba N., Masque P., Duarte C. M. (2018). Sequestration of macroalgal carbon: the elephant in the blue carbon room. Biol. Lett. 14, 20180236. doi: 10.1098/rsbl.2018.0236
Livanou E., Lagaria A., Psarra S., Lika K. (2019). A DEB-based approach of modeling dissolved organic matter release by phytoplankton. J. Sea Res. 143, 140–151. doi: 10.1016/j.seares.2018.07.016
Li H., Zhang Z., Xiong T., Tang K., He C., Shi Q., et al. (2022). Carbon sequestration in the form of recalcitrant dissolved organic carbon in a seaweed (Kelp) farming environment. Environ. Sci. Technology. 56, 9112–9122. doi: 10.1021/acs.est.2c01535
Ma B. (2021). Spatial distribution characteristics of phytoplankton chlorophyll and its relationship with environmental factors in the pearl river estuary in summer (Guangdong Ocean University).
Mao Y., Li J., Xue S., Lin F., Jiang Z., Fang J., et al. (2018). Ecological functions of the kelp Saccharina japonica in integrated multi-trophicaquaculture, sanggou bay, China. Acta Ecologica Sin. 38, 3230–3237. doi: 10.5846/stxb201703160444
Nellemann C., Corcoran E., Duarte C. M., Valdés L., Young C. D., Fonseca L. E., et al. (2009). Blue Carbon : The Role of Healthy Oceans in Binding carbon. A Rapid Response Assessment. (Arendal, Norway: GRID-Arendal).
Ortega A., Geraldi N. R., Alam I., Kamau A. A., Acinas S. G., Logares R., et al. (2019). Important contribution of macroalgae to oceanic carbon sequestration. Nat. Geosci. 12, 748–754. doi: 10.1038/s41561-019-0421-8
Paine E. R., Schmid M., Boyd P. W., Diaz-Pulido G., Hurd C. L. (2021). Rate and fate of dissolved organic carbon release by seaweeds: A missing link in the coastal ocean carbon cycle. J. Phycol 57, 1375–1391. doi: 10.1111/jpy.13198
Pregnall A. M. (1983). Release of dissolved organic-carbon from the estuarine intertidal macroalga Enteromorpha prolifera. Mar. Biol. 73, 37–42. doi: 10.1007/BF00396283
Raven J. (2018). Blue carbon: past, present and future, with emphasis on macroalgae. Biol. Lett. 14, 20180336. doi: 10.1098/rsbl.2018.0336
Reef R., Pandolfi J. M., Lovelock C. E. (2012). The effect of nutrient enrichment on the growth, nucleic acid concentrations, and elemental stoichiometry of coral reef macroalgae. Ecol. Evol. 2, 1985–1995. doi: 10.1002/ece3.330
Schlesinger W. H., Bernhardt E. S. (2020). Biogeochemistry : an analysis of global change (Amsterdam: Elsevier).
Shriwastav A., Sudarsan G., Bose P., Tare V. (2017). A modified winkler's method for determination of dissolved oxygen concentration in water: Dependence of method accuracy on sample volume. Measurement 106, 190–195. doi: 10.1016/j.measurement.2017.05.004
Sieburth J. M. (1969). Studies on algal substances in the sea. III. the production of extracellular organic matter by littoral marine algae. J. Exp. Mar. Biol. Ecol. 3, 290–309. doi: 10.1016/0022-0981(69)90052-5
Solórzano L., Sharp J. H. (1980). Determination of total dissolved phosphorus and particulate phosphorus in natural waters. Limnology and Oceanograp. 25, 754–758. doi: https://doi.org/10.4319/lo.1980.25.4.0754.
Thornton D. C. O. (2014). Dissolved organic matter (DOM) release by phytoplankton in the contemporary and future ocean. Eur. J. Phycology 49, 20–46. doi: 10.1080/09670262.2013.875596
Tian S., Chen B., Wu M., Cao C., Gu Z., Zheng T., et al. (2023). Are there environmental benefits derived from coastal aquaculture of Sargassum fusiforme? Aquaculture 563, 738909. doi: 10.1016/j.aquaculture.2022.738909
Wang H., Shan L., Yang J., Xie T., Shi Y., Li M., et al. (2022). Effects of precipitation changes on the carbon, nitrogen and phosphorus ecological stoichiometry of Reaumuria soongorica and Salsola passerine leaves. Sci. Soil Water Conserv. 20, 48–55. doi: 10.16843/j.sswc.2022.01.007
Watanabe K., Yoshida G., Hori M., Umezawa Y., Moki H., Kuwae T. (2020). Macroalgal metabolism and lateral carbon flows can create significant carbon sinks. Biogeosciences 17, 2425–2440. doi: 10.5194/bg-17-2425-2020
Wen J., Xu K., Ji D., Xu Y., Chen C., Wang W., et al. (2022). The mechanism of maintaining intracellular homeostasis in the red alga Pyropia haitanensis under hyposaline stress. Front. Mar. Sci. 9, 928617. doi: 10.3389/fmars.2022.928617
Wu H., Ding G., Xu Z. (2015). Effects of salt stress on growth and photosynthesis of Pyropia haitanensis(rhodophyta) under different nitrogen conditions. Oceanologia Et Limnologia Sin. 46, 1210–1217. doi: 10.11693/hyhz20150400102
Xiao X., Agusti S., Lin F., Li K., Pan Y., Yu Y., et al. (2017). Nutrient removal from Chinese coastal waters by large-scale seaweed aquaculture. Sci. Rep. 7, 46613. doi: 10.1038/srep46613
Xu N., Wang W., Xu Y., Ji D., Chen C., Xie C., et al. (2021). Effects of nutrient availability on the release of dissolved and particulate organic carbon by Pyropia haitanensis and its implications. Front. Mar. Sci. 8. doi: 10.3389/fmars.2021.696938
Xu N., Wang W., Xu K., Xu Y., Ji D., Chen C., et al. (2022). Cultivation of different seaweed species and seasonal changes cause divergence of the microbial community in coastal seawaters. Front. Microbiol. 13, 988743. doi: 10.3389/fmicb.2022.988743
Xu S., Wen S., Wu W., He P. (2008). Bioremediation of caged fish aquaculture by the red alga gracilaria verrucosa in an integrated multi-trophic aquaculture system. Acta Ecologica Sin. 1466–1475. doi: 10.3321/j.issn:1000-0933.2008.04.013
Yang Y., Luo H., Wang Q., He Z., Long A. (2021). Large-Scale cultivation of seaweed is effective approach to increase marine carbon sequestration and solve coastal environmental problems. China Acad. J. 36, 11. doi: 10.16418/j.issn.1000-3045.20210217103
Keywords: macroalgae, hyposaline stress, dissolved organic carbon, photosynthesis, elemental ratios, release
Citation: Chen J, Ji D, Xu Y, Chen C, Wang W, Xie C and Xu K (2023) Effect of hyposaline stress on the release of dissolved organic carbon from five common macroalgal species. Front. Mar. Sci. 9:1106703. doi: 10.3389/fmars.2022.1106703
Received: 24 November 2022; Accepted: 19 December 2022;
Published: 09 January 2023.
Edited by:
Bin Xia, Qingdao Agricultural University, ChinaReviewed by:
Zhijian Jiang, South China Sea Institute of Oceanology (CAS), ChinaShanying Tong, Ludong University, China
Copyright © 2023 Chen, Ji, Xu, Chen, Wang, Xie and Xu. This is an open-access article distributed under the terms of the Creative Commons Attribution License (CC BY). The use, distribution or reproduction in other forums is permitted, provided the original author(s) and the copyright owner(s) are credited and that the original publication in this journal is cited, in accordance with accepted academic practice. No use, distribution or reproduction is permitted which does not comply with these terms.
*Correspondence: Chaotian Xie, Y3R4aWVAam11LmVkdS5jbg==; Kai Xu, a2FpeHVAam11LmVkdS5jbg==