- School of Earth, Ocean, and Environment, University of South Carolina, Columbia, SC, United States
The emissions of nitrous oxide (N2O), a potent greenhouse gas and ozone-depleting agent, have been steadily increasing from coastal environments, such as salt marsh sediments, as a result of anthropogenic nutrient loading. Biotic processes, including nitrification and denitrification, are the largest sources of N2O from salt marsh sediments. While it is assumed that the bulk of N2O from salt marsh sediment is produced by nitrification and bacterial denitrification, recent reports suggest fungal denitrification may contribute significantly. In this study, four fungi capable of growth under sulfidic conditions were isolated from salt marsh sediments in North Inlet, South Carolina, USA. Fungal species included Purpureocillium lilacinum, Trichoderma harzianum, Trichoderma virens, and Rhodotorula glutinis, as determined by sequencing the18S and 28S rRNA genes. The isotopomer signatures of N2O produced by these fungi were measured using isotope ratio mass spectrometry, which can be used to estimate the contribution of different sources of N2O. Up to 22.8% of nitrite provided in growth media was converted to N2O by fungal strains isolated from salt marsh sediments. The site preference (SP) of N2O produced by salt marsh sediment fungi ranged from 7.5 ± 1.6‰ to 33.4 ± 1.2‰. These values are lower than the SP of N2O from the model fungal denitrifier Fusarium oxysporum (37.1 ± 2.5‰), which is the SP typically used as an endmember in isotope mass balance considerations. The N2O SP values we measured expand the range of N2O SP used for isotope mass balances calculations to determine the relative contribution of fungi to N2O production in salt marsh sediments.
1 Introduction
Salt marshes represent one of the largest carbon sinks on Earth and effectively filter out excess nutrients, organic matter, and pollutants which would otherwise enter the ocean and potentially lead to coastal eutrophication, harmful algal blooms, and oxygen depletion (Vernberg, 1993; Teal and Howes, 2000; Burden et al., 2013). High amounts of primary production lead to the depletion of oxygen by aerobic respiration, allowing diverse anaerobic metabolisms to aid in the removal of nutrients, such as nitrate through denitrification (Kaplan et al., 1979; Kostka et al., 2002; Mcowen et al., 2017; Wu et al., 2021). Denitrification has been shown to impact water, sedimentary, and atmospheric chemistry by removing nitrate () and producing nitrous oxide (N2O) and N2-gas (Philippot et al., 2013; Lecomte et al., 2018; Zheng et al., 2018). The recent increases in N2O emissions from coastal environments is primarily due to the increases in anthropogenic N in riverine discharge (Murray et al., 2015; Martin et al., 2018; Al-Haj and Fulweiler, 2020; Tian et al., 2020). N2O is of concern as it is a greenhouse gas with a warming potential 296 times higher than carbon dioxide (CO2) and is an ozone-depleting agent (IPCC, 2014). Understanding N2O sources in salt marshes and how the sources may change with climate change is, therefore, crucial. Multiple studies have indicated that anaerobic biogeochemical processes mediated by fungi are understudied in marine environments. This study focuses on marine fungal denitrifiers, which have been hypothesized to significantly contribute to salt marsh N2O production (Gadd, 2006; Grossart et al., 2016; Wankel et al., 2017; Amend et al., 2019; Gutiérrez et al., 2020; Aldossari and Ishii, 2021).
Denitrification is a stepwise reaction where is reduced to nitrite (), nitric oxide (NO), N2O, and dinitrogen gas (N2) through a series of enzymatic reactions encoded by the following genes: respiratory nitrate reductase/periplasmic nitrate reductase (narG/napA), copper/iron containing nitrite reductase (nirK/nirS), nitric oxide reductase (nor in bacteria/p450nor in fungi), and nitrous oxide reductase (nosZ) (Nakahara et al., 1993; Zumft, 1997). Fungi differ from bacteria and archaea in that they lack nosZ (Shoun et al., 1992; Hayatsu et al., 2008). Fungal N2O is thereby released into the environment where it can be consumed by N2O-reducing bacteria or emitted to the atmosphere (Higgins et al., 2018).
Since the turn of the century, terrestrial studies regarding N2O emissions have shown that fungi, rather than bacteria, are a dominant source of soil N2O production and account for up to 80% of released N2O (Laughlin and Stevens, 2002; Ma et al., 2008). While bacterial and archaeal processes are considered the dominant source of N2O from salt marshes, recent studies suggest fungal denitrification may contribute a greater share of N2O emissions in coastal sediments than initially thought, similar to terrestrial counterparts (Wankel et al., 2017; Aldossari and Ishii, 2021). Recent advancements in sequencing technology and cultivation efforts have been instrumental in uncovering the estimated 10,000 species of undiscovered marine fungi, many of which may be capable of denitrification, a widespread trait amongst fungi (Jones, 2011; Maeda et al., 2015; Amend et al., 2019). Furthermore, recent studies have shown that the relative abundance of fungal denitrifiers is positively correlated with nutrient loading and is a significant N2O source in estuaries (Kearns et al., 2019; Kim et al., 2020; Gao et al., 2022). The lack of fungal denitrifiers in recovering salt marshes has also been shown to limit bioavailable nitrogen () removal, thus demonstrating the role denitrifying fungi play in salt marsh sediment biogeochemistry (Starr et al., 2022). These studies provide a basis to rethink salt marsh N2O dynamics and study salt marsh fungi to determine the significance of fungi in acting as an N2O source.
Isotopic approaches can be used to distinguish fungal N2O production from other sources (Sutka et al., 2008; Rohe et al., 2014; Rohe et al., 2017; Wankel et al., 2017). N2O is an asymmetric molecule where the centrally positioned alpha nitrogen (Nα) atom is bonded to the beta positioned nitrogen (Nβ) and oxygen. Differences in isotopic fractionation between fungi, bacteria, and archaea produce changes in the positioning of light and heavy nitrogen atoms (14N, 15N) in the N2O molecule (Sutka et al., 2006; Maeda et al., 2015). The intramolecular distribution of 15N is defined by site preference (SP).
N2O SP is independent of both the initial isotopic composition of the substrate and changes with subsequent consumption (Toyoda et al., 2002; Sutka et al., 2006). Therefore, N2O SP is thought to be only process-dependent and has been used as a tracer to identify the source of N2O in terrestrial and marine environments (Butterbach-Bahl et al., 2013; Bourbonnais et al., 2017; Casciotti et al., 2018; Kelly et al., 2021). Fungal N2O SP measured for the model denitrifying fungi Fusarium oxysporum (37.1 ± 2.5‰) has been used by multiple studies to estimate the contribution of fungi to N2O production from soil and coastal sediments (Rohe et al., 2014; Wankel et al., 2017; Rohe et al., 2021; Su et al., 2021). However, studies have indicated that F. oxysporum is not a well-represented species in fungal communities where Spartina dominates, which is a common feature of salt marshes in Europe, the United States, and China (though invasive), questioning the use of this endmember in isotope mass balances in these environments (Parrondo et al., 1978; Newell et al., 1996; Buchan et al., 2002; Buchan et al., 2003; Torzilli et al., 2006; Ge et al., 2016). Furthermore, the N2O SP of soil-isolated F. oxysporum may not be representative of salt marsh fungi, as saline environments are known to cause physiological responses in fungi, with some studies suggesting fungal denitrification may be enhanced with at higher salinities (Thiem et al., 2018; Yu et al., 2019; Pérez-Llano et al., 2020; Aldossari and Ishii, 2021; Calabon et al., 2021; Jones et al., 2022). N2O SP from salt marsh sediment fungi would therefore be more representative of fungi isolated from saline environments. A recent metabarcoding survey showed that fungi from the families Teratospheariaceae, Mycosphaerellaceae, Physalacriaceae, and Lasiosphaeriaceae and from the orders Capnodiales and Rhytismatales dominated sediment mycobiomes in a New England salt marsh (Kearns et al., 2019). The same study found that the relative abundance of putative denitrifying fungi from the orders Sordariales and Hypocreales were the highest (Kearns et al., 2019). In this study, we isolated four N2O-producing fungal strains from salt marsh sediments and measured their N2O yield and SP.
2 Materials and methods
The first part of this study is designed to isolate N2O-producing fungi from salt marsh sediments. Published studies on fungal N2O production obtained their isolates either from a culture collection (e.g. Maeda et al., 2015) or from the environment using aerobic media (e.g. Jirout, 2015). To the best of our knowledge, we made the first attempt using anaerobic enrichment cultures to isolate fungi from salt marsh sediments, which typically become anoxic below just a few millimeters depth. Given previous reports on the presence of fungi in anoxic parts of salt marsh sediments (e.g. Kearns et al., 2019), we expect the use of anaerobic media will select for fungal lineages well adapted to anoxia. Previous studies on N2O-producing fungi all used complex media including undefined components such as potato infusion and peptone. To select for fungi adapted to low nutrient supply, a defined mineral media recipe (detailed below) was included in our isolation efforts.
2.1 Aerobic media
Aerobic media were prepared with an artificial seawater base containing 20 g L-1 sodium chloride (Fisher Scientific), 3 g L-1 magnesium chloride hexahydrate (Fisher Scientific), 0.15 g L-1 calcium chloride (Fisher Scientific), 0.5 g L-1 potassium chloride (Fisher Scientific) in ultrapure water produced by Milli-Q® EQ 7000 Ultrapure Water Purification System (MilliporeSigma, Merck KGaA, Darmstadt, Germany). Mineral media included 10 mM 3-(N-Morpholino)-Propanesulfonic Acid (MOPS) buffer (pH = 7.2, from 1 M stock solution (209.26 g L-1 MOPS free acid from EMP Millipore Corp., 100 mL L-1 5 M sodium hydroxide from Spectrum Chemical Mfg Corp.), 2 mM ammonium chloride (Fisher Scientific), 0.2 mM sodium sulfate (J.T. Baker), 0.146 mM dipotassium phosphate (J.T. Baker), and 0.0588 mM monopotassium phosphate (J.T. Baker), and supplemented with trace elements. The final concentration of trace elements included: 20 μM hydrochloric acid (VWR Chemicals), 7.5 μM ferrous ammonium sulfate (Fisher Scientific), 0.48 μM boric acid (Sigma Chemical Co.), 0.5 μM manganese chloride (Fisher Scientific), 6.8 μM cobalt sulfate (Sigma Chemical Co.), 1.0 μM nickel chloride (Acros Organics), 12 nM copper chloride (Acros Organics), 0.5 μM zinc sulfate (Sigma Chemical Co.), 0.15 μM sodium molybdate (Acros Organics), 25 nM metavanadate (Acros Organics), 9 μM sodium tungstate (Acros Organics), 23 nM sodium selenite (Sigma Chemical Co.). Spartina Alterniflora stems, collected from North Inlet salt marshes, were cleaned and chopped to about 3 mm in size, and included as a carbon substrate in vials (1% w v-1). Complex media were prepared with the same recipe and two additional components, namely 2.5 g L-1 of yeast extract (Fluka BioChemika) and 2.5 g L-1 of peptone (Fluka BioChemika). Solid media were prepared by including 2% (w v-1) agar (Thermo Scientific). After autoclave sterilization and cooling (20 minutes at 121°C), a mixture of penicillin-G sodium (Alfa Aesar) and streptomycin sulfate (Acros Organics) was added to reach a final concentration of 0.2 g L-1 to minimize bacterial growth.
2.2 Anaerobic media
The composition of anaerobic media used to isolate and maintain fungal cultures was identical to aerobic media with the following exceptions. Resazurin, 1 μg L-1, (Acros Organics) was added as a redox indicator; 0.1 mM of sodium sulfide (Acros Organics) and 1 g L-1 of L-cysteine (Acros Organics) were included as reducing reagents. Liquid media were purged with ultra-high purity N2 (Airgas) for 20 to 30 minutes, and 20-ml were dispensed into N2-flushed 65-ml serum vials sealed with butyl rubber septa and aluminum crimps. After autoclave sterilization, each vial of anaerobic media was supplemented with a mixture of 0.2 g L-1 of penicillin-G sodium (Alfa Aesar), 0.2 g L-1streptomycin sulfate (Acros Organics), and 10 μM sodium nitrite (Fisher Chemicals). For isotopic analysis (described below), the anaerobic media were amended with 100 μM . Anaerobic roll tubes for colony picking were prepared as described previously (Peng et al., 2018).
2.3 Isolation and maintenance of N2O-producing fungal strains
Triplicate 30-cm sediment cores were taken from two sites, Clambank and Oyster Landing, at the North Inlet-Winyah Bay National Estuarine Research Reserve in Georgetown, South Carolina (33.35°N, 79.20°W) on June 17th, 2021 (Figure 1). The North Inlet salt marsh is dominated by Spartina alterniflora (Allen et al., 2014). The cores were put on ice during transportation from Georgetown to Columbia, South Carolina, where initial enrichment culture inoculation occurred on the same day.
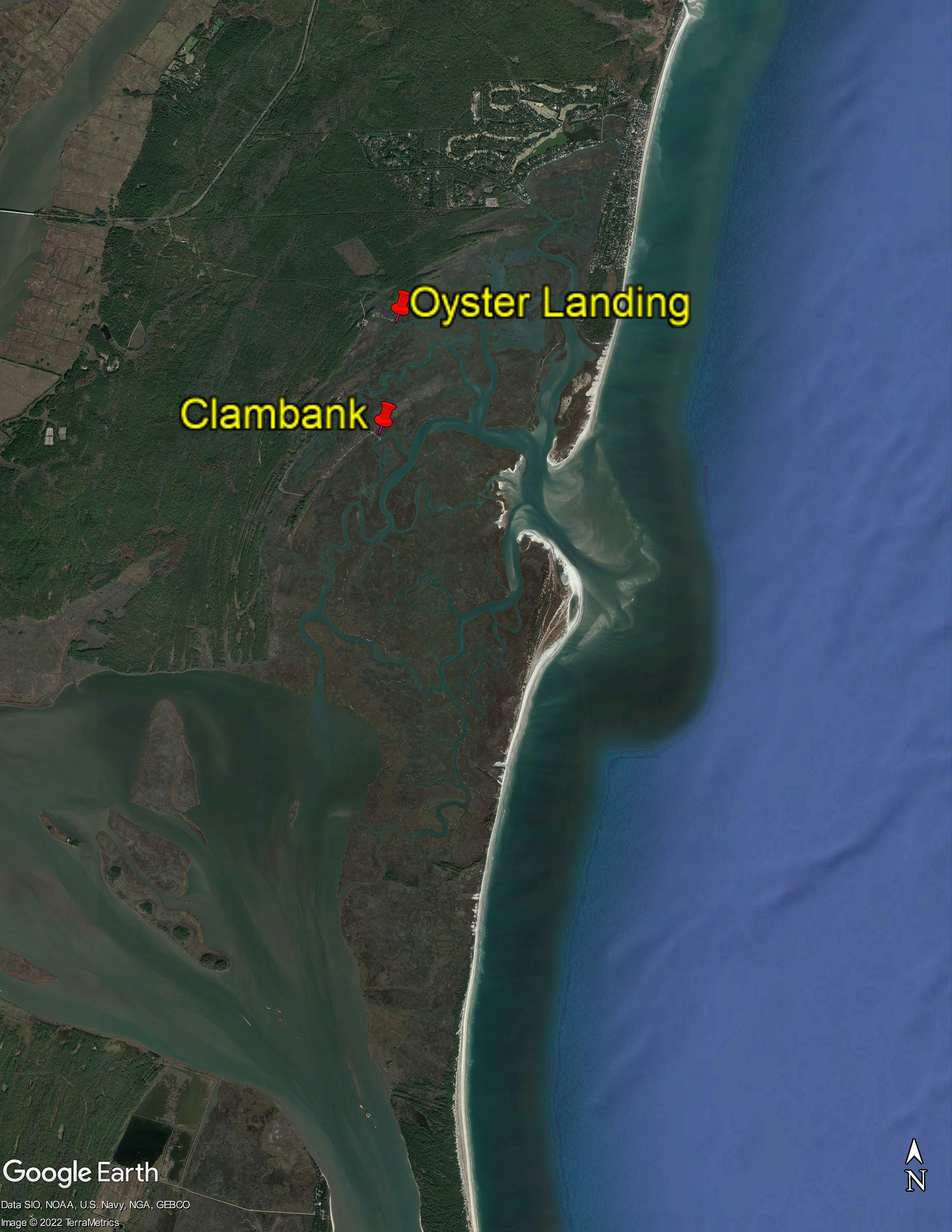
Figure 1 Samling locations (marked by pins) for salt marsh sediments at the North Inlet-Winyah Bay National Estuarine Research Reserve (33.35°N, 79.20°W). The image is produced using Google Earth Pro with data from SIO, NOAA, U.S. Navy, NGA, and GEBCO satellites.
As marine fungi are typically hard to isolate (Edwards et al., 2017; Amend et al., 2019), we used the common plate dilution technique (Warcup, 1950) to isolate N2O-producing fungi that are facultative anaerobes. Sediments from 1 cm and 10 cm depths were diluted in aerobic media and were used to streak agar plates. After three rounds of colony picking, isolated fungal cultures were inoculated into anaerobic media and screened for the ability to grow under sulfidic conditions and produce N2O. Two fungal strains isolated using complex media and one fungal strain isolated using mineral media demonstrated high potential for N2O production.
Anaerobic enrichment cultures of salt marsh sediment fungi were established in an Aldrich® AtmosBag (SKU Z555525) pre-flushed three times with ultra-high purity nitrogen (N2). Approximately 0.1 g of sediment was placed into the serum vials containing anaerobic media using aseptic techniques. All enrichment cultures were screened for N2O production in the headspace using a gas chromatograph (described below) equipped with an electron capture detector (ECD). N2O-producing enrichment cultures were selected for fungal isolation using the anaerobic roll tube technique (Peng et al., 2018). One N2O-producing fungal strain was isolated from anaerobic enrichment cultures. This was the first known case of anaerobic marine fungi isolated under sulfidic conditions.
Isolated fungal cultures were maintained in anaerobic batch cultures, which were transferred to fresh media every 7-10 days. When inoculating fresh media, 0.2 mL of media containing fungal cells were drawn from the inoculum culture using techniques that avoided oxygen contamination. For yeasts, the batch culture serving as the inoculum was thoroughly homogenized before inoculation. For filamentous fungi, we performed careful visual inspection to include similar amounts of filamentous fungi in each 0.2-ml inoculum to ensure replicate cultures had similar amount of initial biomass.
2.4 DNA extraction and rRNA gene sequencing
Fungal cells were harvested by centrifugation and DNA were extracted using the DNeasy Plant Pro kit (QIAGEN). The quantity and quality of the DNA were measured using a Nanodrop 2000C spectrophotometer (ThermoFisher Scientific).
The small (SSU) and large (LSU) subunits of the rRNA genes were amplified using the primers Fun18S1 (5’-CCATGCATGTCTAAGTWTAA-3’) (Lord et al., 2002) and FR1 (5’-ANCCATTCAATCGGTANT-3’) (Vainio and Hantula, 2000) targeting the V1 to V8 regions of the SSU rRNA gene and LR0R (5’-ACCCGCTGAACTTAAGC-3’) and LR5 targeting the D1 to D3 regions of the LSU rRNA gene (Tedersoo et al., 2015). The PCR reactions were performed using Phusion® high-fidelity DNA polymerase (New England BioLabs, M0530, Ipswich, MA). The thermal cycle started with 30 seconds at 98°C, followed by 30 cycles of 10 seconds at 98°C, 30 seconds at 60°C, and 30 seconds at 72°C. The final elongation at 72°C was 5 minutes long. The PCR products were gel purified using Zymo DNA Clean & Concentrator -5 following the manufacturer’s protocol and sent to Etonbio (Research Triangle Park, North Carolina, USA) for Sanger sequencing.
SSU and LSU sequences were searched against the NCBI nt database using the web portal for blastn (Johnson et al., 2008). Sequences for phylogenetic analysis were retrieved from the NCBI GenBank. Sequences were aligned using MUSCLE and manually trimmed using MEGA version 11 (Edgar, 2004; Tamura et al., 2021). Maximum-likelihood trees were constructed using FastTree 2.1 with default settings (1,000 bootstrap replicates, Jukes-Cantor model) (Price et al., 2010). The tree was then imported to Interactive Tree of Life (iTOL v6.6) for visualization (Letunic and Bork, 2019).
2.5 Measurements of headspace gas composition
SRI Greenhouse Gas Monitoring Gas Chromatograph, model 8610-0040, equipped with an ECD and a flame ionized detector (FID) was used to measure N2O and CO2 headspace gas production. Prior to gas extraction, 5 mL of ultra-high purity N2 gas was added to vials with a gas-tight syringe and a sterilized needle to avoid air contamination. A gas-tight syringe and a sterilized needle was used to extract 5 mL of headspace gas, which was manually injected into the sampling port on the instrument. Ultra-high purity N2 gas was used as the carrier gas, maintained at 30 psi. The column oven temperature was set at 90 °C. The ECD and FID, coupled to a methanizer for CO2, measurements, were set at 300 °C. N2O standard curves were constructed using 5 ppm, 10 ppm, and 100 ppm N2O (GASCO, Cal Gas Direct Incorporated, Huntington Beach, California, USA). CO2 standard curves were constructed using 1%, 5%, and 10% CO2 calibration standards from GASCO. Multiple reference samples served as controls, including uninoculated media and N2O producing fungal cultures terminated with concentrated sodium hydroxide and purged with N2.
2.6 N2O isotopic analysis
Analysis of N2O stable isotopes and isotopomers were made using an Elementar Americas Inc. isoprime precisION continuous flow, multicollector, isotope-ratio mass spectrometer (CF-MC-IRMS) equipped with a custom purge-and-trap and gas extraction systems, as described in Charoenpong et al. (2014). The CF-MC-IRMS has the necessary collector configuration for simultaneous determination of masses 30, 31 for the NO+ fragment of N2O (determination of δ15Nα) and 44, 45, and 46 (determination of bulk δ15N and δ18O). Cultures for isotopic analysis had a final concentration of 100 μM. A gas tight syringe was used to extract 3-mL to 5-mL headspace gas, which was then injected into the CF-MC-IRMS injection port. N2O was purified in a purge and trap system under helium continuous flow (40 mL/min), CO2 was chemically removed, and H2O vapor was eliminated with both chemical and cryogenic traps. N2O was cryofocused with two liquid N2 traps and passed through a capillary GC column prior to IRMS analysis. These latter steps, including GC column backflushing to eliminate interferences in the SP determination, were nearly identical to what was described by McIlvin and Casciotti (2010). Helium flow was optimized to achieve quantitative extraction and reproducible results, even at low N2O concentrations. N2O concentrations in our samples were calculated from relative peak heights between the samples and a dilution series of pure N2O gas mixtures (in N2) of known N2O concentrations (500 and 7500 ppm). The reproducibility of bulk δ15N and δ18O and SP as well as any instrumental drift were determined from measurements of an internal reference gas distributed through the analytical run. The measurements were calibrated from a four-point calibration correction using N2O standards covering a large range of SP (-92.7‰ - 18.9‰), as well as bulk δ15N and δ18O composition calibrated by S. Toyoda (Tokyo Institute of Technology), and obtained from Joaquim Mohn (EMPA, Swiss Federal Laboratories for Materials Science & Technology). These standards were analyzed in duplicate for each run to quantify the scrambling effect, potential offset and iteratively solve for the different calibration parameters (Frame and Casciotti, 2010; Mohn et al., 2014). Correction for isobaric interference from 17O was included in these procedures. Standard deviations for triplicate measurements of our N2O standards were typically below 0.1‰ for δ15N-N2Obulk, 0.2‰ for δ18O-N2O and 1‰ for SP, which were comparable to values reported by Mohn et al. (2014).
3 Results
3.1 Phylogeny and classification
The four fungal strains we isolated and investigated in this study were Purpureocillium lilacinum BL2022, Trichoderma virens XP2022, Trichoderma harzianum MB2022, and Rhodotourla glutinis MT2022, as identified by blastn and phylogenetic analysis (Supplementary Figures S1, S2, and S3; Supplementary Table 1). T. harzianum and T. virens were closely related to terrestrial and marine derived species, such as T. polysporum and T. citrinoviride. R. glutinis MT2022 was closely related to other species which have been isolated from both marine (R. diobovata) and terrestrial environments (R. graminis and R. babjeavae). P. lilacinum was closely related to terrestrially derived species but was distinct from P.lilacinum CBS284.36. T. harzianum was the only strain isolated using mineral media (Table 1), which did not contain yeast extract or peptone.
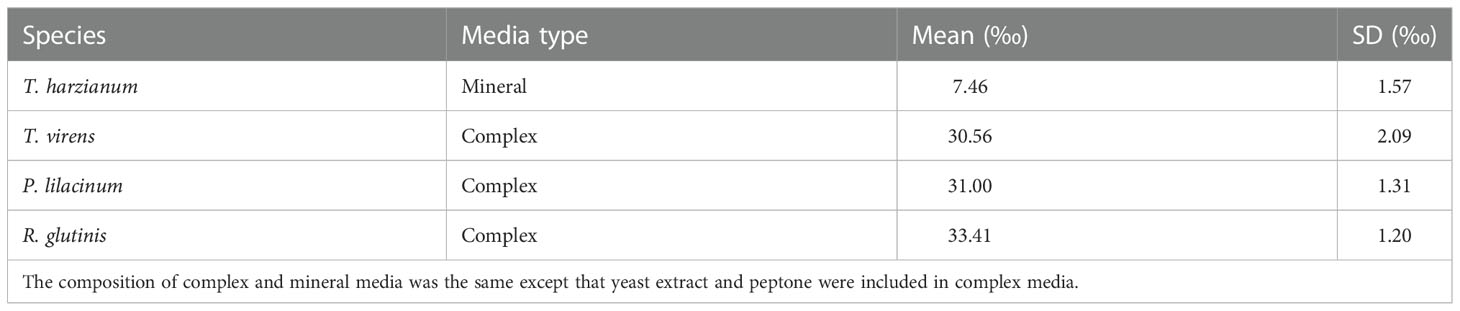
Table 1 The mean and standard deviation (n = 3) of N2O site preference (SP) produced by salt marsh sediment fungal strains from this study.
3.2 N2O and CO2 production from salt marsh sediment fungi
P. lilacinum produced the greatest amount of N2O and CO2 (22.8 ± 7.8 nmol of N2O and 179 ± 24 μmol of CO2, Figures 2A, E). T. virens, T. harzianum, and R. glutinis. produced an average of 17.4 ± 5.4 nmol, 4.45 ± 0.48 nmol, and 4.24 ± 1.57 nmol of N2O (Figures 2B–D) and 39.0 ± 20.0, 5.00 ± 0.44, and 88.1 ± 37.5 μmol of CO2 (Figures 2F–H), respectively. The production of N2O by P. lilacinum did not plateau until the 22nd day. The production of N2O by T. virens plateaued after the 16th day. The production of N2O by T. harzianum and R. glutinis reached a maximum in only two to three days. N2O yield for each fungal strain was calculated using the average maximum N2O produced, where N2O yield is the fraction of N converted to N2O-N (see Supplementary Information for additional details). P.lilacinum, T. virens, T. harzianum, and R. glutinis had respective yields of 22.8 ± 7.8%, 17.4 ± 5.4%, 4.45 ± 0.48%, and 4.24 ± 1.57%. There was a significant correlation between N2O and CO2 production (p< 0.01) for each replicate culture of the filamentous fungi P. lilacinum, T. virens, and T. harzianum (Figure 3). N2O and CO2 production by R. glutinis were not significantly correlated.
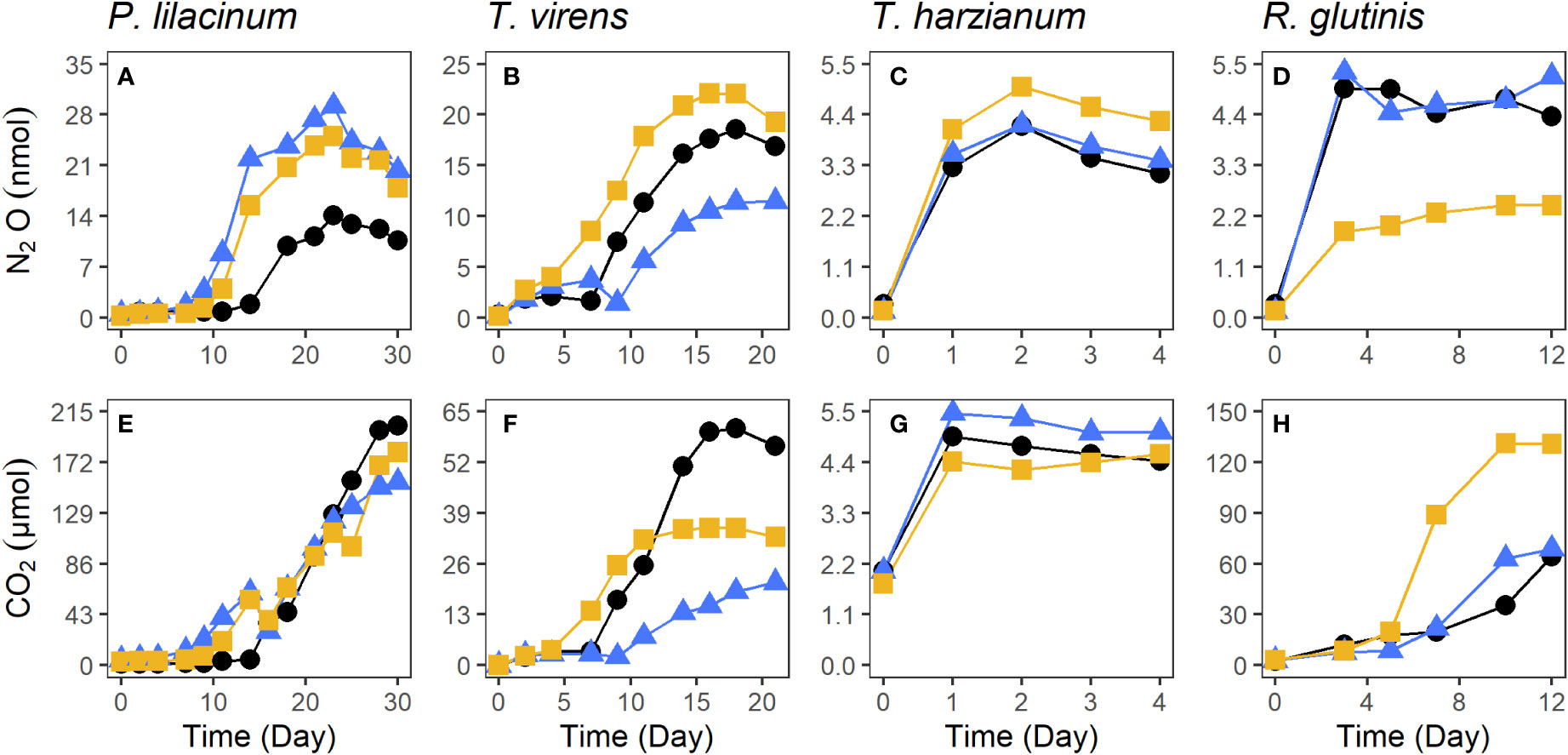
Figure 2 The production of N2O and CO2 by P. lilacinum (A, E), T. virens (B, F), T. harzianum (C, G), and R. glutinis (D, H) isolated from salt marsh sediments. Each color and symbol combination represents one of the three replicates.
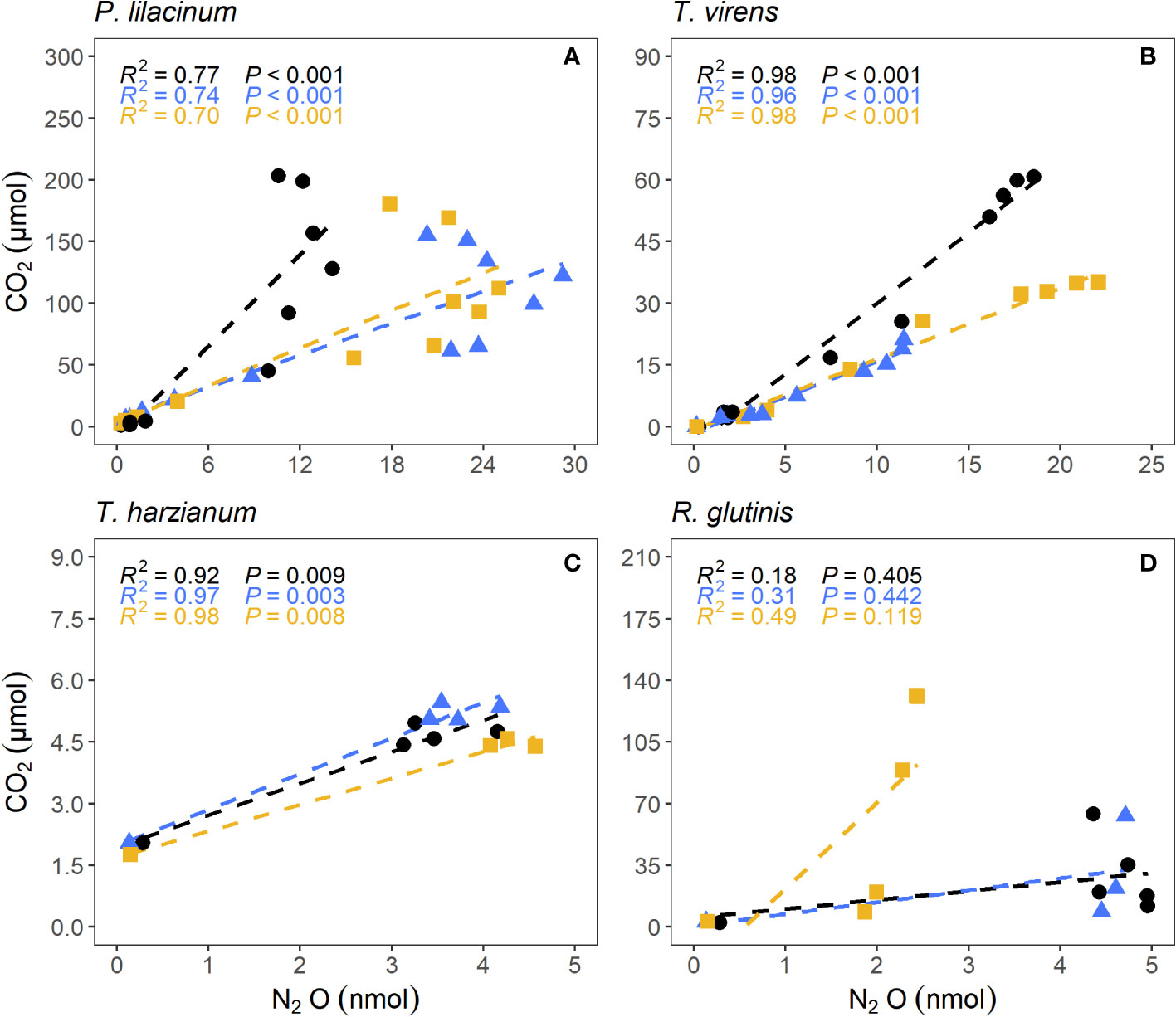
Figure 3 Correlation between N2O and CO2 production by salt marsh sediment fungi P. lilacinum (A), T. virens (B), T. harzianum (C), and R. glutinis (D). Each color represents one of the three replicates.
To further explore the effect of concentration on fungal N2O production, the species with the highest cumulative N2O production, P. lilacinum, was cultivated with 0 μM, 10 μM, 100 μM in triplicate. When grown without , P. lilacinum produced <1 nmol of N2O (Figure 4). The addition of 100 μM to growth media resulted in a >10-fold increase in N2O production for two of the three replicates when compared to the three replicates grown with 10 μM (285.0 ± 20.1 nmol and 22.8 ± 7.8 nmol of N2O, respectively).
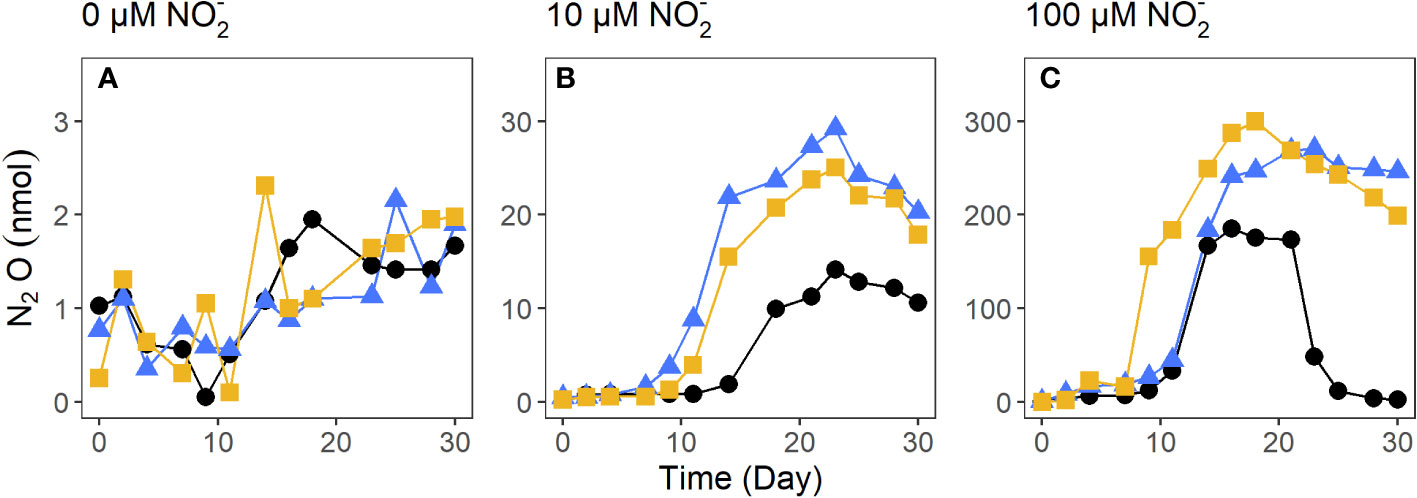
Figure 4 N2O production by the salt marsh sediment fungi P. lilacinum cultivated with 0 μM (A), 10 μM (B), and 100 μM (C). Each color and symbol combination represents one of the three replicates.
3.3 Site preference of N2O from salt marsh sediment fungi
Site preference (SP) values of N2O from salt marsh sediment fungi ranged from 7.49 ± 1.57‰ for T. harzianum, to 33.41 ± 1.20‰ for R. glutinis (Table 1), which were all lower than N2O SP values (37.1 ± 2.5‰) measured from the model fungal denitrifier F. oxysporum (Sutka et al., 2008).
4 Discussion
4.1 N2O production by salt marsh sediment fungi
We used both aerobic and anaerobic cultivation techniques to isolate N2O-producing fungi from salt marsh sediments in North Inlet, South Carolina, USA. The aerobic technique is similar to previous efforts to isolate N2O-producing fungi from soil (Jirout et al., 2013; Mothapo et al., 2013) except that our media were prepared with a seawater base, which selected for fungi adapted to salinity in the range of 30 to 35 ppt. All filamentous species (P. lilacinum, T. virens, and T. harzianum) we isolated from salt marsh sediments have been isolated from soil and shown to produce N2O (Lavrent’ev et al., 2008; Jirout, 2015; Maeda et al., 2015). A comparative genomics studies showed that all these three species possess the diagnostic gene for fungal denitrification, the cytochrome P450 nitric oxide reductase (P450nor) (Higgins et al., 2018). The positive correlations between N2O and CO2 production by filamentous fungi indicate respiratory denitrification is responsible for N2O production. Variability in N2O production, within individual strains’ cultures, is likely due to small differences in the number of cells in the inoculum between triplicates. While this study was not intended as an exhaustive search for N2O-producing fungi from salt marsh sediments, our results indicate that at least a subset of N2O-producing fungi from terrestrial environments (Mothapo et al., 2015) are present in salt marsh sediments and have the potential to produce N2O at high yields.
The ability to produce N2O was widespread among the hundreds of strains tested by Maeda and colleagues (Maeda et al., 2015), but only one (Mucorales) of the 70 N2O-producing strains in their study was not from the phylum Ascomycota. A survey of P450nor in over 700 fungal genomes also showed that this diagnostic gene for fungal denitrification was primarily found in Ascomycota (Higgins et al., 2018). While Ascomycota seem to be the main lineage capable of N2O production, fungi from other phyla, particularly early diverging lineages, are poorly represented in these studies, partially due to their low abundance in terrestrial environments (Berbee et al., 2017). There is evidence that early diverging fungi play a more important role in marine environments than in terrestrial environments, and their potential to produce N2O remains to be examined (Peng and Valentine, 2021).
To the best of our knowledge, this is the first study that used strictly anaerobic techniques to isolate fungi from salt marsh sediments, where oxygen is typically depleted a few millimeters below the surface (Peng et al., 2021). The only N2O-producing fungus isolated anaerobically was the basidiomycetous yeast R. glutinis, which is not known to possess P450nor in its genome (Higgins et al., 2018), but it does possess a fungal nitrite reductase (fungal nirK). The decoupling between N2O and CO2 production in R. glutinis cultures (Figure 3) suggests that N2O production by R. glutinis may not be from respiratory denitrification. Instead, energy production by R. glutinis, an oleaginous yeast, was likely from fermentation (Yeeh, 1999; Xue et al., 2008). This could also explain the low N2O yield by R. glutinis (3.25 ± 1.20%). While this is the first report of N2O production by R. glutinis grown under sulfidic conditions, an unidentified Rhodotorula species grown under aerobic conditions produced N2O after it reached stationary phase (Bleakley and Tiedje, 1982). Therefore, regardless of the mechanism of N2O production by R. glutinis, it can contribute significantly to N2O production from salt marsh sediments where the redox conditions fluctuate due to daily tides. A strong positive correlation was observed between N2O production rate and the relative abundance of Rhodotorula in estuarine sediments in Xiamen, China (Su et al., 2021).
4.2 The influence of cultivation conditions on fungal N2O production
Nearly all previous studies on N2O production by fungi used media containing 10 mM of (Shoun et al., 1992; Mouton et al., 2012; Jirout et al., 2013; Mothapo et al., 2013; Maeda et al., 2015; Zou et al., 2021), which is orders of magnitude higher than the in situ concentrations in soil or marine sediments. It is known that different nutrient levels can cause different physiological responses in fungi, including changes which affect the ability of the cell to transport nutrients and degrade carbon (Ozcan and Johnston, 1999; Zaman et al., 2008). In this study we cultivated N2O-producing fungi using sulfidic media containing at a level (10 μM) much closer to in situ conditions. Consequently, the total amounts of N2O production in our study were lower than previously reported value, but the yield of N2O from by salt marsh sediment fungi (up to 22.8 ± 7.8%) was comparable to some of the highest levels in previous studies (Mothapo et al., 2013; Jirout, 2015; Maeda et al., 2015). Furthermore, the salt marsh sediment fungi were cultivated under sulfidic conditions, demonstrating their relevance in N2O production even at sulfidic depths of the sediments.
The ~10-fold increase in both N2O and CO2 production by P. lilacinum grown with 100 μM (compared to 10 μM ) further supports the notion that respiratory reduction of was the primary mechanism for N2O production, although we cannot rule out the possibility that secondary metabolisms also contribute to N2O production (Higgins et al., 2018). Such a response by P. lilacinum implies that fungal N2O production from salt marsh sediments will scale linearly with nutrient inputs from anthropogenic sources. A recent report demonstrated the drastic increase in N2O production from salt marsh sediments under long-term fertilization (Peng et al., 2021), and fungi may have played a major role in the observed N2O production by the bulk sediment community.
4.3 Site preference of fungi isolated from salt marsh sediment
Stable isotope mass balance has become a useful tool to determine the contribution of fungi to total N2O production (Wankel et al., 2017; Rohe et al., 2021; Su et al., 2021). This approach constrains the contribution of different sources and sinks of N2O by measuring N and O isotopes and isotopomers of N2O from a complex system, which requires the knowledge of endmembers for each pathway (e.g. bacterial denitrification, fungal denitrification). However, studies employing this method so far have relied primarily on the N2O isotopomer signature determined for the model organism F. oxysporum (Sutka et al., 2008), which is not necessarily representative of N2O produced by marine fungi from salt marsh sediments. A recent study using an isotope mass balance approach found that the site preference value and δ18O of N2O from estuarine sediments sometimes exceeded the values for the fungal endmember based on F. oxysporum (Su et al., 2021). We interpret this as additional evidence for the need to evaluate the N2O isotopologue composition produced by fungi from salt marsh sediments.
A study on soil fungi showed that N2O SP ranged from 15.8‰ - 37.1‰, depending on the species (Maeda et al., 2015). Yet, work by Maeda and colleagues (Maeda et al., 2015) has since not been used in any published isotope mass balances calculations. Our findings add to evidence presented by Maeda et al. (2015) that N2O SP depends on the fungal isolate at the species and even strain level. We further present evidence that N2O SP may differ based on growth conditions, even for fungi of the same species. N2O SP values measured by Maeda et al. (2015) for several strains of T. harzianum ranged from 30 - 33.4‰. In contrast, the SP values of N2O produced by the salt marsh sediment T. harzianum from this study (7.46 ± 1.57‰) was much lower, and was the lowest fungal N2O SP reported to date. This may be attributed to two factors. Firstly, the T. harzianum isolated from salt marsh sediments in this study is the first and only N2O-producing fungal culture grown on mineral media to the best of our knowledge. It has been shown that different organic nitrogen sources can impact N2O emissions, though little is known about how N2O SP values would be impacted (Pelster et al., 2012). Secondly, fungal culture media we prepared did not include any soluble sugars (e.g. dextrose), which was a staple ingredient in all previously published studies (Sutka et al., 2008; Rohe et al., 2014; Maeda et al., 2015). Instead, the stems of Spartina alterniflora (lignocellulose) was provided as the sole carbon source to T. harzianum grown on mineral media. It has recently been shown that the type of carbon substrates used to cultivate freshwater bacteria has a significant impact on the SP values of bacterial N2O (Li et al., 2022). It is possible that this is true for N2O-producing fungi as well, though future work is needed to verify.
Along with T. harzianum, other salt marsh sediment fungi in this study produced N2O SP values lower than that from the model fungal denitrifier F. oxysporum (Sutka et al., 2008), indicating that it is inaccurate and oversimplifying to use the N2O SP from one single strain as an endmember for stable isotope mass balance calculations. Many of the N2O SP values measured from core incubations by Wankel and colleagues (Wankel et al., 2017) are within the range of the N2O SP values reported in this study and by Maeda et al. (2015). Future work is needed to determine how carbon and nitrogen substrate types influence fungal N2O SP values. While the range of fungal N2O SP values overlaps with the N2O SP range from abiotic N2O production, abiotic denitrification is favored in high pH conditions with solid iron (III) or copper (II) catalysts (Zhu-Barker et al., 2015) and hence irrelevant or negligible in our cultures (pH buffered at 7.2) and in salt marsh sediments (typically < 7.5). Given the wide range of fungal N2O SP values, the selection of endmember is therefore critical in studies using stable isotope mass balance calculations. The endmember selection can be informed by metabarcoding analysis of the fungal community in general (e.g. targeting the rRNA gene) and the functional genes for fungal denitrification (e.g. nirK gene) (Long et al., 2015; Chen et al., 2016; Maeda et al., 2017).
Data availability statement
The datasets presented in this study are deposited at NCBI GenBank with accession number PRJNA901534. The code to generate figures and calculations implemented in R is deposited at https://github.com/birchmaxwell/SaltMarshFungiN2O.
Author contributions
BL-M is a PhD student supervised by XP and AB. Samples were collected and maintained by BL-M, SL, SS, HB, and XP. BL-M, SS, HB, and JM conducted headspace gas analysis. BL-M and AB conducted IRMS analysis. XP and BL-M conducted sequencing analysis. BL-M conducted data analysis and led the writing of the manuscript. All authors contributed to the article and approved the submitted version.
Funding
This work was supported by the Advanced Support Program for Innovative Research Excellence-I (ASPIRE-I) at the University of South Carolina (Award #216100-21-56788).
Acknowledgments
The authors are grateful for the assistance of Erik Smith during sediment core collection.
Conflict of interest
The authors declare that the research was conducted in the absence of any commercial or financial relationships that could be construed as a potential conflict of interest.
Publisher’s note
All claims expressed in this article are solely those of the authors and do not necessarily represent those of their affiliated organizations, or those of the publisher, the editors and the reviewers. Any product that may be evaluated in this article, or claim that may be made by its manufacturer, is not guaranteed or endorsed by the publisher.
Supplementary material
The Supplementary Material for this article can be found online at: https://www.frontiersin.org/articles/10.3389/fmars.2022.1098508/full#supplementary-material
References
Aldossari N., Ishii S. (2021). Fungal denitrification revisited – recent advancements and future opportunities. Soil Biol. Biochem. 157, 108250. doi: 10.1016/j.soilbio.2021.108250
Allen D. M.Allen W.B.Feller R. F.Plunket J. S., editors. (2014). Site Profile of the North Inlet – Winyah Bay National Estuarine Research Reserve. Georgetown, S.C.: North Inlet – Winyah Bay National Estuarine Research Reserve. 432 pp.
Al-Haj A. N., Fulweiler R. W. (2020). A synthesis of methane emissions from shallow vegetated coastal ecosystems. Glob. Change Biol. 26, 2988–3005. doi: 10.1111/gcb.15046
Amend A., Burgaud G., Cunliffe M., Edgcomb V. P., Ettinger C. L., Gutiérrez M. H., et al. (2019). Fungi in the marine environment: Open questions and unsolved problems. mBio 10, e01189–e01118. doi: 10.1128/mBio.01189-18
Berbee M. L., James T. Y., Strullu-Derrien C. (2017). Early diverging fungi: Diversity and impact at the dawn of terrestrial life. Annu. Rev. Microbiol. 71, 41–60. doi: 10.1146/annurev-micro-030117-020324
Bleakley B. H., Tiedje J. M. (1982). Nitrous oxide production by organisms other than nitrifiers or denitrifiers. Appl. Environ. Microbiol. 44, 1342–1348. doi: 10.1128/aem.44.6.1342-1348.1982
Bourbonnais A., Letscher R. T., Bange H. W., Échevin V., Larkum J., Mohn J., et al. (2017). N2O production and consumption from stable isotopic and concentration data in the Peruvian coastal upwelling system. Glob. Biogeochem. Cycles 31, 678–698. doi: 10.1002/2016GB005567
Buchan A., Newell S. Y., Butler M., Biers E. J., Hollibaugh J. T., Moran M. A. (2003). Dynamics of bacterial and fungal communities on decaying salt marsh grass. Appl. Environ. Microbiol. 69, 6676–6687. doi: 10.1128/AEM.69.11.6676-6687.2003
Buchan A., Newell S. Y., Moreta J. I. L., Moran M. A. (2002). Analysis of internal transcribed spacer (ITS) regions of rRNA genes in fungal communities in a southeastern U.S. salt marsh. Microb. Ecol. 43, 329–340. doi: 10.1007/s00248-001-1062-0
Burden A., Garbutt R. A., Evans C. D., Jones D. L., Cooper D. M. (2013). Carbon sequestration and biogeochemical cycling in a saltmarsh subject to coastal managed realignment. Estuar. Coast. Shelf Sci. 120, 12–20. doi: 10.1016/j.ecss.2013.01.014
Butterbach-Bahl K., Baggs E. M., Dannenmann M., Kiese R., Zechmeister-Boltenstern S. (2013). Nitrous oxide emissions from soils: how well do we understand the processes and their controls? Philos. Trans. R. Soc B Biol. Sci. 368. doi: 10.1098/rstb.2013.0122
Calabon M. S., Jones E. B. G., Promputtha I., Hyde K. D. (2021). Fungal biodiversity in salt marsh ecosystems. J. Fungi 7, 648. doi: 10.3390/jof7080648
Casciotti K. L., Forbes M., Vedamati J., Peters B. D., Martin T. S., Mordy C. W. (2018). Nitrous oxide cycling in the Eastern tropical south pacific as inferred from isotopic and isotopomeric data. Deep Sea res. part II top. Stud. Oceanogr. 156, 155–167. doi: 10.1016/j.dsr2.2018.07.014
Charoenpong C. N., Bristow L. A., Altabet M. A. (2014). A continuous flow isotope ratio mass spectrometry method for high precision determination of dissolved gas ratios and isotopic composition. Limnology and Oceanography: Methods 12, 323–337. doi: 10.4319/lom.2014.12.323
Chen H., Yu F., Shi W. (2016). Detection of N2O-producing fungi in environment using nitrite reductase gene (nirK)-targeting primers. Fungal Biol. 120, 1479–1492. doi: 10.1016/j.funbio.2016.07.012
Edgar R. C. (2004). MUSCLE: multiple sequence alignment with high accuracy and high throughput. Nucleic Acids Res. 32, 1792–1797. doi: 10.1093/nar/gkh340
Edwards J. E., Forster R. J., Callaghan T. M., Dollhofer V., Dagar S. S., Cheng Y., et al. (2017). PCR and omics based techniques to study the diversity, ecology and biology of anaerobic fungi: Insights, challenges and opportunities. Front. Microbiol. 8. doi: 10.3389/fmicb.2017.01657
Frame C. H., Casciotti K. L. (2010). Biogeochemical controls and isotopic signatures of nitrous oxide production by a marine ammonia-oxidizing bacterium. Biogeosciences 7, 2695–2709. doi: 10.5194/bg-7-2695-2010
Gadd G. M. Ed. (2006). Fungi in biogeochemical cycles. (British Mycological Society symposia; No. 24) (Cambridge, England: Cambridge University Press). doi: 10.1017/CBO9780511550522
Gao D., Hou L., Liu M., Zheng Y., Yin G., Niu Y. (2022). N2O emission dynamics along an intertidal elevation gradient in a subtropical estuary: Importance of N2O consumption. Environ. Res. 205, 112432. doi: 10.1016/j.envres.2021.112432
Ge Z.-M., Wang H., Cao H.-B., Zhao B., Zhou X., Peltola H., et al. (2016). Responses of eastern Chinese coastal salt marshes to sea-level rise combined with vegetative and sedimentary processes. Sci. Rep. 6, 28466. doi: 10.1038/srep28466
Grossart H.-P., Wurzbacher C., James T. Y., Kagami M. (2016). Discovery of dark matter fungi in aquatic ecosystems demands a reappraisal of the phylogeny and ecology of zoosporic fungi. Fungal Ecol. 19, 28–38. doi: 10.1016/j.funeco.2015.06.004
Gutiérrez M. H., Vera J., Srain B., Quiñones R. A., Wörmer L., Hinrichs K.-U., et al. (2020). Biochemical fingerprints of marine fungi: implications for trophic and biogeochemical studies. Aquat. Microb. Ecol. 84, 75–90. doi: 10.3354/ame01927
Hayatsu M., Tago K., Saito M. (2008). Various players in the nitrogen cycle: Diversity and functions of the microorganisms involved in nitrification and denitrification. Soil Sci. Plant Nutr. 54, 33–45. doi: 10.1111/j.1747-0765.2007.00195.x
Higgins S. A., Schadt C. W., Matheny P. B., Löffler F. E. (2018). Phylogenomics reveal the dynamic evolution of fungal nitric oxide reductases and their relationship to secondary metabolism. Genome Biol. Evol. 10, 2474–2489. doi: 10.1093/gbe/evy187
Intergovernmental Panel on Climate Change ed (2014). “Anthropogenic and natural radiative forcing,” in Climate change 2013 – the physical science basis: Working group I contribution to the fifth assessment report of the intergovernmental panel on climate change (Cambridge: Cambridge University Press), 659–740. doi: 10.1017/CBO9781107415324.018
Jirout J. (2015). Nitrous oxide productivity of soil fungi along a gradient of cattle impact. Fungal Ecol. 17, 155–163. doi: 10.1016/j.funeco.2015.07.003
Jirout J., Šimek M., Elhottová D. (2013). Fungal contribution to nitrous oxide emissions from cattle impacted soils. Chemosphere 90, 565–572. doi: 10.1016/j.chemosphere.2012.08.031
Johnson M., Zaretskaya I., Raytselis Y., Merezhuk Y., McGinnis S., Madden T. L. (2008). NCBI BLAST: a better web interface. Nucleic Acids Res. 36, W5–W9. doi: 10.1093/nar/gkn201
Jones E. B. G. (2011). Fifty years of marine mycology. Fungal Divers. 50, 73. doi: 10.1007/s13225-011-0119-8
Jones E. B. G., Ramakrishna S., Vikineswary S., Das D., Bahkali A. H., Guo S.-Y., et al. (2022). How do fungi survive in the Sea and respond to climate change? J. Fungi Basel Switz. 8, 291. doi: 10.3390/jof8030291
Kaplan W., Valiela I., Teal J. M. (1979). Denitrification in a salt marsh ecosystem. Limnol. Oceanogr. 24, 726–734. doi: 10.4319/lo.1979.24.4.0726
Kearns P. J., Bulseco-McKim A. N., Hoyt H., Angell J. H., Bowen J. L. (2019). Nutrient enrichment alters salt marsh fungal communities and promotes putative fungal denitrifiers. Microb. Ecol. 77, 358–369. doi: 10.1007/s00248-018-1223-z
Kelly C. L., Travis N. M., Baya P. A., Casciotti K. L. (2021). Quantifying nitrous oxide cycling regimes in the Eastern tropical north pacific ocean with isotopomer analysis. Glob. Biogeochem. Cycles 35, e2020GB006637. doi: 10.1029/2020GB006637
Kim J., Chaudhary D. R., Kang H. (2020). Nitrogen addition differently alters GHGs production and soil microbial community of tidal salt marsh soil depending on the types of halophyte. Appl. Soil Ecol. 150, 103440. doi: 10.1016/j.apsoil.2019.103440
Kostka J. E., Roychoudhury A., Van Cappellen P. (2002). Rates and controls of anaerobic microbial respiration across spatial and temporal gradients in saltmarsh sediments. Biogeochemistry 60, 49–76. doi: 10.1023/A:1016525216426
Laughlin R. J., Stevens R. J. (2002). Evidence for fungal dominance of denitrification and codenitrification in a grassland soil. Soil Sci. Soc Am. J. 66, 1540–1548. doi: 10.2136/sssaj2002.1540
Lavrent’ev R. B., Zaitsev S. A., Sudnitsyn I. I., Kurakov A. V. (2008). Nitrous oxide production by fungi in soils under different moisture levels. Mosc. Univ. Soil Sci. Bull. 63, 178–183. doi: 10.3103/S0147687408040054
Lecomte S. M., Achouak W., Abrouk D., Heulin T., Nesme X., Haichar F.el Z. (2018). Diversifying anaerobic respiration strategies to compete in the rhizosphere. Front. Environ. Sci. 6. doi: 10.3389/fenvs.2018.00139
Letunic I., Bork P. (2019). Interactive tree of life (iTOL) v4: recent updates and new developments. Nucleic Acids Res. 47, W256–W259. doi: 10.1093/nar/gkz239
Li S., Wang S., Ji G. (2022). Influences of carbon sources on N2O production during denitrification in freshwaters: Activity, isotopes and functional microbes. Water Res. 226, 119315. doi: 10.1016/j.watres.2022.119315
Long A., Song B., Fridey K., Silva A. (2015). Detection and diversity of copper containing nitrite reductase genes (nirK) in prokaryotic and fungal communities of agricultural soils. FEMS Microbiol. Ecol. 91, 1–9. doi: 10.1093/femsec/fiu004
Lord N. S., Kaplan C. W., Shank P., Kitts C. L., Elrod S. L. (2002). Assessment of fungal diversity using terminal restriction fragment (TRF) pattern analysis: comparison of 18S and ITS ribosomal regions. FEMS Microbiol. Ecol. 42, 327–337. doi: 10.1111/j.1574-6941.2002.tb01022.x
Maeda K., Spor A., Edel-Hermann V., Heraud C., Breuil M.-C., Bizouard F., et al. (2015). N2O production, a widespread trait in fungi. Sci. Rep. 59697. doi: 10.1038/srep09697
Maeda K., Toyoda S., Philippot L., Hattori S., Nakajima K., Ito Y., et al. (2017). Relative contribution of nirK- and nirS- bacterial denitrifiers as well as fungal denitrifiers to nitrous oxide production from dairy manure compost. Environ. Sci. Technol. 51, 14083–14091. doi: 10.1021/acs.est.7b04017
Ma W. K., Farrell R. E., Siciliano S. D. (2008). Soil formate regulates the fungal nitrous oxide emission pathway. Appl. Environ. Microbiol. 74, 6690–6696. doi: 10.1128/AEM.00797-08
Martin R. M., Wigand C., Elmstrom E., Lloret J., Valiela I. (2018). Long-term nutrient addition increases respiration and nitrous oxide emissions in a new England salt marsh. Ecol. Evol. 8, 4958–4966. doi: 10.1002/ece3.3955
McIlvin M. R., Casciotti K. L. (2010). Fully automated system for stable isotopic analyses of dissolved nitrous oxide at natural abundance levels. Limnology and Oceanography: Methods 8, 54–66. doi: 10.4319/lom.2010.8.54
Mcowen C. J., Weatherdon L. V., Bochove J.-W. V., Sullivan E., Blyth S., Zockler C., et al. (2017). A global map of saltmarshes. Biodivers. Data J., e11764. doi: 10.3897/BDJ.5.e11764
Mohn J., Wolf B., Toyoda S., Lin C.-T., Liang M.-C., Brüggemann N., et al. (2014). Interlaboratory assessment of nitrous oxide isotopomer analysis by isotope ratio mass spectrometry and laser spectroscopy: current status and perspectives. Rapid Commun. Mass Spectrom. 28, 1995–2007. doi: 10.1002/rcm.6982
Mothapo N., Chen H., Cubeta M. A., Grossman J. M., Fuller F., Shi W. (2015). Phylogenetic, taxonomic and functional diversity of fungal denitrifiers and associated N2O production efficacy. Soil Biol. Biochem. 83, 160–175. doi: 10.1016/j.soilbio.2015.02.001
Mothapo N. V., Chen H., Cubeta M. A., Shi W. (2013). Nitrous oxide producing activity of diverse fungi from distinct agroecosystems. Soil Biol. Biochem. 66, 94–101. doi: 10.1016/j.soilbio.2013.07.004
Mouton M., Postma F., Wilsenach J., Botha A. (2012). Diversity and characterization of culturable fungi from marine sediment collected from st. Helena bay, south Africa. Microb. Ecol. 64, 311–319. doi: 10.1007/s00248-012-0035-9
Murray R. H., Erler D. V., Eyre B. D. (2015). Nitrous oxide fluxes in estuarine environments: response to global change. Glob. Change Biol. 21, 3219–3245. doi: 10.1111/gcb.12923
Nakahara K., Tanimoto T., Hatano K., Usuda K., Shoun H. (1993). Cytochrome p-450 55A1 (P-450dNIR) acts as nitric oxide reductase employing NADH as the direct electron donor. J. Biol. Chem. 268, 8350–8355. doi: 10.1016/S0021-9258(18)53102-1
Newell S., Porter D., Lingle W. l. (1996). Lignocellulolysis by ascomycetes (fungi) of a saltmarsh grass (smooth cordgrass). Microsc. Res. Tech. 33, 32–46. doi: 10.1002/(SICI)1097-0029(199601)33:1<32::AID-JEMT5>3.0.CO;2-2
Ozcan S., Johnston M. (1999). Function and regulation of yeast hexose transporters. Microbiol. Mol. Biol. Rev. MMBR 63, 554–569. doi: 10.1128/MMBR.63.3.554-569.1999
Parrondo R. T., Gosselink J. G., Hopkinson C. S. (1978). Effects of salinity and drainage on the growth of three salt marsh grasses. Bot. Gaz. 139, 102–107. doi: 10.1086/336975
Pelster D. E., Chantigny M. H., Rochette P., Angers D. A., Rieux C., Vanasse A. (2012). Nitrous oxide emissions respond differently to mineral and organic nitrogen sources in contrasting soil types. J. Environ. Qual. 41, 427–435. doi: 10.2134/jeq2011.0261
Peng X., Ji Q., Angell J. H., Kearns P. J., Bowen J. L., Ward B. B. (2021). Long-term fertilization alters nitrous oxide cycling dynamics in salt marsh sediments. Environ. Sci. Technol. 55, 10832–10842. doi: 10.1021/acs.est.1c01542
Peng X., Swift C. L., Theodorou M. K., O’Malley M. A. (2018). “Methods for genomic characterization and maintenance of anaerobic fungi,” in Fungal genomics: Methods and protocols methods in molecular biology. Eds. de Vries R. P., Tsang A., Grigoriev I. V. (New York, NY: Springer), 53–67. doi: 10.1007/978-1-4939-7804-5_5
Peng X., Valentine D. L. (2021). Diversity and N2O production potential of fungi in an oceanic oxygen minimum zone. J. Fungi 7, 218. doi: 10.3390/jof7030218
Pérez-Llano Y., Rodríguez-Pupo E. C., Druzhinina I. S., Chenthamara K., Cai F., Gunde-Cimerman N., et al. (2020). Stress reshapes the physiological response of halophile fungi to salinity. Cells 9, 525. doi: 10.3390/cells9030525
Philippot L., Raaijmakers J. M., Lemanceau P., van der Putten W. H. (2013). Going back to the roots: the microbial ecology of the rhizosphere. Nat. Rev. Microbiol. 11, 789–799. doi: 10.1038/nrmicro3109
Price M. N., Dehal P. S., Arkin A. P. (2010). FastTree 2 – approximately maximum-likelihood trees for Large alignments. PloS One 5, e9490. doi: 10.1371/journal.pone.0009490
Rohe L., Anderson T.-H., Braker G., Flessa H., Giesemann A., Lewicka-Szczebak D., et al. (2014). Dual isotope and isotopomer signatures of nitrous oxide from fungal denitrification – a pure culture study. Rapid Commun. Mass Spectrom. 28, 1893–1903. doi: 10.1002/rcm.6975
Rohe L., Apelt B., Vogel H.-J., Well R., Wu G.-M., Schlüter S. (2021). Denitrification in soil as a function of oxygen availability at the microscale. Biogeosciences 18, 1185–1201. doi: 10.5194/bg-18-1185-2021
Rohe L., Well R., Lewicka-Szczebak D. (2017). Use of oxygen isotopes to differentiate between nitrous oxide produced by fungi or bacteria during denitrification. Rapid Commun. Mass Spectrom. 31, 1297–1312. doi: 10.1002/rcm.7909
Shoun H., Kim D.-H., Uchiyama H., Sugiyama J. (1992). Denitrification by fungi. FEMS Microbiol. Lett. 94, 277–281. doi: 10.1111/j.1574-6968.1992.tb05331.x
Starr S. F., Mortazavi B., Tatariw C., Kuehn K. A., Cherry J. A., Ledford T., et al. (2022). Poor recovery of fungal denitrification limits nitrogen removal capacity in a constructed gulf coast marsh. Soil Biol. Biochem. 170, 108692. doi: 10.1016/j.soilbio.2022.108692
Sutka R. L., Adams G. C., Ostrom N. E., Ostrom P. H. (2008). Isotopologue fractionation during N2O production by fungal denitrification. Rapid Commun. Mass Spectrom. 22, 3989–3996. doi: 10.1002/rcm.3820
Sutka R. L., Ostrom N. E., Ostrom P. H., Breznak J. A., Gandhi H., Pitt A. J., et al. (2006). Distinguishing nitrous oxide production from nitrification and denitrification on the basis of isotopomer abundances. Appl. Environ. Microbiol. 72, 638–644. doi: 10.1128/AEM.72.1.638-644.2006
Su X., Wen T., Wang Y., Xu J., Cui L., Zhang J., et al. (2021). Stimulation of N2O emission via bacterial denitrification driven by acidification in estuarine sediments. Glob. Change Biol. 27, 5564–5579. doi: 10.1111/gcb.15863
Tamura K., Stecher G., Kumar S. (2021). MEGA11: Molecular evolutionary genetics analysis version 11. Mol. Biol. Evol. 38, 3022–3027. doi: 10.1093/molbev/msab120
Teal J. M., Howes B. L. (2000). “Salt marsh values: Retrospection from the end of the century,” in Concepts and controversies in tidal marsh ecology. Eds. Weinstein M. P., Kreeger D. A. (Dordrecht: Springer Netherlands), 9–19. doi: 10.1007/0-306-47534-0_2
Tedersoo L., Anslan S., Bahram M., Põlme S., Riit T., Liiv I., et al. (2015). Shotgun metagenomes and multiple primer pair-barcode combinations of amplicons reveal biases in metabarcoding analyses of fungi. MycoKeys 10, 1–43. doi: 10.3897/mycokeys.10.4852
Thiem D., Gołębiewski M., Hulisz P., Piernik A., Hrynkiewicz K. (2018). How does salinity shape bacterial and fungal microbiomes of alnus glutinosa roots? Front. Microbiol. 9. doi: 10.3389/fmicb.2018.00651
Tian H., Xu R., Canadell J. G., Thompson R. L., Winiwarter W., Suntharalingam P., et al. (2020). A comprehensive quantification of global nitrous oxide sources and sinks. Nature 586, 248–256. doi: 10.1038/s41586-020-2780-0
Torzilli A. P., Sikaroodi M., Chalkley D., Gillevet P. M. (2006). A comparison of fungal communities from four salt marsh plants using automated ribosomal intergenic spacer analysis (ARISA). Mycologia 98, 690–698. doi: 10.1080/15572536.2006.11832641
Toyoda S., Yoshida N., Miwa T., Matsui Y., Yamagishi H., Tsunogai U., et al. (2002). Production mechanism and global budget of N2O inferred from its isotopomers in the western north pacific. Geophys. Res. Lett. 29, 7–4. doi: 10.1029/2001GL014311
Vainio E. J., Hantula J. (2000). Direct analysis of wood-inhabiting fungi using denaturing gradient gel electrophoresis of amplified ribosomal DNA. Mycol. Res. 104, 927–936. doi: 10.1017/S0953756200002471
Vernberg F. J. (1993). Salt-marsh processes: A review. Environ. Toxicol. Chem. 12, 2167–2195. doi: 10.1002/etc.5620121203
Wankel S. D., Ziebis W., Buchwald C., Charoenpong C., de Beer D., Dentinger J., et al. (2017). Evidence for fungal and chemodenitrification based N2O flux from nitrogen impacted coastal sediments. Nat. Commun. 8, 15595. doi: 10.1038/ncomms15595
Warcup J. H. (1950). The soil-plate method for isolation of fungi from soil. Nature 166, 117–118. doi: 10.1038/166117b0
Wu J., Hong Y., Liu X., Hu Y. (2021). Variations in nitrogen removal rates and microbial communities over sediment depth in daya bay, China. Environ. pollut. 286, 117267. doi: 10.1016/j.envpol.2021.117267
Xue F., Miao J., Zhang X., Luo H., Tan T. (2008). Studies on lipid production by rhodotorula glutinis fermentation using monosodium glutamate wastewater as culture medium. Bioresour. Technol. 99, 5923–5927. doi: 10.1016/j.biortech.2007.04.046
Yeeh Y. (1999). “RHODOTORULA,” in Encyclopedia of food microbiology. Ed. Robinson R. K. (Oxford: Elsevier), 1900–1905. doi: 10.1006/rwfm.1999.1340
Yu Y., Zhao C., Zheng N., Jia H., Yao H. (2019). Interactive effects of soil texture and salinity on nitrous oxide emissions following crop residue amendment. Geoderma 337, 1146–1154. doi: 10.1016/j.geoderma.2018.11.012
Zaman S., Lippman S. I., Zhao X., Broach J. R. (2008). How saccharomyces responds to nutrients. Annu. Rev. Genet. 42, 27–81. doi: 10.1146/annurev.genet.41.110306.130206
Zheng B., Zhu Y., Sardans J., Peñuelas J., Su J. (2018). QMEC: a tool for high-throughput quantitative assessment of microbial functional potential in c, n, p, and s biogeochemical cycling. Sci. China Life Sci. 61, 1451–1462. doi: 10.1007/s11427-018-9364-7
Zhu-Barker X., Cavazos A. R., Ostrom N. E., Horwath W. R., Glass J. B. (2015). The importance of abiotic reactions for nitrous oxide production. Biogeochemistry 126, 251–267. doi: 10.1007/s10533-015-0166-4
Zou Y.-N., Wu Q.-S., Kuča K. (2021). Unravelling the role of arbuscular mycorrhizal fungi in mitigating the oxidative burst of plants under drought stress. Plant Biol. 23, 50–57. doi: 10.1111/plb.13161
Keywords: nitrous oxide, fungi, stable isotop, site preference (SP), sulfidic, salt marsh, sediment, isotopomer
Citation: Lazo-Murphy BM, Larson S, Staines S, Bruck H, McHenry J, Bourbonnais A and Peng X (2022) Nitrous oxide production and isotopomer composition by fungi isolated from salt marsh sediments. Front. Mar. Sci. 9:1098508. doi: 10.3389/fmars.2022.1098508
Received: 15 November 2022; Accepted: 05 December 2022;
Published: 22 December 2022.
Edited by:
Jing Wei, Sun Yat-sen University, ChinaCopyright © 2022 Lazo-Murphy, Larson, Staines, Bruck, McHenry, Bourbonnais and Peng. This is an open-access article distributed under the terms of the Creative Commons Attribution License (CC BY). The use, distribution or reproduction in other forums is permitted, provided the original author(s) and the copyright owner(s) are credited and that the original publication in this journal is cited, in accordance with accepted academic practice. No use, distribution or reproduction is permitted which does not comply with these terms.
*Correspondence: Xuefeng Peng, eHBlbmdAc2VvZS5zYy5lZHU=