- Institute of Marine Science and Technology, Shandong University, Qingdao, China
The increasing presence of pollutants such as engineered nanoparticles (ENPs) and nanoplastics (NPLs) poses potential risks to the marine environment. However, there is a scarcity of information on their joint toxic effects. In this study, we investigated the toxicity of copper oxide nanoparticles (nCuO) combined with polystyrene nanoplastics (PS-NPLs) on the marine microalgae Platymonas helgolandica var. tsingtaoensis. The inhibitory effect of nCuO increased with increasing concentrations, and nCuO ≥ 100 mg/L significantly restrained the growth and chlorophyll content of microalgae. nCuO could be adsorbed by algal cells, which was responsible for membrane lipid oxidation and the disruption of membrane permeability. Simultaneous exposure to nCuO and PS-NPLs had a.n antagonistic effect on the growth inhibition of the microalgae, and nCuO played a leading role in the joint toxicity of nCuO and PS-NPLs. In comparison to nCuO exposure alone, combined exposure decreased the oxidative stress and alleviated the increase in the cell membrane permeability of microalgae. PS-NPLs could heteroaggregate with nCuO, which reduced the interaction between nCuO and microalgae, inducing decreased joint toxicity. Findings of this study will clarify our understanding of the joint toxicity of ENPs and NPLs.
1. Introduction
Nanoplastics (NPLs, size of 1 nm−1 μm) have been regarded as one of the least investigated types of marine debris, but also potentially one of the most dangerous (Koelmans, 2019). NPLs are predominantly formed through fragmentation and degradation of larger plastic debris as a result of mechanical wear, UV irradiation, and biodegradation (Liu et al., 2019; Roager and Sonnenschein, 2019; Enfrin et al., 2020). Only a small fraction of NPLs occurring in the environment are intentionally manufactured as an added component in some products (e.g., nanobeads for personal care products and industrial cleaners) (Alimi et al., 2018; Ivleva, 2021). Although recent works have reported the chemical signatures of NPLs contamination in ocean waters, the abundance of NPLs in the environment still remains largely unknown due to methodological challenges in their identification and quantification (Ter Halle et al., 2017; Materić et al., 2020; Xu et al., 2022). However, given the increasing distribution and concentration of microplastics (< 5 mm) in environmental samples, it can also be assumed that NPLs have a high abundance in the environment.
Microalgae are primary producers at the lowest nutritional level in the marine food chain. Due to their unique properties, NPLs were reported to exhibit high toxicity to microalgae. NPLs can cause physical damage to microalgae, reduce the synthesis of chlorophyll, induce high production of reactive oxygen species (ROS), or form heteroaggregates with algae cells, which significantly inhibit the normal growth of microalgae (Gomes et al., 2020; Nava and Leoni, 2021; Yang et al., 2021). Moreover, NPLs may adsorb large amounts of external toxic compounds because of their high surface-to-volume ratio (Feng et al., 2022; Xi et al., 2022), which contributes to the flux of contaminants in organisms. The joint toxicity of NPLs with heavy metals or organic pollutants to microalgae have been investigated (Cao et al., 2022; Gao et al., 2022; Liu et al., 2022). However, the natural environment is a complicated system, and the fate and behaviors of NPLs in the presence of other contaminants still need further investigation.
Engineered nanoparticles (ENPs) have been widely used in various fields due to their specific physicochemical and biological properties. The large-scale use of nanomaterials makes ENPs inevitably enter the environment during production, transportation, and utilization processes, generating potential environmental risks (Keller and Lazareva, 2014; Lead et al., 2018). It has been reported that the concentrations of ENPs in real water environment reach up to several tens of microgram per litter (Bathi et al., 2022), which can cause the mechanical damage to cell membranes that promotes the internalization of ENPs (Ma et al., 2015; Zhao et al., 2016; Yan et al., 2022). In addition, metal-based ENPs can be partly dissolved to release soluble metal ions, which increase their bioavailability to microalgae (Chen et al., 2012; Yung et al., 2015). ENPs can also adsorb nutrients in the medium and lead to nutrient depletion by microalgae, thus causing indirect toxicity to algae cells (Zhao et al., 2017; Yan et al., 2022). Although both NPLs and ENPs share some similar properties and toxicity mechanisms toward microalgae, NPLs still should be considered a unique class of contaminants, distinct from ENPs (Gigault et al., 2021). Therefore, considering the cooccurrence of NPLs and ENPs in the natural environment, their joint toxicity toward organisms needs to be further studied.
To date, there have been some studies on the joint toxicity of microplastics and ENPs; however, research on the combined effects of NPLs and ENPs is limited, and the relative mechanism of action is unclear. It was found that microplastics could be adsorbed on the algal cells and inhibit the interaction between algae and ENPs (eg. nano-ZnO, nano-Cu) (Gunasekaran et al., 2020; Zhu et al., 2020). In addition, microplastics may also reduce the surface reactivity of ENPs, leading to a reduction in the ROS content generated by microalgae, which results in a decrease in their joint toxicity (Gunasekaran et al., 2020). Natarajan et al. (2022) also found that nano-TiO2 and nano-polystyrene (nano-PS) had antagonistic interactions with the marine algae Chlorella sp. because the heteroaggregation of nano-TiO2 and nano-PS reduced the bioavailability of the particles to the algae. Thiagarajan et al. (2021) obtained similar results that the carboxylated PS microplastics decreased the toxicity of nano-TiO2 in Chlorella sp., but the aminated and plain PS microplastics enhanced the toxicity of nano-TiO2. Huang et al. (2019) investigated the joint toxicity of Ag and PS nanoparticles to two kinds of microalgae, and found that nano-PS showed opposite effects on Chlamydomonas rheinisch (increased) and Dinoflagellates (decreased) in terms of intracellular Ag accumulation. However, the joint toxicity of AgNPs and nano-PS had synergistic interactions with the algae, regardless of the differences in Ag accumulation. Therefore, the complicated joint toxicity mechanism of ENPs and NPLs pollution to microalgae needs to be further explored.
Copper oxide nanoparticles (nCuO), one of the most common ENPs, have been widely used in agriculture, textiles, food preservation, anti-fouling coatings, and water treatment (Roubeau Dumont et al., 2022). By 2025, the global annual production of nCuO is estimated to reach 1600 tons/year (Hou et al., 2017). nCuO can be indirectly released into the aquatic ecosystem through runoff, leaching from agricultural and industrial sites, or directly into the marine environment through the application of antifouling paints (Muller-Karanassos et al., 2021). Although data on nCuO concentrations in the marine environment are scarce (Zhao et al., 2021), once present in the environment, the chance of nCuO contact with organisms and transfer between organisms of different trophic levels increases with time (Wu et al., 2020).
In this study, nCuO was used as a typical representative of ENPs to study its joint toxicity with PS-NPLs (PS, and PS-COOH representing aged PS). Platymonas helgolandica var. tsingtaoensis (P. helgolandica var. tsingtaoensis) was used as the test species because it distributes widely as aquaculture high-quality feed. The cell growth, chlorophyll content, oxidative stress indicators, and cell membrane permeability were evaluated in the presence of nCuO alone or in combination with PS-NPLs. Interactions among nCuO, PS-NPLs, and microalgae were further characterized, and the type of joint action of PS-NPLs and nCuO on microalgae were clarified by both of Abbott and the independent action models. The findings will facilitate more realistic evaluations of the environmental risks of ENPs and NPLs.
2. Materials and methods
2.1. Material characterization
PS-NPLs including PS and PS-COOH were obtained from Jingjie Technology Co., Ltd. (Changchun, China), which have been fully characterized in our previous study (Gao et al., 2022). nCuO (< 50 nm) was purchased from Sigma-Aldrich and could form homogeneous aggregates of several hundred nanometers in f/2 culture medium (Table S1) under the observation of scanning electron microscopy with focused ion beam (FIB-SEM; Crossbeam 550, ZEISS, Germany) (Figure S1). The zeta potential and hydrodynamic diameter of nCuO in the f/2 culture medium were determined by dynamic light scattering (Zetasizer Nano ZS90, Malvern, UK). The amount of Cu(II) released by nCuO in the f/2 culture medium was detected to investigate the contribution of soluble metal ions to the toxicity of nCuO (Supporting Information).
2.2. Microalgae cultivation and growth inhibition experiments
The microalgae were obtained from Guangyu Biotechnology Co. Ltd. (Shanghai, China), and the detailed cultivation method was provided in the Supporting Information. The growth inhibition experiments were first conducted using algal cells in log phase (2 × 105 cells/mL). Single nCuO exposure experiments were carried out with the nCuO concentrations of 10, 50, 100, and 200 mg/L according to the preexperiment (Figure S2). To determine the joint effect of PS-NPLs and nCuO, the concentration of PS-NPLs was fixed at 100 mg/L, and the nCuO concentration was set at 50, 100, and 200 mg/L. The changes in cell concentration were measured every 24 hours, and the percent growth inhibition rate (IR) was determined by IR (%) =100%*(C0-Ci)/C0, where C0 and Ci represent the cell concentration of the control and experimental groups at average, respectively.
2.3. Determination microalgae physiological and biochemical responses
Contents of chlorophyll including chlorophyll a and chlorophyll b were detected by ethanol extraction method. Three oxidative stress indicators were determined in this study, including superoxide dismutase (SOD) activity, reduced glutathione (GSH), and malondialdehyde (MDA) contents (Gao et al., 2022). Besides, the cell membrane permeability of microalgae was also assessed in both single and combined exposure experiments at 96 h by the fluorescein diacetate (FDA) method (He et al., 2019; Yan et al., 2022). To investigate the morphological changes in algal cells, microscopy analysis was carried out by optical microscopy and scanning electron microscopy (SEM) at 96 h of the experiment. The detailed measurement procedures of the above physiological and biochemical responses were provided in the Supporting Information.
2.4. Data analysis
All experiments were set up with three replicates, and the results were expressed as the mean ± standard deviation (SD). One-way ANOVA was used to test the significant differences between the control and treatment groups, and P < 0.05 indicated a statistically significant difference. The independent action (IA) model and Abbott model were used to evaluate the results to clarify the type of combined action of PS-NPLs and nCuO (Gao et al., 2022). Detail on the two models was supplied in the Supporting Information.
3. Results and discussion
3.1. Growth inhibition
The growth inhibitory effect of nCuO on the microalgae increased with increasing exposure concentration and time (Figure S3). At 96 h, no obvious difference was observed in cell concentration between the nCuO ≤ 50 mg/L groups and the control group, while the cell concentrations of the 100 and 200 mg/L nCuO groups were significantly reduced compared with the control group, decreasing by 21.43% and 29.56% (Figure 1), respectively. Previous studies revealed that the toxic effects of metal-based nanoparticles could be attributed to the release of soluble metal ions (Chen et al., 2012; Yung et al., 2015; Huang et al., 2019). Therefore, the concentration of Cu(II) released from nCuO in f/2 medium was detected at 24 h and 96 h (Figure S4). The highest Cu(II) concentration was 0.21 ± 0.032 mg/L released from 200 mg/L nCuO at 96 h. Our previous study reported that the calculated growth inhibition rate was lower than 7% on P. helgolandica var. tsingtaoensis with Cu(II) below 0.21 mg/L (Gao et al., 2022), indicating that a small part of the toxicity of nCuO to the microalgae was due to the released Cu(II). Zhao et al. (2016) found that nCuO inhibited the growth of Chlorella vulgaris, and the median effect concentration (EC50) value at 72 h was 45.7 mg/L. However, in our study, the EC50 value of nCuO on P. helgolandica var. tsingtaoensis was not obtained even when the concentrations reached 500 mg/L (Figure S2). High salinity could enhance the homogeneous aggregation of nanoparticles, which reduced the interaction between algal cells and nanoparticles (Callegaro et al., 2015; Conway et al., 2015). The mean hydrodynamic diameter for nCuO was 862.53 ± 59.90 nm in f/2 media, indicating the high aggregation of nCuO. In addition, nCuO underwent rapid aggregation and sedimentation after being added to the algal suspension (Figure S5); therefore, the strong aggregation of nCuO resulted in their relatively weakened toxicity to algal cells.
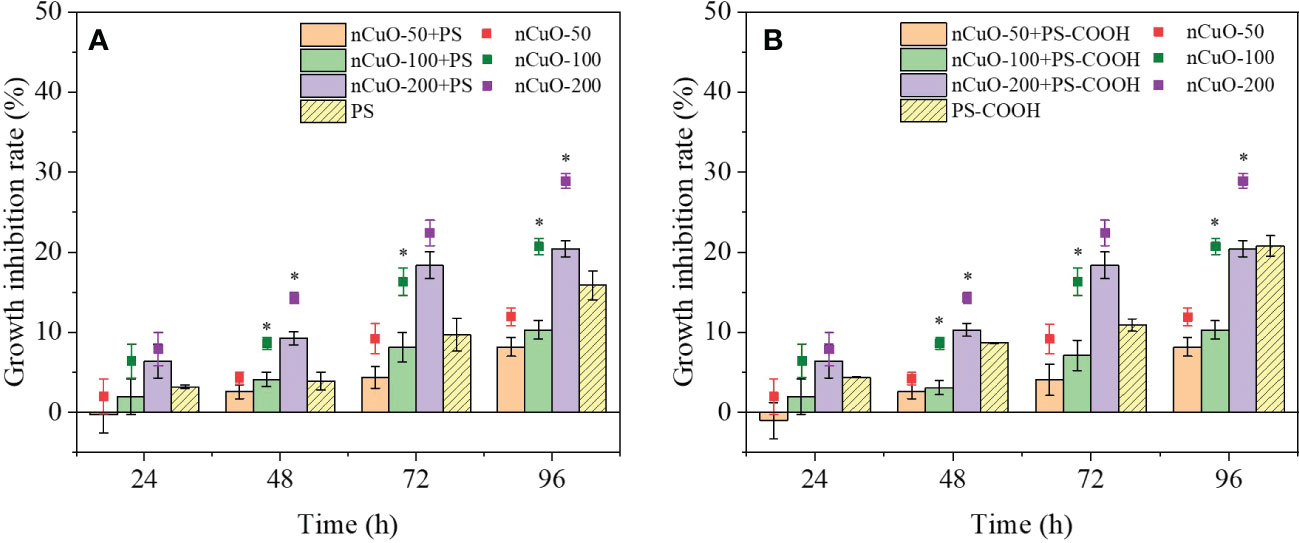
Figure 1 Combined effects of different concentrations of nCuO with 100mg/L PS (A) and PS-COOH (B) on the growth inhibition of P. helgolandica var. tsingtaoensis. Error bars represent standard deviations (n = 3). Asterisks indicate significant differences between single nCuO groups and the combined groups (P < 0.05).
The results of the combined exposure to PS-NPLs and nCuO on the growth inhibition of microalgae are displayed in Figure 1. It was obvious that the growth inhibition rates of the combined exposure groups were positively correlated with the nCuO concentrations, indicating that the joint toxicity was mainly influenced by nCuO. The growth inhibition rates increased with increasing exposure time, and the highest values were 20.45% in the presence of 200 mg/L nCuO combined with PS-NPLs at 96 h. During the whole exposure period, the addition of PS-NPLs reduced the growth inhibition rates of single nCuO exposure. For example, at 96 h, compared with the single nCuO exposure results, the growth inhibition rates of 50, 100, and 200 mg/L nCuO combined with PS decreased by 3.70%, 10.32%, and 8.40%, respectively. Meanwhile, the antagonistic effects between nCuO and PS-NPLs were obtained by the IA and Abbott models (Table S2), which were in accord with the above results. These results may be attributed to the reduction in the nCuO effective concentration induced by the heterogeneous aggregation between PS-NPLs and nCuO (Conway et al., 2015), which will be further discussed in Section 3.5.
3.2. Effect on chlorophyll content
Figure S3B illustrates the chlorophyll content in P. helgolandica var. tsingtaoensis affected by nCuO. The chlorophyll content decreased at all tested concentrations during the experiment, and showed a decreasing trend with increasing nCuO concentration. At 24 h, compared with the control group, the chlorophyll a content in the four experimental groups (10, 50, 100 and 200 mg/L nCuO) significantly decreased by 24.53%, 27.89%, 30.87% and 29.75%, respectively. Chlorophyll b content showed a similar trend. At 48 h and 72 h, the chlorophyll content of the nCuO groups recovered with increasing exposure time and showed no significant difference from that of the control group. At 96 h, nCuO > 100 mg/L significantly inhibited chlorophyll synthesis, and the chlorophyll content in the 100 and 200 mg/L nCuO groups decreased by 19.11% and 31.81%, respectively. The surface coverage of nCuO on P. helgolandica var. tsingtaoensis could impede the access of algal cells to external light, oxygen and other substances (Zhao et al., 2016); alternatively, the turbidity of nanoparticles in the medium could produce a shading effect to inhibit the growth of microalgae (Chen et al., 2018).
The chlorophyll content in the 100 mg/L PS-NPLs treatment groups was higher than that in the control group at 96 h (Figure S6). However, compared with the control, the chlorophyll a content exposure to the combined nCuO (50, 100 and 200 mg/L) with PS and PS-COOH decreased by 9.86%, 23.46%, 20.62% and 5.87%, 21.39%, 20.66%, respectively (Figure 2). The chlorophyll b content showed a similar trend. No statistically significant difference was observed in chlorophyll content between the combined exposure groups and the single nCuO groups, which further indicated that the inhibition effect of combined nCuO and PS-NPLs on the chlorophyll content was mostly affected by nCuO.
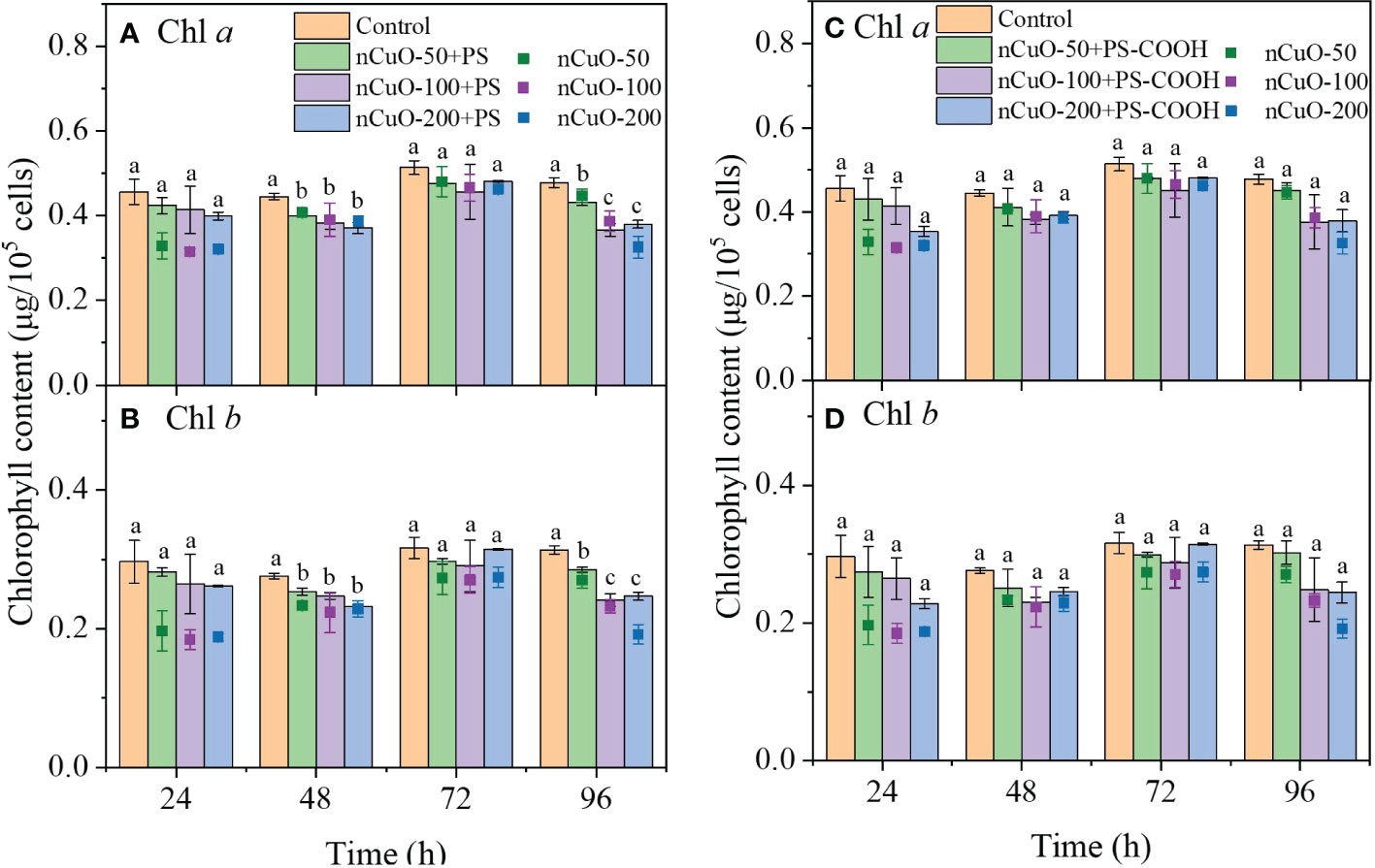
Figure 2 Combined effects of nCuO with 100mg/L PS (A, B) and PS-COOH (C, D) on the chlorophyll content of P. helgolandica var. tsingtaoensis. Error bars represent standard deviations (n = 3). Different letters indicate significant differences among the treatment groups (P < 0.05).
3.3. Effect on oxidative stress
Figure S7A shows that the SOD activity in all nCuO groups was higher than that in the control group, and the SOD activity increased with increasing nCuO concentration. This result indicated that nCuO induced oxidative stress in the microalgae, and higher exposure concentrations led to increased oxidative stress. Che et al. (2018) also found that nCuO could increase the ROS content and SOD activity of Chlorella vulgaris, and these two indicators were positively correlated. In our study, the difference in SOD activity between the nCuO groups and the control group gradually decreased as the exposure time increased, suggesting that the ROS inside the algal cells decreased with prolonged exposure time due to the self-adaptation and antioxidant defense of algae (Sabatini et al., 2009). Unlike SOD activity, nCuO at all concentrations resulted in a much lower GSH content compared with the control group at 24 h, and the higher the exposure concentration of nCuO was, the lower the GSH content of algal cells (Figure S7B). This might be attributed to the inhibition of GSH synthesis and the high consumption of GSH in algal cells. It has been reported that nCuO could cause dysfunction of intracellular biomolecules, alteration of antioxidant enzyme activity and depletion of GSH content (Naz et al., 2020). After 24 h, the GSH content in the 10 mg/L nCuO groups had no significant difference from that in the control group. Meanwhile, GSH contents in the nCuO > 10 mg/L groups increased with the exposure time, and were not significantly different from those in the 10 mg/L nCuO groups at 96 h, indicating the recovery of GSH synthesis capacity and a decrease in GSH consumption due to the reduction in ROS in algal cells (Melegari et al., 2013).
During the exposure period, the MDA content was significantly higher in the nCuO groups than that in the control and showed a positive correlation with the nCuO exposure concentrations (Figure S7C). At 24 h, the MDA content of the four experimental nCuO groups (10, 50, 100, and 200 mg/L) was 1.17, 1.39, 1.48, and 1.79 times higher than that of the control, indicating that nCuO exposure caused lipid peroxidation to P. helgolandica var. tsingtaoensis. This was one of the reasons for the growth inhibition effect of nCuO. After 24 h, the MDA content of all nCuO groups did not change significantly with increasing exposure time. This suggested that the oxidative damage produced by nCuO decreased with time, which was related to the scavenging effect of SOD on ROS and the breaking of lipid peroxidation-related chains by GSH (Ribeiro et al., 2017; Estrela et al., 2021).
The combined effects of nCuO and PS-NPLs on the three oxidative stress indicators are presented in Figure 3. During the whole exposure time, the SOD activity of microalgae in the combined exposure groups significantly increased compared with that in the control group (Figs. 3A-B). SOD activity was positively correlated with the concentration of nCuO in the combined exposure groups, indicating that algae cells in the combined exposure groups were also subjected to oxidative stress. Compared with nCuO exposure, the presence of PS-NPLs reduced the SOD activity of microalgae. Meanwhile, as shown in Figures 3C, D, the GSH content of algae cells in the combined exposure groups was always higher than that in the nCuO exposure groups. These results indicated that the oxidative stress in the combined exposure groups was weaker than that in the single nCuO exposure groups.
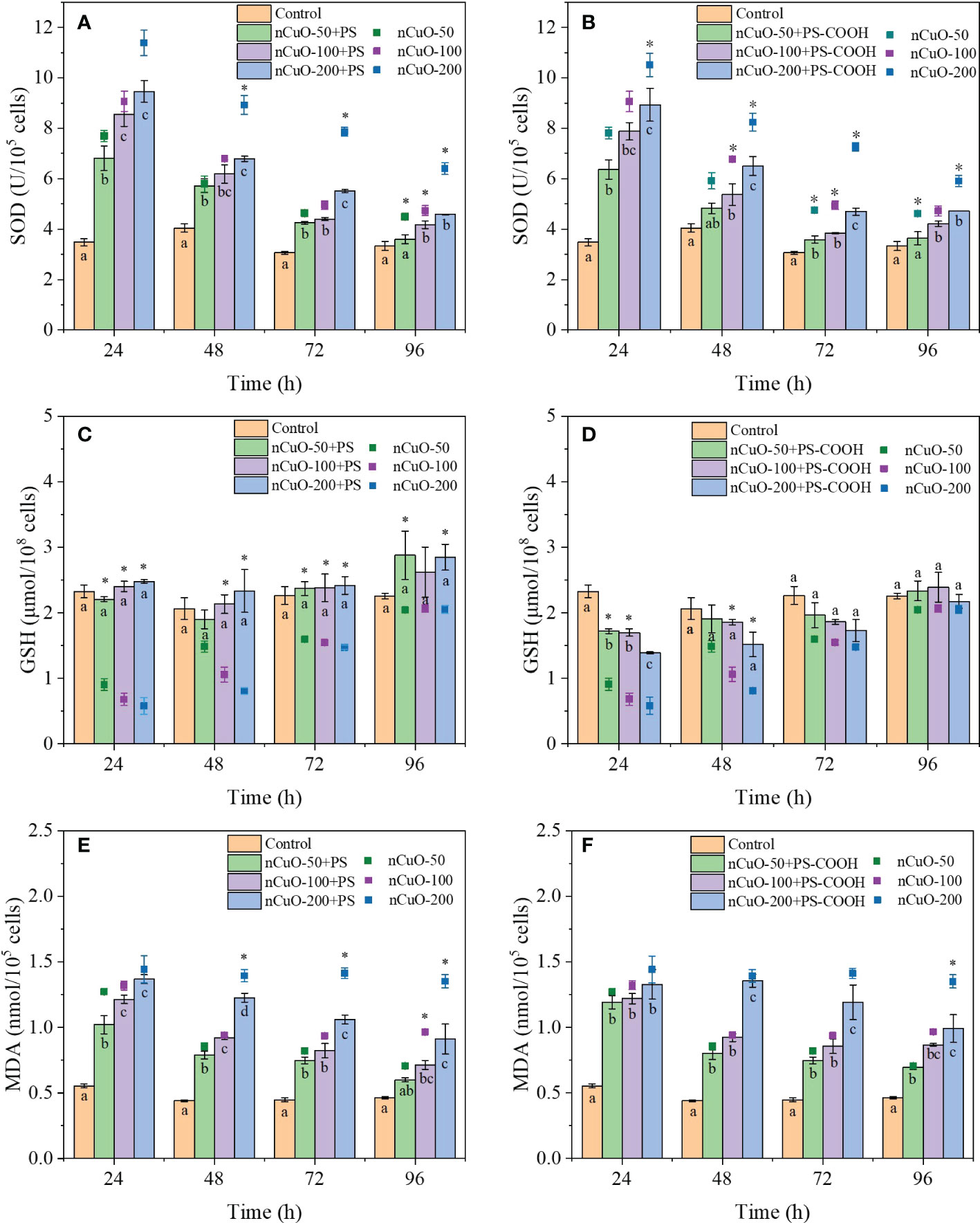
Figure 3 Combined effects of different concentrations of nCuO with 100mg/L PS and PS-COOH on SOD activity (A, B), GSH content (C, D) and MDA content (E, F) in P. helgolandica var. tsingtaoensis. Error bars represent standard deviations (n = 3). Different letters indicate significant differences among the treatment groups (P < 0.05). Asterisks indicate significant differences between single nCuO groups and the combined groups (P < 0.05).
Figures 3E, F demonstrate the effect of the combined PS-NPLs and nCuO on the MDA content in the microalgae. The MDA contents in the combined exposure groups were higher than those in the control group, and the MDA content was positively correlated with the nCuO concentration. However, MDA contents in the combined exposure groups were consistently lower than those in single nCuO exposure groups throughout the entire exposure period. MDA content in microalgae in the 200 mg/L nCuO combined with PS and PS-COOH groups was 34.91% and 28.12% lower than that in the nCuO group at 96 h. This indicated that PS-NPLs could reduce the membrane oxidative damage of algal cells caused by nCuO, which was also the reason for the reduced combined toxicity. In addition, as shown in Table S3, the combined effect of nCuO and PS-NPLs was antagonistic, which was evaluated by the Abbott model based on the three oxidative stress indicators data (oxidative stress indictors of microalgae exposed to PS-NPLs are shown in Figure S8).
3.4. Effect on cell membrane permeability
To further investigate the degree of membrane damage when exposed to nCuO alone or in combination with PS-NPLs, the cell membrane permeability of the microalgae was evaluated. There was a negative correlation between the fluorescence intensity and the nCuO concentrations (Figure S9A), indicating that the cell membrane permeability in all nCuO groups significantly increased compared with that in the control group. The abrasiveness of particles can cause physical damage when they contact with algal cells (Pakrashi et al., 2013). Besides, the attachment of ENPs on the cell can disturb the electron/ion transport chains of cell membrane (Lei et al., 2016), resulting in an increase in membrane permeability. In addition, ENPs also induced lipid peroxidation of the cell membrane, leading to an increase in permeability. The dose dependent increase in MDA content in the nCuO groups (Figure S7C) demonstrated that nCuO at higher concentrations induced stronger cell membrane lipid peroxidation, thus leading to an increase in cell permeability. He et al. (2019) found similar results that the cell membrane permeability of Chlorella pyrenoidosa increased with increasing nThO2 concentrations, which was attributed to increasing oxidative stress.
Figure S9B presents the combined effect of nCuO and PS-NPLs on the cell membrane permeability of P. helgolandica var. tsingtaoensis. PS-NPLs significantly alleviated the increase in cell membrane permeability caused by nCuO, which was in accord with the reduction in cell membrane oxidative damage. This result might be attributed to the reduced interaction between nCuO and algal cells in the combined exposure groups. Similarly, Zhao et al. (2018) observed that Al2O3 nanoparticles could heterogeneously aggregate with graphene oxide (GO), leading to reduced physical contact between GO and algal cells, thus significantly alleviating the cell membrane damage of GO on Chlorella pyrenoidosa.
3.5. Interactions among nCuO, PS-NPLs, and microalgae
As the cell growth and chlorophyll content in P. helgolandica var. tsingtaoensiss was considerably reduced in the nCuO ≥ 100 mg/L groups at 96 h, optical microscopy observation was employed to examine the interaction between nCuO and algal cells in the 100 and 200 mg/L nCuO groups. As shown in Figure S10, large heterogeneous aggregates composed of algal cells and nCuO were observed. SEM images also showed that nCuO was mainly in an agglomeration state and could be adsorbed on the surface of algal cells (Figures 4A, B). This could be explained by the electrostatic attraction between the negatively surface charged P. helgolandica var. tsingtaoensis (zeta potential = -7.75 ± 1.66 mV) and the positively surface charged nCuO (zeta potential = 3.66 ± 0.54 mV). Various ENPs have been found to form heteroaggregates with algal cells (Ma et al., 2015; Zhao et al., 2016; He et al., 2019); as a result of cell membrane damage, thus inhibiting algae growth. Meanwhile, there were a large number of deciduous flagella surrounding the algal cells (Figure S10), indicating that nCuO could reduce the cell activity by disrupting the integrity of P. helgolandica var. tsingtaoensis.
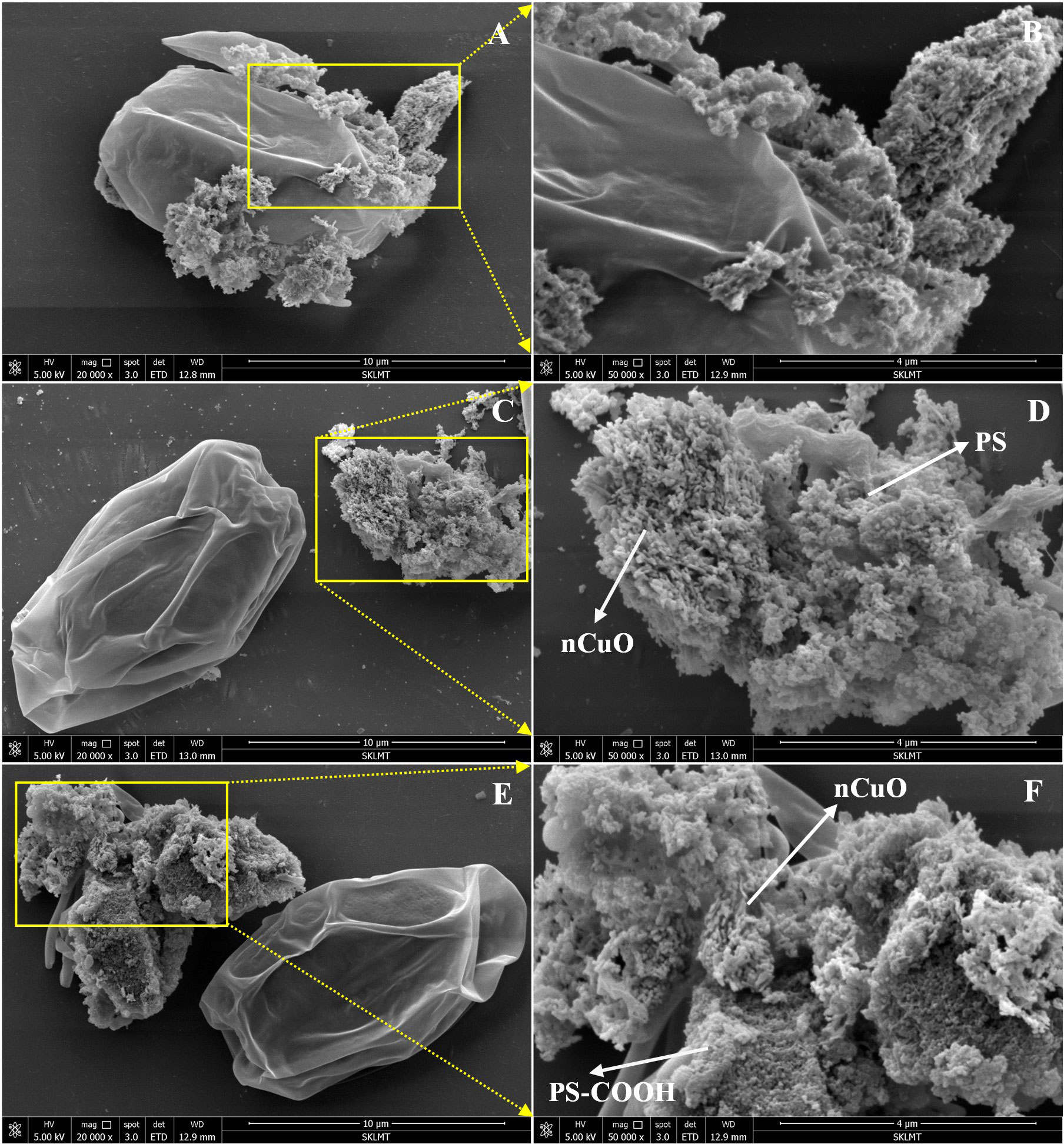
Figure 4 SEM images of P. helgolandica var. tsingtaoensis exposed to nCuO (A, B), nCuO + PS (C, D), and nCuO + PS-COOH (E, F).
As the combined effects of PS-NPLs and nCuO on the growth and oxidative stress indicators were antagonistic according to Sections 3.1 and 3.3, we hypothesized that PS-NPLs might aggregate with nCuO and thus reduce the bioavailability of nCuO on the microalgae. Indeed, both types of PS-NPLs could interact with nCuO to form heteoaggregates (Figure S11). Natarajan et al. (2022) observed a similar heteroaggregation of nano-TiO2 and PS-NPLs, and the toxic effects of nano-TiO2 to marine algae Chlorella sp. was significantly reduced in the presence of PS-NPLs. In our study, both PS and PS-COOH were negatively charged with zeta potentials of -8.24 ± 1.5 mV and -15.15 ± 1.06 mV, respectively. Therefore, PS-NPLs and P. helgolandica var. tsingtaoensis might compete for the interaction with nCuO, and heteroaggregation between PS-NPLs and nCuO would reduce the interaction between nCuO and microalgae. As shown in Figures 4C-F, PS-NPLs in the combined groups formed heterogeneous aggregates with nCuO, resulting in reduced surface interactions between nCuO and algal cells. Therefore, the simultaneous exposure of nCuO and PS-NPLs showed antagonistic effects on the toxicity of P. helgolandica var. tsingtaoensis.
4. Conclusions
This study evaluated the toxic effects of nCuO with and without PS-NPLs to P. helgolandica var. tsingtaoensis, specifically focusing on the growth, chlorophyll, oxidative stress, and membrane permeability of the microalgae. The heteroaggregation of nCuO and P. helgolandica var. tsingtaoensis induced severe membrane lipid peroxidation, decreased the chlorophyll content, and increased the membrane permeability of microalgae, which were the toxic mechanisms of nCuO toward microalgae. In combined exposure experiments, nCuO and PS-NPLs had an antagonistic effect on P. helgolandica var. tsingtaoensis. The addition of PS-NPs alleviated the growth inhibition, oxidative stress, and cell membrane permeability caused by nCuO to microalgae. This could be attributed to the heterogeneous aggregation of PS-NPLs with nCuO, which inhibited the interactions between nCuO and microalgae. This study provides a better understanding of the joint toxicity of nanoplastics and nanoparticles to microalgae.
Data availability statement
The raw data supporting the conclusions of this article will be made available by the authors, without undue reservation.
Author contributions
F-FL: Conceptualization, Methodology, Validation, Formal analysis, Resources, Writing - original draft, Writing - review and editing, Supervision, Project administration, Funding acquisition. Z-YG: Conceptualization, Methodology, Validation, Investigation, Formal analysis, Writing - original draft. W-CC: Investigation, Writing - original draft. S-CW: Methodology, Writing - review and editing. All authors contributed to the article and approved the submitted version.
Funding
This work was financially supported by the National Natural Science Foundation of China (U1906224), the Natural Science Foundation of Shandong Province (ZR2020MD114), Shandong Provincial Key Research and Development Program (2020CXGC011406), Young Scholars Program of Shandong University, and Shandong University Interdisciplinary Research and Innovation Team of Young Scholars (2020QNQT20).
Conflict of interest
The authors declare that the research was conducted in the absence of any commercial or financial relationships that could be construed as a potential conflict of interest.
Publisher’s note
All claims expressed in this article are solely those of the authors and do not necessarily represent those of their affiliated organizations, or those of the publisher, the editors and the reviewers. Any product that may be evaluated in this article, or claim that may be made by its manufacturer, is not guaranteed or endorsed by the publisher.
Supplementary material
The Supplementary Material for this article can be found online at: https://www.frontiersin.org/articles/10.3389/fmars.2022.1089282/full#supplementary-material
References
Alimi O. S., Farner Budarz J., Hernandez L. M., Tufenkji N. (2018). Microplastics and nanoplastics in aquatic environments: Aggregation, deposition, and enhanced contaminant transport. Environ. Sci. Technol. 52, 1704–1724. doi: 10.1021/acs.est.7b05559
Bathi J. R., Wright L., Khan E. (2022). Critical review of engineered nanoparticles: Environmental concentrations and toxicity. Curr. pollut. Rep. doi: 10.1007/s40726-022-00237-4
Callegaro S., Minetto D., Pojana G., Bilanicová D., Libralato G., Volpi Ghirardini A., et al. (2015). Effects of alginate on stability and ecotoxicity of nano-TiO2 in artificial seawater. Ecotoxicol. Environ. Saf. 117, 107–114. doi: 10.1016/j.ecoenv.2015.03.030
Cao J., Liao Y., Yang W., Jiang X., Li M. (2022). Enhanced microalgal toxicity due to polystyrene nanoplastics and cadmium co-exposure: From the perspective of physiological and metabolomic profiles. J. Hazard. Mater. 427, 127937. doi: 10.1016/j.jhazmat.2021.127937
Che X., Ding R., Li Y., Zhang Z., Gao H., Wang W. (2018). Mechanism of long-term toxicity of CuO NPs to microalgae. Nanotoxicology 12, 923–939. doi: 10.1080/17435390.2018.1498928
Chen P., Powell B. A., Mortimer M., Ke P. C. (2012). Adaptive interactions between zinc oxide nanoparticles and chlorella sp. Environ. Sci. Technol. 46, 12178–12185. doi: 10.1021/es303303g
Chen X., Zhang C., Tan L., Wang J. (2018). Toxicity of Co nanoparticles on three species of marine microalgae. Environ. pollut. 236, 454–461. doi: 10.1016/j.envpol.2018.01.081
Conway J. R., Adeleye A. S., Gardea-Torresdey J., Keller A. A. (2015). Aggregation, dissolution, and transformation of copper nanoparticles in natural waters. Environ. Sci. Technol. 49, 2749–2756. doi: 10.1021/es504918q
Enfrin M., Lee J., Gibert Y., Basheer F., Kong L., Dumée L. F. (2020). Release of hazardous nanoplastic contaminants due to microplastics fragmentation under shear stress forces. J. Hazard. Mater. 384, 121393. doi: 10.1016/j.jhazmat.2019.121393
Estrela F. N., Batista Guimarães A. T., Silva F. G., Marinho da Luz T., Silva A. M., Pereira P. S., et al. (2021). Effects of polystyrene nanoplastics on ctenopharyngodon idella (grass carp) after individual and combined exposure with zinc oxide nanoparticles. J. Hazard. Mater. 403, 123879. doi: 10.1016/j.jhazmat.2020.123879
Feng H., Liu Y., Xu Y., Li S., Liu X., Dai Y., et al. (2022). Benzo[a]pyrene and heavy metal ion adsorption on nanoplastics regulated by humic acid: Cooperation/competition mechanisms revealed by molecular dynamics simulations. J. Hazard. Mater. 424, 127431. doi: 10.1016/j.jhazmat.2021.127431
Gao Z.-y., Wang S.-c., Zhang Y.-x., Liu F.-f. (2022). Single and combined toxicity of polystyrene nanoplastics and copper on platymonas helgolandica var. tsingtaoensis: Perspectives from growth inhibition, chlorophyll content and oxidative stress. Sci. Total Environ. 829, 154571. doi: 10.1016/j.scitotenv.2022.154571
Gigault J., El Hadri H., Nguyen B., Grassl B., Rowenczyk L., Tufenkji N., et al. (2021). Nanoplastics are neither microplastics nor engineered nanoparticles. Nat. Nanotechnol. 16, 501–507. doi: 10.1038/s41565-021-00886-4
Gomes T., Almeida A. C., Georgantzopoulou A. (2020). Characterization of cell responses in rhodomonas baltica exposed to PMMA nanoplastics. Sci. Total Environ. 726, 138547. doi: 10.1016/j.scitotenv.2020.138547
Gunasekaran D., Chandrasekaran N., Jenkins D., Mukherjee A. (2020). Plain polystyrene microplastics reduce the toxic effects of ZnO particles on marine microalgae dunaliella salina. J. Environ. Chem. Eng. 8, 104250. doi: 10.1016/j.jece.2020.104250
He X., Xie C., Ma Y., Wang L., He X., Shi W., et al. (2019). Size-dependent toxicity of ThO2 nanoparticles to green algae chlorella pyrenoidosa. Aquat. Toxicol. 209, 113–120. doi: 10.1016/j.aquatox.2019.02.003
Hou J., Wang X., Hayat T., Wang X. (2017). Ecotoxicological effects and mechanism of CuO nanoparticles to individual organisms. Environ. pollut. 221, 209–217. doi: 10.1016/j.envpol.2016.11.066
Huang B., Wei Z.-B., Yang L.-Y., Pan K., Miao A.-J. (2019). Combined toxicity of silver nanoparticles with hematite or plastic nanoparticles toward two freshwater algae. Environ. Sci. Technol. 53, 3871–3879. doi: 10.1021/acs.est.8b07001
Ivleva N. P. (2021). Chemical analysis of microplastics and nanoplastics: Challenges, advanced methods, and perspectives. Chem. Rev. 121, 11886–11936. doi: 10.1021/acs.chemrev.1c00178
Keller A. A., Lazareva A. (2014). Predicted releases of engineered nanomaterials: From global to regional to local. Environ. Sci. Technol. Lett. 1, 65–70. doi: 10.1021/ez400106t
Koelmans A. A. (2019). Proxies for nanoplastic. Nat. Nanotechnol. 14, 307–308. doi: 10.1038/s41565-019-0416-z
Lead J. R., Batley G. E., Alvarez P. J. J., Croteau M.-N., Handy R. D., McLaughlin M. J., et al. (2018). Nanomaterials in the environment: Behavior, fate, bioavailability, and effects–an updated review. Environ. Toxicol. Chem. 37, 2029–2063. doi: 10.1002/etc.4147
Lei C., Zhang L., Yang K., Zhu L., Lin D. (2016). Toxicity of iron-based nanoparticles to green algae: Effects of particle size, crystal phase, oxidation state and environmental aging. Environ. pollut. 218, 505–512. doi: 10.1016/j.envpol.2016.07.030
Liu X., Ma J., Guo S., Shi Q., Tang J. (2022). The combined effects of nanoplastics and dibutyl phthalate on streptomyces coelicolor M145. Sci. Total Environ. 826, 154151. doi: 10.1016/j.scitotenv.2022.154151
Liu P., Qian L., Wang H., Zhan X., Lu K., Gu C., et al. (2019). New insights into the aging behavior of microplastics accelerated by advanced oxidation processes. Environ. Sci. Technol. 53, 3579–3588. doi: 10.1021/acs.est.9b00493
Materić D., Kasper-Giebl A., Kau D., Anten M., Greilinger M., Ludewig E., et al. (2020). Micro- and nanoplastics in alpine snow: A new method for chemical identification and (semi)quantification in the nanogram range. Environ. Sci. Technol. 54, 2353–2359. doi: 10.1021/acs.est.9b07540
Ma S., Zhou K., Yang K., Lin D. (2015). Heteroagglomeration of oxide nanoparticles with algal cells: Effects of particle type, ionic strength and pH. Environ. Sci. Technol. 49, 932–939. doi: 10.1021/es504730k
Melegari S. P., Perreault F., Costa R. H. R., Popovic R., Matias W. G. (2013). Evaluation of toxicity and oxidative stress induced by copper oxide nanoparticles in the green alga chlamydomonas reinhardtii. Aquat. Toxicol. 142-143, 431–440. doi: 10.1016/j.aquatox.2013.09.015
Muller-Karanassos C., Arundel W., Lindeque P. K., Vance T., Turner A., Cole M. (2021). Environmental concentrations of antifouling paint particles are toxic to sediment-dwelling invertebrates. Environ. pollut. 268, 115754. doi: 10.1016/j.envpol.2020.115754
Natarajan L., Jenifer M. A., Chandrasekaran N., Suraishkumar G. K., Mukherjee A. (2022). Polystyrene nanoplastics diminish the toxic effects of nano-TiO2 in marine algae chlorella sp. Environ. Res. 204, 112400. doi: 10.1016/j.envres.2021.112400
Nava V., Leoni B. (2021). A critical review of interactions between microplastics, microalgae and aquatic ecosystem function. Water Res. 188, 116476. doi: 10.1016/j.watres.2020.116476
Naz S., Gul A., Zia M. (2020). Toxicity of copper oxide nanoparticles: a review study. IET Nanobiotechnol. 14, 1–13. doi: 10.1049/iet-nbt.2019.0176
Pakrashi S., Dalai S., T.C P., Trivedi S., Myneni R., Raichur A. M., et al. (2013). Cytotoxicity of aluminium oxide nanoparticles towards fresh water algal isolate at low exposure concentrations. Aquat. Toxicol. 132-133, 34–45. doi: 10.1016/j.aquatox.2013.01.018
Ribeiro F., Garcia A. R., Pereira B. P., Fonseca M., Mestre N. C., Fonseca T. G., et al. (2017). Microplastics effects in scrobicularia plana. Mar. pollut. Bull. 122, 379–391. doi: 10.1016/j.marpolbul.2017.06.078
Roager L., Sonnenschein E. C. (2019). Bacterial candidates for colonization and degradation of marine plastic debris. Environ. Sci. Technol. 53, 11636–11643. doi: 10.1021/acs.est.9b02212
Roubeau Dumont E., Elger A., Azéma C., Castillo Michel H., Surble S., Larue C. (2022). Cutting-edge spectroscopy techniques highlight toxicity mechanisms of copper oxide nanoparticles in the aquatic plant myriophyllum spicatum. Sci. Total Environ. 803, 150001. doi: 10.1016/j.scitotenv.2021.150001
Sabatini S. E., Juárez Á. B., Eppis M. R., Bianchi L., Luquet C. M., Ríos de Molina M. (2009). Oxidative stress and antioxidant defenses in two green microalgae exposed to copper. Ecotoxicol. Environ. Saf. 72, 1200–1206. doi: 10.1016/j.ecoenv.2009.01.003
Ter Halle A., Jeanneau L., Martignac M., Jardé E., Pedrono B., Brach L., et al. (2017). Nanoplastic in the north Atlantic subtropical gyre. Environ. Sci. Technol. 51, 13689–13697. doi: 10.1021/acs.est.7b03667
Thiagarajan V., Alex S. A., Seenivasan R., Chandrasekaran N., Mukherjee A. (2021). Toxicity evaluation of nano-TiO2 in the presence of functionalized microplastics at two trophic levels: Algae and crustaceans. Sci. Total Environ. 784, 147262. doi: 10.1016/j.scitotenv.2021.147262
Wu F., Harper B. J., Crandon L. E., Harper S. L. (2020). Assessment of Cu and CuO nanoparticle ecological responses using laboratory small-scale microcosms. Environ. Sci.: Nano 7, 105–115. doi: 10.1039/C9EN01026B
Xi X., Ding D., Zhou H., Baihetiyaer B., Sun H., Cai Y., et al. (2022). Interactions of pristine and aged nanoplastics with heavy metals: Enhanced adsorption and transport in saturated porous media. J. Hazard. Mater. 437, 129311. doi: 10.1016/j.jhazmat.2022.129311
Xu Y., Ou Q., Jiao M., Liu G., van der Hoek J. P. (2022). Identification and quantification of nanoplastics in surface water and groundwater by pyrolysis gas chromatography–mass spectrometry. Environ. Sci. Technol. 56, 4988–4997. doi: 10.1021/acs.est.1c07377
Yang W., Gao P., Li H., Huang J., Zhang Y., Ding H., et al. (2021). Mechanism of the inhibition and detoxification effects of the interaction between nanoplastics and microalgae chlorella pyrenoidosa. Sci. Total Environ. 783, 146919. doi: 10.1016/j.scitotenv.2021.146919
Yan Z., Yang X., Lynch I., Cui F. (2022). Comparative evaluation of the mechanisms of toxicity of graphene oxide and graphene oxide quantum dots to blue-green algae microcystis aeruginosa in the aquatic environment. J. Hazard. Mater. 425, 127898. doi: 10.1016/j.jhazmat.2021.127898
Yung M. M. N., Wong S. W. Y., Kwok K. W. H., Liu F. Z., Leung Y. H., Chan W. T., et al. (2015). Salinity-dependent toxicities of zinc oxide nanoparticles to the marine diatom thalassiosira pseudonana. Aquat. Toxicol. 165, 31–40. doi: 10.1016/j.aquatox.2015.05.015
Zhao J., Cao X., Liu X., Wang Z., Zhang C., White J. C., et al. (2016). Interactions of CuO nanoparticles with the algae chlorella pyrenoidosa: adhesion, uptake, and toxicity. Nanotoxicology 10, 1297–1305. doi: 10.1080/17435390.2016.1206149
Zhao J., Cao X., Wang Z., Dai Y., Xing B. (2017). Mechanistic understanding toward the toxicity of graphene-family materials to freshwater algae. Water Res. 111, 18–27. doi: 10.1016/j.watres.2016.12.037
Zhao J., Dai Y., Wang Z., Ren W., Wei Y., Cao X., et al. (2018). Toxicity of GO to freshwater algae in the presence of Al2O3 particles with different morphologies: Importance of heteroaggregation. Environ. Sci. Technol. 52, 13448–13456. doi: 10.1021/acs.est.8b00815
Zhao J., Lin M., Wang Z., Cao X., Xing B. (2021). Engineered nanomaterials in the environment: Are they safe? Crit. Rev. Environ. Sci. Technol. 51, 1443–1478. doi: 10.1080/10643389.2020.1764279
Keywords: engineered nanoparticles, nanoplastics, antagonistic, aggregation, microalgae
Citation: Liu F-f, Gao Z-y, Chu W-c and Wang S-c (2022) Polystyrene nanoplastics alleviate the toxicity of CuO nanoparticles to the marine algae Platymonas helgolandica var. tsingtaoensis. Front. Mar. Sci. 9:1089282. doi: 10.3389/fmars.2022.1089282
Received: 04 November 2022; Accepted: 02 December 2022;
Published: 16 December 2022.
Edited by:
Xiaoshan Zhu, Hainan University, ChinaReviewed by:
Chuanxin Ma, Guangdong University of Technology, ChinaZhuanxi Luo, Huaqiao University, China
Copyright © 2022 Liu, Gao, Chu and Wang. This is an open-access article distributed under the terms of the Creative Commons Attribution License (CC BY). The use, distribution or reproduction in other forums is permitted, provided the original author(s) and the copyright owner(s) are credited and that the original publication in this journal is cited, in accordance with accepted academic practice. No use, distribution or reproduction is permitted which does not comply with these terms.
*Correspondence: Fei-fei Liu, bGl1ZmVpZmVpQHNkdS5lZHUuY24=