- 1GEOMAR, Helmholtz Centre for Ocean Research, Kiel, Germany
- 2School of Engineering, Federal Polytechnic of Oil and Gas, Bonny, Nigeria
- 3Faculty of Engineering, Computing and Science, Swinburne University of Technology Sarawak Campus, Kuching, Sarawak, Malaysia
- 4Centre for Coastal Biogeochemistry, Faculty of Science and Engineering, Southern Cross University, Lismore, NSW, Australia
- 5Institute for Biodiversity and Environmental Research (IBER), BE 1410 Universiti Brunei Darussalam, Jerudong, Brunei
- 6State Key Laboratory of Estuarine and Coastal Research, East China Normal University, Shanghai, China
Tropical peat swamps are essential ecosystems, which provide numerous services, and also serve as a rich source of dissolved organic carbon (DOC), hydrogen ions and trace elements to peat draining rivers. However, not much is known about trace element export from tropical peat swamps. We investigated trace element dynamics in rivers and estuaries draining tropical peat swamps on Borneo, and examined the influence of estuarine processes as well as dissolved organic carbon (DOC) on the distribution and concentration of trace elements. Our results indicate acidic conditions (pH = 3.3) and high DOC concentration (3500 µmol L−1) at salinities<1. We observed an initial release of trace elements at low salinity (0.05<S< 0.5), followed by scavenging to particles at intermediate salinities (0.5<S<10) due to an increasing ionic strength and pH. Peak concentrations (µmol kg −1) of Al (24.9), Si (96.2), Mn (4.9), Cu (0.035) and Ni (0.047) were observed during the dry season (July), and Fe concentrations (43.2) were highest during the wet season (December). We used the NICA-Donnan model to investigate the combined impact of DOC and pH on the formation of solid iron hydroxide (Fe(OH)3(s)). The Maludam river was predicted to be supersaturated for Fe hydroxides and the results affirmed our model prediction. The output showed Fe and Cu had a strong affinity for DOC and to a lesser extent Al and Ni in the conditions prevailing at the study sites. Statistical analyses also indicated strong correlation between Cu and Ni (r2 = 0.97, 0.94 and 0.82) in Maludam, Sebuyau and Belait rivers and estuaries, respectively. The results obtained in this study are comparable to values published for southeast Asia and other continents for pristine peat draining rivers.
Highlights
✔ We observed initial remobilization of dissolved Fe, Al, Si, Mn, Cu and Ni at low salinity and pH, followed by scavenging with increasing ionic strength.
✔ The combination of pH, salinity and DOC regulates the behaviour and distribution of trace elements.
✔ Low pH and high DOC concentration are directly proportional to increased apparent Fe solubility (SFe(III)app).
✔ Linear regression shows a strong correlation between trace elements investigated.
✔ Concentration and distributions of trace elements are subject to seasonal variations in peat-draining rivers.
✔ Trace element concentrations in Borneo tropical peat swamps are comparable with other global rivers.
1 Introduction
Tropical peatlands are essential ecosystems which sequester carbon (Cooper et al., 2019), provide habitats and biodiversity conservation, and protect endangered species (Nurulita et al., 2016). Other services include water purification and storage, flood prevention, cultural heritage, education, tourism, and food for local communities (Tonks et al., 2017; Dhandapani et al., 2020). Despite occupying only 0.3% of the global land area (Graham et al., 2017), peatlands store about 89 Gt carbon and serve as a large terrestrial CO2 sink (Wu et al., 2019). It is estimated that southeast Asian tropical peat swamp forests store about 68.5 Gt of carbon (Jauhiainen et al., 2005; Kiew et al., 2018), accounting for 11-14% of the global peat carbon stock (Verwer & Meer, 2010; Page et al., 2011), primarily in huge peat deposits in Sumatra and Borneo.
Peat swamp forests are facing increased anthropogenic activities, in the form of land-use, drainage canals, biomass burning and lowering of the water table. Peat swamps are therefore at risk of becoming potential carbon sources (Martin et al., 2018). The fresh waters draining the ombrotrophic peat swamp forest (i.e., a system where atmospheric deposition supplies nutrients and trace elements over time) in southeast Asia are rich in dissolved organic matter (DOM) that reduces the riverine pH to<5 (Wu et al., 2019). The anthropogenic activities in the regions result in increased fluvial export of DOM, an important contributor to the marine carbon budget (Gandois et al., 2020). The transfer of DOM from tropical peat swamp forests through estuaries could have an impact on the biogeochemical cycles of other elements. In particular, the close association between trace elements and DOM could affect trace element fluxes from the land to the ocean in these regions. The export of major elements and trace elements from peat swamps to surface ocean waters has been reported (Weiss et al., 2002; Rothwell et al., 2007; Broder and Biester, 2017; Jeremiason et al., 2018). There is substantial literature to facilitate a better understanding of the biogeochemistry of major and trace elements in high-latitude peatlands, peaty riparian zones (Broder and Biester, 2017), peatlands and bogs in the UK and Europe (Rothwell et al., 2007), but to the best of our knowledge scant information exists on transport of trace elements in low latitude peatlands, despite increasing destruction of peat swamps in southeast Asia.
Some trace elements (e.g., iron (Fe), manganese (Mn), copper (Cu) and nickel (Ni)) are essential as micro nutrients for organisms including phytoplankton to form enzyme cofactors (Morel et al., 2003; de Carvalho et al., 2021). However, at elevated concentrations in the environment trace elements, including Cu (Mason, 2013) and Al (Simonsen et al., 2019) can be toxic to living organisms. A number of trace elements such as Fe (II and III), Cu (I and II) and Mn (II-IV) are redox sensitive under specific environmental conditions, with Fe (II), Cu (II), Mn (II), Ni (II) as the more soluble and bioavailable forms (Yang et al., 2019; de Carvalho et al., 2021). The association between Fe and organo-mineral complexes, results in the formation of colloids, which provide suitable surfaces for binding of elements, and have important implications for trace element export in peatland (Krachler et al., 2010). Iron and Cu, and to lesser extent Aluminum (Al) and Ni, have very strong affinity for DOM, and DOM is thought to play a role in the mobilization and transport of trace elements through estuaries (Rothwell et al., 2007; Gandois et al., 2020). Aluminum, for example, is an element whose chemistry is driven essentially by the pH of the medium and that usually forms complexes with organic matter and exists in the form of oxides, hydroxides and aluminosilicates (Simonsen et al., 2019). Aluminum tends to be present in estuaries as a positively charged low molecular mass (LMM) cation species at pH< 6, while at about pH > 6, Al may exist as colloids, particles and natural species (Simonsen et al., 2019). The behavioral differences exhibited by trace elements underscores the need for better understanding of their sources, export (Gandois et al., 2020), redox conditions, mobility, bioavailability, environmental concentrations, reactivity and interactions in rivers draining tropical peat swamps. Trace element export from such a dynamic and diverse ecosystem is considered to be regulated by biological, chemical and physical conditions (Rothwell et al., 2007). Specific contributing factors include pH, ionic strength, fluvial regime, suspended particulate matter (SPM) levels, concentration of complexing agents and DOM (Achterberg et al., 2003; Broder and Biester, 2017; Jeremiason et al., 2018).
The dynamic nature of estuarine environments implies that transported materials will be subjected to pronounced changes in pH, ionic strength and DOM levels as fresh waters mix with sea waters (Zhou et al., 2016). Previous studies highlighted the interparticle forces that exist between colloidal materials and the role of humic substances in regulating the behaviour (response of an element to changes in environmental conditions) of trace elements in natural waters (Mosley et al., 2003; Sander et al., 2004). The surfaces of river-borne particles are usually enveloped by a film of natural organic matter, thereby rendering them negatively charged and creating an electrostatic repulsive force. The process of flocculation is initiated by increasing ionic strength and availability of seawater cations (e.g., Mg2+ and Ca2+) as particles enter the estuary from the freshwater region, resulting in gradual partitioning from the dissolved to particulate phase. These processes contribute to the rapid removal of trace elements from natural waters in particulate form (Sholkovitz, 1976; Zhou et al., 1994; Mosley et al., 2003; Sander et al., 2004; Zhou et al., 2016).
The biogeochemically diverse nature of the tropical peat swamps in southeast Asia, coupled with the rapid rate of their destruction due to land use change, peat fires, agricultural activities and drainage canals may contribute to enhanced trace element export. There is little research on the export of trace elements such as Fe, Al, Si, Mn, Cu and Ni to rivers draining tropical peat swamps. The purpose of this research is to a) resolve the concentrations of trace elements in rivers draining pristine tropical peat swamp forests, b) examine the role of seasonal variations on trace element distributions in the rivers and estuaries and, c) unravel the influence of estuarine processes on the supply of trace elements from black water rivers to the ocean.
2 Description of the study area
Southeast Asia contains about 23 million hectares of lowland tropical peatlands with characteristic waterlogged and predominantly peat swamp forests vegetation (Wetlands International, 2010). Borneo Island, covering some parts of Malaysia, Indonesia, and Brunei, with its rich biodiversity has been accorded a global conservation priority to ensure sustainability and protection of certain endangered species. It is classified as tropical ever-wet because of the climatic conditions with an average rainfall of 3700 mm year−1 (Wu et al., 2019). Malaysia is rich in peat swamp forests distributed across the various states with 23 percent retaining near-pristine nature; 17 percent are located in the state of Sarawak (Wetlands International, 2010). The state of Sarawak on the island of Borneo has the largest tropical peat swamp, thereby making Malaysia the second largest home to tropical peat swamp forests with an area of approximately 2.6 x 104 km2 (Zhang et al., 2020). The establishment of major industries such as metal, food processing, electronics, petroleum, rubber, and textile in Malaysia (the mid-1980s) contributed to an increase in the deforestation rate in the country. The conversion of tropical peat swamp forests for agricultural purposes including rubber, oil palm, and sago plantations between 1990 and 2010 accounted for a 2 percent increase in the deforestation rate yearly in Sarawak (Zhang et al., 2020). Indonesia accounts for about 80 percent of the tropical peat swamp forests globally, although about 94,000 km2 (above 36,000 square miles) have been lost due to anthropogenic activities (Dargie et al., 2017). Brunei Darussalam is a small oil-rich country located on Borneo Island, covering 5,765 km2 (2,226 square miles) and rich in pristine tropical peat swamp forests (Omar et al., 2022). Brunei’s forests, peat swamps, mangroves, and rivers in protected areas are home to various unique species which are endemic to Borneo. The three rivers under investigation, namely Maludam and Sebuyau in Sarawak state of Malaysia and Belait river in Brunei drain peat swamp forest waters into the South China Sea.
Maludam is a town and sub-district in Betong Division, in the state of Sarawak, Malaysia. The Maludam River, is known for its pristine nature with little anthropogenic influence since it is located in the Maludam national park, Sarawak’s second-largest national park. The catchment area of the Maludam River covers about 91.4 km2 and has an average discharge rate of 4.4 -5.9 m3 s−1 (see Table 1). The blackwater river draining the peat swamp forests is about 33 km long with its majority running through the national park. This biologically diverse national park is pristine with minimal anthropogenic impact (Müller et al., 2015).
The Sebuyau River with characteristic black colored, oxygen-deficient and acidic waters stretches for about 58 km and has increased human activities compared to the Maludam River. Sebuyau River has a discharge rate of 34.5 m3 s−1 and drains the least pristine forest of the three rivers investigated, with some peat swamps within its catchment converted to sago and oil palm plantations (Wu et al., 2019).
Belait River, the longest river in Brunei at about 32 km, is located near the southwestern border of Sarawak. The Belait river flows through peat swamps and discharges into the South China Sea. The river meets the ocean at Kuala Belait port, an economic nerve center and home to some of Brunei’s oilfields. Located about 10 km inland is the water treatment plant and other facilities at Badas with different abstraction points (Brunei Liquified Natural Gas intake, Public Works intake and Brunei Shell Petroleum intake) (Chuan, 1993). Upstream, the forests draining the Belait river is pristine with relatively little agricultural activities and human impact. The possible sources of pollution in the Belait river include industrial discharges such as the shipping harbour, wastewater treatment plants, oil facilities, agricultural outlets, domestic waste, surface runoff, and polluted streams occasioned by increase in population density. The country’s tropical climate is characterized by enhanced rainfall (average annual rainfall 3000 mm year−1) and temperatures (annual mean 27.4°C) (Harris et al., 2020). The river’s capacity for self-purification is an essential ecosystem service despite its use for various purposes such as transportation and waste disposal (Parenti and Meisner, 1995).
3 Materials and methods
3.1 Sample collection and treatment
Samples were collected in July 2019 (dry season) and December 2019 (wet season) to study seasonal variations in the transport of trace elements from peat swamps to adjacent rivers and estuaries. A total of 42 dissolved and 10 particulate samples were collected from the blackwaters of Belait, Maludam and Sebuyau rivers and estuaries using a small boat (Figures 1A–D). Intense flooding and strong currents during December 2019 limited the number of locations that could be sampled. pH, salinity and temperature were measured at the stations, using a multiprobe (Aquaread AP-2000).
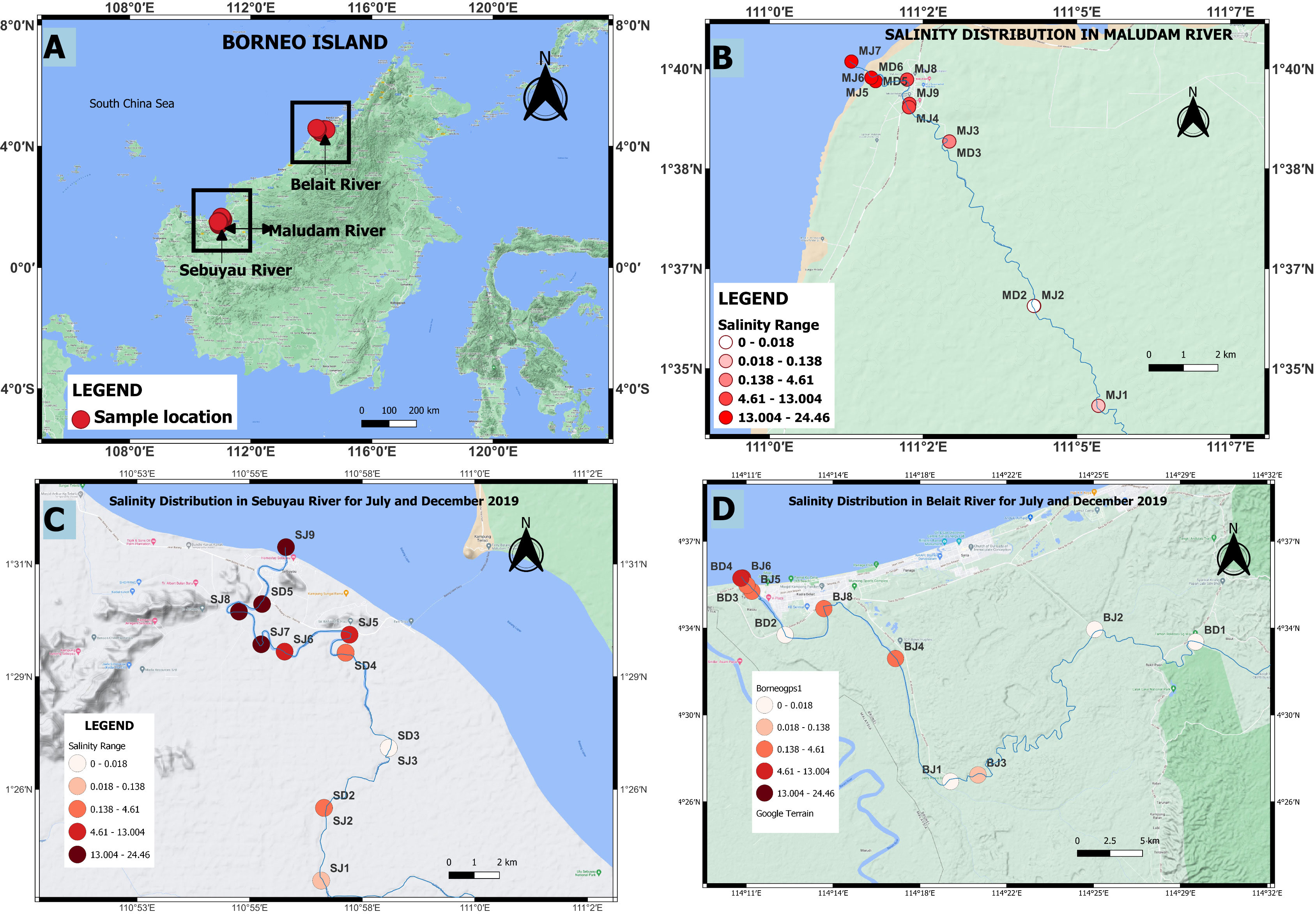
Figure 1 Distribution of sampling stations and salinity in the study area (A) Borneo Island (B) Maludam river (C) Sebuyau river (D) Belait river in July and December, 2019. MJ, Maludam July; MD, Maludam December; SJ, Sebuyau July; SD, Sebuyau December; BJ, Belait July and BD, Belait December.
All low density polyethylene bottles used for sample collection and storage were acid cleaned in a trace element clean laboratory: the bottles were rinsed three times with deionized water (Milli-Q, Millipore), immersed in 2% Mucasol detergent for 24-48 hours, rewashed 3-5 times with deionized water, leached in 1 M reagent grade hydrochloric acid (HCl; 37%, Thermo Fisher Scientific) for 7 days, rinsed with deionized water 3-5 times, transferred to 1.5 M nitric acid (HNO3; ≥65%) for 7 days, rinsed for 5-7 times in deionized water, dried for 48 hours in laminar flow hood and sealed in double plastic bags (Rapp et al., 2017). The collected sample waters were immediately filtered using polyethersulfone (PES; 0.45 µm poresize, 47 mm, Sterlitech Corporation) membrane filters for trace elements. Filtered trace element samples were stored in acid cleaned 60 ml polyethylene bottles. The samples were acidified with HCl (37%, Optima Thermo Fisher Scientific) to pH 1.7in a trace element clean laboratory and preserved for six months.
3.2 Trace element analysis
The trace element samples were processed and analyzed in trace element clean laboratories at GEOMAR. The samples were ultraviolet (UV) digested in acid cleaned quartz tubes in order to breakdown metal complexing organic matter. The tubes were rinsed 5-7 times with deionized water, cleaned with 1 M HCl (37%, Thermo Fisher) for 24-48 hours, and rinsed again with deionized water 5-10 times between digestion cycles. To each tube containing about 30 ml of sample, 150 µl of hydrogen peroxide (H2O2; ≥30%, Sigma Aldrich) was added to speed up the destruction of organic matter (Achterberg et al., 2003). Each batch of samples was exposed to UV light from a medium pressure mercury lamp (Photochemical Reactors; 400 W) for 4-5 hours, and then transferred into clean acid washed bottles. All samples with salinity > 2 were diluted to salinity 2 prior to analysis. Samples were prepared in duplicate to confirm reproducibility. Trace elements were analyzed using high resolution inductively coupled plasma mass spectrometry (HR-ICP-MS; Element XR, Thermo Fisher Scientific). Indium was used as the internal standard throughout the analytical process. River water certified reference material (CRM, SLRS 6 Riverine Water, Canada) for trace elements from National Research Council, Canada was prepared using the same procedure as the water samples and analyzed using HR-ICP-MS. Table 2 shows the results for the CRM and trace element analyses, including the percentage recovery for the CRM. We prepared standards for both fresh and seawater samples using trace element concentrations in Southeast Asia (Ishak et al., 2016; Tashakor et al., 2018; Zhang et al., 2020). 150 µl of seawater sample was added to the standards to create similar conditions and used for calculating trace element concentration in seawater samples. The ICP-MS counts were blank and drift corrected before calculating concentration using the slope. The full procedural blanks were obtained during expedition by passing deionized water through the same procedure as samples. The reported concentrations are the blank corrected average of two runs (8 replicates – 4 each) ± % error (using average and average deviation). We reported the median trace element concentration as measured in the study area without normalization to the method recovery because the sample concentration is higher than the CRM. The duplicates were reproducible as shown in Table 2.
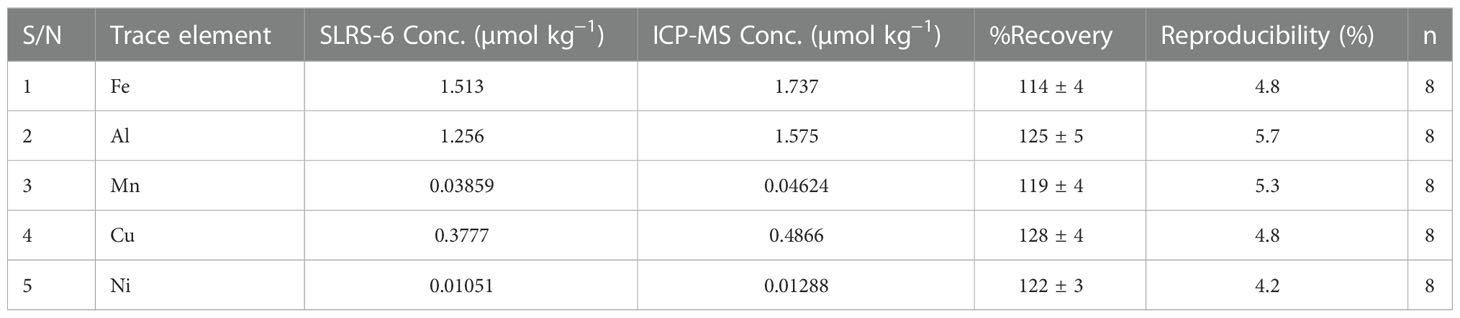
Table 2 Certified reference material concentration vs measured ICP-MS concentration showing percentage recovery.
3.3 Particulate trace element analysis
Particulate samples were obtained by filtering water samples through 0.45 µmpore size polyethersulfone (PES) membrane filters. The filters, containing particulate matter, were retained and stored frozen. Prior to analysis, samples were defrosted. Each filter was then carefully stuck to the wall of an acid-cleaned 30 ml vial (savillex) and 2.5 ml of digest reagent (1 M HNO3 – 50%HNO3:49%MQ:1%Re) was added into each vial. Vials were then placed on a hot plate at 150°C overnight, for at least of 15 hours. The next day, the vials were uncapped and the samples evaporated for about 2 hours on the hotplate at 110°C to near dryness. Samples were allowed to cool for ~30 mins before addition of 0.5 ml oxidizing reagent (35%MQ:15%H2O2:50%HNO3 solution) to each vial. The caps were left, loosely capped for 30 mins, then placed uncapped on the hotplate at 110°C for 35-45 mins. After this, samples were rediluted in 4.5ml of 1 M HNO3 (63ml conc. HNO3:936ml MQ:1ml Indium (1mg/ml)) and heated at 80°C for 1 hour. Cooled samples were then decanted into pre-cleaned 15ml tubes. Standards were prepared following the same method for 0.015-0.020g of plankton (BCR 414) and 0.015-0.020g marine sediment (PACS 3) certified reference material (CRM) (typically 3 vials for each CRM), and procedural blanks applied the same method to blank filters. At least 3 vials were filled with re-dilution acid as blanks. Some membrane filters (for particulate samples) were lost in the process and this resulted in the presentation of only 10 samples.
3.4 Measurement of dissolved organic carbon and nutrient concentrations
DOC samples were collected in 3 ml glass vials (Nalgenunc, Nalgene) with Teflon-lined caps. The vials were rinsed 3 times in deionized water, cleaned in 10% HCl for 1-2 days, rinsed 5-7 times in deionized water, and combusted at 450°C for 4 hours. The vials were then pre-killed with 5 drops of concentrated phosphoric acid. DOC samples were filtered into the vials in the field using ashed (450°C for 4 hours) glass fibre filters (GF/F Whatman; 47 mm diameter). The samples were analyzed at the Centre for Coastal Biogeochemistry at Southern Cross University (Lismore, Australia) via continuous-flow wet-oxidation isotope-ratio mass spectrometry using an Aurora 1030W total organic carbon analyzer coupled to a Thermo Delta V Plus IRMS (Oakes et al., 2010). Glucose of known isotopic composition dissolved in He-purged deionized water was used as a standard to correct for drift and to verify sample concentrations. Reproducibility for concentrations was ± 0.2 mg L−1.
Water samples collected for nutrient analyses were filtered via polycarbonate membrane filters (0.4 µm pore size, Whatman®) into 60 mL sampling bottles. Concentrations of nutrients were determined in the State Key Laboratory of Estuarine and Coastal Research (SKLEC), Shanghai utilizing a Skalar SANplus auto analyser (Grasshoff et al., 1999). After thawing, water samples were thoroughly mixed prior to nutrient analyses. Dissolved silicate (DSi) was analyzed using a Skalar San++ Flow Injection Analysis system (Netherland) according to standard colorimetric methods modified by the manufacturer (Grasshoff et al., 1999).
3.5 Flux calculations
The difference between precipitation and evapotranspiration was used to calculate total flux based on catchment area and element concentration. The discharge in the catchment (Q) is given by the difference between the precipitation (P) and the evapotranspiration (ET), Q = P - ET. Precipitation rates were downloaded from DWD (https://www.dwd.de/EN/ourservices/cdc/cdc_ueberblick-klimadaten_en.html) as monthly averages. The nearest stations to our sampling locations are Kuching and Brunei. We calculated evapotranspiration (ET) using three relevant references (Kumagai et al., 2005; Moore et al., 2013; Hirano et al., 2015) and averaged the results for subsequent calculations. We obtained the monthly ET by dividing the annual ET by 12. Monthly total loads for July and December were then calculated by multiplying the discharge with the elemental concentrations.
3.6 Statistical analysis
All statistical analyses were done using R, 2021 (Version 4.1.1), R Studio Team, 2021 (Version 1.4.1103), tidyverse (2019) packages, plots with Origin 2018 and maps with QGIS (Version 3.24.1).
3.7 Visual MINTEQ and NICA-Donnan modeling
The chemical equilibrium model Visual MINTEQ (version 3.0) was used to calculate metal binding affinity to dissolved organic matter (DOM) (Chahal et al., 2016) in the blackwater rivers of Borneo Island. The generic fulvic acid NICA constants for H+, Mg2+, Ca2+ and Sr2+ were used to calculate trace element affinity to DOM (2003; Milne et al., 2001). Calculations were performed at ambient temperature, pH, ionic strength (calculated from ion pairing) obtained from the sample sites and major ions of seawater; Na+, K+, Mg2+, Ca2+, Sr2+, Cl−, , , Br−, H3BO3 and F− to calculate metal binding affinity to DOM (Zhu et al., 2021). Table 3 shows constants for Al3+, Cu2+, Fe3+ and Ni2+ used to determine metal affinity to DOM as described in (Gledhill et al., 2022). The NICA-Donnan model uses a non-ideal competitive adsorption (NICA) isotherm to describe binding to heterogenous substances and the Donnan electrostatic sub-model to explain the electrostatic interaction between humic substances and ions (Milne et al., 2003). The NICA-Donnan model is widely used in different studies because of its ability to calculate the binding affinity between ions, fulvic and humic acid under varying environmental conditions (Milne et al., 2001). Hence, the application of this model allowed us evaluate the role of humic substances in the mobility and distribution of trace elements in our estuaries. The pH range, trace element and DOC concentrations measured in the rivers are provided in Table 3. We used DOC, pH and dissolved Fe data to interpret apparent iron solubility (SFe(III)app) in the systems. The concentration of total Fe was modified to 100 µmol kg−1 in the model to assess the saturation state of iron hydroxides or oxyhydroxides.
4 Results and discussion
4.1 Water chemistry in Borneo rivers and estuaries
The conditions under which samples were collected during expeditions in the wet (December 2019) and dry (July 2019) seasons are presented in Figures 1A–D and Table 4. Flooding during the wet season (December) affected the number of samples collected and by extension the available data on the chemistry of the various rivers and estuaries across fresh and intermediate salinity. Furthermore, a range of stations were in different places in July and December. In Maludam, the salinity in our samples in July 2019 (dry season) ranged from 0.04-24.46, and 0-17.57 in December, 2019 (wet season). This river is strongly acidic with a minimum pH of 3.3 at salinity 0.05 in July, 2019 and pH 4.23 at salinity 0 in December, 2019. In Sebuyau river and estuaries, the minimum pH was 5.22 at salinity 0.02 in July and pH 4.56 at salinity 0 in December. Belait river and estuary recorded minimum pH of 4.6 at salinity 0.01 in July and pH 4.35 at salinity 0 in December (Figure 2A–C). In terms of flow rate in the river (freshwater endmember), Maludam has the lowest discharge 4.5 m3 s−1 and is a more acidic system (pH 3.3), followed by Sebuyau with discharge 34.5 m3 s−1 with pH 4.6 and Belait with higher discharge rate 145 m3 s−1 with pH 4.4 (Table 1). The acidic conditions measured in these rivers are consistent with reported values for Maludam (pH 3.7), Sebuyau (pH 4.3) and Simunjan (pH 4.7) rivers (Zhang et al., 2020). The data obtained for pH and salinity during the wet and dry seasons show a similar trend with little variation in Maludam, Sebuyau and Belait rivers and estuaries. However, the sampled salinity range in Belait is limited with fewer data points compared to others (Figure 2A). In the Maludam estuary, for example, at salinity 18.3 the pH was 7.51 in the dry season, while during the wet season, pH 7.62 was measured at salinity 17.6. In Sebuyau estuary, at salinity 14.6 the pH was 7.51 during the dry season whereas in the wet season, pH 6 was measured at salinity 13.02.
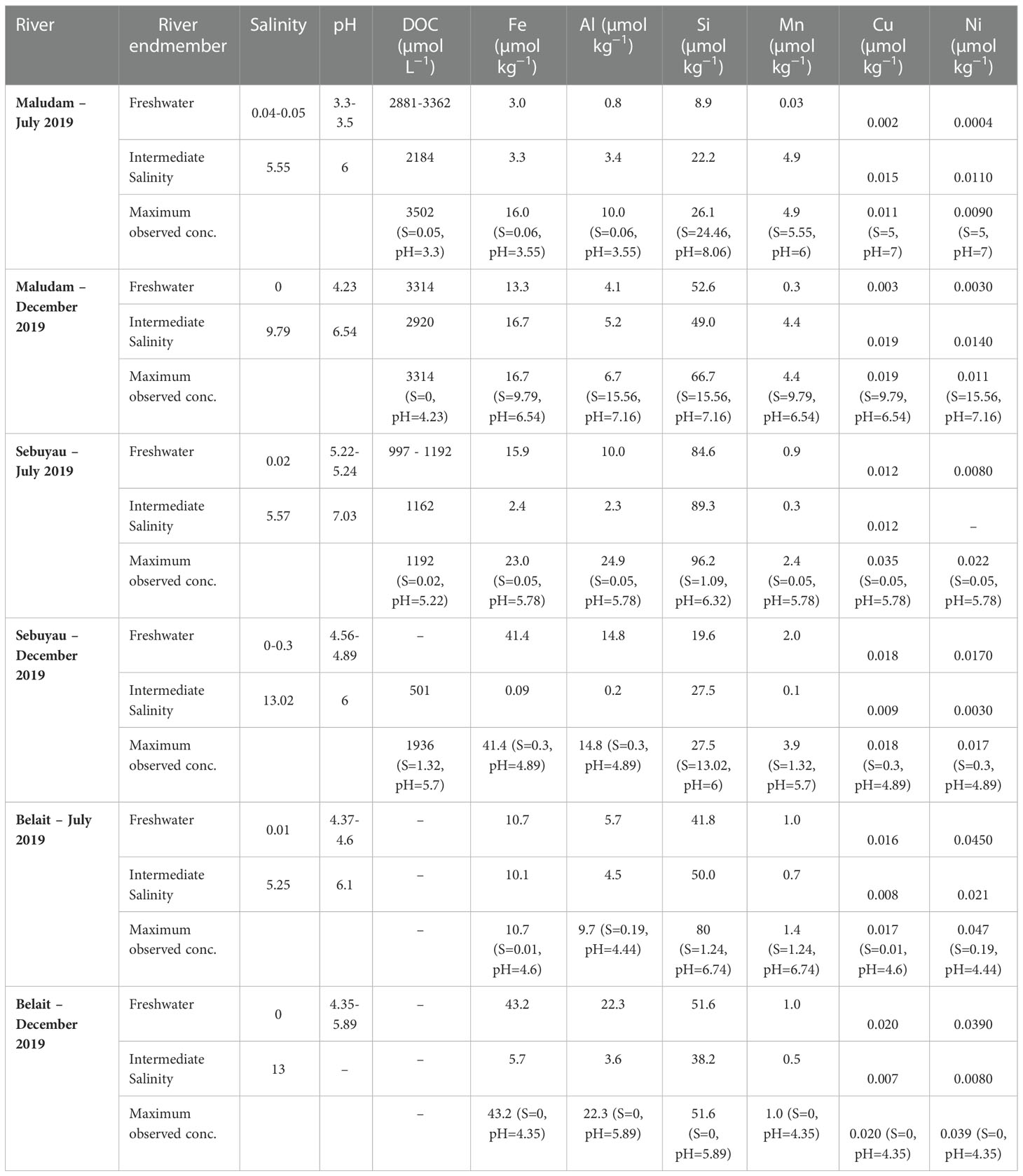
Table 4 Range of the master variables. Salinity, pH, and dissolved organic carbon (DOC) in three backwater rivers and estuaries, and the corresponding concentrations of Fe, Al, Si, Mn, Cu and Ni in the fresh water endmember and intermediate salinity.
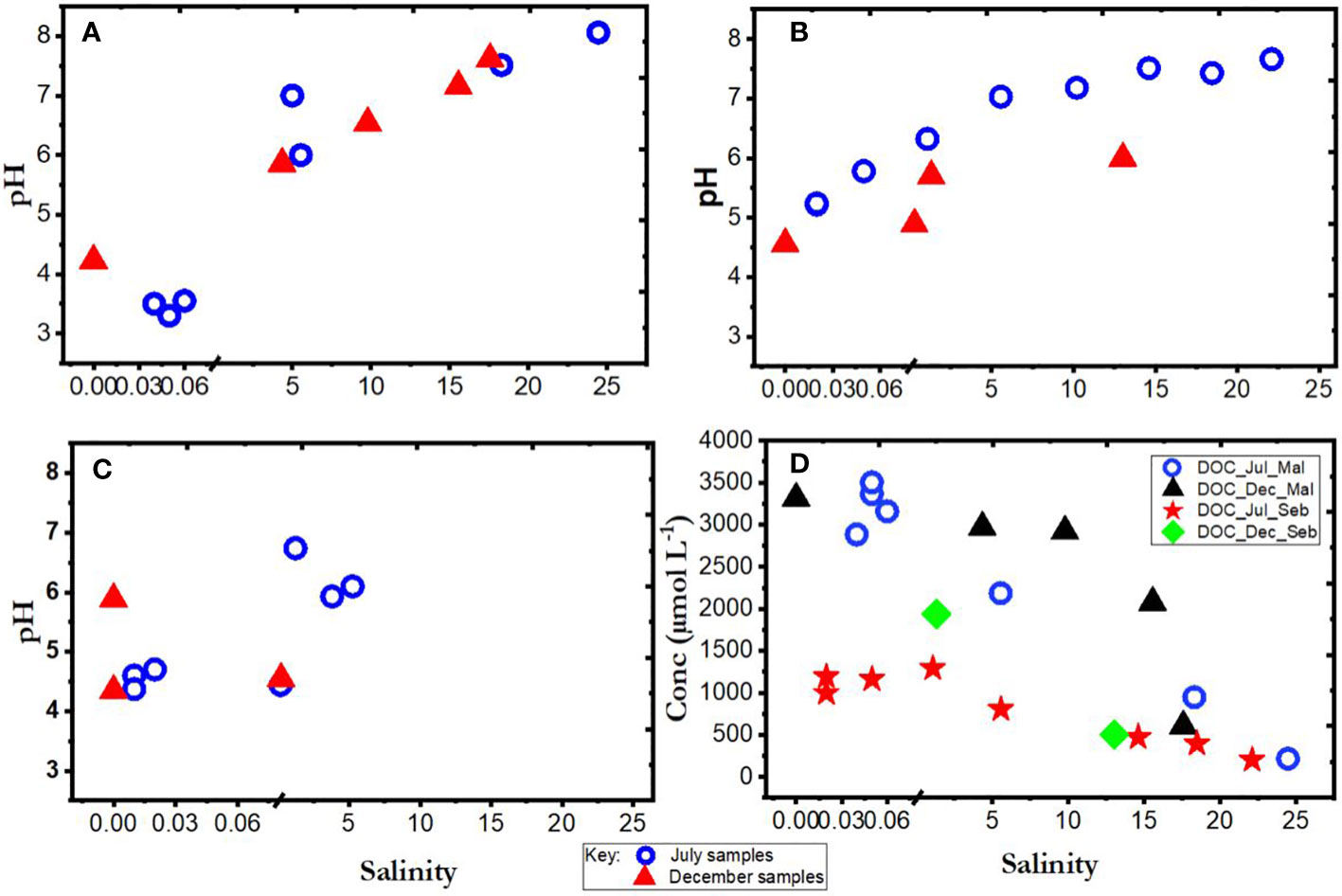
Figure 2 Plots of pH as a function of salinity in (A) Maludam (B) Sebuyau and (C) Belait, and (D) DOC as a function of salinity for Maludam (Mal) & Sebuyau (Seb) rivers, as measured during July and December 2019.
We present only DOC data for Maludam and Sebuyau here because that of Belait is unavailable due to challenges encountered during sampling. The DOC concentrations in Maludam in July 2019 ranged between 215 and 3502 µmol L−1, indicating gradual dilution of the DOC rich blackwater river as it travels through the pristine peat swamp park to waters with intermediate salinity (Zhang et al., 2020). DOC peak concentrations measured in Maludam river were attributed mainly to the organic rich nature of woodlands, peat swamps and forests (Jiann et al., 2013). The DOC concentrations in Sebuyau varied from 201 to 1290 µmol L−1 with the maximum concentration observed in the freshwater endmember and a decrease along the salinity gradient due to dilution with relatively DOC poor seawaters (Table 4; Figure 2D).
4.2 Trace element concentrations in blackwater rivers in Borneo Island
The concentrations of dissolved Fe, Al, Si, Mn, Cu and Ni in the blackwater rivers and estuaries are shown in Figures 3A–C and Table 4. The overall maximum concentrations for dissolved Al (24.9 µmol kg−1), Si (96 µmol kg−1), Mn (4.9 µmol kg−1), Cu (0.035 µmol kg−1), and Ni (0.047 µmol kg−1) were observed during the dry season, while dissolved Fe (43.2 µmol kg−1) reached peak concentrations during the wet season. The subsection on seasonal variation for each trace element in the different rivers shows interesting perspectives on their distribution. The concentration of dissolved (D) and particulate (P) trace elements at a range of salinities and pH values in Maludam, Sebuyau and Belait rivers are shown in Table SI 1. The data highlights the role of salinity and pH in driving trace element fluxes in the dissolved and particulate phases. Concentrations of dissolved trace elements were higher in the freshwater endmember, while particulate trace element concentrations were higher in the mid to high salinity and pH region. At salinity 9.79 in the Maludam estuary, Fe concentration was 139 µmol/kg (particulate – P) and 16.7 µmol kg−1 (dissolved – D), Al concentration was 935 µmol kg−1 (P) and 5.2 (D), Cu concentration was 0.052 µmol kg−1 (P) and 0.019 µmol kg−1 (D) and Ni concentration was 0.119 µmol kg−1 (P) and 0.014 µmol kg−1. All trace elements had higher particulate than dissolved concentrations, except for Mn, with a dissolved concentration (4.4 µmol kg−1 - D) that was higher than the particulate concentration (1.6 µmol kg−1 - P) (Dupré et al., 1996).
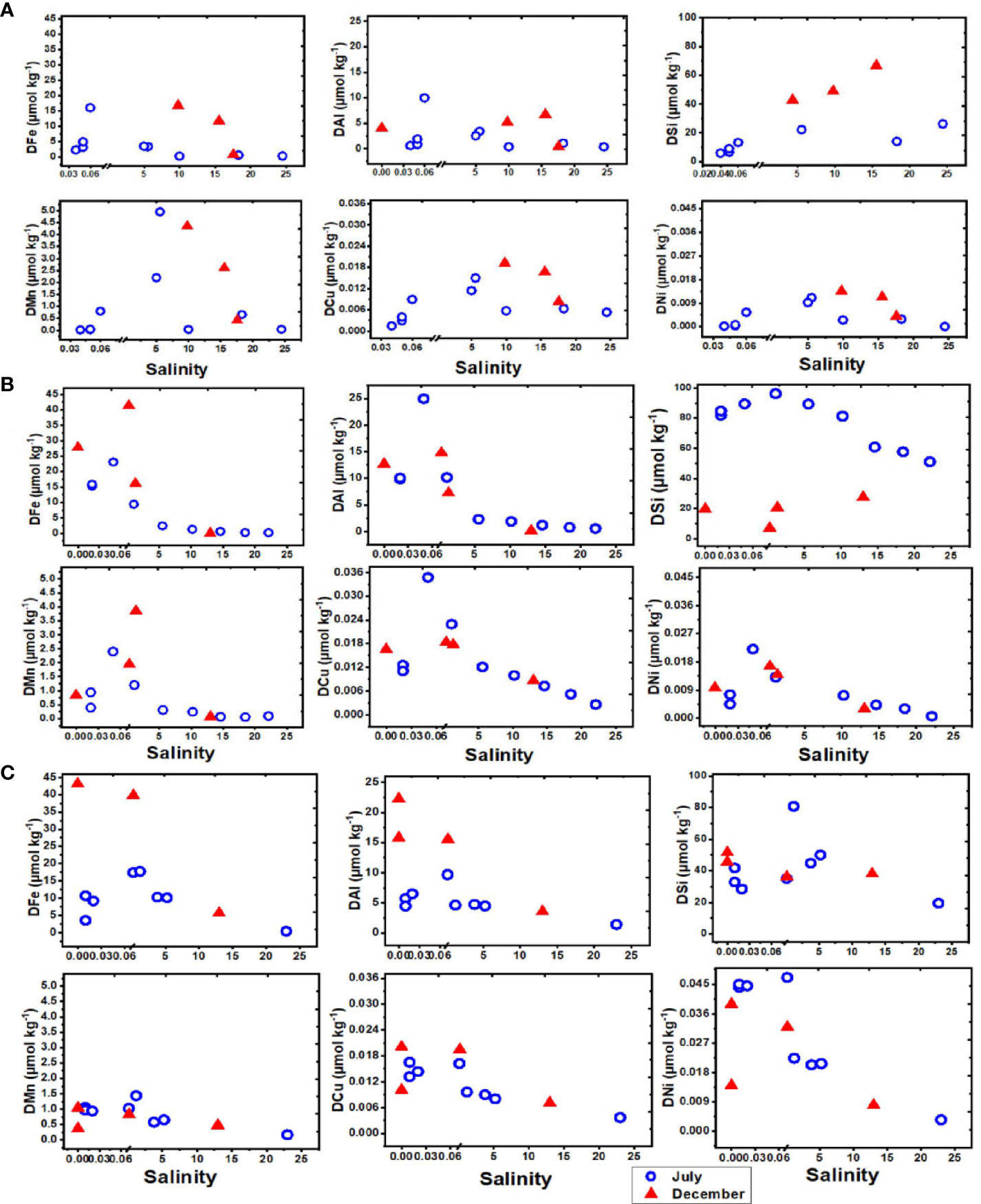
Figure 3 Dissolved elements as a function of salinity in the blackwater rivers and estuaries of Borneo in July (dry) and December (wet), 2019. Each plot shows the distribution of elements (Fe, Al, Si, Mn, Cu and Ni) in (A) Maludam (B) Sebuyau (C) Belait rivers and estuaries.
Concentrations of dissolved Fe, Al, Mn, Cu and Ni were considerably higher at lower salinities (< 1) than at mid-salinities in the Borneo rivers (Figure 3). This is consistent with patterns observed in the river near the city of Pontianak, West Kalimantan, Indonesia (Gandois et al., 2020), and dissolved Fe concentrations in Maludam, Sebuyau and Rajang rivers (Zhang et al., 2020). Previous studies attributed the higher concentrations in peat draining blackwater rivers to the strong metal organic associations between Fe, Cd, Zn, As, Ni and DOM which stabilize the elements and prevent their removal through precipitation (Krachler et al., 2010; Neubauer et al., 2013; Broder and Biester, 2017), and the corresponding discharge of DOM rich water from the peat swamp which creates acidic conditions (Krachler et al., 2012; Gaillardet et al., 2013). At low salinity,< 0.5, there was an initial increase in the concentration of dissolved trace elements in the estuary. This suggests trace elements were released from particles containing exchangeable elements during the initial mixing between river and saline waters with enhanced ionic strength in the estuary. Subsequent to this release, elements were scavenged or precipitated at salinities between 0.5 and 10 due to further increases in ionic strength and pH which destabilized colloidal metal fractions (Achterberg et al., 2003; Mosley et al., 2003). The observed increase in particulate trace element concentrations with increasing ionic strength (Table SI 1) and the decrease in dissolved trace element concentrations support the role of estuarine processes in metal fluxes in the mixing zone (Thanh-Nho et al., 2018). Geochemical processes involving Fe play a central role in metal removal. Iron solubility is much greater at low pH and salinity, and an increase in pH and salinity in the estuary thus induces the precipitation of Fe and Mn oxyhydroxides (Achterberg et al., 2003; de Souza Machado et al., 2016). The behaviour of other trace elements is then regulated by Fe and Mn oxyhydroxide precipitation in the estuary as it creates suitable surfaces for adsorption (Turner and Millward, 2002; Mari et al., 2012). Furthermore, microorganisms and phytoplankton may also play a contributary role in trace element removal by releasing transparent exopolymer particles (TEP) in estuaries around mid to high salinity reaches that facilitate aggregation processes (Wetz et al., 2009; Mari et al., 2012).
4.3 Seasonal variation in blackwater rivers and estuaries
Seasonal variations in temperature and discharge may regulate the concentrations of trace elements; e.g., dissolved Fe delivered to the rivers may change as a function of mechanical and chemical weathering processes (Caccia and Millero, 2003). Seasonality plays an important role in the concentration dynamics of trace elements in these blackwater peat draining rivers and estuaries (Zhang et al., 2020). Our results in each river and estuary across the wet and dry seasons indicate maximum observed concentrations in Maludam (DOC – dry, Fe – wet, Al – dry, Si – wet, Mn - dry, Cu – wet and Ni – wet), Sebuyau (DOC – wet, Fe – wet, Al – dry, Si – dry, Mn -wet, Cu – dry and Ni – dry) and Belait (Fe – wet, Al – wet, Si – dry, Mn - dry, Cu – wet and Ni – dry), respectively. In the different rivers, variability exists in the distribution and concentration of dissolved and particulate trace elements in relation to seasonal variation. We observed maximum DFe concentrations in Maludam (16.7 µmol kg−1) and Sebuyau (41.4 µmol kg−1) and Belait (43.2 µmol kg−1) rivers during the wet season. The maximum concentration at salinity 9.79 observed in Maludam for particulate Fe (139.5 µmol kg−1) during the wet season was an order of magnitude higher compared to the dissolved Fe (16.7 µmol kg−1) concentration. This can be attributed to the mobilization of mobile reduced Fe (FeII) from anoxic peat swamps (Zhang et al., 2020). The observed DFe concentrations are consistent with those reported in Maludam (23.8 µmol kg−1), Sebuyau (33.6 µmol kg−1), Rajang (8.3 µmol kg−1) and Simunjan (59.2 µmol kg−1) (Zhang et al., 2020) rivers. We observed variations in the maximum concentrations of the other trace elements in the different rivers during the dry and wet seasons (see details in Table 4). The variations in trace element distributions across both seasons underscore the influence of different factors including pH, salinity, DOC, residence time, and temperature on their behaviour in the systems (Caccia and Millero, 2003). The formation of Fe oxide in the estuary mixing zone provides suitable surfaces for the removal of other trace elements, thereby influencing their mobilization and removal. To further examine the relationship between seasonal variation and trace element fluxes, we calculated the discharge for July and December in 2019 using the parameters indicated in Table SI 2 (Kumagai et al., 2005; Moore et al., 2013; Hirano et al., 2015) and calculated DOC and trace element fluxes in the black water rivers. The output indicates the influence of seasonal variation on trace element and DOC fluxes (Figure 4); this can be attributed to increased weathering of rocks, flushing of the peat swamp environment, and enhanced delivery of particles during the wet season. To better understand these interactions, we used principal component analysis (PCA) to reduce the dimensionality of the dataset and enhance its interpretability for the following parameters: pH, salinity, DOC, Fe, Al, Mn, Cu and Ni. PCA1 is the horizontal axis with the highest variation, while PCA2 is the vertical axis with the second-most variation in the dataset. The PCA results in Figure 5A explain 74.9% of variance (PC1 = 53.0% and PC2 = 21.9%) in Maludam, Sebuyau and Belait without DOC data, while, the PCA results in Figure 5B explain 84% of variance (PC1 = 52.9% and PC2 = 31.1%) in Maludam and Sebuyau only with DOC data. The output shows relationship between the different trace elements and DOC; although the relationship is not as strong as expected, it implies that similar estuarine processes affect their distribution and fluxes. However, pH and salinity exhibit an inverse relationship with the trace elements; this implies that increases in pH and salinity are related to decreases in metal concentrations in the estuary (Braungardt et al., 2003). In addition, Cu and Ni exhibited strong correlations in Maludam (r2 = 0.97), Sebuyau (r2 = 0.94) and Belait (r2 = 0.82) rivers (see supplementary information – Figure S1) and this may be due to their similar geochemical properties in the estuaries (Sholkovitz, 1976).
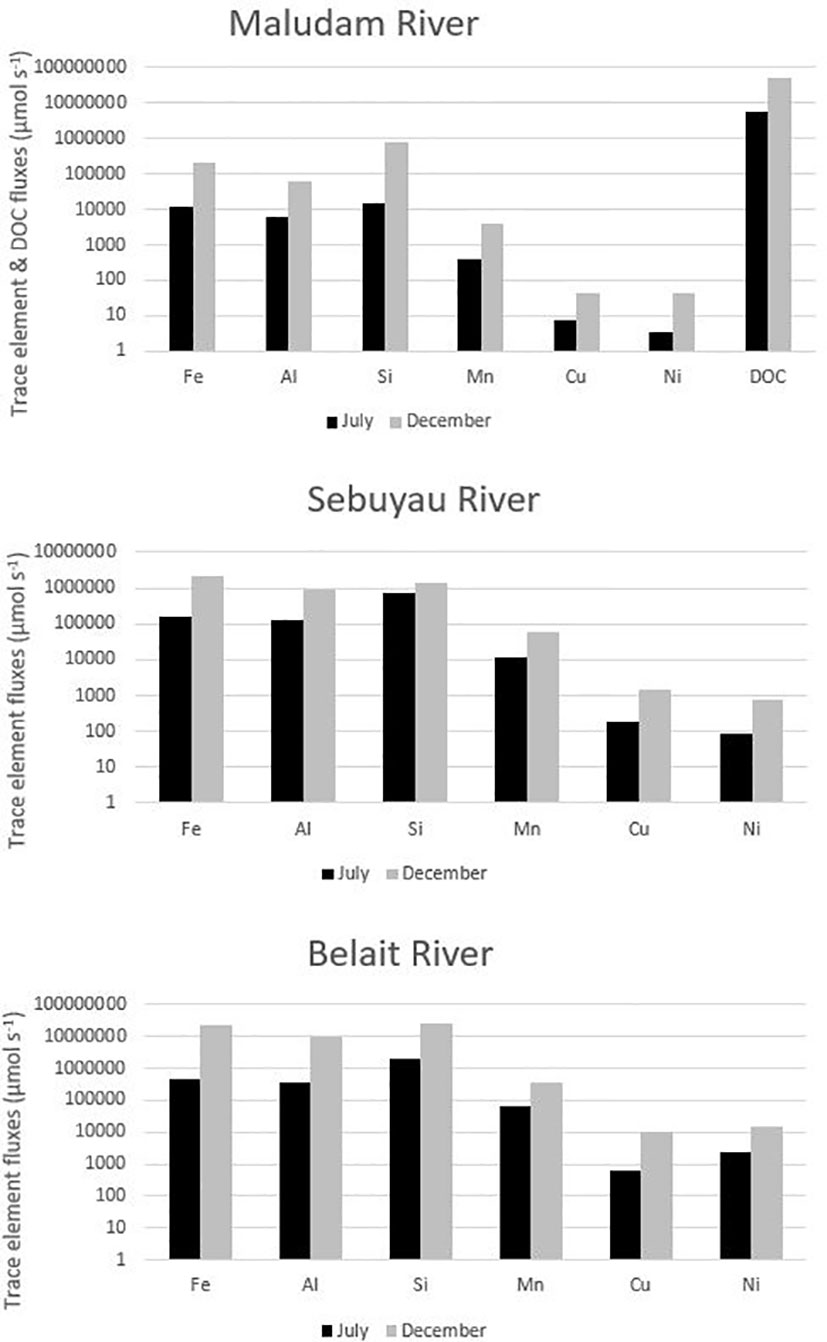
Figure 4 Trace element and DOC fluxes (µmol s−1) as a function of seasonal variation in the freshwater endmember of Maludam, Sebuyau and Belait rivers. Key: July (Dry Season) and December (Wet Season).
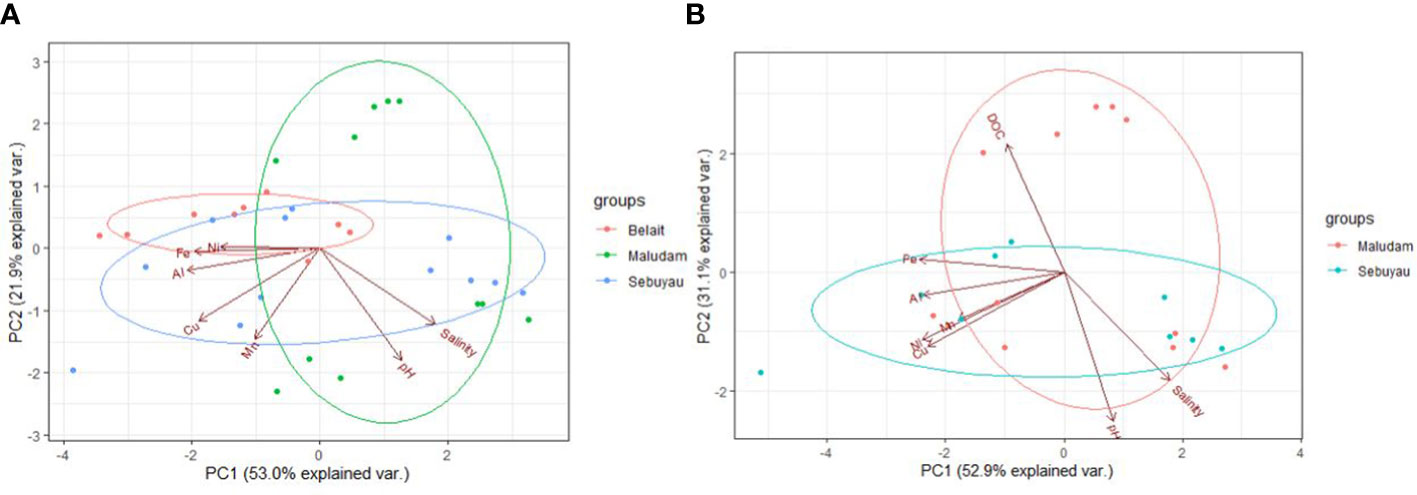
Figure 5 Principal Component Analysis showing the first two principal component. (A) Trace elements in the three rivers without DOC data and (B) Trace elements and DOC in Maludam and Sebuyau rivers. PC1 and PC2 in plot (A) for trace elements accounts for 74.9% of the data, while PC1 and PC2 in plot (B) for trace elements and DOC accounts for 84% of the data respectively. The circles on the plots are visual aids for the sample groups.
4.4 Trace element distribution as a function of pH in the peat draining rivers and estuaries of Borneo
pH plays an important role in determining the behaviour and distribution of trace elements in aquatic ecosystems and forms a master variable for biological and chemical processes (Santschi et al., 1997; Turner and Millward, 2002; Mosley and Liss, 2020). pH affects metal adsorption and solubility, thereby regulating their dissolved concentrations (Millward, 1995; de Souza Machado et al., 2016). The role of pH is particularly important (Millward, 1995; Braungardt et al., 2003) for the blackwater rivers of Borneo Island as these are acidic (pH 3.3) (Table 2) and this is expected to influence metal distribution along the pH gradient (Mosley and Liss, 2020). In Maludam river (pH 4.23), the concentrations of Fe, Al, Mn, Cu and Ni were consistently higher in the dissolved than the particulate phase (Table SI 1) and this is largely related to the acidic medium. At pH 4.35 in Belait river, the concentrations of Fe, Mn, Cu and Ni are higher in the dissolved than the particulate phase. Aluminum in Belait river had higher particulate phase, which may be related to enhanced weathering run-off during the wet season. Dissolved Fe concentration varied from 10 to 23 µmol kg−1 within the low pH (3-5.5) waters in July across Maludam, Sebuyau and Belait rivers, but the concentration doubled in December to 41.4 µmol kg−1 in Sebuyau and 43.2 µmol kg−1 in Belait. However, dissolved Fe remained within the same range in July (15.9 µmol kg−1) and December (16.7 µmol kg−1) in Maludam river (Figure 6). Earlier studies found similar concentrations in the river (Elbaz-Poulichet et al., 1999; Zhang et al., 2020). Aluminum concentrations varied from 9.9 to 24.9 µmol kg−1 with pH range 3-6 in both July and December in the three rivers. Manganese concentrations at pH< 6 varied from 1 to 5 µmol kg−1 in July and December across the rivers. Copper concentrations varied from 0.012 to 0.035 µmol kg−1 at pH< 6 and Ni concentrations varied from 0.009 to 0.047 µmol kg−1 at pH< 6 in July and December in the various rivers. The tendency for trace elements to remain in dissolved phase at low pH (< 6) explains the relationship between them and how pH regulates their geochemistry in the estuary as the master variable (Millward, 1995). As the pH approaches the point of neutralization, scavenging to particles facilitates metal removal from the water column, hence the observed decline in trace element concentrations with increasing pH and salinity (Braungardt et al., 2003). Figure 6 indicates that peak concentrations of trace elements are found in the low pH< 6 range and can be attributed to the influence of available dissolved metal species, positive surface charges and electrostatic force (Achterberg et al., 2003; de Souza Machado et al., 2016). The initial release of Mn, Cu, and Ni at low salinity (<0.5) and low pH (<5.5) implies the presence of a specific source. One possible explanation could be sediments or particles which were formed at higher salinity and pH further downstream in the estuary and subsequently resuspended and transported upstream as a result of tidal mixing (de Souza Machado et al., 2016). The subsequent interaction between the sediments and the low salinity, low pH water may release the trace elements back into the dissolved phase. The subsequent downstream increase in pH and salinity once again promotes scavenging of elements onto Fe hydroxides or colloids (Gaillardet et al., 2013; Mosley and Liss, 2020). In this way the trace elements are likely trapped in a cycle in the upper estuary. These processes are summarized in the schematic Figure 7.
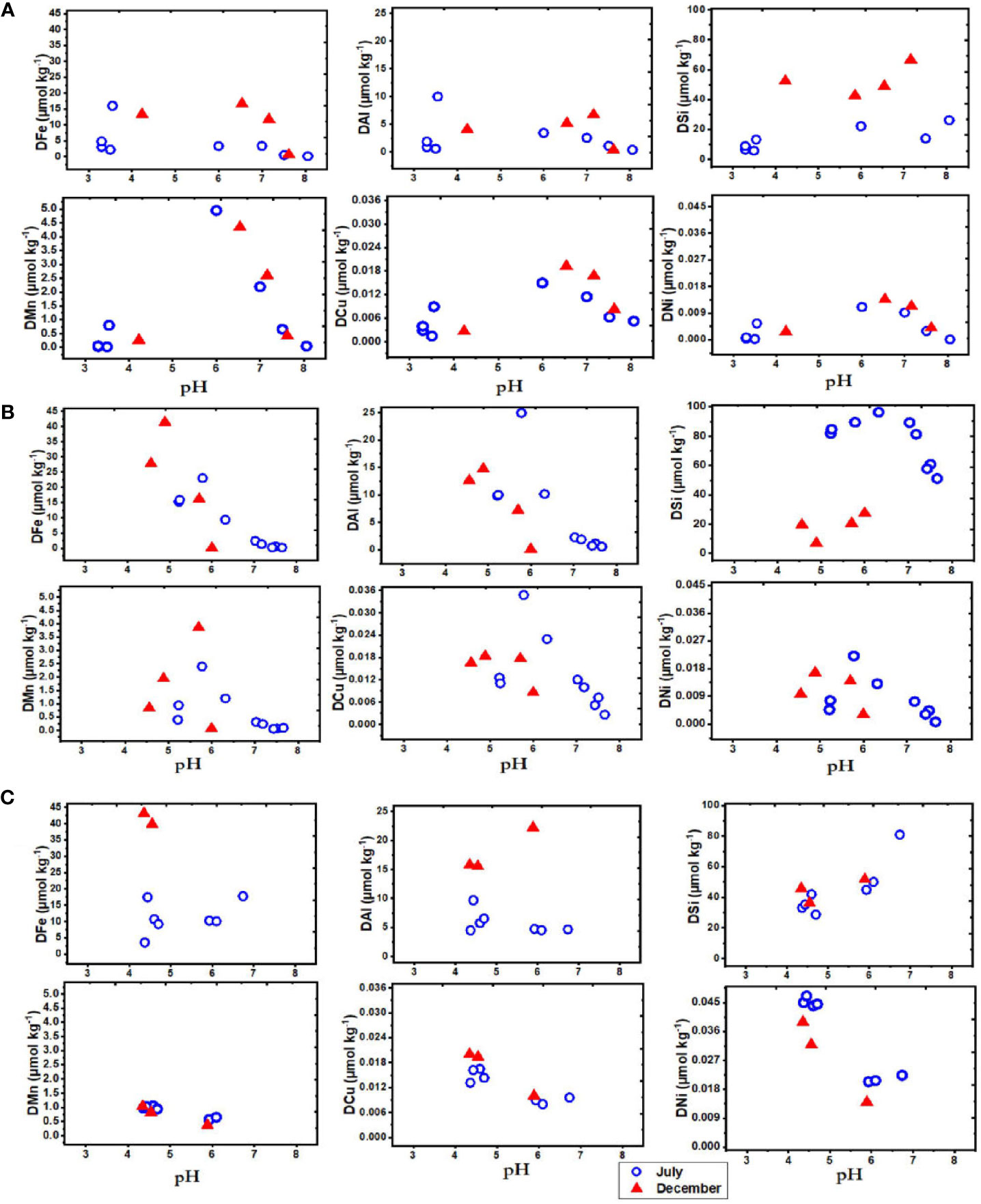
Figure 6 Dissolved elements as a function of pH in the blackwater rivers and estuaries of Borneo in July (dry) and December (wet), 2019. Each plot describes the distribution of elements (Fe, Al, Si, Mn, Cu and Ni) in (A) Maludam (B) Sebuyau (C) Belait rivers and estuaries.
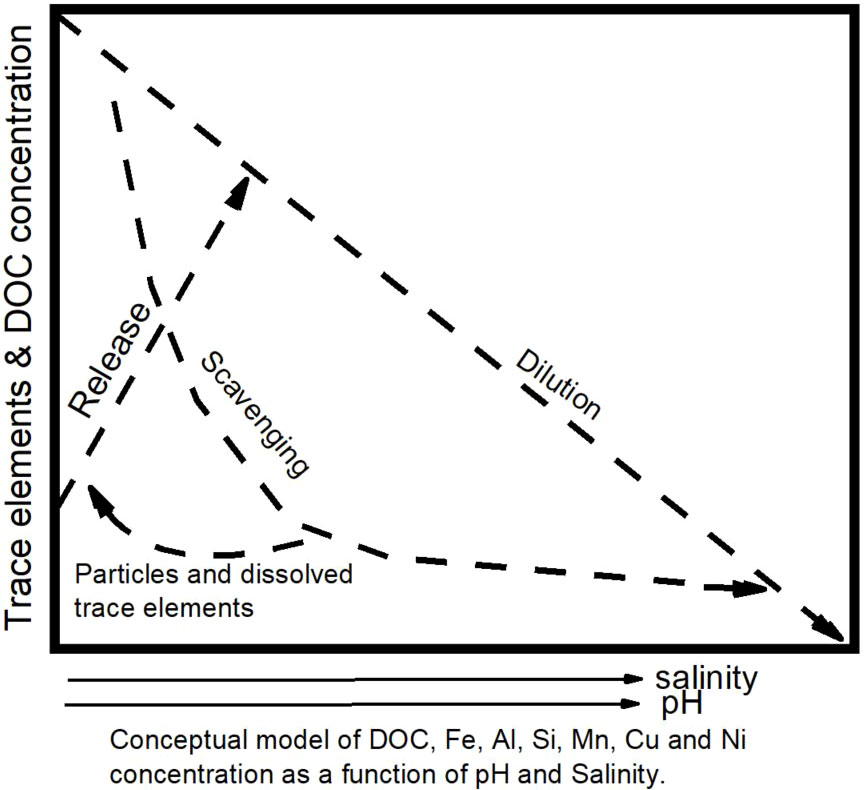
Figure 7 Schematic representation of trace element behaviour in blackwater river and estuarine system.
Figure 7 is a pictorial representation which summarizes the central theme of our findings. It captures the behaviour of trace elements as they travel through the river-estuarine system and the influence of estuarine processes. Trace elements entering the freshwater endmember showed an initial remobilization to the system at low salinity (< 1) and pH (< 5). However, with increasing ionic strength, scavenging and eventual dilution occurred as shown in the schematic representation (Braungardt et al., 2003). Hence, their behaviour was approximately conservative at salinities > 10.
4.5 Modeling of trace element affinity for DOM using Visual MINTEQ
The blackwaters of Borneo rivers and estuaries are rich in DOM, especially at the freshwater endmember, with peak DOC concentrations of 3500 µmol L−1 and 1290 µmol L−1 at the Maludam and Sebuyau, respectively (Figures 2A, B). The role of DOM in mobilizing and transporting trace elements across freshwater and seawater endmembers has been reported (Rothwell et al., 2007) in temperate and tropical ecological settings, but binding of elements to DOM is strongly regulated by pH (Gaillardet et al., 2013). We used the NICA-Donnan model (Milne et al., 2003), to model trace element affinity to DOM in the study area. Figure 8 shows the output of dissolved Fe, Al, Mn, Cu and Ni as a function of pH in Maludam and Sebuyau rivers and estuaries. We calculated 100% Fe and 98-100% Cu bound to DOM in Maludam, and 70-100% Fe and 96-100% Cu bound to DOM in Sebuyau river and estuaries. The percentage of Fe and Cu bound to DOM at pH 3.3 – 6 varied (70-100%), however, at higher pH (> 6) it became stable at 90-100%. This may be due to increased proton concentrations in the freshwater and mid-salinity regions of the river and estuaries. Our field data showed high proton concentrations in the freshwater endmember compared to mid-salinity in Maludam. The percentage of Al, Mn and Ni complexed with organic matter ranged from 0-100%, 1-4% and 0-6% in Maludam; 0-100%, 1-2.5% and 0-23% in Sebuyau, respectively.
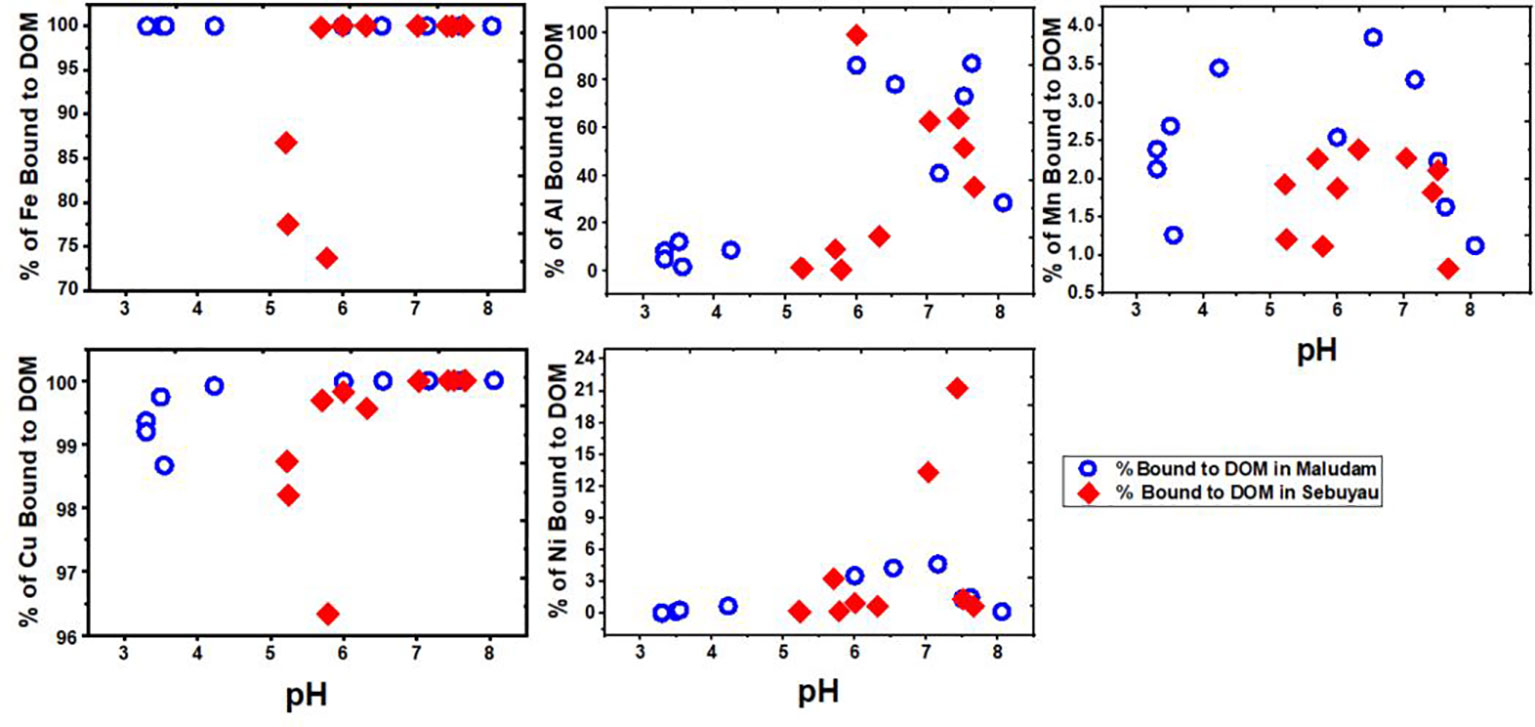
Figure 8 Model results of trace element affinity to DOM as a function of pH in Maludam and Sebuyau rivers and estuaries for dissolved Fe, Al, Mn, Cu and Ni. The output shows the percentage of trace elements bound to DOM according to the NICA-Donnan model.
Tropical peat swamps are a rich source of DOM to adjoining waters which eventually travel to the estuaries. The results of our modeling indicate strong dissolved Fe and Cu association with DOM in Maludam and Sebuyau rivers and estuaries across the pH gradient (Figure 8). The combination of low pH (<5.5) and high DOM therefore hampered the formation of Fe hydroxides with resultant implication for apparent Fe solubility. This supports the assertion that DOM transfer enhances mobilization and transport of trace elements in tropical (Gaillardet et al., 2013) and high-latitude peatlands (Rothwell et al., 2007). The association between trace elements and DOM derived from high-latitude peatlands is thought to influence metal transfer to the Atlantic Ocean (2012; Rothwell et al., 2007; Krachler et al., 2010). At higher salinity and pH, association with Fe oxides may become dominant, leading to co-removal of other trace elements with flocculating colloids (Braungardt et al., 2003).
We modified Fe concentrations to 100 µmol kg−1 in the model to determine the apparent Fe solubility (SFe(III)app) (Zhu et al., 2021). Apparent Fe solubility is defined as the total of Fe(III) bound to DOM and aqueous inorganic Fe(III) species formed at a free (Fe3+) concentration equal to the limiting solubility of Fe hydroxide (Fe(OH)3(s)) (Zhu et al., 2021). Here we used the derived equilibrium constants to calculate SFe(III)app in Maludam and Sebuyau and compare the output with observed DFe concentrations (Figure 9). In Maludam river, the SFe(III)app was higher (100 µmol kg−1) than the observed DFe concentration (< 20 µmol kg−1) at low salinity. However, with increasing salinity (5-25) the values were comparable in the system for both SFe(III)app and observed DFe concentrations, although, there was a slight increase in the observed DFe concentration at salinity 5 and 10 compared to SFe(III)app. The increased SFe(III)app at low salinity region can be attributed to low pH (3.3) and high DOC concentrations (3500 µmol L−1). This was different in Sebuyau river, where at low salinity the observed DFe concentration was higher (> 40 µmol kg−1) compared to SFe(III)app (< 10 µmol kg−1), although similar concentration was observed in the river with increasing salinity (Figure 7). The variation in Sebuyau river can be attributed to increased pH (4.56) and low DOC concentrations (1937 µmol L−1) compared to Maludam river. However, the SFe(III)app and DFe concentrations within the estuarine mixing zones in Maludam and Sebuyau (Figure 8) may be due to other processes that could influence DFe distribution such as redox conditions, scavenging to particles and possibly variation in the nature of the DOM in both rivers (Zhu et al., 2021).
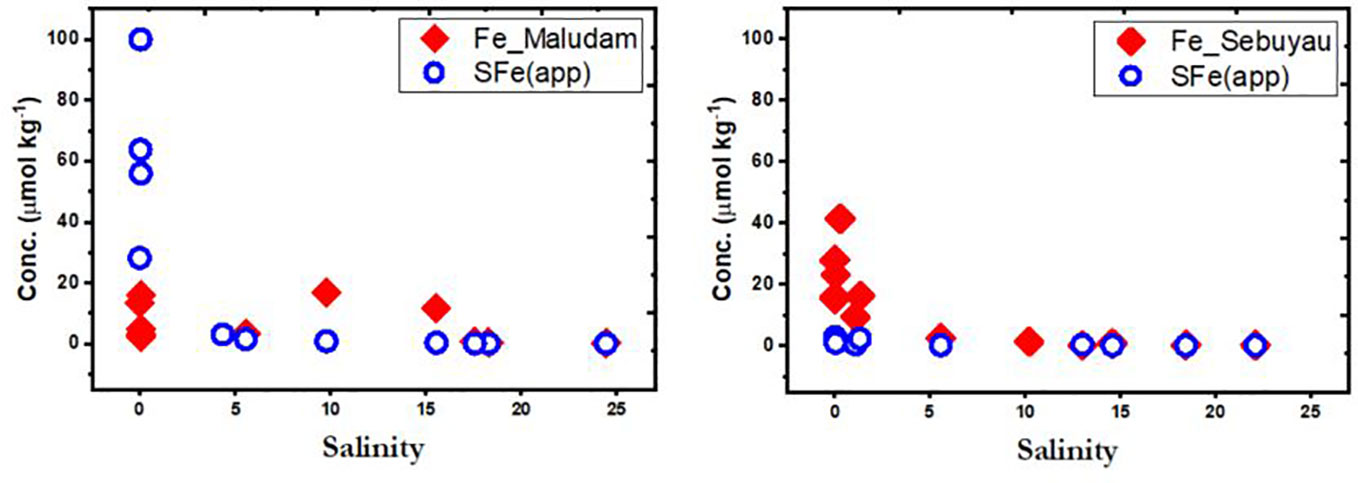
Figure 9 Plots showing Fe concentration and modelled output of Apparent Fe Solubility (SFe(app)) as a function of salinity in Maludam and Sebuyau rivers.
4.6 Comparisons with other peatlands and riverine endmembers within and outside the region
We compared surface water data from different riverine endmembers in southeast Asia and around the world with observations from the present study on the basis of pH as indicated in Table 5. The different rivers of interest range from small peat draining rivers in southeast Asia (e.g., Maludam, Sebuyau and Belait on Borneo Island), tropical rivers in Africa (e.g., Mengong, Nyong, Sanga and Congo Brazzaville), blackwater rivers in North America (e.g., Fraser and Mistassini) and natural rivers in South America (e.g. Amazon river). Reported pH values in the riverine endmember of the different rivers vary from 3.7 to 8.3. In southeast Asia, Maludam river (present study) has a lowest riverine Fe concentration (13.3 µmol kg−1) at pH 4.23 and Curtin lake the maximum Fe (35.2 µmol kg−1) at pH 6.46 (Prasanna et al., 2012). The concentrations of Cu (5.21 µmol kg−1) and Ni (1.62 µmol kg−1) in Sabah river are higher when compared to other rivers in the region and this was attributed to local Cu-rich acid mine drainage (Tashakor et al., 2018). Pahang river shows peak Al (50.3 µmol kg−1) concentration at pH 5.72 (Tashakor et al., 2018) and Mn (12.5 µmol kg−1) at pH 6.4 in Kuala Selangor river (Ishak et al., 2016).

Table 5 Concentration of DOC (µmol L−1) and trace elements from Borneo riverine endmember (Maludam, Sebuyau and Belait) compared to studies in Southeast Asia and different continents (µmol kg−1).
The data in Table 5 provide an insight into the dissolved concentration of trace elements in the different continents. Although the size of the rivers in our study area is comparatively smaller than others, they are natural and black water peat draining rivers like the Mengong in Cameroon (Viers et al., 1997), Negro in South America and Mistassini in Canada (Gaillardet et al., 2013). The DOC data indicate varying concentrations across the riverine endmembers, with Maludam river having the highest of the rivers presented in Table 5 (4600 µmol L−1); the observed peak concentration in Maludam may be due to the pristine nature of the national park (Zhang et al., 2020). The dissolved concentrations of Fe, Al, Mn, Cu and Ni indicate variation across the various natural waters with Curtin lake (Fe), Pahang (Al), Kuala Selangor (Mn), Sabah (Cu) and Sabah (Ni) rivers showing peak concentrations which can be linked to anthropogenic signatures in Southeast Asia (Tashakor et al., 2018). Dissolved Cu and Ni concentrations also show little variation from those obtained in the present study; however, peak concentrations were observed in Sabah for (Cu), (Ni) and lowest concentrations in Maludam (Cu) and Changjiang (Ni) (Gaillardet et al., 2013). The peak concentrations observed in Southeast Asia may be indicative of the effect of the ongoing land use change, although not obvious in the study area due to minimal anthropogenic impact. It may well serve as a pointer to the fact that continuous destruction of peat swamp forests will introduce increasing concentration of trace elements into rivers and estuaries.
5 Conclusion
We used samples collected during 2019 expeditions to investigate the dynamics of trace elements in ombrotrophic tropical peat-draining rivers and estuaries in Sarawak (Maludam and Sebuyau rivers and estuaries), Malaysia and Belait river and estuary in Brunei. We further examined the role of estuarine processes driven by salinity, pH, ionic strength and DOC variability on the behaviour and distribution of trace elements (Fe, Al, Mn, Cu and Ni) in the blackwater rivers and estuaries. Dissolved trace element concentrations were higher in the freshwater endmember of the different rivers in comparison with the values obtained at intermediate salinity in the estuaries. Particulate trace element concentrations were lower in the freshwater endmember, but increased with salinity due to estuarine mixing and increased ionic strength, scavenging and precipitation processes. The rivers are acidic with pH as low as 3.3 and DOC concentrations up to 3500 µmol L−1 in the freshwater endmembers. Seasonal variations played a contributory role in the concentration and distribution of trace elements in the rivers. We observed peak concentration of dissolved Fe during the wet season (December) which can be attributed to the higher water table, resulting in mobilization of Fe from the anoxic peat swamp. In the dry season, the water table is lower, hence the organic bound elements are more important. In both cases the combination of pH and salinity sets the playing field; it determines the behaviour of the different elements. Our modelled output indicated a high proportion of Fe and Cu associated with DOM (about 100%) and to a lower extent Al and Ni at high pH, with Mn showing little association (<5%). This underscores the importance of Fe hydroxides in metal dynamic in the estuaries. pH and DOC influence SFe(III)app and trace element distribution in the rivers and estuaries. Other factors include ionic strength, scavenging, residence time and seawater cations. Comparatively, the trace element concentrations obtained in this study are similar to those reported in other Southeast Asian rivers and in natural and peat draining rivers around the world, despite differences in geology, topography, catchment area and discharge rate.
Data availability statement
The original contributions presented in the study are included in the article/Supplementary Material. Further inquiries can be directed to the corresponding authors.
Author contributions
The first draft of this manuscript was written by [PU-I] and all authors commented on the subsequent versions of the manuscript as well as approved the final manuscript. The study conception and design were done by [PU-I, ZS, MM, MG and EA]. Material preparation [PU-I, ZS and MG], data collection [MM and RS] and analysis [PU-I, ZS, MG, EA, JO and SJ] respectively. All authors contributed to the article and approved the submitted version.
Funding
This study was supported by the Petroleum Technology Development Fund (PTDF) in partnership with the Deutscher Akademischer Austauschdienst (DAAD).
Acknowledgments
Special thanks to Tim Steffens and Dominik Jasinski for analyzing the samples using ICP-MS at GEOMAR and to Matheus Carvalho at SCU for DOC analysis. We are grateful to the Sarawak Forestry Department and Sarawak Biodiversity Centre for permission to conduct collaborative research in Sarawak under permit numbers NPW.907.4.4(Jld.14)-161, SBC-RA-0097-MM, and Park Permit WL83/2017. We further thank the Brunei Forestry Department, for the use and collection permit ([254]/JPH/UND/17 PT.1) in Belait and Universiti Brunei Darussalam for permission to conduct collaborative research; Ref. No. UBD/AVC-RI/1/21/1[a]. We are very grateful to the boatmen who helped us to collect samples and Salwana Md Jaafar for assistance with sampling and logistics support in Brunei.
Conflict of interest
The authors declare that the research was conducted in the absence of any commercial or financial relationships that could be construed as a potential conflict of interest.
Publisher’s note
All claims expressed in this article are solely those of the authors and do not necessarily represent those of their affiliated organizations, or those of the publisher, the editors and the reviewers. Any product that may be evaluated in this article, or claim that may be made by its manufacturer, is not guaranteed or endorsed by the publisher.
Supplementary material
The Supplementary Material for this article can be found online at: https://www.frontiersin.org/articles/10.3389/fmars.2022.1075155/full#supplementary-material
References
Achterberg E. P., Herzl V. M. C., Braungardt C. B., Millward G. E. (2003). Metal behaviour in an estuary polluted by acid mine drainage: The role of particulate matter. Environ. pollut. 121 (2), 283–292. doi: 10.1016/S0269-7491(02)00216-6
Braungardt C. B., Achterberg E. P., Elbaz-Poulichet F., Morley &., N. H. (2003). Metal geochemistry in a mine-polluted estuarine system in Spain. Appl. Geochem. 18 (11), 1757–1771. doi: 10.1016/S0883-2927(03)00079-9
Broder T., Biester H. (2017). Linking major and trace element concentrations in a headwater stream to DOC release and hydrologic conditions in a bog and peaty riparian zone. Appl. Geochem. 87 (November), 188–201. doi: 10.1016/j.apgeochem.2017.11.003
Caccia V. G., Millero F. J. (2003). “The distribution and seasonal variation of dissolved trace metals in Florida bay and adjacent waters,” in Aquatic geochemistry. (Netherlands: Kluwer Academic Publishers), vol. 9.
Cameron E. M., Hall G. E. M., Veizer J., Krouse H. R. (1995). Isotopic and elemental hydrogeochemistry of a major river system: Fraser river, British Columbia, Canada. Chem. Geol. 122 (1–4), 149–169. doi: 10.1016/0009-2541(95)00007-9
Chahal M. K., Shi Z., Flury M. (2016). Nutrient leaching and copper speciation in compost-amended bioretention systems. Sci. Total Environ. 556, 302–309. doi: 10.1016/j.scitotenv.2016.02.125
Chuan G. K. (1993). Hydrological characteristics and water resources. Singapore J. Trop. Geogr. 13 (1), 25–37. doi: 10.1111/j.1467-9493.1992.tb00039.x
Cooper H. V., Vane C. H., Evers S., Aplin P., Girkin N. T., Sjögersten S. (2019). From peat swamp forest to oil palm plantations: The stability of tropical peatland carbon. Geoderma 342 (February), 109–117. doi: 10.1016/j.geoderma.2019.02.021
Dargie G. C., Lewis S. L., Lawson I. T., Mitchard E. T. A., Page S. E., Bocko Y. E., et al. (2017). Age, extent and carbon storage of the central Congo basin peatland complex. Letter Res. Nat. 542, 86–104. doi: 10.1038/nature21048
Deberdt S., Viers J., Dupre B. (2002). New insights about the rare earth elements (REE) mobility in river waters. Bull. Soc Geol. France 173 (2), 147–160. doi: 10.2113/173.2.147
de Carvalho L. M., Hollister A. P., Trindade C., Gledhill M., Koschinsky A. (2021). Distribution and size fractionation of nickel and cobalt species along the Amazon estuary and mixing plume. Mar. Chem. 236 (August), 104019. doi: 10.1016/j.marchem.2021.104019
de Souza Machado A. A., Spencer K., Kloas W., Toffolon M., Zarfl C. (2016). Metal fate and effects in estuaries: A review and conceptual model for better understanding of toxicity. Sci. Total Environ. 541, 268–281. doi: 10.1016/j.scitotenv.2015.09.045
Dhandapani S., Evers S., Ritz K., Sjögersten S. (2020). Nutrient and trace element concentrations influence greenhouse gas emissions from Malaysian tropical peatlands. Soil Use Manage. 742, 1–13. doi: 10.1111/sum.12669
Dupré B., Gaillardet J., Rousseau D., Allègre C. J. (1996). Major and trace elements of river-borne material: The Congo basin. Geochim. Cosmochim. Acta 60 (8), 1301–1321. doi: 10.1016/0016-7037(96)00043-9
Elbaz-Poulichet F., Morley N. H., Cruzado A., Velasquez Z., Achterberg E. P., Braungardt C. B. (1999). Trace metal and nutrient distribution in an extremely low pH (2.5) river-estuarine system, the ria of huelva (South-West Spain). Sci. Total Environ. 227 (1), 73–83. doi: 10.1016/S0048-9697(99)00006-6
Gaillardet J., Viers J., Dupré B. (2003). Trace elements in river waters. Treat. Geochem. 5–9, 225–272. doi: 10.1016/B0-08-043751-6/05165-3
Gaillardet J., Viers J., Dupré B. (2013). “Trace elements in river waters,” in Treatise on geochemistry: Second edition. (Netherlands: Elsevier Ltd), vol. 7. doi: 10.1016/B978-0-08-095975-7.00507-6
Gandois L., Hoyt A. M., Mounier S., Roux G. L., Harvey C. F., Claustres A., et al. (2020). From canals to the coast: Dissolved organic matter and trace metal composition in rivers draining degraded tropical peatlands in Indonesia. Biogeosciences 17 (7), 1897–1909. doi: 10.5194/bg-17-1897-2020
Gledhill M., Hollister A., Seidel M., Zhu K., Achterberg E. P., Dittmar T., et al. (2022). Trace metal stoichiometry of dissolved organic matter in the Amazon plume. Sci. Adv. 8 (31), 1–11. doi: 10.1126/sciadv.abm2249
Graham L. L. B., Giesen W., Page S. E. (2017). “A common-sense approach to tropical peat swamp forest restoration in southeast Asia,” in Restoration ecology, vol. 25. (Washington, D.C. USA: ACS Publications), 312–321. doi: 10.1111/rec.12465
Grasshoff K., Kremling M., Ehrhardt M. (1999). “Methods of seawater analysis,” in Marine chemistry, 3rd ed, vol. 7. (Weinheim: Wiley-VCH). doi: 10.1016/0304-4203(78)90045-2
Harris I., Osborn T. J., Jones P., Lister D. (2020). Version 4 of the CRU TS monthly high-resolution gridded multivariate climate dataset. Sci. Data 7 (1), 1–18. doi: 10.6084/m9.figshare.11980500
Hirano T., Kusin K., Limin S., Osaki M. (2015). Evapotranspiration of tropical peat swamp forests. Global Change Biol. 21 (5), 1914–1927. doi: 10.1111/gcb.12653
Hiscott R. N. (2001). Depositional sequences controlled by high rates of sediment supply, sea-level variations, and growth faulting: The quaternary baram delta of northwestern Borneo. Mar. Geol. 175 (1–4), 67–102. doi: 10.1016/S0025-3227(01)00118-9
Ingri J., Öhlander B., Widerlund A., Andersson P., Land M., Gustafsson Ö. (2000). Temporal variations in the fractionation of the rare earth elements in a boreal river: the role of colloidal particles. Chem. Geol. 166 (1–2), 23–45. doi: 10.1016/S0009-2541(99)00178-3
Ishak A. R., Mohamad S., Soo T. K., Hamid F. S. (2016). Leachate and surface water characterization and heavy metal health risk on cockles in Kuala selangor. Proc. - Soc. Behav. Sci. 222, 263–271. doi: 10.1016/j.sbspro.2016.05.156
Jauhiainen J., Takahashi H., Heikkinen J. E. P., Martikainen P. J., Vasander H. (2005). Carbon fluxes from a tropical peat swamp forest floor. Global Change Biol. 11 (10), 1788–1797. doi: 10.1111/j.1365-2486.2005.001031.x
Jeremiason J. D., Baumann E. I., Sebestyen S. D., Agather A. M., Seelen E. A., Carlson-Stehlin B. J., et al. (2018). Contemporary mobilization of legacy Pb stores by DOM in a Boreal peatland. Environ. Sci. Technol. 52 (6), 3375–3383. doi: 10.1021/acs.est.7b06577
Jiann K. T., Santschi P. H., Presley B. J. (2013). Relationships between geochemical parameters (pH, DOC, SPM, EDTA concentrations) and trace metal (Cd, Co, Cu, fe, Mn, Ni, Pb, zn) concentrations in river waters of Texas (USA). Aquat. Geochem. 19 (2), 173–193. doi: 10.1007/s10498-013-9187-6
Kiew F., Hirata R., Hirano T., Wong G. X., Aeries E. B., Musin K. K., et al. (2018). CO2 balance of a secondary tropical peat swamp forest in Sarawak, Malaysia. Agric. For. Meteorol. 248 (October 2017), 494–501. doi: 10.1016/j.agrformet.2017.10.022
Krachler R., Krachler R. F., von der Kammer F., Süphandag A., Jirsa F., Ayromlou S., et al. (2010). Relevance of peat-draining rivers for the riverine input of dissolved iron into the ocean. Sci. Total Environ. 408 (11), 2402–2408. doi: 10.1016/j.scitotenv.2010.02.018
Krachler R., von der Kammer F., Jirsa F., Süphandag A., Krachler R. F., Plessl C., et al. (2012). Nanoscale lignin particles as sources of dissolved iron to the ocean. Global Biogeochem. Cycles 26 (3), 1–9. doi: 10.1029/2012GB004294
Kumagai T., Saitoh T. M., Sato Y., Takahashi H., Manfroi O. J., Morooka T., et al. (2005). Annual water balance and seasonality of evapotranspiration in a bornean tropical rainforest. Agric. For. Meteorol. 128 (1–2), 81–92. doi: 10.1016/j.agrformet.2004.08.006
Lawlor A. J., Tipping E. (2003). Metals in bulk deposition and surface waters at two upland locations in northern England. Environ. pollut. 121 (2), 153–167. doi: 10.1016/S0269-7491(02)00228-2
Mari X., Torréton J.-P., Trinh C. B.-T., Bouvier T., van Thuoc C., Lefebvre J.-P., et al. (2012). Aggregation dynamics along a salinity gradient in the Bach dang estuary, north Vietnam. Estuarine Coast. Shelf Sci. 96, 151–158. doi: 10.1016/j.ecss.2011.10.028
Martin P., Cherukuru N., Tan A. S. Y., Sanwlani N., Mujahid A., Müller M. (2018). Distribution and cycling of terrigenous dissolved organic carbon in peatland-draining rivers and coastal waters of Sarawak, Borneo. Biogeosciences 15 (22), 6847–6865. doi: 10.5194/bg-15-6847-2018
Mason R. P. (2013). “Trace metals in aquatic systems,” in Trace metals in aquatic systems. USA: Willey-Blackwell Ltd. doi: 10.1002/9781118274576
Millward G. E. (1995). Processes affecting trace element speciationin estuaries: A review. Analyst 120 (3), 609–614. doi: 10.1039/AN9952000609
Milne C. J., Kinniburgh D. G., Tipping E. (2001). Generic NICA-donnan model parameters for proton binding by humic substances. Environ. Sci. Technol. 35 (10), 2049–2059. doi: 10.1021/es000123j
Milne C. J., Kinniburgh D. G., van Riemsdijk W. H., Tipping E. (2003). Generic NICA - donnan model parameters for metal-ion binding by humic substances. Environ. Sci. Technol. 37 (5), 958–971. doi: 10.1021/es0258879
Moore S., Evans C. D., Page S. E., Garnett M. H., Jones T. G., Freeman C., et al. (2013). Deep instability of deforested tropical peatlands revealed by fluvial organic carbon fluxes. Nature 493 (7434), 660–663. doi: 10.1038/nature11818
Morel F. M. M., Milligan A. J., Saito M. A. (2003). Marine bioinorganic chemistry: The role of trace metals in the oceanic cycles of major nutrients. Treat. Geochem. 6–9, 113–143. doi: 10.1016/B0-08-043751-6/06108-9
Mosley L. M., Hunter K. A., Ducker W. A. (2003). Forces between colloid particles in natural waters. Environ. Sci. Technol. 37 (15), 3303–3308. doi: 10.1021/es026216d
Mosley L. M., Liss P. S. (2020). Particle aggregation, pH changes and metal behaviour during estuarine mixing: Review and integration. Mar. Freshw. Res. 71 (3), 300–310. doi: 10.1071/MF19195
Motelay-Massei A., Ollivon D., Tiphagne K., Garban B. (2005). “Atmospheric bulk deposition of trace metals to the seine river basin, France: Concentrations, sources and evolution from 1988 to 2001 in Paris,” in Water, air, and soil pollution. Switzerland: Springer Nature, vol. 164. doi: 10.1007/s11270-005-1659-x
Müller D., Warneke T., Rixen T., Müller M., Jamahari S., Denis N., et al. (2015). Lateral carbon fluxes and CO2 outgassing from a tropical peat-draining river. Biogeosci. Discuss. 12 (13), 10389–10424. doi: 10.5194/bgd-12-10389-2015
Neubauer E., Kammer F. V. D., Hofmann T. (2013). Using FLOWFFF and HPSEC to determine trace metal-colloid associations in wetland runoff. Water Res. 47 (8), 2757–2769. doi: 10.1016/j.watres.2013.02.030
Nurulita Y., Adetutu E. M., Gunawan H., Zul D., Ball A. S. (2016). Restoration of tropical peat soils: The application of soil microbiology for monitoring the success of the restoration process. Agricult. Ecosyst. Environ. 216, 293–303. doi: 10.1016/j.agee.2015.09.031
Oakes J. M., Eyre B. D., Middelburg J. J., Boschker H. T. S. (2010). Composition, production, and loss of carbohydrates in subtropical shallow subtidal sandy sediments: Rapid processing and long-term retention revealed by 13C-labeling. Limnol. Oceanogr. 55 (5), 2126–2138. doi: 10.4319/lo.2010.55.5.2126
Omar M. S., Ifandi E., Sukri R. S., Kalaitzidis S., Christanis K., Lai D. T. C., et al. (2022). Peatlands in southeast Asia: A comprehensive geological review. Earth-Sci. Rev. 232 (August), 104149. doi: 10.1016/j.earscirev.2022.104149
Page S. E., Rieley J. O., Banks C. J. (2011). Global and regional importance of the tropical peatland carbon pool. Global Change Biol. 17, 798–818. doi: 10.1111/j.1365-2486.2010.02279.x
Picouet C., Dupré B., Orange D., Valladon M. (2002). Major and trace element geochemistry in the upper Niger river (Mali): Physical and chemical weathering rates and CO2 consumption. Chem. Geol. 185 (1–2), 93–124. doi: 10.1016/S0009-2541(01)00398-9
Prasanna M. V., Praveena S. M., Chidambaram S., Nagarajan R., Elayaraja A. (2012). Evaluation of water quality pollution indices for heavy metal contamination monitoring: A case study from curtin lake, miri city, East Malaysia. Environ. Earth Sci. 67 (7), 1987–2001. doi: 10.1007/s12665-012-1639-6
Rapp I., Schlosser C., Rusiecka D., Gledhill M., Achterberg E. P. (2017). Automated preconcentration of fe, zn, Cu, Ni, cd, Pb, Co, and Mn in seawater with analysis using high-resolution sector field inductively-coupled plasma mass spectrometry. Anal. Chim. Acta 976, 1–13. doi: 10.1016/j.aca.2017.05.008
Rember R. D., Trefry J. H. (2004). Increased concentrations of dissolved trace metals and organic carbon during snowmelt in rivers of the alaskan arctic. Geochim. Cosmochim. Acta 68 (3), 477–489. doi: 10.1016/S0016-7037(03)00458-7
Rothwell J. J., Evans M. G., Daniels S. M., Allott T. E. H. (2007). Baseflow and stormflow metal concentrations in streams draining contaminated peat moorlands in the peak district national park (UK). J. Hydrol. 341 (1–2), 90–104. doi: 10.1016/j.jhydrol.2007.05.004
Sa’adi Z., Shahid S., Pour S. H., Ahmed K., Chung E. S., Yaseen Z. M. (2020). Multi-variable model output statistics downscaling for the projection of spatio-temporal changes in rainfall of Borneo island. J. Hydro-Environ. Res. 31 (February 2019), 62–75. doi: 10.1016/j.jher.2020.05.002
Sander S., Mosley L. M., Hunter K. A. (2004). Investigation of interparticle forces in natural waters: Effects of adsorbed humic acids on iron oxide and alumina surface properties. Environ. Sci. Technol. 38 (18), 4791–4796. doi: 10.1021/es049602z
Santschi P. H., Lenhart J. J., Honeyman B. D. (1997). Heterogeneous processes affecting trace contaminant, distribution in estuaries: The role of natural organic matter. Mar. Chem. 58 (1–2), 99–125. doi: 10.1016/S0304-4203(97)00029-7
Sholkovitz E. R. (1976). Flocculation of dissolved organic and inorganic matter during the mixing of river water and seawater. Geochim. Cosmochim. Acta 40 (7), 831–845. doi: 10.1016/0016-7037(76)90035-1
Simonsen M., Teien H. C., Lind O. C., Saetra Ø., Albretsen J., Salbu B. (2019). Modeling key processes affecting Al speciation and transport in estuaries. Sci. Total Environ. 687 (0313), 1147–1163. doi: 10.1016/j.scitotenv.2019.05.318
Tang D., Warnken K. W., Santschi P. H. (2002). Distribution and partitioning of trace metals (Cd, Cu, Ni, Pb, zn) in Galveston bay waters. Mar. Chem. 78 (1), 29–45. doi: 10.1016/S0304-4203(02)00007-5
Tashakor M., Modabberi S., van der Ent A., Echevarria G. (2018). Impacts of ultramafic outcrops in peninsular Malaysia and sabah on soil and water quality. Environ. Monit. Assess. 190 (6), 1–19. doi: 10.1007/s10661-018-6668-5
Thanh-Nho N., Strady E., Nhu-Trang T. T., David F., Marchand C. (2018). Trace metals partitioning between particulate and dissolved phases along a tropical mangrove estuary (Can gio, Vietnam). Chemosphere 196, 311–322. doi: 10.1016/j.chemosphere.2017.12.189
Tonks A. J., Aplin P., Beriro D. J., Cooper H., Evers S., Vane C. H., et al. (2017). Impacts of conversion of tropical peat swamp forest to oil palm plantation on peat organic chemistry, physical properties and carbon stocks. Geoderma 289, 36–45. doi: 10.1016/j.geoderma.2016.11.018
Turner A., Millward G. E. (2002). Suspended particles: Their role in estuarine biogeochemical cycles. Estuarine Coast. Shelf Sci. 55 (6), 857–883. doi: 10.1006/ecss.2002.1033
Verwer C., Meer P.v. d. (2010). Carbon pools in tropical peat forest - towards a reference value for forest biomass in relatively undisturbed peat swamp forest in southeast Asia. Natherlands: Alterra Wageningen.
Viers J., Dupré B., Polvé M., Schott J., Dandurand J. L., Braun J. J. (1997). Chemical weathering in the drainage basin of a tropical watershed (Nsimi-zoetele site, cameroon): Comparison between organic-poor and organic-rich waters. Chem. Geol. 140 (3–4), 181–206. doi: 10.1016/S0009-2541(97)00048-X
Weiss D., Shotyk W., Rieley J., Page S., Gloor M., Reese S., et al. (2002). The geochemistry of major and selected trace elements in a forested peat bog, kalimantan, SE Asia, and its implications for past atmospheric dust deposition. Geochim. Cosmochim. Acta 66 (13), 2307–2323. doi: 10.1016/S0016-7037(02)00834-7
Wetlands International (2010). A quick scan of peatlands Vol. 1 (Netherlands: Wetlands International Malaysia), 1–80.
Wetz M. S., Robbins M. C., Paerl H. W. (2009). Transparent exopolymer particles (TEP) in a river-dominated estuary: Spatial-temporal distributions and an assessment of controls upon TEP formation. Estuaries Coast. 32, 447–455. doi: 10.1007/s12237-009-9143-2
Wu Y., Zhu K., Zhang J., Müller M., Jiang S., Mujahid A., et al. (2019). Distribution and degradation of terrestrial organic matter in the sediments of peat-draining rivers, Sarawak, Malaysian Borneo. Biogeosciences 16 (22), 4517–4533. doi: 10.5194/bg-16-4517-2019
Yang T., Chen Y., Zhou S., Li H. (2019). Impacts of aerosol copper on marine phytoplankton: A review. Switzerland: MPDI, Basel. doi: 10.3390/atmos10070414
Zhang X., Müller M., Jiang S., Wu Y., Zhu X., Mujahid A., et al. (2020). Distribution and flux of dissolved iron in the peatland-draining rivers and estuaries of Sarawak, Malaysian Borneo. Biogeosciences 17 (7), 1805–1819. doi: 10.5194/bg-17-1805-2020
Zhou J. L., Rowland S., Fauzi R., Mantoura C., Braven J. (1994). The formation of humic coatings on mineral particles under simulated estuarine conditions-a mechanistic study. Water Res. 28 (3), 571–579. doi: 10.1016/0043-1354(94)90008-6
Zhou Z., Stolpe B., Guo L., Shiller A. M. (2016). Colloidal size spectra, composition and estuarine mixing behavior of DOM in river and estuarine waters of the northern gulf of Mexico. Geochim. Cosmochim. Acta 181, 1–17. doi: 10.1016/j.gca.2016.02.032
Zhu K., Birchill A. J., Milne A., Ussher S., Humphreys M. P., Carr N., et al. (2021). Equilibrium calculations of iron speciation and apparent iron solubility in the celtic Sea at ambient seawater pH using the NICA-donnan model. Mar. Chem. 237, 104038. doi: 10.1016/J.MARCHEM.2021.104038
Keywords: peat swamps, estuaries, blackwater rivers, ombrotrophic, trace elements, Borneo Island
Citation: Ukotije-Ikwut PR, Steiner Z, Gledhill M, Müller M, Oakes JM, Sukri RS, Jiang S and Achterberg EP (2023) The distribution and behaviour of Fe, Al, Si, Mn, Cu and Ni in ombrotrophic tropical peat draining blackwater estuaries on Borneo Island. Front. Mar. Sci. 9:1075155. doi: 10.3389/fmars.2022.1075155
Received: 20 October 2022; Accepted: 16 December 2022;
Published: 09 January 2023.
Edited by:
David C. Podgorski, University of New Orleans, United StatesReviewed by:
Zachary C. Redman, University of Alaska Anchorage, United StatesArindam Sarkar, Bidhan Chandra Krishi Viswavidyalaya, India
Copyright © 2023 Ukotije-Ikwut, Steiner, Gledhill, Müller, Oakes, Sukri, Jiang and Achterberg. This is an open-access article distributed under the terms of the Creative Commons Attribution License (CC BY). The use, distribution or reproduction in other forums is permitted, provided the original author(s) and the copyright owner(s) are credited and that the original publication in this journal is cited, in accordance with accepted academic practice. No use, distribution or reproduction is permitted which does not comply with these terms.
*Correspondence: Peter R. Ukotije-Ikwut, cHVrb3RpamVpa3d1dEBnZW9tYXIuZGU=; cGV0ZXJyb3dsYW5kdUBnbWFpbC5jb20=; Eric P. Achterberg, ZWFjaHRlcmJlcmdAZ2VvbWFyLmRl