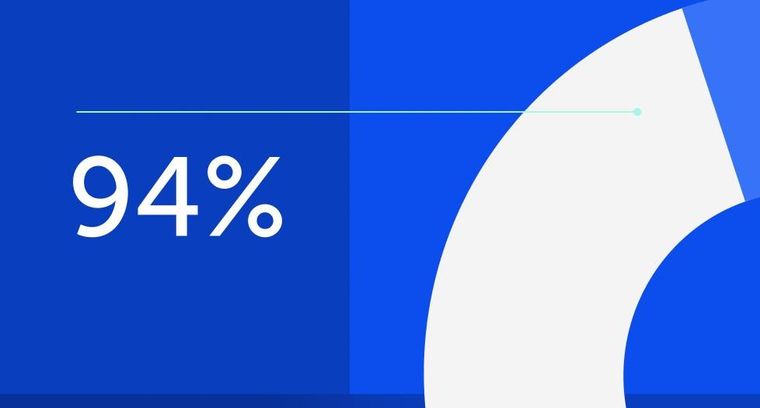
94% of researchers rate our articles as excellent or good
Learn more about the work of our research integrity team to safeguard the quality of each article we publish.
Find out more
ORIGINAL RESEARCH article
Front. Mar. Sci., 09 December 2022
Sec. Marine Biology
Volume 9 - 2022 | https://doi.org/10.3389/fmars.2022.1074816
This article is part of the Research TopicBiological Rhythms of Aquatic Organisms in a Changing ClimateView all 9 articles
The circadian clock is an endogenous regulation mechanism that coordinates biological processes with daily changes, which are regulated by circadian clock genes. Bmal1 and Period are key circadian clock genes and their roles in reproductive development have been widely studied. The spawning time of Sinonovacula constricta is limited to the night even under external artificial stimulation, and it might be regulated by the internal circadian clock. In this study, the heart rate of S. constricta was higher between 20:00-04:00 at night and lower between 12:00-16:00 during the day, and the sex hormone contents were the highest at 00:00 and the lowest at 18:00 (P < 0.01). Therefore, these obvious changes in the circadian rhythm indicate that S. constricta is a nocturnal animal. The open reading frame (ORF) of Bmal1 comprises 1944 bp encoding 647 aa, while the ORF of Period comprises 3111 bp encoding 1036 aa. Bmal1 and Period were both expressed in four tissues, but they had opposite rhythmic expression patterns. Bmal1 expression was higher at 00:00-06:00 and lower at 12:00-18:00, and Period expression was opposite, thereby suggesting that Bmal1 and Period are involved in positive and negative pathways regulated by the circadian clock, respectively. Strong protein fluorescence signals of Bmal1 and Period proteins were observed in mature oocytes, spermatids, hepatocytes, and epithelial cells of siphons. After siRNA interference, the expression of both Bmal1 and Period significantly decreased (P < 0.01), and the sex hormone contents decreased significantly from 3 to 7 days in the siRNA treatment groups (P < 0.01). Therefore Bmal1 and Period may regulate nocturnal spawning by controlling sex hormone secretion. These findings provide a theoretical basis for understanding the molecular mechanism related to spawning, and may facilitate the artificial propagation of mollusks.
The circadian clock is an endogenous timing mechanism that produces a 24 h circadian rhythm, and is self-driven. It is affected/synchronized by light, temperature, food availability, and other environmental factors (Gachon et al., 2004), which regulates various physiological, developmental, reproductive, and metabolic processes (Bass and Takahashi, 2010). The suprachiasmatic nucleus (SCN) in mammals, is the main pacemaker for the circadian clock system, where it plays a leading role in generating, maintaining, and regulating the circadian rhythm. When the light signal is transmitted to the SCN through the hypothalamic retinal tract, the SCN generates a rhythm signal by regulating the periodic oscillation expression of circadian clock genes (Weaver, 1998; Reppert and Weaver, 2002). In addition, the peripheral clock systems (e.g., liver, stomach, kidney, and ovary) can receive rhythm signals from the central nervous system to regulate the body’s physiological activities together with the SCN (Durgan et al., 2005). The main regulators of circadian rhythms are believed to be composed of transcription–translation feedback loops (TTFLs), which drive the periodic expression of circadian clock genes and proteins (Ray et al., 2020). The clock protein is a transcription factor that forms a heterodimer with Bmal1 (or Arntl) protein through the PAS region (a “sticky” region that can attach to other proteins) when the circadian time starts, and it drives the transcription of Period and Cry by combining with the E-box sequence. After some critical posttranslational modifications, including phosphorylation by casein kinases, the mRNA expression levels of Period and Cry increase to a certain extent, and they form heterodimers that translocate to the nucleus where they inhibit the activity of the Bmal1 and Clock enhancer complex and inhibit their own transcription. The interactions between promoting and inhibitory factors result in the changes in the 24 h circadian rhythm (Gallego and Virshup, 2007; Kojima et al., 2011; Sellix, 2015).
Many studies have investigated the roles of circadian clock genes in reproduction. In particular, Bmal1 and Period are regarded as key circadian clock genes, and their roles in reproductive development have been widely studied. In mammals, the rhythmic expression of Bmal1 and Period existed in both the male and female reproductive systems in Rattus norvegicus (Bittman et al., 2003; Alvarez and Sehgal, 2005). Moreover, Bmal1 and Period might play important roles in follicular development, ovulation, and other processes by controlling the production of sex steroid hormones (Ratajczak et al., 2009). In Mus musculus, the lack of Bmal1 caused ovulation and impaired luteinization, which led to infertility (Alvarez et al., 2008). Similarly, knocking out Bmal1 decreased the ovarian weight and ovulation in M. musculus (Boden et al., 2010; Xu et al., 2016). In addition, after injection with chorionic gonadotropin, the expression of Bmal1 increased in mouse ovaries and the maximum value was reached at 16 h after injection (Momoko et al., 2018). Similarly, mutation of the Period gene can reduce the fertility of mice (Pilorz and Steinlechner, 2008). The application of follicle-stimulating hormone (FSH) to stimulate apoptotic ovarian cells increased the rhythm of Period expression in granulosa cells but decreased that in luteal cell due to apoptosis (Chu et al., 2011). In fish, Bmal1 and Period circadian rhythms have also been found in the ovaries of the swordfish Xiphias gladius (Danilo et al., 2020). In addition, mutations of Bmal1 can reduce the levels of sex hormones such as estradiol to affect the reproductive ability of the zebrafish Danio rerio (Wang, 2013; Wang, 2017). In mollusks, previous studies mainly focused on the functions of sex hormones in regulating reproduction, such as in the bay scallop Argopecten irradians (Li et al., 2020), short necked clam Ruditapes philippinarum (Wu, 2019), Fujian oyster Crassostrea angulata (Ni, 2013), and Zhikong scallop Chlamys farreri (Liu et al., 2014). However, the relationships between circadian clock genes and sex hormones have rarely been explored in mollusks.
The razor clam Sinonovacula constricta is an economically important bivalve with fast growth, high yield and short production cycle. Artificial cultivation of this clam has been developed in recent two decades. However, the spawning time for S. constricta is limited to the night (22:00-06:00) even under external artificial stimulation, such as drying in shade, running water stimulation, and shading (Mo, 2008). Therefore, we speculated that spawning might be regulated by the internal circadian clock. In the present study, we detected and analyzed physiological indicators, including the heart rate, sex hormones (estradiol and testosterone), mRNA and protein expression patterns of Bmal1 and Period within 72 h. Small interfering RNA (siRNA) techniques were also used to explore the relationships between clock genes (Bmal1 and Period) and the secretion of sex hormones. Our findings suggest that the circadian clock might be involved in nocturnal spawning of S. constricta, thereby providing a theoretical basis for understanding the molecular mechanism of spawning, and facilitating the artificial breeding of mollusks.
Razor clams were obtained from Ningbo Ocean and Fishery Science and Technology Innovation Base (Ningbo, Zhejiang province, China) in October, which was the reproductive period of S. constricta. Prior to the experiment, one-year-old clams with mature gonads (average shell length = 6.2 ± 0.5 cm, average body weight=19.0 ± 1.5g) were selected for culture in the mud under a light cycle of 12L: 12D for three days. The artificial lights were turned on (the light intensity 359 ± 20 lx), and the light shines directly into the tank from 08:00 to 20:00 as simulating the daytime (12h light, 08:00–20:00), and black clothes were covered on the tank from 20:00 to 08:00 as simulating the nighttime (12 h dark, 20:00–08:00). The water temperature and salinity were maintained at 20 ± 1°C and 20 ± 1 ppt, respectively. The culture water was natural seawater, and was continuously aerated and changed once a day. The razor clams were fed with the live microalgae of Chaetoceros muelleri with the concentration of (2.5 ± 0.2) × 108 cell/L every day.
The razor clams were randomly placed into three tanks under the same experimental conditions. Considering the pre-experiment results and nocturnal spawning of S. constricta, the samples were collected at four time points (00:00, 06:00, 12:00, and 18:00) per day over a period of three days (72 h) (Figure 1A). For each time, it corresponded to three parallel tanks. Twelve individuals (six males and six females) were randomly selected at each time, and their gender was distinguished by aspirating mature oocytes and sperm cells for observation using an optical microscope. In order to explore the molecular mechanisms of nocturnal spawning of S. constricta, four tissues of ovary, testis, hepatopancreas, and siphon were dissected, immediately frozen in liquid nitrogen, and stored at –80°C. Fresh tissues were also fixed in 4% paraformaldehyde, and then kept in 70% alcohol at 4°C.
Figure 1 Circadian rhythm analysis of the physiological indicators in S. constricta. (A) The experimental design of circadian rhythm, the moon represents the nighttime point, the sun represents the daytime point, and the arrow represents the sampling time point. (B) The heart rate changes of female and male clams. (C) The circadian rhythm changes of estradiol and testosterone within 72 h in S. constricta (E2, estradiol; T, testosterone; n=6).
The heart rates of male and female clams were recorded using a non-invasive infrared monitoring method to assess biological heartbeat parameters. Monitoring equipment facilities containing infrared sensors (CNY-70, Newshift®, Portugal), a heartbeat monitor amplifier (AMP-03U, Newshift®, Portugal), PowerLab 8/35 eight-channel research high-speed recording host (Biomart, Australia), and Blu-Tag (Bostik, Australia) were used to acquire the signals. LabChart software was used to record heart activity waves (AD Instruments, Australia). The diurnal changes in heartbeat fluctuations were measured under the conditions of 12 h light: 12 h dark over a period of three days (72h), and the heart rate data were analyzed at intervals of 4 h.
Sex hormone contents (estradiol and testosterone, n=6) were determined with mature gonads (ovary and testis) using enzyme-linked immunosorbent assay kits (Biomart, Ningbo, China). Briefly, 0.1 g of ovary or testis tissue sample was mixed with 0.9 mL of normal saline and mechanically homogenized. Subsequently, the mixture was centrifuged for 10 min at 1,000g and 4°C, and 700 μL of the supernatant was collected and kept at –80°C. After coating, 10 μL of the sample was added to a microtiter plate and incubated at room temperature for 1 h (with sample wells, standard wells, and blank wells), before washing with buffer solution. Next, 100 μL of horseradish peroxidase-labeled detection antibody was mixed with the standard wells and sample wells, before incubating at room temperature for 1 h and then washing. Finally, the reaction was terminated by incubation with tetramethylbenzidine at room temperature in darkness for 15 min. The OD450nm values were recorded with a microplate reader (Tecan, Switzerland) to calculate the sample concentrations.
RNA was collected and prepared using TRIzol reagent (Sangon, Shanghai, China). The quality of RNA was assessed by agarose gel (1.5%) electrophoresis and the RNA concentration was measured with a nucleic acid detector (Nanovue Plus, Thermo Scientific, USA). First-strand cDNA of 5’ and 3’ RACE were synthesized using SMART RACE reagent (Clontech, USA).
The partial coding sequences of Bmal1 and Period were detected in the genome of S. constricta (WSYO00000000.1). The primers for RACE were designed by using Primer Premier 5 (Table 1). PCR amplification was conducted following the instructions in the SMARTer™ RACE cDNA amplification kit (Clontech). PCR was performed in a reaction volume of 25 µL, which contained 18 µL of DEPC water, 2.5 µL of 10 × Advantage 2 PCR buffer, 0.5 µL of 10 mM dNTPs, 0.5 µL of 10 µM primer, 2.5 µL of 10 × Universal Primer A mix (UPM), 0.5 µL of diluted RACE cDNA, and 0.5 µL of 50 × Advantage 2 Polymerase Mix. The 1.0% agarose gels containing the products were purified using gel extraction kits (Tiangen, China). The purified PCR product was ligated with pEasy-T5 for 25 min at 30°C and transformed into Escherichia coli DH5α (Trans, China). The positive plasmid was then screened and sequenced to obtain the full-length sequences. The primers used to confirm the accuracy of Bmal1 and Period cloning and sequencing are shown in Table 1.
EMBL-EBI was used to assemble the cDNA sequences. The open reading frames (ORFs) of Bmal1 and Period cDNA were identified using ORF Finder at the National Center for Biotechnology Information (NCBI, https://www.ncbi.nlm.nih.gov/) website. NCBI CD-search (https://www.ncbi.nlm.nih.gov/Structure/cdd/wrpsb.cgi)was used to analyze functional domains, and the results were visualized by TBtools. Phylogenetic trees were constructed using Mega11.0 software with the maximum likelihood method [a JTT with freqs. model plus gramma distributed (JTT+F+G) for Bmal1, and a JTT with freqs. model plus gramma distributed with invariant Site (JTT+F+G+I) for Period], and Homo sapiens was selected as the out group for Bmal1 and Period. Bootstrap values were determined from 1,000 replicates. All the GenBank accession numbers for sequences are listed in Supplementary Table 1.
Total RNAs from ovary, testis, hepatopancreas, and siphon tissues (n=6) were reverse transcribed into cDNA by using RT-PCR kits (Takara, Japan). The mRNA expression levels of Bmal1 and Period were assessed by qRT-PCR using Cham Q SYBR qPCR Master Mix (Vazyme, Nanjing, China) and primers with the sequences listed in Table 1. The reaction volume of 20 μL used for amplification contained 10 μL of SYBR qPCR Master Mix, 1 μL of each primer (10 μM), and 8 μL of cDNA sample (10 ng/μL). The reaction program was as follows: 95°C for 10 s, followed by 40 cycles at 95°C for 5 s and 60°C for 30 s. The 18S rRNA gene was selected as the housekeeping gene, and the expression levels of Bmal1 and Period were normalized relative to that of 18S rRNA by using the 2–ΔΔCt method.
Western bolts was used to verify the specificity of Bmal1 and Period antibodies (rabbit anti-Bmal1, rabbit anti-Period, produced by HuaBio, China) on the S. constricta. Total proteins of ovary, testis, hepatopancreas, and siphon tissues were extracted using high-efficiency RIPA tissue lysis buffer (Solarbio, Beijing, China), and the protein concentrations were determined by the BSA kit (Thermofisher, China). The protein extract was separated by SDS-PAGE gel, and then the protein glue with target protein was transferred to polyvinylidene difluoride membrane (PVDF) (Sangon, Shanghai, China). The PVDF membranes were blocked for 1.5 h with a blocking solution (10% milk buffer, 5% TBS and 0.1% Tween-20), and then incubated overnight at 4°C with primary antibodies (rabbit anti-Bmal1, rabbit anti-Period, produced by HuaBio, China, 1:500). Subsequently, the membranes were incubated with secondary antibodies (anti-rabbit labeled with biotin HRP, 1:8,000) (Sangon, Shanghai, China) for 1h at room temperature. Finally, the membranes were incubated with ECL luminescent substrate mixture, and the western blots results were observed and photographed using gel imagers (Bio-Rad, USA).
Immunofluorescence analysis was used to analyze the subcellular distribution and circadian expression of Bmal1 or Period protein in S. constricta. To obtain paraffin sections, samples were dehydrated with an ethanol gradient, embedded in paraffin, cut in sections with a thickness of 4 μm, and spread on polylysine-treated glass slides. Before immunofluorescence analysis, the paraffin sections were heated for 2–3 h at 50°C, dewaxed with xylene, and dehydrated using an alcohol gradient. The slices were incubated in citrate buffer for 30 min at 95 °C, washed with phosphate-buffered saline (PBS), and blocked for 1 h at room temperature in blocking fluid containing PBS, 5% bovine serum albumin (BSA), and 0.2% Tween-20. The sections were incubated overnight at 4°C with primary Bmal1 or Period antibody (rabbit anti-Bmal1, rabbit anti-Period, produced by HuaBio, China, 1:400). Next, the sections were washed in PBS supplemented with 5% BSA and 0.2% Tween-20, and incubated with goat anti-rabbit IgG FITC (diluted 1: 200) (Sangon, Shanghai, China) for 1 h at room temperature, before washing again with PBS. Nuclei were stained with 4′, 6-diamidino-2-phenylindole (Beyotime, Shanghai, China). A fluorescence microscope (Nikon Eclipse 80i, Japan) was used to observe fluorescent signals.
The adult clams with mature gonads (average shell length = 6.2 ± 0.5 cm, average body weight=19.0 ± 1.5g) were selected for siRNA experiments and divided into three groups comprising experimental (small interfering RNA for Bmal1 or Period), siRNA-negative control (NC), and blank (DEPC treated water) groups. Subsequently, 20µL siRNA reagent (4000ng, 200 ng/µL), NC (4000ng, 200 ng/µL), or DEPC were injected into the gonadal tissues of the razor clams in each group. Ovary and testis tissues (n=6) were collected from six clams in each group after 0, 1, 3, 5, 7, and 9 day, and stored at –80°C for detecting gene expressions and sex hormone contents. Gene expressions by qRT-PCR (n=6) and sex hormone analysis using enzyme-linked immunosorbent assay kits (n=6) were conducted as the above steps.
Statistical analysis and figure preparation were performed using GraphPad Prism 8 software. Experimental results were expressed as the mean ± standard deviation and differences were detected by one-way analysis of variance. Multiple comparation in the sex hormone contents (n=6), the circadian expression levels of Bmal1 and Period (n=6) and siRNA interference (n=6) were carried out among different time points, and multiple comparation in the tissue expression levels of Bmal1 and Period (n=6) was carried out among different tissues. P<0.05 was considered to indicate a statistically significant difference, and P<0.01 denoted an extremely significant difference.
The heart rate changes in S. constricta were measured over 72 h and they generally varied between 19–26 bpm, with no significant differences between male and female clams. Moreover, the heart rates were higher between 20:00–04:00 and lower between 12:00–16:00, thereby demonstrating that they changed according to a circadian rhythm (Figure 1B).
The estradiol (ovary) contents over 72 h ranged from 600 to 1800 pg/g and the testosterone (testis) contents ranged from 35 to 95 ng/g (Figure 1C). The testosterone contents in the testis were higher than the estradiol contents in the ovaries. In addition, the estradiol and testosterone contents were both highest at 00:00 and lowest at 18:00, and the differences were extremely significant (P < 0.01), thereby demonstrating that the changes followed an obvious circadian rhythm.
The ORF of Bmal1 comprises 1944 bp encoding 647 amino acids (aa) (GenBank accession number: OP779227) and the ORF of Period comprises 3111 bp encoding 1036 aa (GenBank accession number: OP779228). The predicted molecular masses of the Bmal1 and Period proteins are 71.43 kDa and 116.86 kDa, respectively. The phylogenetic trees showed that Bmal1 and Period proteins of S. constricta has closer relationships to other mollusks, and were firstly clustered with mollusks with high bootstrap support, and then clustered with vertebrates species (Figures 2A1, B1). Fifteen protein sequences from S. constricta and other species were selected to analyze the functional domains, and the results showed two functional domains in the Bmal1 protein were found in all of the test species, and the Period protein was predicted to contain ten functional domains, which generally include the PAS domain (Figures 2A2, B2).
Figure 2 Bioinformatics analysis of Bmal1 (A) and Period (B) in S. constricta. (A1 and B1) Phylogenetic trees of Bmal1 (A1) and Period (B1) constructed from S. constricta and other species with the maximum likelihood method (Bmal1: JTT+F+G model, Period: JTT+F+G+I model). (A2 and B2) The functional domain analysis of Bmal1 (A2) and Period (B2) proteins.
The mRNA and protein expression patterns of Bmal1 and Period in ovary, testis, hepatopancreas, and siphon tissues within 72h were analyzed by qRT-PCR and immunofluorescence assays. The qRT-PCR results showed that the Bmal1 gene was highly expressed in hepatopancreas and testis tissues, and higher expression levels of Period were observed in the hepatopancreas and ovaries (P < 0.01, Figure 3A). Furthermore, the expression of Bmal1 in the four test tissues was higher at night from 00:00–06:00 and lower at 12:00-18:00 during the day (Figure 3B). By contrast, the expression pattern of Period was the opposite of that for Bmal1, with higher expression at 12:00–18:00 during the day and lower expression at 00:00-06:00 in the night (Figure 3B). In general, the opposite rhythmic expression pattern in terms of mRNA level was found for Bmal1 and Period.
Figure 3 Expression pattern analysis of Bmal1 and Period in S. constricta. (A) The relative mRNA expression of Bmal1 and Period in four tissues (n=6). (B) Circadian rhythm expression of Bmal1 and Period within 72 h in four tissues (n=6).
In order to confirm specificity of Bmal1 and Period antibodies in S. constricta, western blots were performed with protein extracts of ovary, testis, hepatopancreas, and siphon tissues. As shown in Figure 4A, a single protein with a molecular mass of about 70 kDa, which corresponds well to the expected Bmal1 mass of 71.43 kDa in S. constricta. Meanwhile, the single protein (about 110 kDa) was also found for the Period protein, and the molecular mass was consistent with the expected mass of 116.86 kDa (Figure 4B). Furthermore, there were no protein bands in the control groups (lacking the primary antibody), which strongly indicated that the Bmal1 and Period antibodies could specifically detect Bmal1 and Period protein in S. constricta.
Figure 4 Specificity of Bmal1 (A) and Period (B) antibodies in S. constricta. Control 1, 2, 3, and 4: lacking the primary antibody in ovary, testis, hepatopancreas, siphons tissues, respectively. 1, 2, 3, and 4: incubated with primary antibody in ovary, testis, hepatopancreas, siphons tissues, respectively.
The immunofluorescence assay results obtained for Bmal1 and Period proteins in the four tissues were consistent under the same experimental conditions (Figures 5A, B). Strong fluorescence signals were observed for the Bmal1 and Period proteins in mature oocytes in ovaries, spermatids in testis, hepatocytes in the hepatopancreas, and epithelial cells at the end of siphon (Figures 5A, B). Furthermore, the samples of the four tissues collected at 00:00 and 12:00 were selected to observe differences in the fluorescent signals from the Bmal1 and Period proteins. Intriguingly, the Bmal1 protein signals in the ovary and siphon tissues were stronger at 00:00 than 12:00, while the Period protein signals in the ovary and hepatopancreas tissues were stronger at 12:00 than 00:00 (Figures 5A, B). However, the immunofluorescence assay results were qualitative, so the quantitative rhythmic expression patterns of Bmal1 and Period proteins in S. constricta are required further exploration and clarification in the next step.
Figure 5 Distribution of Bmal1 and Period proteins used immunofluorescence staining in four tissues. (A) Distribution of Bmal1 protein. (B) Distribution of Period protein. MO, Mature oocytes; ST, Spermatid; HC, Hepatocytes; CC, Ciliated column cells; EC, Epithelial cell. Scale bars were 100 μm.
To further verify the relationships between the circadian clock genes Bmal1 and Period and sex hormone secretion, siRNAs for Bmal1 and Period were injected into the mature ovaries and testis. The mRNA expression level of Bmal1 in the ovary was significantly lower in the siRNA group than the NC and DEPC groups from 3 to 5 days (P < 0.01), while the expression level of Bmal1 decreased significantly in the testis at 5 days (P < 0.01, Figure 6A). In the siRNA group of Bmal1, the estradiol (ovary) and testosterone (testis) concentrations decreased significantly from 3 to 7 days (P < 0.01, Figure 6B). From 7 to 9 days, the testosterone concentrations were still significantly lower in the siRNA group than the NC group (P < 0.01, Figure 6B).
Figure 6 Gene expression of Bmal1 and sex hormone secretion after siRNA interference. (A) Relative expression of Bmal1 in the ovary and testis in siRNA group, NC group, and DEPC groups after siRNA interference (n=6). (B) The sex hormone content changes among groups after siRNA interference (E2, estradiol; T, testosterone; n=6). Asterisks indicate significant differences: *P < 0.05, and **P < 0.01.
Compared with the NC group, the expression of Period in the siRNA group decreased significantly from 3 to 5 days in the ovary (P < 0.01), and decreased significantly at 5 days in the testis (P < 0.01, Figure 7A). In addition, compared with the NC group, the estradiol and testosterone concentrations in the siRNA group decreased significantly from 3 to 9 days (P < 0.01, Figure 7B).
Figure 7 Gene expression of Period and sex hormone secretion after siRNA interference. (A) Relative expression of Period in the ovary and testis among groups after siRNA interference (n=6). (B) The sex hormone contents changes among groups after siRNA interference (E2, estradiol; T, testosterone; n=6). Asterisks indicate significant differences: **P < 0.01.
The circadian clock is an endogenous timing mechanism that regulates many behaviors and physiological indicators (e.g., heart rate and sex hormones) in most organisms (Sallam et al., 2016). Physiological parameters are widely used to evaluate the ability of marine mollusks to respond to environmental changes (Dong and Williams, 2011; Liu et al., 2014; Xing et al., 2019). In C. farreri, the circadian rhythm has a significant effect on the heart rate, which increases significantly at night from 00:00–08:00 (Xing et al., 2019). Similarly, in the present study, we found that the heart rate was significantly higher in the night at 20:00–04:00 than at 12:00–16:00 during the day over a 72 h period, thereby indicating that S. constricta is more active at night. Previous studies also showed that sex hormones may be internal factors that induce spawning and the contents of sex hormones are closely related to the reproductive cycle in mollusks. For instance, in C. farreri, the highest contents of estradiol and testosterone occur before spawning (Liu et al., 2014). In the clam Dosinia corrugata, the estradiol and testosterone contents gradually increase during sexual maturity and ovulation, then decreasing after ovulation (Du, 2017). The spawning times for the great ramshorn Planorbarius corneus and river snail Viviparus are delayed when exposed to freshwater containing estradiol (Benstead et al., 2011). Similarly, we found obvious changes in the circadian rhythms in terms of the estradiol and testosterone contents in S. constricta, which were both highest at 00:00 and lowest at 18:00, and the differences were extremely significant (P < 0.01). The contents of sex hormones (estradiol and testosterone) might play important roles in nocturnal spawning of S. constricta. Thus, there were obvious changes in the circadian rhythm in terms of the physiological activities of S. constricta, which might be controlled by the circadian clock and closely related to the circadian clock genes (Hu et al., 2004; Schibler, 2006).
The coordination of biological time is controlled by the circadian timing system, which involves dynamic molecular interactions among the core clock-controlled genes (Karman and Tischkau, 2006). Fluidity and translocation in the nucleus controls the operation of the circadian rhythm through the phosphorylation process in protein degradation (casein kinase and AMPK modification) to form a 24 h circadian cycle (Boden and Kennaway, 2006; Moore et al., 2014). This process involves many circadian clock genes, which are expressed and play important roles in multiple tissues (He and Chen, 2016). Bmal1 and Period are key circadian clock genes, and their roles in reproductive development have been widely studied. In the brain and ovary of Danio rerio, Bmal1 and Period exhibit rhythmic expression patterns under 12 h light: 12 h dark condition (Khan et al., 2016). Similarly, we found that Bmal1 and Period were expressed in the ovary, testis, hepatopancreas, and siphon tissues of S. constricta, which also exhibited rhythmic expression patterns. Furthermore, the opposite expression patterns were found for Bmal1 and Period in the test tissues. In R. norvegicus, Bmal1 and Period have different circadian rhythm expression patterns in the ovaries and SCN, with opposite expression levels (Karman and Tischkau, 2006) in a similar way to S. constricta. Furthermore, in TTFLs, the expression levels of the “positive element” Bmal1 and “secondary element” Period are the opposite (Shearman et al., 2000), thereby indicating that Bmal1 and Period are involved in positive and negative pathways regulated by the circadian clock.
In addition, the fluorescent signals of the Bmal1 and Period proteins were mainly located in the germ and epithelial cells in S. constricta. In mammals, studies have shown that strong immunoreactivity by Bmal1 and Period protein were observed in granulosa and theca cells, as well as corpora lutea in the ovaries (Karman and Tischkau, 2006), and the Bmal1 protein was also located in the germinal vesicle oocytes (Amano et al., 2009), which are similar to our results. Thus, a peripheral circadian clock exists in mollusks, and physiological activities are regulated by circadian clock genes in different tissues.
The ovarian circadian clock is formed by the connection between the autonomic nervous system SCN and ovary (Buijs et al., 1999), and spawning is controlled precisely by the hypothalamic–pituitary–ovarian (HPO) axis (Sellix, 2015). In particular, sex hormones such as luteinizing hormone or follicle-stimulating hormone might be important signals for triggering the ovarian circadian clock to induce spawning (Karman and Tischkau, 2006). However, no previous studies have reported the relationships between the ovarian circadian clock and sex hormones in mollusks. Several studies have shown that the estradiol and testosterone contents increase during gonad development, and the highest levels are reached before spawning in C. farreri (Liu et al., 2014). Estradiol and testosterone can stimulate the maturation of eggs and sperm in the giant scallop Placopecten magellanicus (Wang and Croll, 2004), and estradiol might regulate the occurrence of oocytes in the Chilean ribbed mussel Aulacomya ater (Saavedra et al., 2012), thereby suggesting that sex hormones might play important roles in spawning of mollusks.
Existing research shows that circadian clock proteins with PAS and BHLH domains play important roles in follicular development (Benedict, 2003; Yamada, 2004). In the present study, we found that both Bmal1 and Period proteins contain PAS and BHLH domains, which suggests that they might be involved in spawning of S. constricta. In order to explore the relationships between Bmal1, Period, and sex hormones, we measured the estradiol and testosterone contents after siRNA interference with Bmal1/Period. The results showed that the contents of estradiol and testosterone decreased after siRNA interference. Similarly, in a previous study, knocking out Bmal1 led to a decrease in progesterone secretion in mice (Ratajczak et al., 2009). Bmal1 interference could inhibit the synthesis of progesterone, testosterone, and prostaglandin E2, increase interstitial cell apoptosis, and decrease the expression of key sex hormone synthesis genes (e.g., Ptgs2, Cyp11a1, and Cyp19a1) in mice and zebrafish (Chen et al., 2013; Ding, 2019). Therefore, nocturnal spawning of S. constricta might be related to the ovarian clock genes (Bmal1 and Period) and sex hormone secretion, in other words, Bmal1 and Period could regulate nocturnal spawning by controlling the secretion of sex hormones. However, the molecular mechanisms that allow circadian clock genes to regulate sex hormone secretion require further analysis in mollusks.
The spawning behavior of razor clam S. constricta is restricted to night, which may be involved in the circadian rhythms in terms of its physiological activities and circadian clock genes. The heart rate and sex hormone contents (estradiol and testosterone) were both higher in the nighttime than the daytime, and the changes with the circadian rhythm were obvious, indicating that S. constricta is a nocturnal animal. The circadian clock genes Bmal1 and Period were expressed in all test tissues with the opposite rhythmic expression patterns, and thus they are involved in positive and negative regulatory pathways. The estradiol and testosterone contents decreased after siRNA interference with Bmal1 and Period, thereby suggesting that biological clock genes might regulate nocturnal spawning by controlling the secretion of sex hormones. The specific regulatory mechanisms involved are still not fully elucidated, and require further exploration and clarification.
The datasets presented in this study can be found in online repositories. The names of the repository/repositories and accession number(s) can be found in the article/Supplementary Material.
The animal study was reviewed and approved by the Institutional Animal Care and Use Committee (IACUC) of Zhejiang Wanli University, China.
YD, HY and ZL designed the experiments. YL and QH performed the experiments and analyzed data. YL drafted the manuscript, and HY revised it. All authors contributed to the article and approved the submitted version.
This work was supported by National Natural Science Foundation of China (31902393), Zhejiang Major Program of Science and Technology (2021C02069-7), Ningbo Major Project of Science and Technology (2021Z114), National College Students’ Innovation and Entrepreneurship Training Program (202010876029) and Zhejiang Provincial Top Discipline of Biological Engineering (Level A) (ZS2020009).
We sincerely thank International Science Editing (http://www.internationalscienceediting.com) for editing our manuscript.
The authors declare that the research was conducted in the absence of any commercial or financial relationships that could be construed as a potential conflict of interest.
All claims expressed in this article are solely those of the authors and do not necessarily represent those of their affiliated organizations, or those of the publisher, the editors and the reviewers. Any product that may be evaluated in this article, or claim that may be made by its manufacturer, is not guaranteed or endorsed by the publisher.
The Supplementary Material for this article can be found online at: https://www.frontiersin.org/articles/10.3389/fmars.2022.1074816/full#supplementary-material
Alvarez J. D., Hansen A., Ord T., Bebas P., Chappell P. E., Giebultowicz J. M. (2008). The circadian clock protein Bmal1 is necessary for fertility and proper testosterone production in mice. J. Biol. Rhythm. 23 (1), 26–36. doi: 10.1177/0748730407311254
Alvarez D. J., Sehgal A. (2005). The thymus is similar to the testis in its pattern of circadian clock gene expression. J. Biol. Rhythm. 20 (2), 111–121. doi: 10.1177/0748730404274078
Amano T., Matsushita A., Hatanaka Y., Watanabe T., Oishi K., Ishida N. (2009). Expression and functional analyses of circadian genes in mouse oocytes and preimplantation embryos: Cry1 is involved in the meiotic process independently of circadian clock regulation. Biol. Reprod. 80 (3), 473–483. doi: 10.1095/biolreprod.108.069542
Bass J., Takahashi J. S. (2010). Circadian integration of metabolism and energetics. Science 330 (6009), 1349–1354. doi: 10.1126/science.1195027
Benedict J. C. (2003). Aryl hydrocarbon receptor regulates growth, but not atresia, of mouse preantral and antral follicles. Biol. Reprod. 68 (5), 1511–1517. doi: 10.1095/biolreprod.102.007492
Benstead R. S., Baynes A., Casey D., Routledge E. J., Jobling S. (2011). 17β-oestradiol may prolong reproduction in seasonally breeding freshwater gastropod molluscs. Aquat. Toxicol. 101 (2), 326–334. doi: 10.1016/j.aquatox.2010.11.005
Bittman E. L., Doherty L., Huang L., Paroskie A. (2003). Period gene expression in mouse endocrine tissues. Am. J. Physiol-Reg. I. 285 (3), 561–569. doi: 10.1152/ajpregu.00783.2002
Boden M., Kennaway D. (2006). Circadian rhythms and reproduction. Reproduction 132 (3), 379–392. doi: 10.1530/rep.1.00614
Boden M. J., Varcoe T. J., Voultsios A., Kennaway D. J. (2010). Reproductive biology of female Bmal1 null mice. Reproduction 139 (6), 1077–1090. doi: 10.1530/rep-09-0523
Buijs R. M., Hermes M. H., Kalsbeek A. (1999). The suprachiasmatic nucleus–paraventricular nucleus interactions: a bridge to the neuroendocrine and autonomic nervous system. Prog. Brain Res. 119, 365–382. doi: 10.1016/s0079-6123(08)61581-2
Chen H., Zhao L., Kumazawa M., Yamauchi N., Shigeyoshi Y., Hashimoto S., et al. (2013). Downregulation of core clock gene Bmal1 attenuates expression of progesterone and prostaglandin biosynthesis-related genes in rat luteinizing granulosa cells. Am. J. Physiol-Cell. Ph. 304 (12), 1131–1140. doi: 10.1152/ajpcell.00008.2013
Chu G., Yoshida K., Narahara S., Uchikawa M., Kawamura M., Yamauchi N. (2011). Alterations of circadian clockworks during differentiation and apoptosis of rat ovarian cells. Chronobiol. Int. 28 (6), 477–487. doi: 10.3109/07420528.2011.589933
Danilo B., Giorgia G., Vittoria T., Michela C., Oliana C. (2020). Opsins and gonadal circadian rhythm in the swordfish (Xiphias gladius) ovary: their potential roles in puberty and reproductive seasonality. Gen. Comp. Endocr. 303 (15), 113707. doi: 10.1016/j.ygcen.2020.113707
Ding H. (2019). Effect of Bmal1 on apoptosis and testosterone secretion in mouse leydig cells (Changchun, Jilin: Jilin Agricultural University).
Dong Y. W., Williams G. A. (2011). Variations in cardiac performance and heat shock protein expression to thermal stress in two differently zoned limpets on a tropical rocky shore. Mar. Biol. 158 (6), 1223–1231. doi: 10.1007/s00227-011-1642-6
Du J. (2017). Annual changes of sex hormones in gonads of dosinia corrugate Vol. 21) (Heilongjiang: New Countryside), 136.
Durgan D. J., Hotze M. A., Tomlin T. M., Egbejimi O., Young M. E. (2005). The intrinsic circadian clock within the cardiomyocyte. Am. J. Physiol-Heart. C. 289 (4), 1530–1541. doi: 10.1152/ajpheart.00406.2005
Gachon F., Nagoshi E., Brown S. A., Ripperger J., Schibler U. (2004). The mammalian circadian timing sytem: from gene expression to physiology. Chromosoma 113 (3), 103–112. doi: 10.1007/s00412-004-0296-2
Gallego M., Virshup D. M. (2007). Post-translational modifications regulate the ticking of the circadian clock. Nat. Rev. Mol. Cell Bio. 8 (2), 139–148. doi: 10.1038/nrm2106
He B., Chen Z. (2016). Molecular targets for small-molecule modulators of circadian clocks. Curr. Drug Metab. 17 (5), 503–512. doi: 10.2174/1389200217666160111124439
Hu K., Pch I., Hilton M. F., Chen Z., Ayers R. T., Stanley H. E., et al. (2004). Endogenous circadian rhythm in an index of cardiac vulnerability independent of changes in behavior. P. Natl. Acad. Sci. U.S.A. 101 (52), 18223–18227. doi: 10.1073/pnas.0408243101
Karman B. N., Tischkau S. A. (2006). Circadian clock gene expression in the ovary: effects of luteinizing hormone. Biol. Reprod. 75 (4), 624–632. doi: 10.1095/biolreprod.106.050732
Khan Z. A., Yumnamcha T., Rajiv C., Sanjita Devi H., Mondal G., Devi S. D. (2016). Melatonin biosynthesizing enzyme genes and clock genes in ovary and whole brain of zebrafish (Danio rerio): differential expression and a possible interplay. Gen. Comp. Endocr. 233, 16–31. doi: 10.1016/j.ygcen.2016.05.014
Kojima S., Shingle D. L., Green C. B. (2011). Post-transcriptional control of circadian rhythms. J. Cell Sci. 124 (3), 311–320. doi: 10.1242/jcs.065771
Liu J., Zhang Z., Zhang L., Liu X., Yang D., Ma X. (2014). Variations of estradiol-17β and testosterone levels correlated with gametogenesis in the gonad of zhikong scallop (Chlamys farreri) during annual reproductive cycle. Can. J. Zool. 92 (3), 195–204. doi: 10.1139/cjz-2013-0202
Li W. R., Zhang L. L., Zhang M. W., Li R. J., Li Y. P., Guo Z. Y. (2020). Cloning and expression analysis of gonadotropin-releasing hormone gene in the bay scallop. Periodical. Ocean. Univ. China. 50 (2), 71–77. doi: 10.16441/j.cnki.hdxb.20180430
Mo Y. K. (2008). Industrialized artificial seedling technology in lianyungang Sinonovacula constricta. China Fisheries. 6, 55–55. doi: 10.3969/j.issn.1002-6681.2008.06.036
Momoko K., Kaya W., Ritsuko M., Nozomi A., Akio M., Hitoshi M. (2018). Involvement of the luteinizing hormone surge in the regulation of ovary and oviduct clock gene expression in mice. Genes Cells 23 (8), 649–657. doi: 10.1111/gtc.12605
Moore H. A., David W., Shizufumi E. (2014). Circadian rhythmicity and light sensitivity of the zebrafish brain. PLoS One 9 (1), 86176. doi: 10.1371/journal.pone.0086176
Ni J. B. (2013). Study on reproductive endocrine of fujian oyster (Crassostrea angulata) (Xiamen Fujian: Xiamen University).
Pilorz V., Steinlechner S. (2008). Low reproductive success in Per1 and Per2 mutant mouse females due to accelerated ageing. Reproduction 135 (4), 559–568. doi: 10.1530/rep-07-0434
Ratajczak C. K., Boehle K. L., Muglia L. J. (2009). Impaired steroidogenesis and implantation failure in Bmal1-/- mice. Endocrinology 150 (4), 1879–1885. doi: 10.1210/en.2008-1021
Ray S., Valekunja U. K., Stangherlin A., Howell S. A., Snijders A. P., Damodaran G., et al. (2020). Circadian rhythms in the absence of the clock gene Bmal1. Science 367, 800–806. doi: 10.1126/science.aaw7365
Reppert S. M., Weaver D. R. (2002). Coordination of circadian timing in mammals. Nature 418 (6901), 935–941. doi: 10.1038/nature00965
Saavedra L., Leonardi M., Morin V., Quiones R. A. (2012). Induction of vitellogenin-like lipoproteins in the mussel Aulacomya ater under exposure to 17β-estradiol. Rev. Biol. Mar. Oceanog. 47 (3), 429. doi: 10.4067/S0718-19572012000300006
Sallam E. D. S., Hassan S. A., Hassaneen E., Ali E. M. (2016). Environmental stress of mobile phone EM radiation on locomotor activity and melatonin circadian rhythms of rats: biological rhythm. Biol. Rhythm. Res. 47 (4), 597–607. doi: 10.1080/09291016.2016.1173361
Schibler U. (2006). Circadian time keeping: the daily ups and downs of genes, cells, and organisms. Prog. Brain Res. 153, 271–282. doi: 10.1016/S0079-6123(06)53016-X
Sellix M. T. (2015). Circadian clock function in the mammalian ovary. J. Biol. Rhythm. 30 (1), 7–19. doi: 10.1177/0748730414554222
Shearman L. P., Sriram S., Weaver D. R., Maywood E. S., Chaves I., Zheng B., et al. (2000). Interacting molecular loops in the mammalian circadian clock. Science 288 (5468), 1013. doi: 10.1126/science.288.5468.1013
Wang Q. L. (2013). Zebrafish Bmal1b is essential for circadian regulation and plays role in the reproductive system (Suzhou, Jiangsu: Soochow University).
Wang C., Croll R. P. (2004). Effects of sex steroids on gonadal development and gender determination in the sea scallop, Placopecten magellanicus. Aquaculture 238 (1-4), 483–498. doi: 10.1016/j.aquaculture.2004.05.024
Weaver D. R. (1998). The suprachiasmatic nucleus: a 25-year retrospective. J. Biol. Rhythm. 13 (2), 100–112. doi: 10.1177/074873098128999952
Wu Q. D. (2019). Molecular mechanism of sex differentiation of ruditapes philippinarum under the action of estradiol (Dalian, Liaoning: DaLian Ocean University).
Xing Q., Zhang L. L., Li Y. Q., Zhu X. H. (2019). Development of novel cardiac indices and assessment of factors affecting cardiac activity in a bivalve mollusc Chlamys farreri. Front. Physiol. 10. doi: 10.3389/fphys.2019.00293
Xu J., Li Y., Wang Y., Xu Y., Zhou C. (2016). Loss of Bmal1 decreases oocyte fertilization, early embryo development and implantation potential in female mice. Zygote 1 (5), 760–767. doi: 10.1017/S0967199416000083
Keywords: Sinonovacula constricta, circadian clock, nocturnal spawning, Bmal1, Period
Citation: Liu Y, He Q, Yao H, Lin Z and Dong Y (2022) Circadian clock genes Bmal1 and Period may regulate nocturnal spawning by controlling sex hormone secretion in razor clam Sinonovacula constricta. Front. Mar. Sci. 9:1074816. doi: 10.3389/fmars.2022.1074816
Received: 20 October 2022; Accepted: 25 November 2022;
Published: 09 December 2022.
Edited by:
Xiaolong Gao, Xiamen University, ChinaReviewed by:
Jilin Xu, Ningbo University, ChinaCopyright © 2022 Liu, He, Yao, Lin and Dong. This is an open-access article distributed under the terms of the Creative Commons Attribution License (CC BY). The use, distribution or reproduction in other forums is permitted, provided the original author(s) and the copyright owner(s) are credited and that the original publication in this journal is cited, in accordance with accepted academic practice. No use, distribution or reproduction is permitted which does not comply with these terms.
*Correspondence: Yinghui Dong, ZG9uZ3lpbmdodWkxMThAMTI2LmNvbQ==; Hanhan Yao, eWFvaGFuaGFuMTAyMEAxMjYuY29t
†These authors have contributed equally to this work
Disclaimer: All claims expressed in this article are solely those of the authors and do not necessarily represent those of their affiliated organizations, or those of the publisher, the editors and the reviewers. Any product that may be evaluated in this article or claim that may be made by its manufacturer is not guaranteed or endorsed by the publisher.
Research integrity at Frontiers
Learn more about the work of our research integrity team to safeguard the quality of each article we publish.