- 1School of Ocean, Yantai University, Yantai, China
- 2Yantai Marine Economic Research Institute, Yantai, China
- 3State Key Laboratory of Marine Environmental Science and College of Ocean and Earth Sciences, Xiamen University, Xiamen, China
As a common macroalga living in the intertidal zone, Sargassum thunbergii (Sargassaceae, Phaeophyta) is often exposed to drastic changes in solar photosynthetically active radiation during a diurnal cycle; thus, the potential photosynthetic adaptation processes deserve attention. In this work, we examined the photosynthetic performance and xanthophyll cycle activity of this alga in response to high light (1,200 μmol photons m–2 s–1, the average in-situ light intensity at noon) by using chlorophyll fluorescence and high-performance liquid chromatography (HPLC). On exposure to high light, a rapid decrease in the effective quantum yield of photosystem II (PSII) (Y(II)) occurred, indicating down-regulation of PSII activity; a corresponding increase in non-photochemical quenching (NPQ) indicated the existence of energy-dissipating cycles. After turning off the light, Y(II) gradually increased to 0.7, accompanied by a decrease in NPQ. However, no complete recovery of NPQ was observed, and its value remained at ≈4, even after an 80-min dark treatment. The size of the xanthophyll cycle pigments pool was quantified using HPLC and was found to be ≈16 mol mol−1 Chl a × 100. The activity of the xanthophyll cycle, characterized by a de-epoxidation state (DPS), could reach up to ≈0.5. Such a large pigments pool and rapid accumulation of zeaxanthin may allow S. thunbergii to induce high values of NPQ (≈10). These results were further complemented by inhibitor (dithiothreitol, DTT) and pre-illumination experiments showing that (1) both NPQ and the xanthophyll cycle could be inhibited by DTT, and there was always a strong positive correlation between NPQ and DPS; (2) the previously formed antheraxanthin exhibited a long retention time and a slow epoxidation; and (3) the long retention of antheraxanthin contributed to a rapid accumulation of zeaxanthin. In conclusion, our present study demonstrated that xanthophyll cycle-induced NPQ can significantly protect S. thunbergii from light fluctuations in the intertidal zone.
Introduction
Photoinhibition, characterized by a decrease in photosynthetic efficiency, is often caused when the harvested light energy exceeds the utilization of Calvin cycle and/or other energy-dissipating mechanisms (Powles, 1984; Long et al., 1994). To cope with diurnal-fluctuating light environments and reduce the potential damage caused by the accumulation of excess energy resulting from photosynthesis, photosynthetic organisms have evolved several mechanisms of light acclimation, of which non-photochemical quenching (NPQ) is thought to be the most common and fastest, protecting intertidal algae in their natural environments., NPQ can be separated into different components, depending on the timescale, including high-energy-state quenching, qE; state transitions, qT; and a photoinhibitory component, qI (Goss and Jakob, 2010; Goss and Lepetit, 2015). Of these components of NPQ, qE has been reported to be the fastest and dominant component (Müller et al., 2001). qE, which is significantly dependent on the proton gradient across the thylakoid membrane (ΔpH), induces the protonation of PsbS in higher plants, of LHCSR (light-harvesting complex stress-related) proteins in green algae, and of LHCX1 (light-harvesting complex 1) in diatoms (Li et al., 2004; Peers et al., 2009; Bailleul et al., 2010; Depauw et al., 2012). qT, which is referred to as the slower component of NPQ, depends on the phosphorylation and migration of antenna proteins from photosystem II (PSII) to photosystem I (PSI) (Krause and Jahns, 2004; Finazzi and Minagawa, 2014, and references therein). qI, the slowest component of NPQ, is often thought to be induced by the degradation of the D1 protein (Müller et al., 2001).
Living in the intertidal zone, the in- and outgoing tides would expose macroalgae to highly variable changes in irradiance diurnally. Consequently, they must perform suitable real-time adjustments in their photosynthetic mechanisms to avoid photoinhibition. As multicellular macroalgae, brown algae (Phaeophyceae) mainly inhabit benthic marine communities as keystone members (Amsler and Fairhead, 2005). Previous studies have shown that the extent of NPQ in brown algae is highly variable, and the differences can be found even within one organism (Harker et al., 1999; Rodrigues et al., 2002). For example, in Macrocystis pyrifera, a species that forms aggregations known as kelp forests, NPQ shows high values in the blades that are located near the seawater surface, whereas the blades in deeper seawater regions exhibit a much lower capacity for NPQ (García-Mendoza and Colombo-Pallotta, 2007; Ocampo-Alvarez et al., 2013). This difference has been shown to correlate with the size of the xanthophyll cycle pigment pool (Goss and Lepetit, 2015). Another example is provided by Laminaria species. The fact that susceptibility to photoinhibition is higher in L. abyssalis than in L. digitata is documented to relate to the de-epoxidation capacity of violaxanthin and the reduced size of the xanthophyll cycle pool in L. abyssalis (Rodrigues et al., 2002). Accordingly, xanthophyll cycle-induced NPQ should play an important role in the photoprotection of brown algae against environmental fluctuations.
Like other terrestrial plants and green algae, the xanthophyll cycle in brown algae also exhibits de-epoxidation of violaxanthin to zeaxanthin, with an intermediate pigment, antheraxanthin, formed in saturating light and a back-epoxidation reaction occurring in conditions of low light or darkness (Rodrigues et al., 2002; Goss and Jakob, 2010; Jahns and Holzwarth, 2012). In some brown unicellular algae, e.g., diatoms, xanthophytes, haptophytes, and dinoflagellates, a homologous and simple xanthophyll cycle comprising the one-step conversion of diadinoxanthin to diatoxanthin occurs, known as the diadinoxanthin cycle (Goss et al., 2006; Goss and Jakob, 2010; Brunet and Lavaud, 2010). Although de-epoxidation is 10 times faster than the epoxidation reaction in terrestrial plants (e.g., Hordeum vulgare; Hartel et al., 1996), the de-epoxidation rate is only twofold faster than the epoxidation rate in brown algae (e.g., M. pyrifera in García-Mendoza and Colombo-Pallotta, 2007). However, the large size of the xanthophyll cycle pigments pool in M. pyrifera is suggested to efficiently protect the photosynthetic apparatus from photoinhibition and/or photodamage (García-Mendoza & Colombo-Pallotta, 2007; Ocampo-Alvarez et al., 2013). In addition, the fast NPQ component, i.e., qE, has previously been reported to be lacking in M. pyrifera (Garcia-Mendoza & Ocampo-Alvarez, 2011); thus, the xanthophyll cycle-related NPQ in brown algae deserves to be investigated.
Sargassum thunbergii (Sargassaceae, Phaeophyta), a common species along the northwestern Pacific coast and the northern coast of China, is often used as a foundation species for the restoration of intertidal seaweed beds (Chu et al., 2012; Yu et al., 2012). On the northern coast of China, S. thunbergii occupies mainly rocky shores, and thalli are exposed to considerable variation in incident irradiance resulting from tidally induced changes in water level and the sun’s changing position (Yu et al., 2013). In the present study, changes in photosynthetic traits and xanthophyll cycle of S. thunbergii were measured, aiming to characterize the variability of photosynthetic activity and evaluate the regulation of xanthophyll cycle in response to high light.
Materials and methods
Algal collection and culture
Thalli of Sargassum thunbergii were collected in May and June from the rocky intertidal zone of Zhanqiao (37°31′44″N, 121°26′4″E), Yantai, Shandong Province, China, and transported to the laboratory in a cooled Styrofoam box within 1 h. Following collection, surface epiphytes and attached sediments were removed and only those thalli that appeared healthy, with a light-brown coloration, were selected as the experimental material. A total of 150 individual thalli of homogeneous length of 5 cm were obtained and then placed in Plexiglas aquaria containing filtered natural seawater. The cultural conditions were set as 12 : 12 h light : dark photoperiod with a light intensity of 300 μmol photons m–2 s–1 and 15°C (corresponding to the in situ surface seawater temperature). The seawater was continuously aerated with air and renewed every day. Before the experiments, thalli were precultured for 3 days under the conditions mentioned above. Every fourth blade was stripped from the same position of each thallus and three independent replicates were used for each experiment. The blades chosen for the experiments were of equal size, 1 cm long and 0.5 cm broad.
Chlorophyll fluorescence measurements and analyses
Chlorophyll fluorescence in S. thunbergii was measured with a portable pulse-amplitude-modulated (PAM) fluorometer (Diving-PAM; Walz, Effeltrich, Germany). To mimic the in situ sunlight conditions, the fiberoptic probe of the PAM device was applied, at a 60° angle, to thalli during the measurement of photosynthetic performance. Blades used for chlorophyll fluorescence were dark-adapted for 20 min before measurement. After dark adaptation, a saturating light pulse of intensity >12,000 μmol photons m–2 s–1 (0.8 s) was applied to obtain the minimum fluorescence intensity (Fo) and the maximum fluorescence intensity (Fm). The blades were then exposed to 1,200 μmol photons m–2 s–1 white light, which was provided by a red–blue LED lamp (the color temperature ranging from 5,000 to 6,000 K), for 60 mins and followed by an 80-min recovery under conditions of darkness. During both the high light treatment and recovery, a saturating light pulse of intensity >12,000 μmol photons m–2 s–1 was applied continuously every 5 min to measure the maximum florescence in the light or dark conditions (Fm′) and the steady-state fluorescence (F). The effective quantum yields of PSII (Y(II)) and the non-photochemical quenching (NPQ) were determined as (Fm′ − F)/Fm′ and (Fm − Fm′)/Fm′, respectively. Meanwhile, three blades treated with the same conditions were quickly frozen in liquid nitrogen and stored at –80°C, for later analysis of the xanthophyll cycle.
Xanthophyll cycle measurement and analyses
The xanthophyll cycle was measured by an Agilent 1260 Infinity (high-performance liquid chromatography, HPLC) system equipped with an Agilent Zorbax Eclipse Plus C18 column (4.6 × 150 mm, 5 μm) attached to a Zorbax Eclipse Plus guard column (Agilent Technologies, USA). These measurements were obtained as described by Colombo-Pallotta et al. (2006): the mobile phase consisted of 70% methanol and 30% 28 mM N, N-tetrabutyladipicamide (pH 6.5, solvent A), and methanol (solvent B) was used to gradient elute at a flow rate of 1.0 ml/min. Before measurement, pigments were extracted in acetone by grinding each blade in liquid nitrogen. Pigment peaks were identified and quantified by comparing retention times and absorption spectra with those of authentic standards purchased from Sigma Chemical Company (St. Louis, MO, USA, for zeaxanthin) and ChromaDex (Irvine, CA, USA, for violaxanthin and antheraxanthin). Data on the xanthophyll cycle pigments were normalized to Chl a (mol mol−1 Chl a × 100). As both antheraxanthin and zeaxanthin are involved in the dissipation of energy as heat (Goss et al., 2006), the de-epoxidation state (DPS) of the xanthophyll cycle can be calculated as [zeaxanthin]+0.5[antheraxanthin]/Σ[xanthophyll cycle pigments], where the xanthophyll cycle pigments is the sum of violaxanthin, antheraxanthin, and zeaxanthin.
Inhibitor treatments
Dithiothreitol (DTT), an inhibitor of the enzyme violaxanthin de-epoxidase (VDE), has been applied to determine the relationship between the xanthophyll cycle and NPQ. Before exposing blades to high light conditions, they were incubated in darkness for 30 min with different concentrations of DTT, including 0, 1, 3, and 5 mM. Subsequently, blades were exposed to 1,200 μmol photons m–2 s–1 for 10 min, and the activity of the xanthophyll cycle and NPQ were assessed at 1-min intervals. It is worth noting that the concentration and incubation time used in this study have previously been shown to have no effect on PSII activity (Li et al., 2014).
Pre-illumination treatments
To characterize the function of antheraxanthin and zeaxanthin during the response to high light, a pre-illumination experiment was applied by exposing S. thunbergii to high light (1200 μmol photons m–2 s–1) for 0, 1, 3, 5, 7, 10, 13, and 15 min. Fo and Fm were measured before the illumination following full (i.e., 20-min) dark adaptation. The treated blades were collected and analyzed by HPLC to determine the accumulation of antheraxanthin and zeaxanthin. In addition, several blades that had been pre-illuminated (for 0, 5, 10, or 15 min) were dark adapted for 5 min and then exposed to high light again for another 20 min. During the second exposure to high light, the kinetics of NPQ and xanthophyll cycle pigments were measured at 1-min intervals. Three independent blades were used as replicates for each parameter.
Statistical analysis
All data were analyzed using SPSS for Windows 21. Prior to all statistical analyses, the homogeneity of variances was verified using Levene’s test. One-way ANOVA and t-tests were used to establish differences among treatments. To evaluate the t1/2 of Y(II), NPQ, and the xanthophyll cycle, the kinetics of NPQ and xanthophyll cycle pigments during exposure and recovery periods were fitted using the equation y = A1exp(–t/τ) + y0, where A1 represents the amplitude, τ represents the lifetime of the first kinetic component, and y0 represents the amplitude of the second component (Ocampo-Alvarez et al., 2013). The rate constant (k) of NPQ relaxation was calculated from NPQ(t) = NPQm+(NPQ0 – NPQm)exp(–kNPQt), where NPQ0 and NPQm are the values measured immediately before the start of the high-light period and after the de-epoxidation of zeaxanthin, and kNPQ is the rate constant of NPQ increase (see Serôdio et al., 2005). De-epoxidation and epoxidation of pigments were calculated as [zeaxanthin] + 0.5[antheraxanthin]/Σ[xanthophyll cycle pigments] (Goss et al., 2006). Fits were performed using the non-linear curve fit procedure by Origin 2018 (OriginLab, Northampton, United States). Differences were statistically significant if the p-value was less than 0.05.
Results
On exposure of S. thunbergii to high light, Y(II) decreased from 0.68 to 0.15 within 1 h, and the t1/2 was calculated to be 7.02 min (Figure 1A). In contrast, NPQ increased to a maximum of approximately 10, with a t1/2 of 6.4 min (Figure 1B). Y(II) gradually increased during recovery in darkness and was completely recovered after 80 min (Figure 1A), whereas relaxation of NPQ was incomplete and reached a value of 4 (Figure 1B). The rate constant (k) of NPQ relaxation was calculated to be 0.04 min–1.
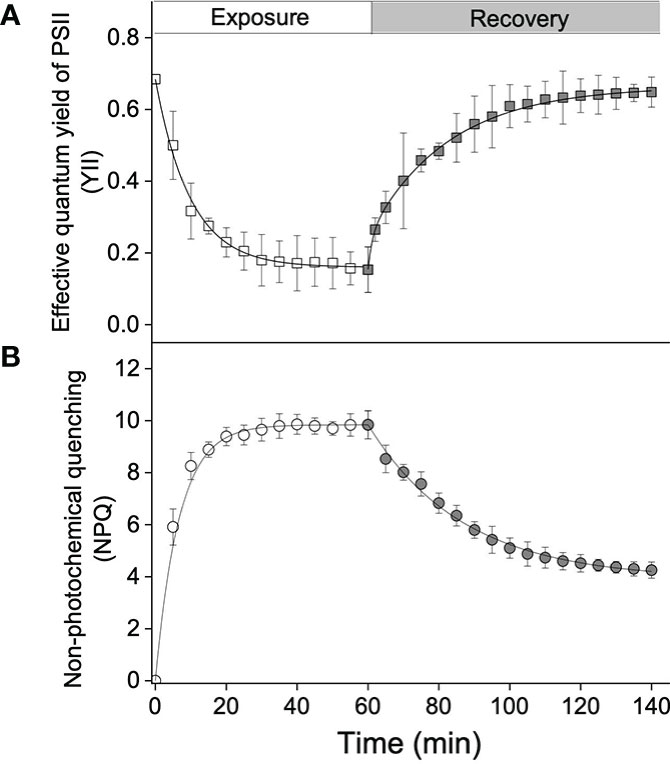
Figure 1 Kinetics of the effective quantum yield of photosystem II (PSII) (A) (Y(II)) and (B) non-photochemical quenching (NPQ), in Sargassum thunbergii during high light exposure for 60 min (1,200 μmol photons m–2 s–1, white points) followed by an 80-min recovery (darkness, gray points). Each curve represents the fit (all R2-values > 0.95) to the model of Ocampo-Alvarez and García-Mendoza (2013). Data represent the mean ± SD (n = 3).
In terms of the xanthophyll cycle, the calculated pigments pool size, i.e., the sum of the concentrations of violaxanthin, antheraxanthin, and zeaxanthin, could reach ≈16 mol mol−1 Chl a × 100. During high light exposure, the concentration of violaxanthin significantly decreased, from 16.3 to 6.8 mol mol−1 Chl a × 100 after 60 min (t1/2 reached ≈6 min; Figure 2A), whereas the concentrations of antheraxanthin and zeaxanthin significantly increased (Figure 2B, C). Compared with the rapid accumulation of antheraxanthin (with a maximum concentration of 3.4 mol mol−1 Chl a × 100 and a t1/2 of ≈2 min), the t1/2 of zeaxanthin formation (≈8 min) was quite low, but the maximum concentration reached 6.2 mol mol−1 Chl a × 100. The calculated de-epoxidation state (DPS) increased from 0.02 to 0.51 (Figure 2D) with an induction t1/2 of around 6 min. After turning off the light, an increase in violaxanthin concentration was observed, accompanied by a decrease in antheraxanthin and zeaxanthin concentrations (Figures 2A–C). After an 80-min recovery in darkness conditions, the concentration of violaxanthin remained ≈11.1 mol mol−1 Chl a × 100, accounting for 71% of the xanthophyll cycle pigments pool, while the concentrations of antheraxanthin and zeaxanthin were 2.1 and 3.2 mol mol−1 Chl a × 100, respectively (Figures 2A–C). DPS was calculated to decrease from 0.51 to 0.3, with a relaxation rate of 0.04 min–1 (Figure 2D).
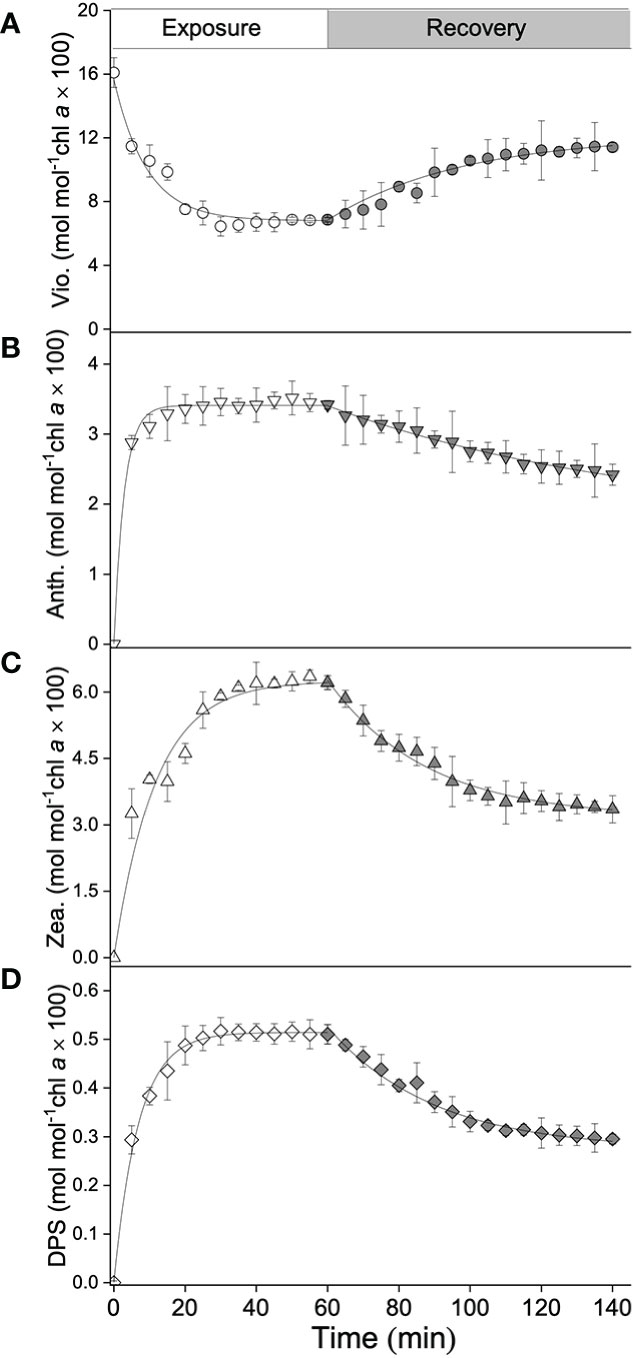
Figure 2 Kinetics of xanthophyll cycle pigments of S. thunbergii, including (A) violaxanthin (Vio., mol mol–1 Chl a × 100), (B) antheraxanthin (Anth., mol mol–1 Chl a × 100), (C) zeaxanthin (Zea., mol mol–1 Chl a × 100), and (D) the de-epoxidation state of the xanthophyll cycle (DPS, mol mol–1 Chl a × 100), during high light exposure for 60 min (1,200 μmol photons m–2 s–1, white points) followed by an 80-min recovery (darkness, gray points). Each curve represents the fit (all R2-values > 0.95) to the model of Ocampo-Alvarez and García-Mendoza (2013). Data represent the mean ± SD (n = 3).
With the addition of DTT at an increasing concentration, the xanthophyll cycle activity of S. thunbergii was gradually impaired. Exposure to high light for 10 min resulted in a significant decrease in Y(II), from 0.32 (control, 0 mM) to 0.2 (1 mM), 0.15 (3 mM), and 0.13 (5 mM), and a corresponding decrease in NPQ, from 8.3 (control, 0 mM) to 2.0 (1 mM), 0.9 (3m M), and 0.4 (5 mM) (Figure 3A). Linear regression analysis showed that the high light-induced NPQ was significantly dependent on DPS (Figure 3B, p<0.05, R2 = 0.98).
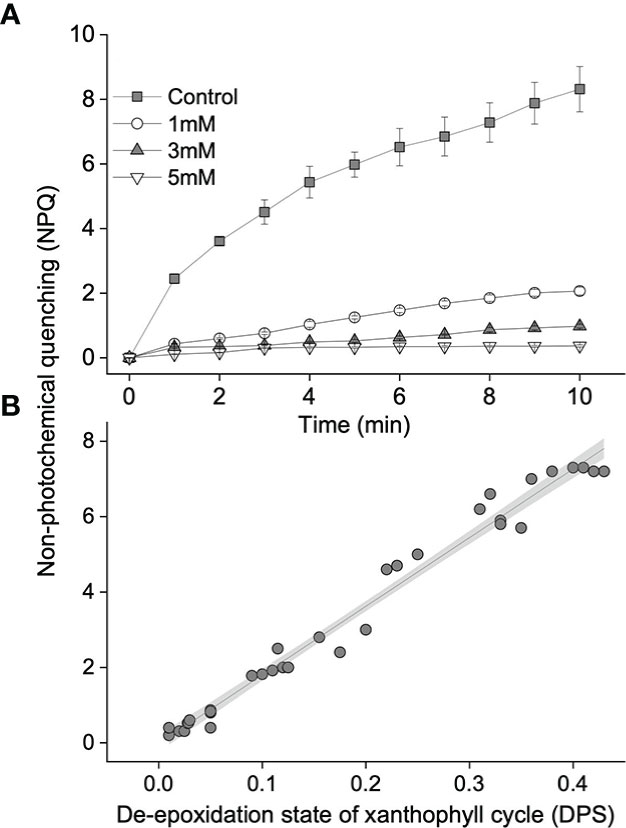
Figure 3 (A) Values of non-photochemical quenching (NPQ) in blades treated with different concentrations of dithiothreitol (DTT) during a 10-min high light exposure (1,200 μmol photons m–2 s–1). Gray squares, white circles, and gray or white tringles represent control (0 mM DTT), 1, 3, and 5 mM DTT, respectively. (B) Relationship between NPQ and the de-epoxidation state of the xanthophyll cycle (DPS). The solid line and gray shadow represent the fit of data to a linear model (p < 0.01 and R2 = 0.99; n = 33) and the 95% confidence interval, respectively. The data shown in A represent the mean ± SD (n = 3).
To further investigate the function of the xanthophyll cycle in S. thunbergii to NPQ, a pre-illumination experiment was carried out. As shown in Figure 4, accumulation of zeaxanthin was positively related to the pre-illumination time (Figure 4A), while the relationship between antheraxanthin and pre-illumination time followed an exponential relationship (Figure 4B). In terms of NPQ, the longer pre-illuminated blades always exhibited a rapid induction of NPQ during the second time high light exposure (Figure 5). The calculated t1/2 of the 15-min pre-illumination was only 2.6 min, which was five times quicker than that of a 0- min pre-illumination (13.1 min). Combined with the data obtained from the pre-illumination experiment, the half-time of NPQ induction was found to be negatively related to the concentration of zeaxanthin (Figure 6A; p<0.05, R2 = 0.98), and there was a significant exponential relationship between the half-time of zeaxanthin accumulation and the concentration of antheraxanthin (Figure 6B, p<0.05).
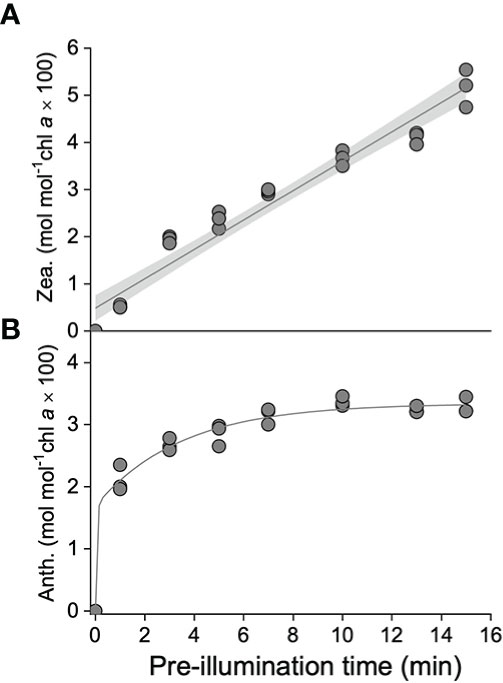
Figure 4 Concentrations of (A) zeaxanthin (Zea., mol mol–1 Chl a × 100) and (B) antheraxanthin (Anth., mol mol–1 Chl a × 100) during the pre-illumination time (1200 μmol photons m–2 s–1). The solid line and gray shadow in A represent the fit of data to a linear model (p < 0.01 and R2 = 0.99; n = 24) and the 95% confidence interval, respectively. The solid line in B represents the fit of data to an exponential model (p < 0.01 and R2 = 0.99, n = 24).
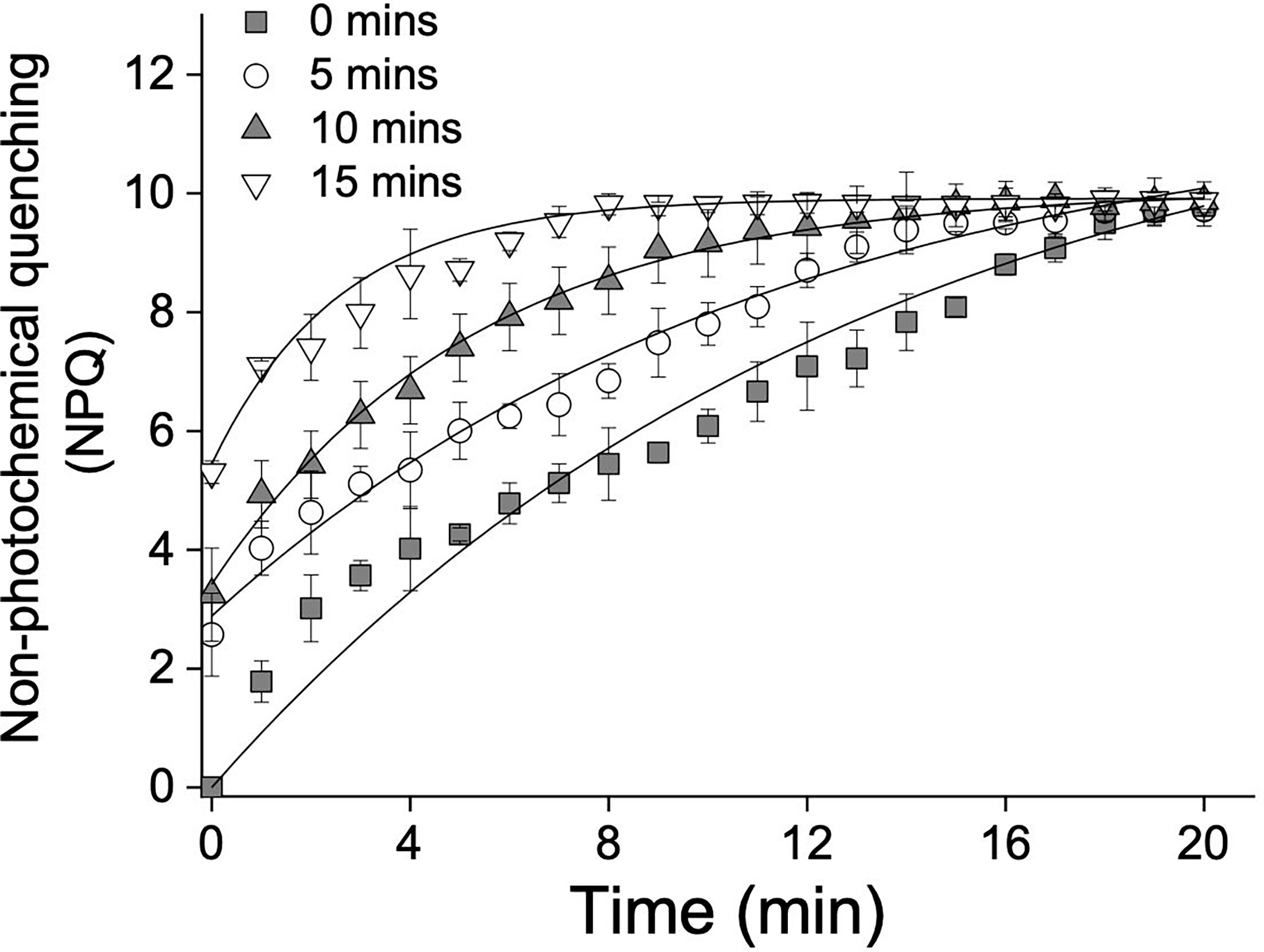
Figure 5 High light (1200 μmol photons m–2 s–1)-induced non-photochemical quenching (NPQ) after 0 min (gray squares), 5 min (white circles), 10 min (gray tringles), and 15 min (white tringles) pre-illumination. Each curve represents the fit (all p < 0.01 and all R2 > 0.95) to the model of Ocampo-Alvarez and García-Mendoza (2013). Data represent the mean ± SD (n = 3).
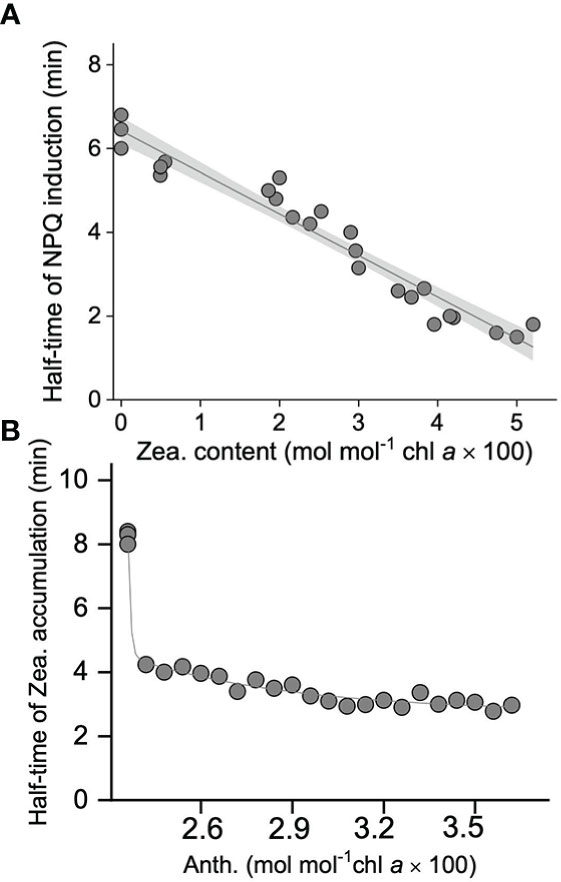
Figure 6 (A) Correlation between the half-time of non-photochemical quenching (NPQ) (min) and retained zeaxanthin content (Zea., mol mol–1 Chl a × 100). (B) Correlation between the half-time of zeaxanthin accumulation (min) and retained antheraxanthin content (Anth., mol mol–1 Chl a × 100). All these data are collected from the high light treatments (1200 umol photons m-2 s-1) with pre-illuminated blades of S. thunbergii. The solid line and gray shadow in A represent the fit of data to a linear model (p < 0.01 and R2 = 0.97; n = 24) and the 95% confidence interval, respectively. The solid line in B represents the fit of data to an exponential model (p < 0.01 and R2 = 0.97, n = 24).
Discussion
In our present study, a significant decrease in the effective quantum yield (Y(II)) in response to high light indicated a down-regulation of photosystem II (PSII) (Figure 1A), while the reversible change in Y(II) is a sign that energy-dissipating mechanisms are highly efficient in protecting Sargassum thunbergii from photoinhibition. Non-photochemical quenching (NPQ), a process in which excess absorbed light energy is dissipated as heat, has been recognized as the first line of biochemical defense against photodamage (Adams and Demmig-Adams, 1994; Ruban, 2016). In this study, in S. thunbergii, we also found an increase in NPQ with exposure to high light, suggesting light-activated photoprotection around PSII (Figure 1B). Following high light exposure, Y(II) gradually recovered to ≈0.68 within 80 min, but NPQ remained at ≈4, which differs from the rapid and full recovery of NPQ that was observed in the seagrass Zostera marina (Yang et al., 2017) and the green alga Ulva prolifera (Zhao et al., 2019). In several evergreens, such sustained energy dissipation has been recognized as qZ, which has been shown to be dependent on zeaxanthin, and critical for maintaining the balance between light absorption and utilization in winter (Ottander and Öquist, 1991; Verhoeven et al., 1998; Verhoeven, 2014). Similarly, the observed sustained NPQ in S. thunbergii is also thought to correlate with the level of zeaxanthin.
The xanthophyll cycle, including the processes of both de-epoxidation of violaxanthin and epoxidation of zeaxanthin, with the production of an intermediate pigment, antheraxanthin, has previously been reported to play an important role in different algal species in their natural environments (Goss and Jakob, 2010, and references therein; Goss and Lepetit, 2015). In our present study, xanthophyll cycle activity was rapidly induced by high light, characterized by a decrease in violaxanthin concentration and accumulation of antheraxanthin and zeaxanthin, as well as a significant increase in de-epoxidation state (DPS) (Figure 2). As documented, the function of the xanthophyll cycle in photoprotection depends mainly on the formation of zeaxanthin, which can (1) interact directly with the Chl a molecule in the excited state and dissipate the energy as heat (Josue and Frank, 2002; Holt et al., 2005); and (2) enhance light-harvesting complex II (LHCII) aggregation and indirectly transform the antenna system of PSII into a state that efficiently dissipates excess excitation energy (Ruban et al., 1997; Horton and Ruban, 2005; Horton et al., 2008). In S. thunbergii, accumulation of zeaxanthin during the previous high light treatment was considered to be responsible for the sustained NPQ. A similar finding has been reported in the brown alga M. pyrifera (Garcia-Mendoza and Ocampo-Alvarez, 2011). As mentioned above, the ratio between violaxanthin de-epoxidation and zeaxanthin epoxidation in brown algae was lower than that of terrestrial plants (Jahns, 1995; Hartel et al., 1996; García-Mendoza and Colombo-Pallotta, 2007 and references therein); however, the observed size of the xanthophyll cycle pigments pool in brown algae [i.e., ≈25 mol mol−1 Chl a × 100 in M. pyrifera (García-Mendoza & Colombo-Pallotta, 2007) and ≈16 mol mol−1 Chl a × 100 in S. thunbergii (present study)] was much larger than that of terrestrial plants (≈8.8 mol mol−1 Chl a × 100 for pea in Jahns, 1995). The larger xanthophyll cycle pigments pool, i.e., a high concentration of violaxanthin under darkness conditions, promotes zeaxanthin accumulation at the onset of illumination, which compensates for the lower ratio of violaxanthin de-epoxidation to zeaxanthin epoxidation and may endow brown algae with a high photoprotective capacity.
To confirm the conclusion that high light-induced NPQ is highly related to the xanthophyll cycle, inhibitor and pre-illumination experiments were carried out. Dithiothreitol (DTT), an inhibitor of the enzyme violaxanthin de-epoxidase (VDE), is widely used to characterize the role of the xanthophyll cycle in photoprotection (Demmig-Adams et al., 1990; García-Mendoza and Colombo-Pallotta, 2007; Li et al., 2014). In our present study, both NPQ and the xanthophyll cycle in S. thunbergii were found to be DTT sensitive; in fact, 5 mM DTT could totally block (≈95%) induction of NPQ and the formation of zeaxanthin (Figure 3A). The observed positive linear relationship between NPQ and DPS indicated that high light-induced NPQ was strongly dependent on the formation of zeaxanthin (Figure 3B). In pre-illumination experiments, zeaxanthin accumulation was correlated with pre-illumination time, while antheraxanthin concentration increased rapidly on initial pre-illumination and remained high (≈3 mol mol−1 Chl a × 100) after 3 min (Figure 4). When the pre-illuminated blades were exposed to high light again, the fastest induction of NPQ was found in those blades that were pre-illuminated for 15 min, with a t1/2 of only 2.6 min (Figure 5). The negative correlation relationship between the t1/2 of NPQ induction and the concentration of zeaxanthin (Figure 6A) confirmed that the zeaxanthin accumulated during pre-illumination could effectively protect S. thunbergii from high light-induced photoinhibition. The t1/2 of zeaxanthin accumulation (Figure 6B) was found to be correlated to the concentration of antheraxanthin, implying that the sustained antheraxanthin may also contribute to the rapid induction of NPQ. Such a typical response has also been observed in M. pyrifera and Chlorella vulgaris (Goss et al., 2006; Ocampo-Alvarez et al., 2013), but in S. thunbergii, a slower epoxidation of antheraxanthin and larger retention (≈70%) were observed. These findings suggest that S. thunbergii is endowed with a higher capacity for photoprotection in response to drastic light fluctuations in the intertidal zone.
In conclusion, our present study demonstrates that (1) the sustained NPQ in S. thunbergii under darkness conditions is highly related to the presence of zeaxanthin formed during the previous high light treatment; (2) the rapid formation and slow epoxidation of antheraxanthin are prerequisites for the rapid accumulation of zeaxanthin; and (3) xanthophyll cycle-induced NPQ could significantly protect S. thunbergii from high light.
Data availability statement
The raw data supporting the conclusions of this article will be made available by the authors, without reservation.
Author contributions
G-NN and X-QZ: data collection and curation, data analysis, and writing the draft. MXZ: data analysis and formal analysis. Q-SZ, Z-MH, and R-PH: writing—review and editing. DZ: conceptualization, data collection and curation, data analysis, visualization, and writing—original draft, and review and editing. All authors contributed to the article and approved the submitted version.
Funding
This study was supported by start-up funds from Yantai University (HX22B98).
Conflict of interest
The authors declare that the research was conducted in the absence of any commercial or financial relationships that could be construed as a potential conflict of interest.
Publisher’s note
All claims expressed in this article are solely those of the authors and do not necessarily represent those of their affiliated organizations, or those of the publisher, the editors and the reviewers. Any product that may be evaluated in this article, or claim that may be made by its manufacturer, is not guaranteed or endorsed by the publisher.
References
Adams III, W. W., Demmig-Adams B. (1994). Carotenoid composition and down regulation of photosystem II in three conifer species during the winter. Physiologia Plantarum. 92 (3), 451–458 doi: 10.1111/j.1399-3054.1994.tb08835.x
Amsler C. D., Fairhead V. A. (2005). Defensive and sensory chemical ecology of brown algae. Adv. Botanical Res. 43, 1–91. doi: 10.1016/S0065-2296(05)43001-3
Bailleul B., Rogato A., De Martino A., Coesel S., Cardol P., Bowler C., et al. (2010). An atypical member of the light-harvesting complex stress-related protein family modulates diatom responses to light. Proc. Natl. Acad. Sci. 107 (42), 18214–18219. doi: 10.1073/pnas.1007703107
Brunet C., Lavaud J. (2010). Can the xanthophyll cycle help extract the essence of the microalgal functional response to a variable light environment? J. Plankton Res. 32 (12), 1609–1617. doi: 10.1093/plankt/fbq104
Chu S. H., Zhang Q. S., Tang Y. Z., Zhang S. B., Lu Z. C., Yu Y. Q. (2012). High tolerance to fluctuating salinity allows Sargassum thunbergii germlings to survive and grow in artificial habitat of full immersion in intertidal zone. J. Exp. Mar. Biol. Ecol. 412, 66–71. doi: 10.1016/j.jembe.2011.10.025
Colombo-Pallotta M. F., García-Mendoza E., Ladah L. B. (2006). Photosynthetic performance, light absorption, and pigment composition of Macrocystis pyrifera (Laminariales, phaeophyceae) blades from different depths. J. Phycol. 42 (6), 1225–1234. doi: 10.1111/j.1529-8817.2006.00287.x
Demmig-Adams B., Adams W. W. III, Heber U., Neimanis S., Winter K., Kr̈ger A., et al. (1990). Inhibition of zeaxanthin formation and of rapid changes in radiationless energy dissipation by dithiothreitol in spinach leaves and chloroplasts. Plant Physiol. 92 (2), 293–301. doi: 10.1104/pp.92.2.293
Depauw F. A., Rogato A., Ribera d’Alcalá M., Falciatore A. (2012). Exploring the molecular basis of responses to light in marine diatoms. J. Exp. Bot. 63 (4), 1575–1591. doi: 10.1093/jxb/ers005
García-Mendoza E., Colombo-Pallotta M. F. (2007). The giant kelp Macrocystis pyrifera presents a different nonphotochemical quenching control than higher plants. New Phytol. 173, 526–536. doi: 10.1111/j.1469-8137.2006.01951.x
Garcia-Mendoza E., Ocampo-Alvarez H. (2011). Photoprotection in the brown alga Macrocystis pyrifera: evolutionary implications. J. Photochem. Photobiol. B: Biol. 104 (1-2), 377–385. doi: 10.1016/j.jphotobiol.2011.04.004
Goss R., Jakob T. (2010). Regulation and function of xanthophyll cycle-dependent photoprotection in algae. Photosynthesis Res. 106 (1), 103–122. doi: 10.1007/s11120-010-9536-x
Goss R., Lepetit B. (2015). Biodiversity of NPQ. J. Plant Physiol. 172, 13–32. doi: 10.1016/j.jplph.2014.03.004
Goss R., Pinto E. A., Wilhelm C., Richter M. (2006). The importance of a highly active and ΔpH-regulated diatoxanthin epoxidase for the regulation of the PS II antenna function in diadinoxanthin cycle containing algae. J. Plant Physiol. 163 (10), 1008–1021. doi: 10.1016/j.jplph.2005.09.008
Harker M., Berkaloff C., Lemoine Y., Britton G., YOUNG A. J., Duval J. C., et al. (1999). Effects of high light and desiccation on the operation of the xanthophyll cycle in two marine brown algae. Eur. J. Phycol. 34 (1), 35–42. doi: 10.1080/09670269910001736062
Hartel H., Lokstein H., Grimm B., Rank B. (1996). Kinetic studies on the xanthophyll cycle in barley leaves (influence of antenna size and relations to nonphotochemical chlorophyll fluorescence quenching). Plant Physiol. 110 (2), 471–482. doi: 10.1104/pp.110.2.471
Holt N. E., Zigmantas D., Valkunas L., Li X. P., Niyogi K. K., Fleming G. R. (2005). Carotenoid cation formation and the regulation of photosynthetic light harvesting. Science 307 (5708), 433–436. doi: 10.1126/science.1105833
Horton P., Johnson M. P., Perez-Bueno M. L., Kiss A. Z., Ruban A. V. (2008). Photosynthetic acclimation: does the dynamic structure and macro-organisation of photosystem II in higher plant grana membranes regulate light harvesting states? FEBS J. 275 (6), 1069–1079. doi: 10.1111/j.1742-4658.2008.06263.x
Horton P., Ruban A. (2005). Molecular design of the photosystem II light-harvesting antenna: photosynthesis and photoprotection. J. Exp. Bot. 56 (411), 365–373. doi: 10.1093/jxb/eri023
Jahns P. (1995). The xanthophyll cycle in intermittent light-grown pea plants (possible functions of chlorophyll a/b-binding proteins). Plant Physiol. 108 (1), 149–156. doi: 10.1104/pp.108.1.149
Jahns P., Holzwarth A. R. (2012). The role of the xanthophyll cycle and of lutein in photoprotection of photosystem II. Biochim. Biophys. Acta (BBA)-Bioenergetics 1817 (1), 182–193. doi: 10.1016/j.bbabio.2011.04.012
Josue J. S., Frank H. A. (2002). Direct determination of the S1 excited-state energies of xanthophylls by low-temperature fluorescence spectroscopy. J. Phys. Chem. A 106 (19), 4815–4824. doi: 10.1021/jp014150n
Li X. P., Gilmore A. M., Caffarri S., Bassi R., Golan T., Kramer D., et al. (2004). Regulation of photosynthetic light harvesting involves intrathylakoid lumen pH sensing by the PsbS protein. J. Biol. Chem. 279 (22), 22866–22874. doi: 10.1074/jbc.M402461200
Li X. M., Zhang Q. S., Tang Y. Z., Yu Y. Q., Liu H. L., Li L. X. (2014). Highly efficient photoprotective responses to high light stress in Sargassum thunbergii germlings, a representative brown macroalga of intertidal zone. J. Sea Res. 85, 491–498. doi: 10.1016/j.seares.2013.08.004
Long S. P., Humphries S., Falkowski P. G. (1994). Photoinhibition of photosynthesis in nature. Annu. Rev. Plant Biol. 45 (1), 633–662. doi: 10.1146/annurev.pp.45.060194.003221
Müller P., Li X. P., Niyogi K. K. (2001). Non-photochemical quenching. a response to excess light energy. Plant Physiol. 125, 1558–1566. doi: 10.1104/pp.125.4.1558
Ocampo-Alvarez H., García-Mendoza E., Govindjee (2013). Antagonist effect between violaxanthin and de-epoxidated pigments in nonphotochemical quenching induction in the qE deficient brown alga Macrocystis pyrifera. Biochim. Biophys. Acta (BBA)-Bioenergetics 1827, 427–437. doi: 10.1016/j.bbabio.2012.12.006
Ottander C., Öquist G. (1991). Recovery of photosynthesis in winter-stressed scots pine. Plant. Cell Environ. 14 (3), 345–349. doi: 10.1111/j.1365-3040.1991.tb01511.x
Peers G., Truong T. B., Ostendorf E., Busch A., Elrad D., Grossman A. R., et al. (2009). An ancient light-harvesting protein is critical for the regulation of algal photosynthesis. Nature 462 (7272), 518–521. doi: 10.1038/nature08587
Powles S. B. (1984). Photoinhibition of photosynthesis induced by visible light. Annu. Rev. Plant Physiol. 35 (1), 15–44. doi: 10.1146/annurev.pp.35.060184.000311
Rodrigues M. A., Dos Santos C. P., Young A. J., Strbac D., Hall D. O. (2002). A smaller and impaired xanthophyll cycle makes the deep sea macroalgae Laminaria abyssalis (Phaeophyceae) highly sensitive to daylight when compared with shallow water Laminaria digitata. J. Phycol. 38, 939–947. doi: 10.1046/j.1529-8817.2002.t01-1-01231.x
Ruban A. V. (2016). Nonphotochemical chlorophyll fluorescence quenching: Mechanism and effectiveness in protecting plants from photodamage. Plant Physiol. 170 (4), 1903–1916. doi: 10.1104/pp.15.01935
Ruban A. V., Phillip D., Young A. J., Horton P. (1997). Carotenoid-dependent oligomerization of the major chlorophyll a/b light harvesting complex of photosystem II of plants. Biochemistry 36 (25), 7855–7859. doi: 10.1021/bi9630725
Serôdio J., Cruz S., Vieira S., Brotas V. (2005). Non-photochemical quenching of chlorophyll fluorescence and operation of the xanthophyll cycle in estuarine microphytobenthos. J. Exp. Mar. Biol. Ecol. 326 (2), 157–169. doi: 10.1016/j.jembe.2005.05.011
Verhoeven A. (2014). Sustained energy dissipation in winter evergreens. New Phytol. 201, 57–65. doi: 10.1111/nph.12466
Verhoeven A. S., Adams W. I., Demmig-Adams B. (1998). Photoinhibition during winter stress in sun and shade Euonymus kiautschovicus leaves; The xanthophyll cycle and leaf adenylate status. Plant Cell Environ. 21, 893–903. doi: 10.1046/j.1365-3040.1998.00338.x
Yang X. Q., Zhang Q. S., Zhang D., Sheng Z. T. (2017). Light intensity dependent photosynthetic electron transport in eelgrass (Zostera marina l.). Plant Physiol. Biochem. 113, 168–176. doi: 10.1016/j.plaphy.2017.02.011
Yu Y. Q., Zhang Q. S., Tang Y. Z., Li X. M., Liu H. L., Li L. X. (2013). Diurnal changes of photosynthetic quantum yield in the intertidal macroalga Sargassum thunbergii under simulated tidal emersion conditions. J. Sea Res. 80, 50–57. doi: 10.1016/j.seares.2013.02.008
Yu Y. Q., Zhang Q. S., Tang Y. Z., Zhang S. B., Lu Z. C., Chu S. H., et al. (2012). Establishment of intertidal seaweed beds of Sargassum thunbergii through habitat creation and germling seeding. Ecol. Eng. 44, 10–17. doi: 10.1016/j.ecoleng.2012.03.016
Keywords: xanthophyll cycle, non-photochemical quenching, Sargassum thunbergii, photoinhibition, light fluctuations
Citation: Nan G-N, Zhou X-Q, Zhang X-M, Zhang Q-S, Hu Z-M, Huang R-P and Zhang D (2022) Xanthophyll cycle-related non-photochemical quenching protects Sargassum thunbergii from high light-induced photoinhibition. Front. Mar. Sci. 9:1067596. doi: 10.3389/fmars.2022.1067596
Received: 13 October 2022; Accepted: 06 December 2022;
Published: 22 December 2022.
Edited by:
Rui Pereira, A4F-Algafuel, PortugalReviewed by:
Maxwell A. Ware, Freie Universität Berlin, GermanyPaulo Cartaxana, University of Aveiro, Portugal
Copyright © 2022 Nan, Zhou, Zhang, Zhang, Hu, Huang and Zhang. This is an open-access article distributed under the terms of the Creative Commons Attribution License (CC BY). The use, distribution or reproduction in other forums is permitted, provided the original author(s) and the copyright owner(s) are credited and that the original publication in this journal is cited, in accordance with accepted academic practice. No use, distribution or reproduction is permitted which does not comply with these terms.
*Correspondence: Di Zhang, emhhbmdkaUB5dHUuZWR1LmNu
†These authors have contributed equally to this work