- 1Erhai Watershed Ecological Environment Quality Testing Engineering Research Center of Yunnan Provincial Universities, Erhai Research Institute, West Yunnan University of Applied Sciences, Dali, China
- 2Key Laboratory of Animal Ecology and Conservation Biology, Institute of Zoology, Chinese Academy of Sciences, Beijing, China
- 3Institute of Eastern-Himalaya Biodiversity Research, Dali University, Dali, China
- 4Institute of Ecology, China West Normal University, Nanchong, China
The outer cornea plays an important role in animal adaptation and survival in different environments. however, research on the morphological and ecological adaptation of corneal structure in amphibious fishes is limited. In this study, scanning electron microscopy (SEM) was used to evaluate the microstructure and adaptation of corneal epithelial cells in Oxudercinae. The results showed that the corneas of Oxudercinae species possess microridges, microvilli, and microplicae, as well as different numbers of epithelial cells. The morphological structure of corneal epithelial cells, observed by collecting samples and comparing the results with previous results, also showed different adaptive characteristics for moving between water and land. Further analyses revealed significant differences in epithelial cell density (F4, 22 = 5.436, P=0.003) and microridge width (F4, 22 = 8.392, P<0.001) among species with different levels of aquatic dependence. In addition, significant negative correlations of epithelial cell density with microridge width and separation width were confirmed (P<0.05). Interestingly, significant negative correlations of habitat type with cell density and microridges were uncovered, as well as a positive correlation between habitat type and separation width (P<0.05). The results indicated that the corneal structure of Oxudercinae species has characteristics of adaptation to an amphibious lifestyle.
Introduction
Understanding the mechanisms by which organisms adapt to changing environments has long been one of the central goals of ecology (Reuter and Peichl, 2008; Savolainen et al., 2013). Due to differences in habitat heterogeneity, fish species diversity is greatly influenced by the evolutionary transition between marine and terrestrial environments (Vega and Wiens, 2012). As changes in the light condition (floodplains transition to deep water, or diurnal to nocturnal), the environment becomes darker, and fishes show unique sensory, motor, and respiratory systems that evolved via adaptation to different bathymetric habitats, especially in regard to vision (Hu et al., 2022; Derbalah et al., 2022). Independent adaptation to different aquatic niches across fishes has been accompanied by widespread changes in the visual structures, systems, and mechanisms for coping with different light conditions (Graham, 1997; Simmich et al., 2012). The morphology of vertebrate corneal epithelial cells is affected by various environmental conditions, showing variation in cell types, densities, and microstructures, which play an important role in visual imaging (Collin and Collin, 2000a; Collin and Collin, 2000b; Subramanian et al., 2008; Simmich et al., 2012).
Vertebrate corneal epithelial cells are mainly irregularly pentagonal or hexagonal and contain many microstructures, such as microvilli, microplicae, microridges and microholes (Collin and Collin, 2006; Hu et al., 2016). It has been shown that aquatic and terrestrial vertebrates have different corneal epithelia; moreover, in some amphibious species, the densities of the superior and inferior surfaces of the cornea are significantly different (Mass and Supin, 2007; Subramanian et al., 2008). Previous studies have shown that the epithelium of marine organisms tends to have a higher cell density; conversely, nonaquatic vertebrates have a relatively low cell density on their corneas, through which the fishes can remove water efficiently (Collin and Collin, 2000b). Of the microstructures, microridges are the least efficient at increasing the surface area of cell membranes, and they are often found in highly permeable subsurface environments (mainly in the marine environment) (Simmich et al., 2012). By increasing the surface area of the cells, microvilli make it easier for oxygen and other nutrients to enter the cells, as well as assist in water transport and nutrient metabolism; these microstructures are essential for terrestrial vertebrates, as they maintain the intrinsic shape of the tear film and enable clear vision in the air (Beuerman and Pedroza, 1996). Microplicae are considered an intermediate form between microvilli and microridges and serve to support cellular morphology with increased osmotic exchange in the superficial layer of the cornea (Murdy, 1989; Jaafar and Larson, 2008). Both microstructures require continuous protection by mucus secretions after the evaporation of water. Microholes allow mucus to be secreted onto the corneal surface (Collin and Collin, 2006). It has been confirmed that structural changes are related to selection pressures in different habitats, such as desiccation and abrasion in terrestrial environments and infection in aquatic environments (Subramanian et al., 2008). In Collin et al.’s study, these four microstructures were found to be adapted to the specific habitat of each taxon (Collin and Collin, 2000b). Further studies revealed microstructural changes in the corneas of four-eyed fish (Anableps anableps), and the adaptation patterns of vision-related genes were related to their specific habitat (Avery and Bowmaker, 1982; Owens et al., 2009; Simmich et al., 2012). For a better understanding of how vision evolved to adapt to amphibious environments, it is important to study the physiological characteristics of species with both aquatic and terrestrial vision.
The subfamily Oxudercinae, consisting of 10 genera and 41 species, is a special group showing sharply divergent habitats from aquatic to terrestrial environments. These fishes are mostly found in intertidal mangrove or muddy mudflat areas in tropical, subtropical, and temperate seas (Ishimatsu and Gonzales, 2011). Mudskippers are representative taxa and are the largest group of amphibious teleost fishes, with unique adaptations enabling them to live on mudflats (Nursall, 1981; Burggren, 1997; Sayer, 2005). As a result of adaptation to specific amphibious habitats, their corneal development has special genetic and structural characteristics (Hu et al., 2016), and the cornea-related COL8A2 gene has undergone adaptive evolution (Hu et al., 2022). In addition, different species in this taxon show different degrees of adaptation to amphibious life due to habitat differentiation (Takita et al., 1999; You et al., 2014). Intertidal mudflats are one of the most drastically changing environments in terms of salinity, temperature, and oxygen level, leading to several adaptations in mudskippers. However, there is still a lack of understanding of the corneal adaptation of Oxudercinae to different habitats. To elucidate the adaptation of corneal characteristics of amphibious fishes, we characterized the corneal morphology and histology of five species of Oxudercinae in amphibious habitats. Through correlation analysis, we further investigated the relationships between the histological characteristics of fish eyes and their habitats to provide a reference for further studying the visual adaptation mechanisms allowing movement between aquatic and terrestrial environments.
Materials and methods
Acquisition and treatment of specimens
Specimens sampled from Riau Sumatra, Indonesia and Xiapu, China, were divided into five groups: Pseudapocryptes elongatus (5 individuals), Oxuderces dentatus (5 individuals), Scartelaos histophorus (6 individuals), Boleophthalmus pectinriostris (7 individuals), and Periophthalmus magnuspinnatus (6 individuals). The specimens were deposited in National Animal Collection Resource Center, Institute of Zoology, Chinese Academy of Sciences (CAS). The examined specimens are listed in Table 1, including individual number and aspects of size, such as total length, head length and eye diameter, measured using a 032102 digital Vernier caliper (exploit 200 mm, precision 0.1 mm).
The specimens were mature, although their exact ages were unknown. All the individuals were in good health prior to euthanasia, showing no evidence of ocular disease or abnormality. Specimens were euthanized with tricaine mesylate (MS-222) in accordance with ethical guidelines under Experimental Animal Ethical Approval Number AEI-04-03-2014 ratified by the Institute of Zoology, Chinese Academy of Sciences. The dose of immersion was 400 ppm for 10 minutes.
After observing the location and shape of the eyes through a ZEISS STEISV II microscope, the right eye of the specimen was removed intact, and the cornea of each individual was stripped, fixed in Karnovsky’s fixative (2.5% glutaraldehyde, 2% paraformaldehyde, 0.1 M sodium cacodylate buffer, 2% sucrose and 0.1% calcium chloride, pH 7.2) and then rinsed in 0.1 M sodium cacodylate buffer. The corneas were postfixed in 1% osmium tetroxide and rinsed in 0.1 M cacodylate buffer, followed by a water rinse and alcohol dehydration in a graded series. Cornea samples were desiccated with a critical point desiccator (LEICAEMCPP 300). When completely dry, the samples were mounted on 10-mm aluminum stubs. The mounted samples were coated with 12-15 nm of gold-palladium in a sputter coater (HITA-CHI E-1010) and examined under a scanning electron microscope (SEM, HITACHI S-3000N). Higher-magnification images were recorded digitally all around the cornea. With the fish eyes in a natural open state, the corneas were observed and compared in the middle, upper and lower areas. To ensure the effectiveness of the photos, more than 5 photos were taken at multiple locations in each area to record information, as shown in Figure 1.
Because the corneas of the fish were enlarged many times under a scanning electron microscope (the magnification range of the images used in this paper was 2000-24000 times), the actual area of the picture can be calculated by the plotting scale and the side length of the original image as recorded by the scanning electron microscope. For cell counting, the method adopted in this study was similar to the cell counting plate method, in which each picture was treated as a counting cell in the middle of a cell plate. Because of the obvious cell boundaries in the picture, all the cells in the picture could be counted. For incomplete cells at the edge of the image, only the cells on the left and upper sides (including cells pressed against the line) were counted, and the cells pressed against the lines on the lower and right sides of the image were omitted to reduce the count error. ArcGIS 10 software was used to count the number of cells in each picture and measure the size of the picture. According to the picture scale, the density of corneal epithelial cells in each picture was calculated, and then the corneal density in each area of each specimen was obtained by averaging the data of multiple pictures.
The cell types and microstructures in the images were determined by observing the electron microscope images. ArcGIS 10 software was also used to measure the width and spacing of the microcrest. According to the picture scale, the measured distance was converted, and then the microcrest width and microcrest spacing of each area of each specimen were obtained by averaging the data of multiple points and multiple pictures.
Data analysis
We compared the two positions (upper, lower) of the naturally exposed cornea (Figure 1). In a specified area of each region, the cell type and microstructures were described. The number of cells was determined and converted into density at cell number per mm2, and microridge width and separation were measured using ArcGIS 10. Differences in cell density, microridge width and microridge separation among species were analyzed by one-way analysis of variance (ANOVA). Differences between upper and lower regions within the same species were tested by paired-samples t test using IBM SPSS (Kirkpatrick and Feeney, 2013). All the results are shown as the mean ± standard deviation (SD) (Table S1). To better understand the relationship between habitat type and phylogeny and further explore the correlations between locomotory mode and corneal traits, we obtained the phylogenetic tree and locomotory features of Oxudercinae according to Steppan et al. (2022) (Figure 2). The habitat assignments for the degree of water dependence of different Oxudercinae species were as follows: 1 = mud swimmers (generally eel-like, no substantial use of pectoral fins), 2 = pectoral-aided mud swimmers (generally eel-like with either reported sculling or semilobed fins but the inability to fully lift the body), and 3 = terrestrially crutchers (short, stout bodies with fully developed lobe fins) (Table S2). Then, the correlation analysis was carried out in IBM SPSS (Kirkpatrick and Feeney, 2013). To exclude false positives as much as possible, Bonferroni correction was implemented.
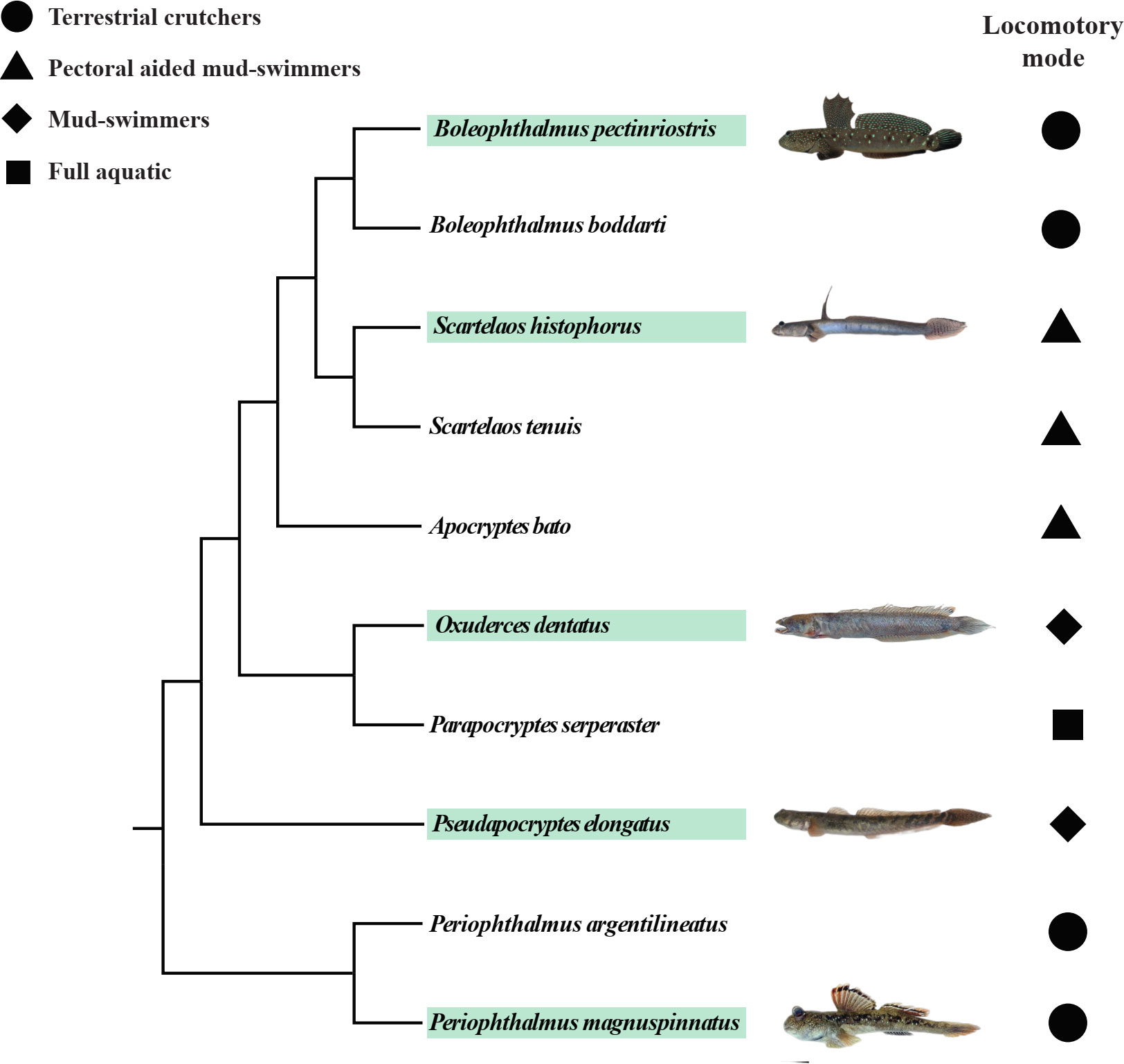
Figure 2 The phylogeny and locomotory modes of subfamily Oxudercinae obtained and adapted from Steppan et al. (2022). The images of fish are from “The Mudskipper: http://www.themudskipper.org/”. The species in the green boxes are the ones that we examined in this study.
Results
Type and microstructure of epithelial cells
The corneal cell types and cellular microstructures of five fishes were observed by using scanning electron microscopy; detailed descriptions are provided below.
Ps. elongatus corneal epithelial cells are shown in Figures 3A, B, where cell borders were evident, microridges were clear and continuous, microridge widths and gaps could be measured, and microholes, microplicae, and microvilli were observed (Figures 3C, D). However, in Ps. elongatus, morphologically specific micropores appeared in the form of a complex subcircular arrangement surrounded by microvilli of varying lengths, composed of 5-8 individual micropores. The cell type was microridge cells.
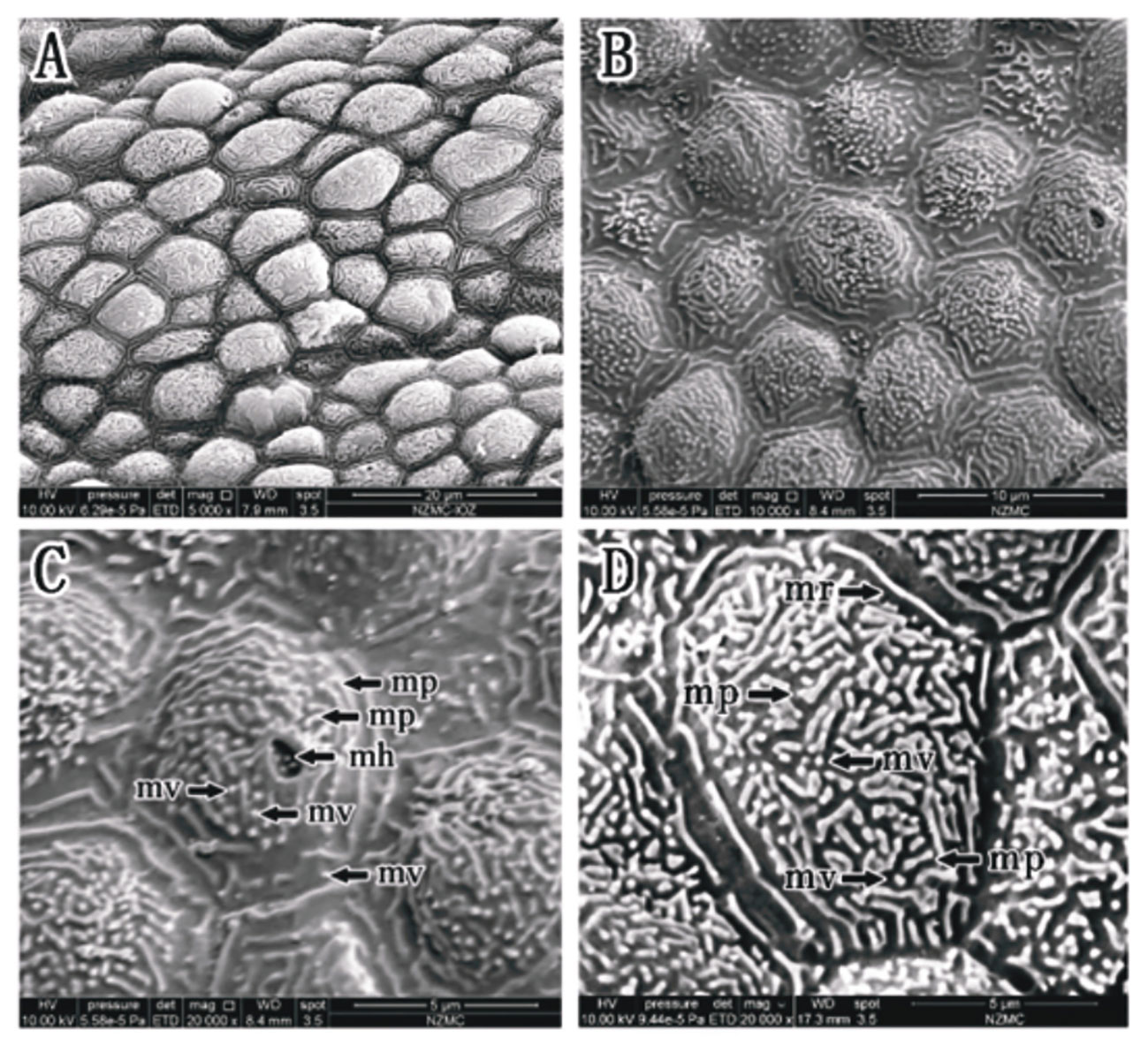
Figure 3 (A–D) Corneal epithelial cells of Ps. elongatus under an SEM. Microridge cell type (A, B). Microplicae, microridge, microvilli and microholes (B–D). (mp, microplicae; mv, microvilli; mh, microholes; mr, microridge).
As shown in Figures 4A, B, the corneal epithelial cells of O. dentatus were clearly demarcated from each other, with loose gaps and measurable gap widths, only a very small number of continuous microridges, and distinct cell borders. There were microholes, microplicae and microvilli (Figure 4B). The cell type was microplicae cells. Taste buds or other kinds of sensory structures may be shown in Figures 4C, D, which were observed on the surface of the cornea for the first and time in our experiment.
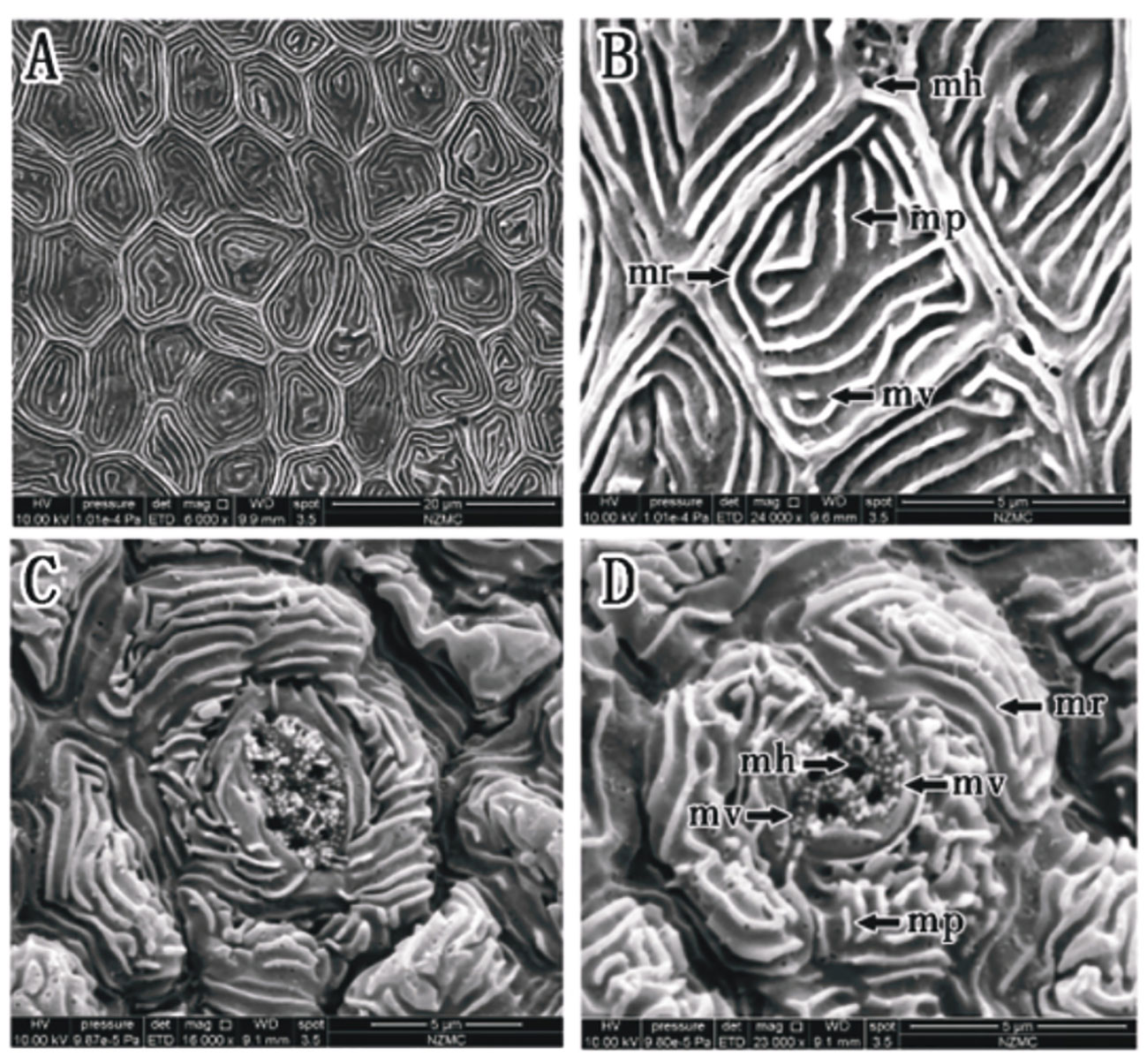
Figure 4 (A–D) Corneal epithelial cells of O. dentatus under an SEM. Microplicae cell type (A, B). Microplicae, microridge, microvilli and microholes (B). (mp, microplicae; mv, microvilli; mh, microholes; mr, microridge). Taste buds or sensory structures (C, D).
In S. histophorus, corneal epithelial cells were observed as the ridge type. The border of corneal epithelial cells was clear (Figures 5A, B). Microplicae, microvilli and microridges were observed under high magnification, but without microholes (Figures 5C, D). The cell type was microridge cells.
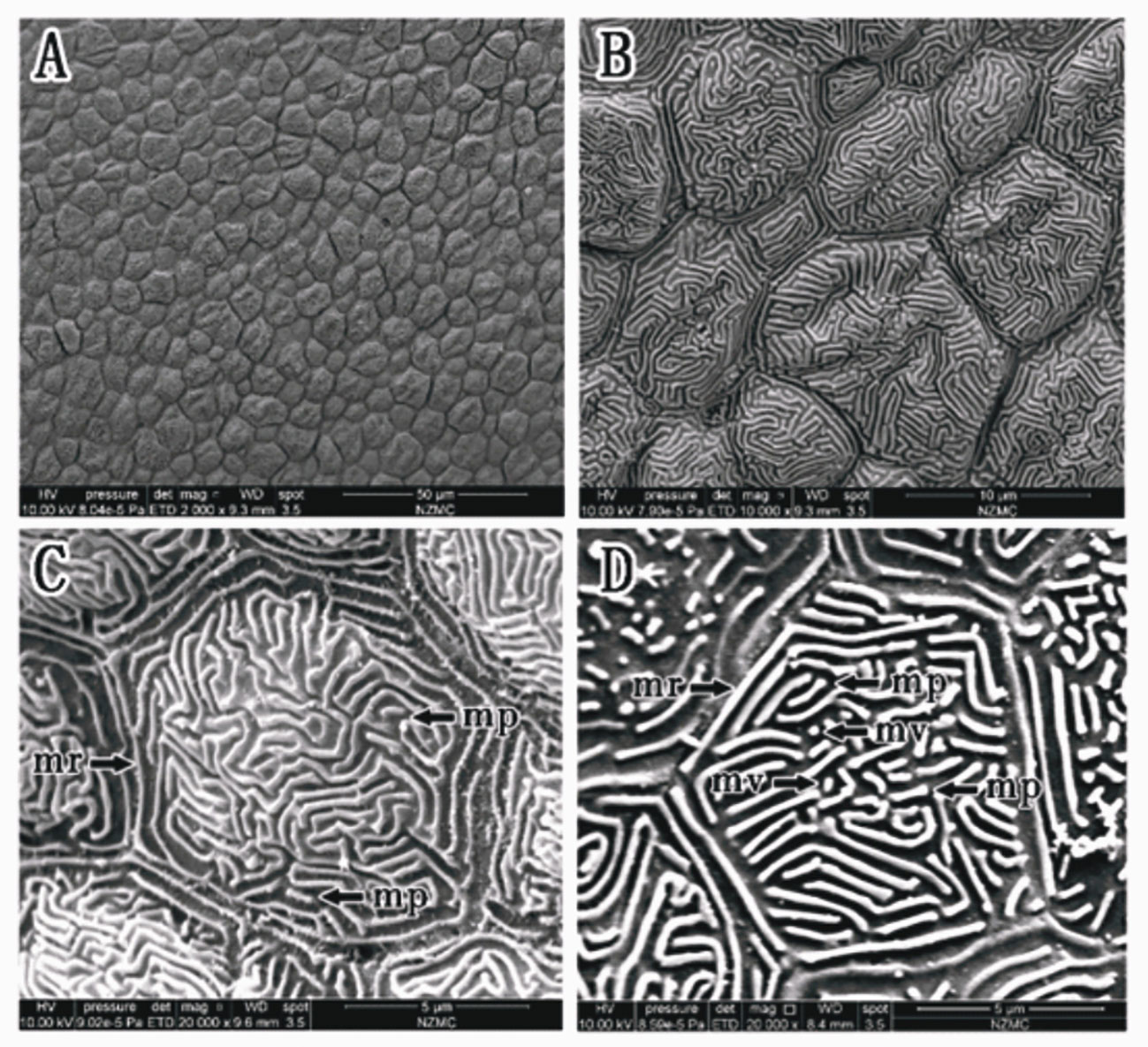
Figure 5 (A–D) Corneal epithelial cells of S. histophorus under an SEM. Microridge cell type (B, C). Microplicae, microridges and microvilli (C, D). (mp, microplicae; mv, microvilli; mh, microholes; mr, microridge).
In B. pectinirostris, the border of corneal epithelial cells was clear (Figure 6A). Cells were differentiated into diverse structures inside as the ridge type, reticular type, and ridge-reticular type (Figures 6A, B, D). Microridges, microplicae, microvilli and microholes were observed under high magnification (Figures 6B, C).
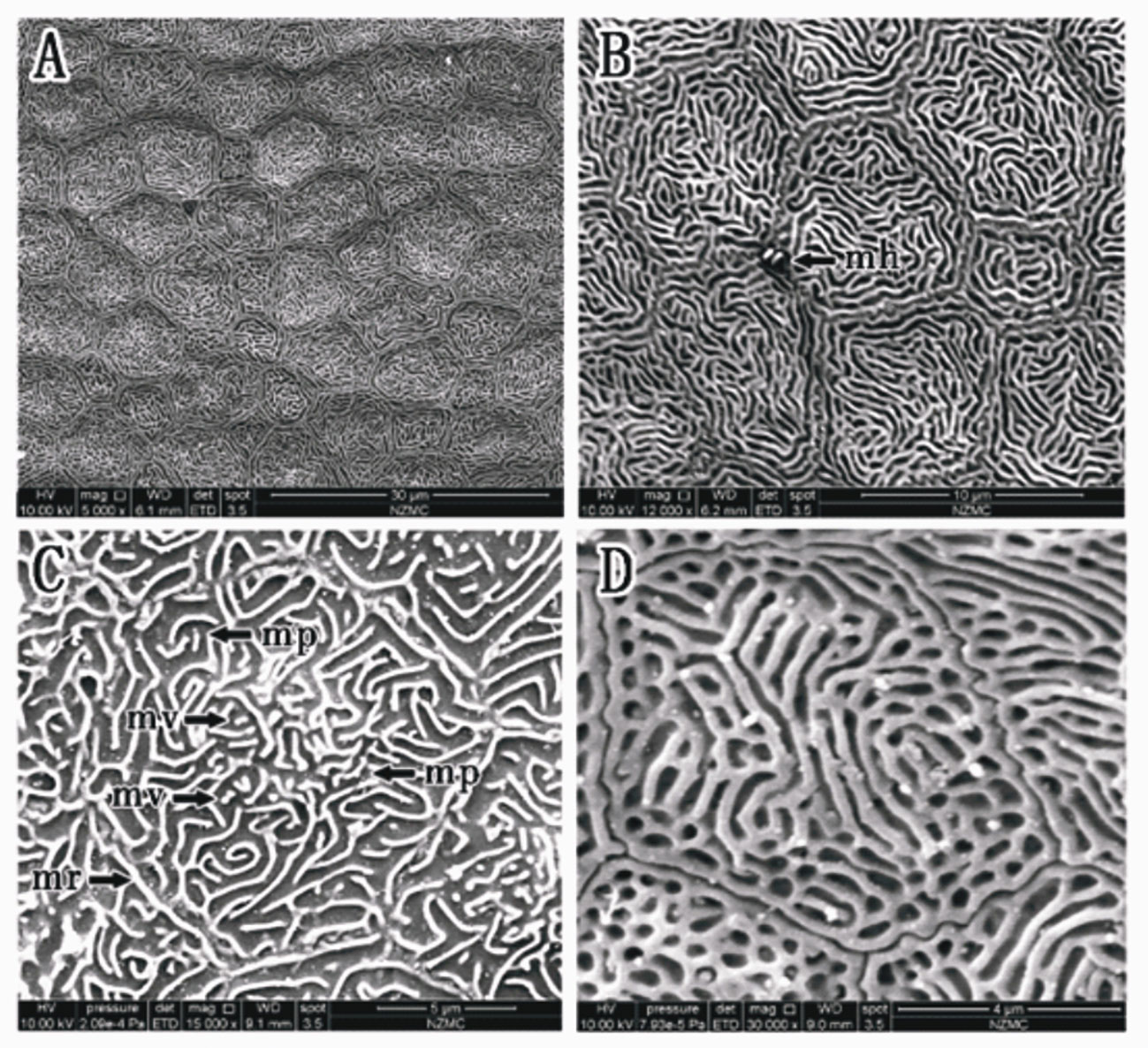
Figure 6 (A–D) Corneal epithelial cells of (B) pectinriostris under an SEM. Microridge cell type (A); Microplicae cell type (C); Reticular cell (D); Mixed cell (Microridge and Reticular) (D). Microplicae, microridges, microvilli and microholes (B, C). (mp, microplicae; mv, microvilli; mh, microholes; mr, microridge).
In P. magnuspinnatus, ridge-type and reticular-type cells were observed (Figures 7A, B). The corneal epithelial cells also contained four types of microstructures: microridges, microplicae, microvilli and microholes (Figures 7B–D). Compared with those of B. pectinirostris, the microridges of corneal epithelial cells of P. magnuspinnatus were rough with more microplicae and microvilli. The corneal epithelial cell surface is characterized by fragmentation and a compact structure. On the inferior side of the cornea of P. magnuspinnatus, some areas covered with microholes were observed (Figure 7C).
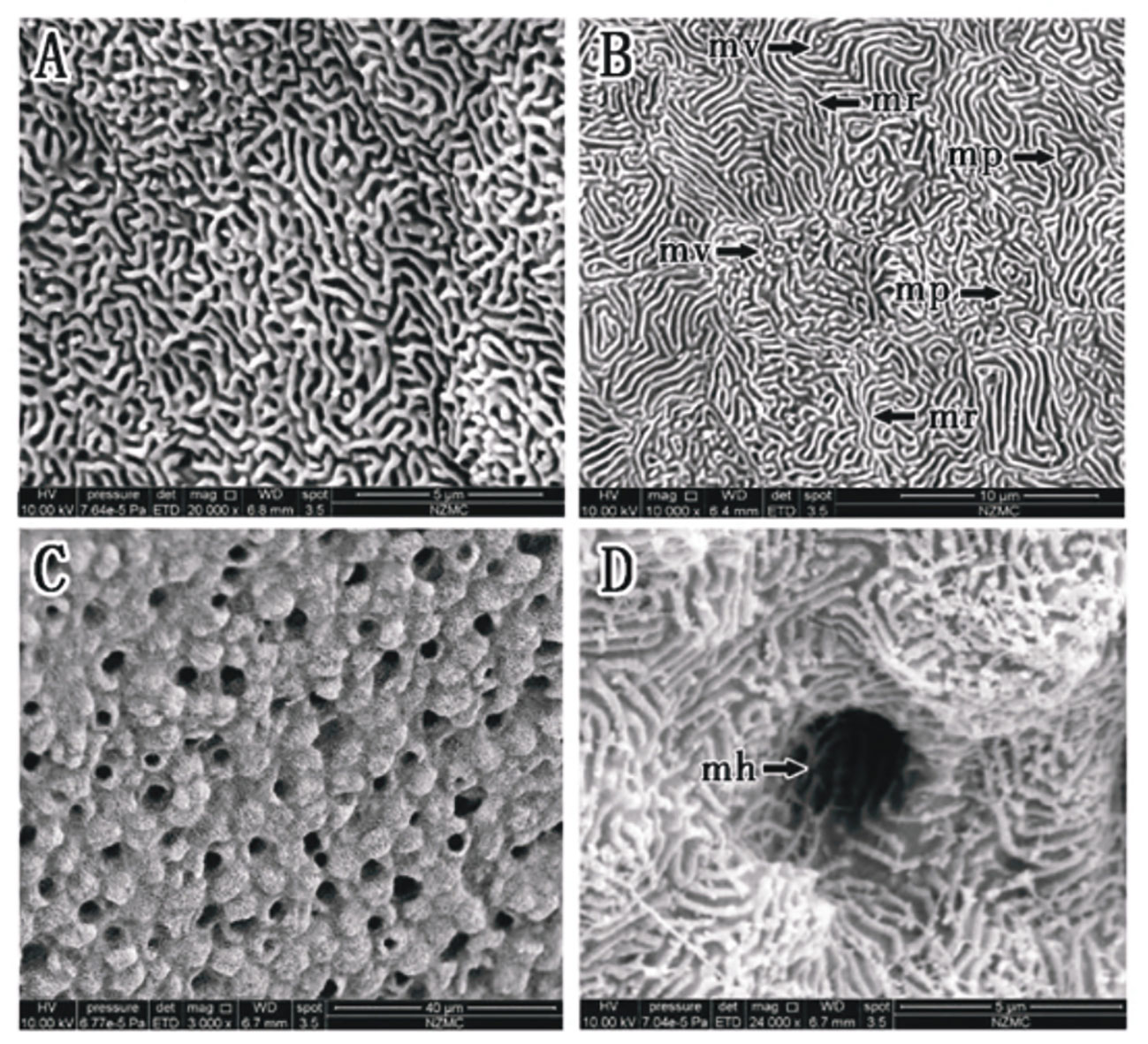
Figure 7 (A–D) Corneal epithelial cells of P. magnuspinnatus under SEM. Microridge cell type (B); Microplicae cell type (B); Microplicae, microridge, microvilli and microholes (B–D). (mp, microplicae; mv, microvilli; mh, microholes; mr, microridge).
Based on the SEM results, there appeared to be differences among taxa in terms of microholes. No microholes were observed in S. histophorus, and similar small numbers of microholes were found in B. pectinriostris and O. dentatus. An increased number of microholes was observed in Ps. elongatus. P. magnuspinnatus was found to have the highest numbers of microholes among the five fishes. Additionally, there were also differences in the types of cells (Table 2).
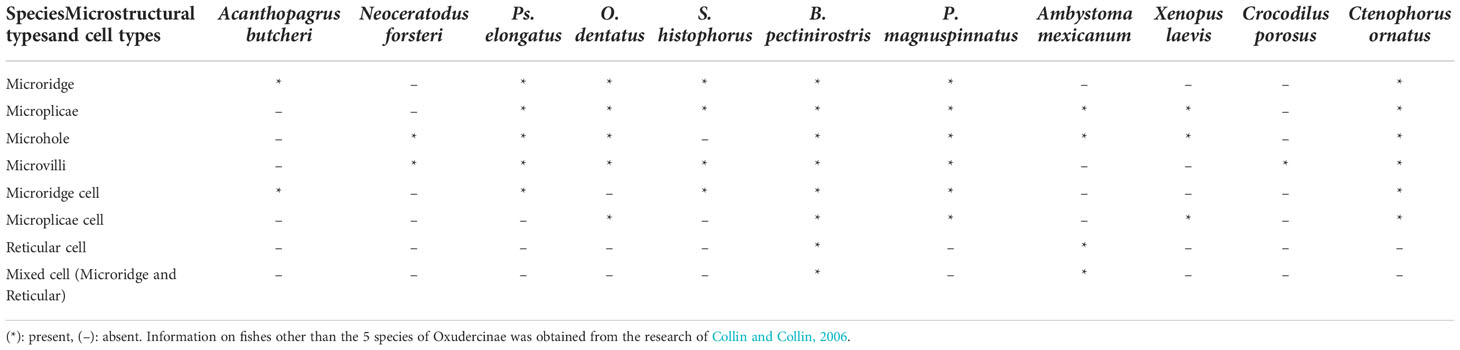
Table 2 The comparison of corneal epithelial cell microstructures and cell types among different species.
Comparison of the microstructure of corneal epithelial cells in various taxa
By using the SEM results above combined with those of Collin et al. (Collin and Collin, 2006), a comparison was performed against the corneal density, microstructure, and cell type of six species other than the five fish species examined in this study, including Acanthopagrus butcheri, Neoceratodus forsteri, Ambystoma mexicanum, Xenopus laevis, Crocodilus porosus, and Ctenophorus ornatus. In terms of cell types, microridge cells were observed in A. butcheri, Ps. elongatus, S. histophorus, B. pectinriostris and C. ornatus. Microplicae cells were found in O. dentatus, B. pectinriostris, P. magnuspinnatus, X. laevis and C. ornatus. Reticular cells were observed in B. pectinriostris, P. magnuspinnatus and A. mexicanum. In adult B. pectinriostris and A. mexicanum, a mixture of microridge cells and reticular cells was observed. Microstructures such as microridges, microplicae, microholes and microvilli appeared to be present and absent in a variety of taxa (Table 2).
Epithelial cell density
The epithelial cell densities of the 5 Oxudercinae species varied between 29632 ± 7766 cells per mm2 in B. pectinriostris and 52861 ± 10664 cells per mm2 in O. dentatus (Figure 7; Table S1), and the difference was significant (F4, 22 = 5.436, P=0.003). The trends in cell density in the upper and lower regions within species, as well as the overall trends, were similar and significantly different (up: F4, 22 = 4.690, P=0.007; down: F4, 22 = 4.157, P=0.012). In addition, the differences between the upper region and the lower region of the corneas in the other four species were not significant (Figure 8), except in S. histophorus (t=3.373, P=0.028).
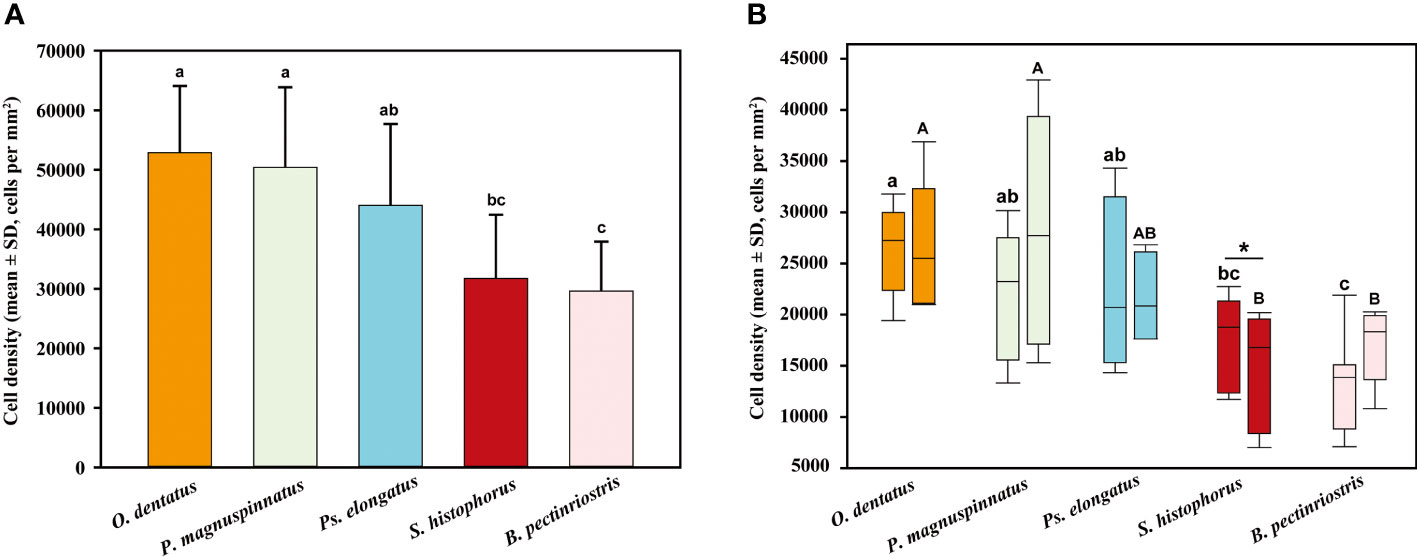
Figure 8 Comparison of epithelial cell density among different Oxudercinae species (the order of corneal epithelial cell densities for the five fish species is shown in (A); the upper and lower cell densities are shown in (B).
Microridge width (nm) and separation width (nm)
Our results showed that the microridge width of corneal epithelial cells differed significantly among the 5 species of Oxudercinae. Microridge widths were significantly higher in Ps. elongatus and B. pectinriostris than in O. dentatus, S. histophorus and P. magnuspinnatus (F4, 22 = 8.392, P<0.001; Figure 8). The trends of microridge widths in the upper region and the lower region among species were similar to the trends of total microridge widths. Microridge width was significantly different among the 5 species in the upper region (F4, 22 = 7.419, P=0.001) but not in the lower region (F4, 22 = 1.671, P=0.193). Additionally, there were no significant differences between the upper and lower regions within species (Figure 9).
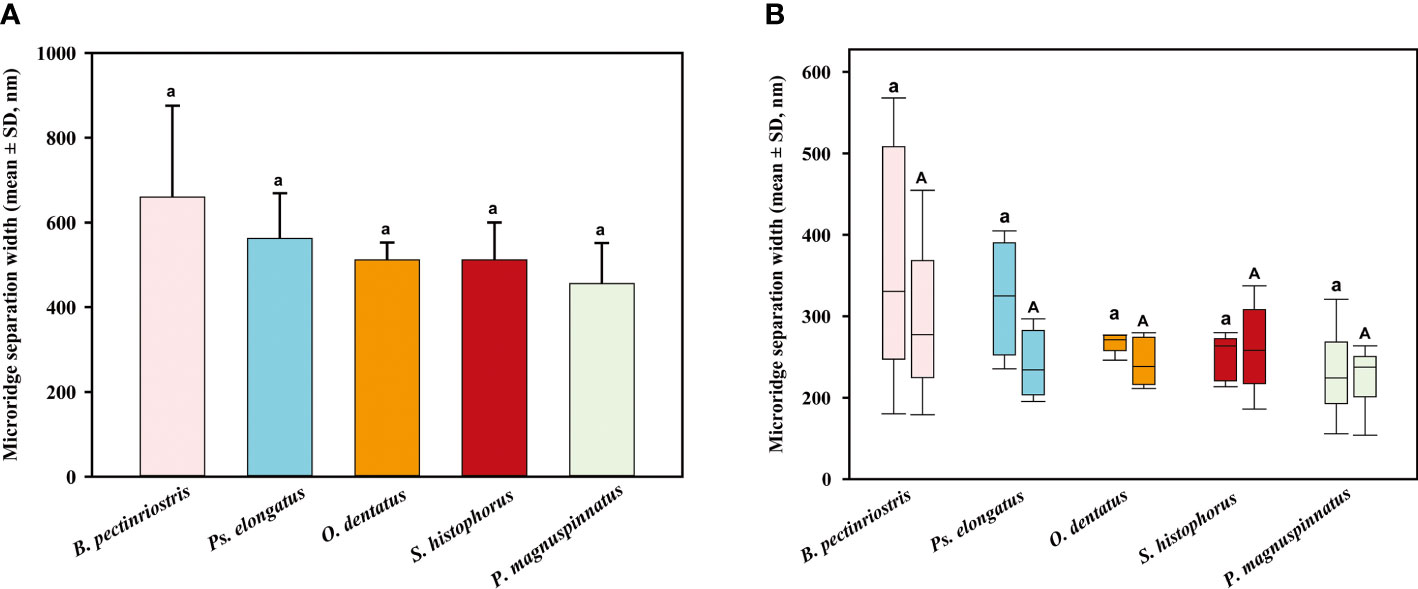
Figure 9 The comparison of microridge width among different Oxudercinae species (the order of corneal epithelial microridge widths for the five fish species is shown in (A); the upper and lower microridge widths are shown in (B).
The separation width trend was similar to the microridge width trend but slightly different from the microridge width trend, as there was no significant difference between the various groups (F4, 22 = 2.350, P=0.086; Figure 9). A similar pattern of microridge widths was observed in both upper regions and lower regions, and this trait did not significantly differ from total microridge width across species (upper: F4, 22 = 2.397, P=0.081; lower: F4, 22 = 1.392, P=0.269). The separation widths of the upper region and lower region did not show significant differences within species (Figure 10).
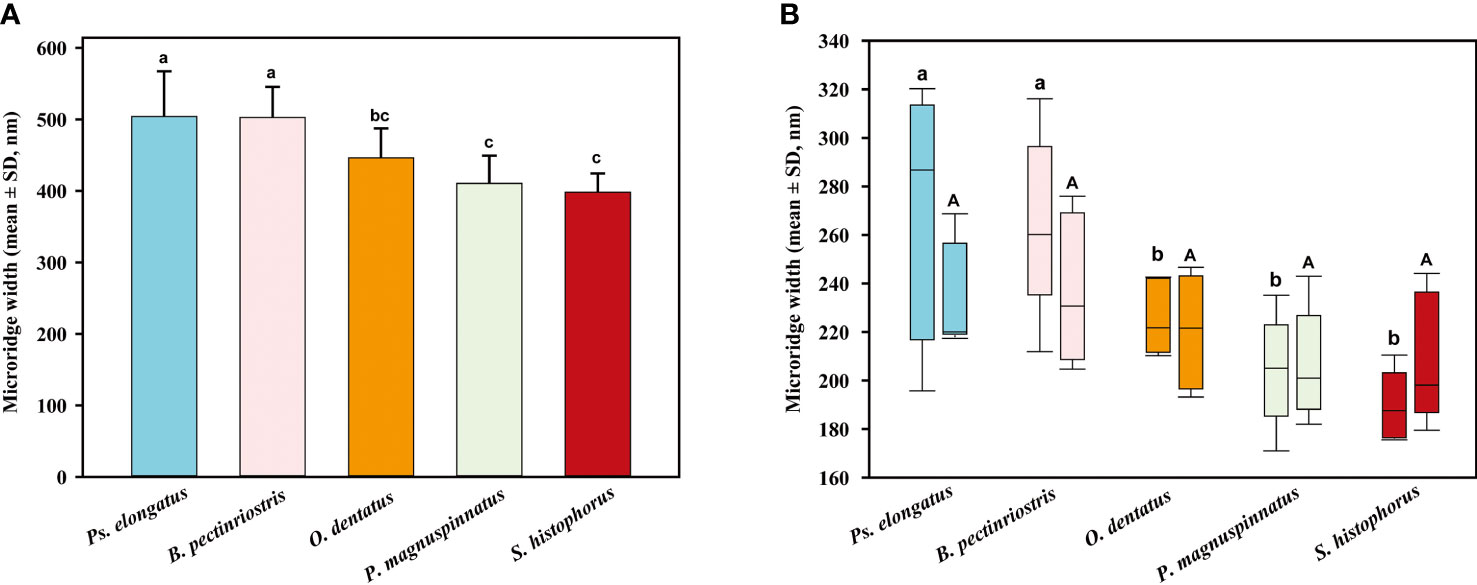
Figure 10 The comparison of microridge separation width among different Oxudercinae species (the order of corneal epithelial microridge separation widths for the five fish species is shown in (A); the upper and lower microridge separation widths are shown in (B).
Interestingly, further analyses revealed significant negative correlations of cell density with microridge width and separation width (Figures 11A, B). To exclude false positive statistics as much as possible, we further performed Bonferroni correction (α = 0.01). However, no significant differences were found (Figure 11A: P=0.024, Figure 11B: P=0.012).
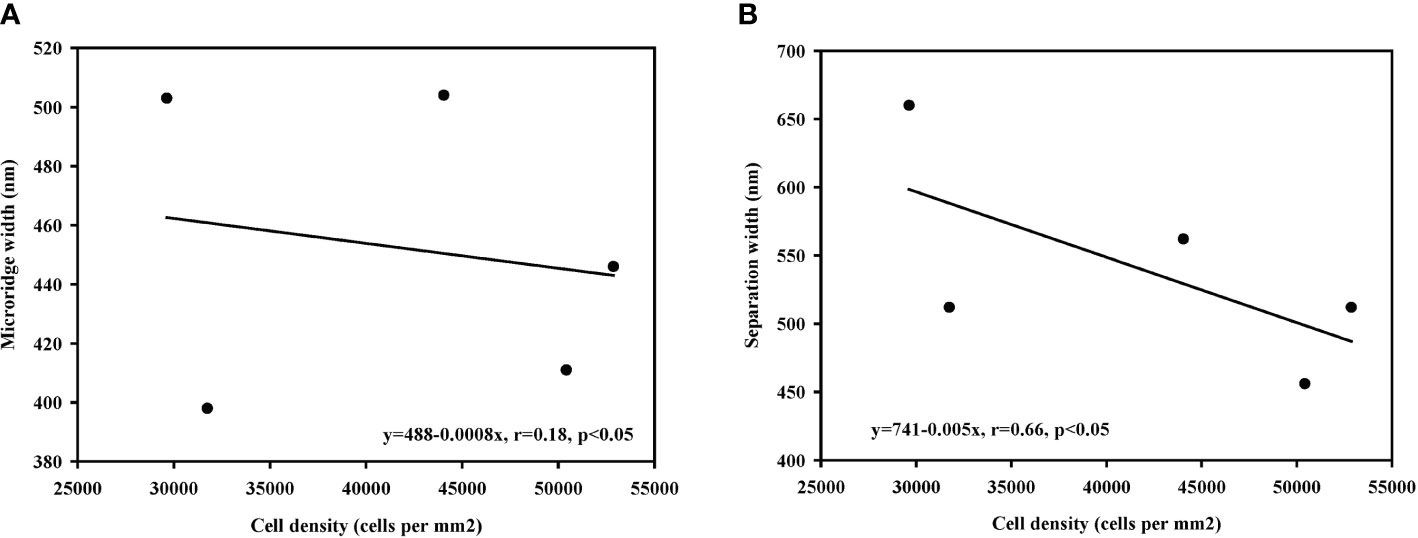
Figure 11 The correlation between cell density and width among different Oxudercinae species. (Cell density vs. microridge width in (A); cell density vs. microridge separation width in (B).
Correlation analysis between locomotory mode state and cell density, microridge width, and separation width
The results of correlation analyses showed significant negative correlations between locomotory mode and cell density and between locomotory mode and microridge width (Figures 12A, B); however, there was a significant positive correlation between locomotory mode and separation width (Figure 12C). After Bonferroni correction (α = 0.01), only the correlation between locomotory mode and microridge width was significant (Figure 12B: P=0.005). The other two correlations were not significant (Figure 12A: P=0.027, Figure 12C: P=0.012).
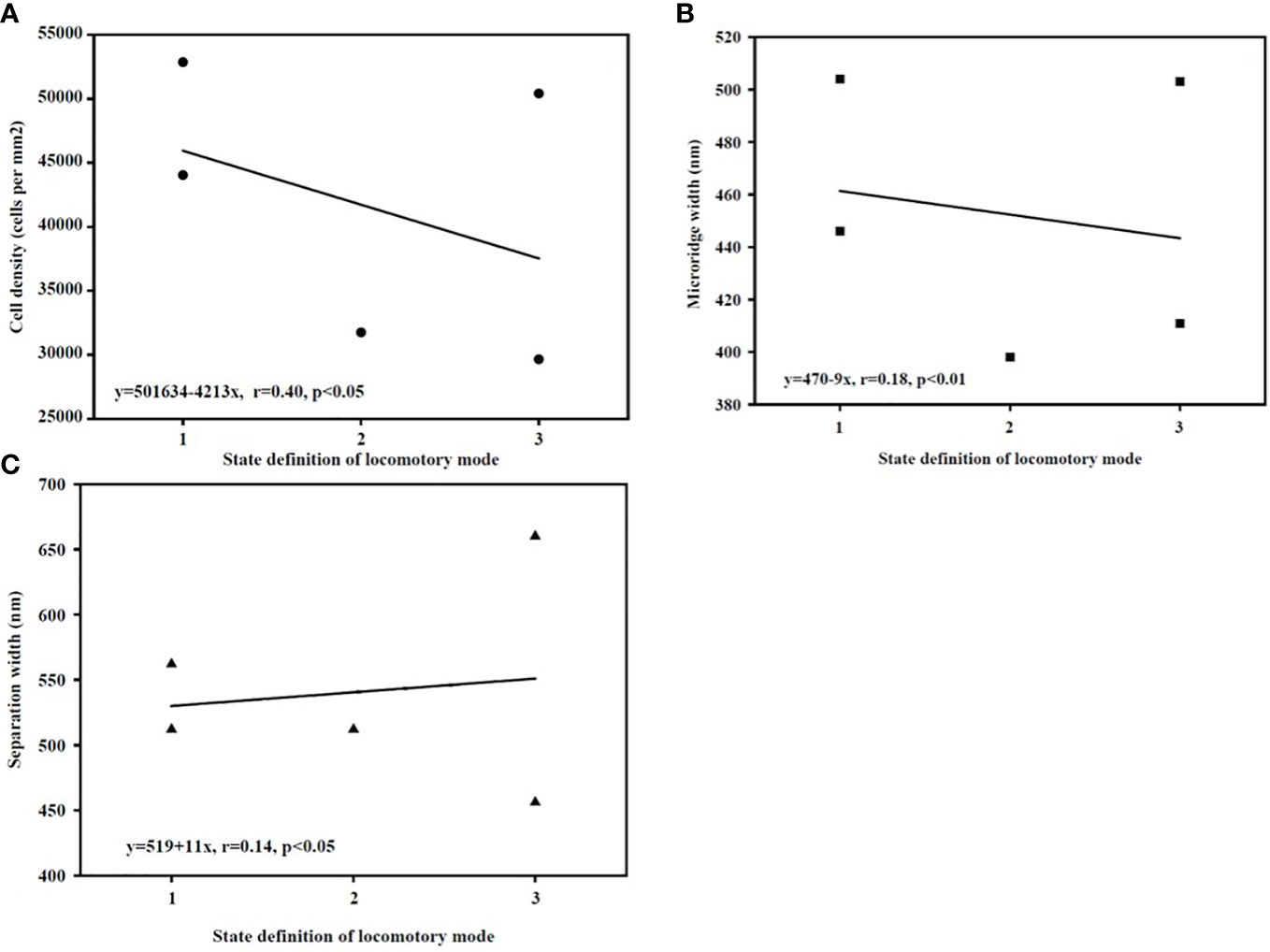
Figure 12 (A–C) The correlation between locomotory mode state and corneal epithelial data. [(Locomotory mode vs. cell density (A); locomotory mode vs. microridge width (B); locomotory mode vs. microridge separation width (C)].
Through a literature review and field observations, we generated detailed descriptions of the preferences and locomotory mode for each species in our study.
O. dentatus: A small warm-water nearshore fish living in saltwater and freshwater zones of estuaries and low-tide areas of nearshore tidal flats; individuals often crawl or jump on mud flats with developed pectoral fin muscle stalks. They can adapt to a wide range of temperatures and salinities and settle in caves. They are sensitive in vision and hearing and usually come out of the cave during the day when the tide is low. When they are slightly frightened, they dive back into the water or drill into the cave (Wu and Zhong, 2008). Adults are more common in the lower part of the tidal flat, while smaller individuals usually appear at the depth of the tidal flat (Murdy, 1989).
Ps. elongatus: Adult individuals are more common in the lower mudflats and creeks; the subadults are mostly found in mangroves, aquatic areas and recluse in neap tide pools of subtidal zones (Bucholtz and Meilvang, 2005). During the dry season, when the tide pools are depleted, this species can aestivate in vertical burrows approximately 60 cm deep (Hora, 1936; Swennen et al., 1995). According to Murdy (1989), P. elongatus can breathe air through the gills, oropharyngeal epithelium, operculum epithelium, and skin and is capable of short-term survival out of water. We observed the fish not only breathing air in shallow water but also making brief crawls on the mudflat surface. This fish is highly halogenic (Bucholtz et al., 2009). It is a benthic omnivore, feeding mainly on phytoplankton and small invertebrates. It jumps out of shallow water (the head part of the body jumps out of the water) and moves its head from side to side to scrape and filter mud, similarly to Boleophthalmus (Swennen et al., 1995; Bucholtz and Meilvang, 2005; Bucholtz et al., 2009).
S. histophorus: A small warm-water fish living in saltwater and freshwater zones of estuaries. During low tide, larger individuals are more common in the lower areas of tidal flats or below the mean sea level, while smaller individuals are usually found in upper areas, such as the respiratory root zone of mangrove forests (Polgar and Bartolino, 2010) and riverbanks (Milward, 1974). They can adapt to various environmental conditions during relatively low tides, such as mudflats, tidal pools with varying degrees of dryness, or areas where plant debris and respiratory roots are mixed (Milward, 1974; Ansell et al., 1993). They leave burrows to breed at low tide. The males use their tails to stand to attract females to their burrows to lay eggs (Milward, 1974; Townsend and Tibbetts, 2005).
P. magnuspinnatus: This species is found in intertidal mudflats with taller vegetation, in mangrove respiratory root zones, and on coastal tidal flats near estuaries. During winter in temperate regions, it lives in caves (Murdy, 1989). In Korea, the active season is from late April to early September. The active season is slightly shorter than that of P. modestus living in the same area (Baeck et al., 2008). In contrast, P. magnuspinnatus makes long-term use of its burrowing sites throughout its active season (Baeck et al., 2008).
B. pectinriostris: This species lives in the open area of coastal mudflats. The largest individuals are found only in the lower unvegetated mudflats, while smaller individuals show a more irregular distribution and enter the respiratory root zone of mangroves along the entrance of temporary bays in the open areas of mudflats (Polgar and Bartolino, 2010). In winter, groups in southern Japan hibernate at the bottom of caves, and they can adapt to various environmental conditions during relatively low tide, handle broad temperature and salt conditions and show short-term amphibious habits with strong vitality (Takegaki et al., 2006). This fish is very sensitive to sound and movement. Individuals drill holes or escape into mangrove trees as soon as they encounter signs of enemies, which plays an important role in maintaining the survival and reproduction of the population. The species lives in damp holes. The skin and tail have an auxiliary respiratory function, and individuals can leave the water to forage for a period of time. In the nonreproductive season, they usually live alone in caves and go into seclusion or under water at high tide. During the reproductive period, male and female adult fish live together, spawn and fertilize in the channels. Viscous eggs adhere to the cave wall or substrate by attaching silk, and the male fish gear protects the eggs. Cave dwelling and reproduction can reduce the threat of natural disasters and enemy organisms and improve their survival ability.
Discussion
Diversity of habitat taxa appeared to affect cell density
In general, aquatic vertebrates have a denser corneal epithelium than terrestrial vertebrates (Collin and Collin, 2000a; Mass and Supin, 2007), which is thought to be an adaptation to differences between air and aquatic osmotic stress (Collin and Collin, 2000a; Collin and Collin, 2006). Our results partly showed a similar trend (Figure 12A). Additionally, cell density decreases as the osmotic stress placed on the cornea decreases among aquatic vertebrates (Kröger, 1992; Collin and Collin, 2006). Marine vertebrates maintain proper osmotic levels because the higher epithelial cell density aids in salt transport and water uptake. In contrast, a relatively low epithelial cell density contributes to corneal water removal in nonaquatic vertebrates (Collin and Collin, 2006). It was suggested that different aquatic and terrestrial habitats, as well as different osmotic pressures in aquatic environments, could influence corneal morphological adaptation.
Our results showed that corneal epithelial dependence on aquatic environments decrease, and corneal epithelial density declines (Figures 7A, B, 11A), which are consistent with previous findings. From marine to estuarine or freshwater to terrestrial environments, there is a progressive decrease in epithelial cell density in vertebrates (Collin and Collin, 2000a; Collin and Collin, 2000b). Three species (P. magnuspinnatus, B. pectinriostris, and O. dentatus) showed a higher cell density on the lower region of the cornea than on the upper region of the cornea (Figure 8). Similar findings were obtained in a previous study of ‘four-eyed fish’ (A. anableps), which further confirmed that osmotic pressure influences the distribution of cell density in the cornea (Owens et al., 2009; Simmich et al., 2012). Additionally, the negative relationship between cell density and locomotory mode (or habitat) further confirmed the decreasing tendency. However, due to the differences in corneal epithelial cell density between the upper region and lower region, subadult S. histophorus live in terrestrial habitats, while the adults prefer lower, wet areas. The cell density of S. histophorus was high in the upper region but low in the lower region (Figure 7B), which was in contrast to the trend of changes in A. anableps as well as the other three species in Oxudercinae (Collin and Collin, 2006; Simmich et al., 2012). Generally, it can be concluded that the density of epithelial cells in Oxudercinae is affected by dependence on the aquatic environment (Davies et al., 2012; Hauser et al., 2017; Hauser and Chang, 2017). Further investigation is required to determine the distribution and structural characteristics of corneal epithelial cells.
The adaptation of cellular microprojections and their microstructures
Mudskippers live in a wide range of habitats, from shallow water to intertidal flats and supralittoral zones, which is the most drastically changing intertidal environment in terms of moisture content, salinity, temperature, and oxygen level. Coastal and intertidal habitats are at the forefront of anthropogenic influence and environmental change, especially global warming (Wolfe et al., 2020). The change of the intertidal ambient (such as temperature, humidity, oxygen content, etc.) will lead to the corresponding change of the biodiversity pattern and the adaptation mechanism of the species living in the area (Bates et al., 2018; Vafeiadou et al., 2018). To adapt to various aquatic and terrestrial environments, the cornea, an important visual structure, has evolved various adaptive features, including changes in cell density, microridges, microplicae, microholes, and microvilli. Previous studies have shown that Periophthalmus, Boleophthalmus, and Scartelaos have evolutionarily adaptive traits related to vision (Ansell et al., 1993; You et al., 2014; DA, 2017; You et al., 2018; Hu et al., 2022).
The corneal surface in the vertebrate eye features microprojections formed by folds of the epithelial cell membranes. These microprojections increase the surface area available for the transport of nutritional and waste products across the cell surface (Collin and Collin, 2000a). Superficial epithelial cells are covered by numerous microprojections (microridges, microvilli, microplicae, and microholes) (Collin and Collin, 2000b; Collin et al., 2021). In different habitats, the corneal microstructure and cellular morphology of these vertebrates have evolved corresponding adaptations. The clear interspecific differences in corneal surface structure suggested adaptive plasticity in the composition and stabilization of the cornea in various aquatic environments.
Collin and Collin (2006) found that microridges narrowed as the dependence on the aquatic environment decreased (from water to land). Moreover, it has been shown that the fingerprint-like patterns of corneal microridges are a ubiquitous feature of marine teleosts, covering many types of sensory epithelia. In other words, terrestrial vertebrates, with a less stringent requirement for a physically stable tear film, feature microplicae and/or microvilli in place of microridges (Collin and Collin, 2000a; Collin and Collin, 2000b; Collin and Collin, 2006). In this study, microridge widths of fish in the Oxudercinae subfamily showed a trend similar to that reported by Collin and Collin. As shown in Table 2, the microridges disappeared in animals that were typically terrestrial or amphibious, while the structures could still be found in the typically aquatic A. butcheri and an amphibious group of Oxudercinae. On the other hand, between the microplicae, there were numerous holes or pits (microholes), which were found only in terricolous species, such as C. ornatus and amphibians, as well as amphibious mudskippers that are usually exposed to air. Generally, corneal microstructures might be influenced by the external environment. Further studies are needed to confirm whether corneal epithelial cell density also affects microstructures.
In terms of cell type, microridge cells were observed in fishes with aquatic characteristics, namely, A. butcheri, Ps. elongatus, S. histophorus, and B. pectinriostris; microridges are among the least efficient microstructures for increasing the surface area of the cell membrane (Hu et al., 2016). Reticular cells were another type of cell with a strong secretion function that appeared in B. pectinriostris, P. magnuspinnatus, and A. mexicanum. This suggested that corneal secretions were higher in these three species, facilitating the maintenance of moist eyes. Both microridge cells and reticular cells were found in adult B. pectinriostris and A. mexicanum at the same time, which suggested that these mixed cell types enhanced the adaptive capacity of eyes in terrestrial environments. Further research is required to determine whether they have any functions other than those associated with osmolality, mucus secretion, and substance exchange.
Conclusion
The amphibious traits of Oxudercinae species are necessary for living under relatively dry conditions on land. This phenomenon suggests that the vision of this group may have special mechanisms in amphibious environments. According to our results, there were microstructures such as microridges, microvilli, and microplicae on the corneal epithelium of Oxudercinae species with different numbers of epithelial cells. In the amphibious habitats to which Oxudercinae species are adapted, the corneal structure has evolved. Adaptive changes have been observed in the corneal epithelium of mudskippers with amphibious characteristics as a result of differences in the level of dependence on the aquatic environment. With increasing dependence, the corneal epithelial cell density tended to decrease, and the microridge width narrowed. More studies will be required to investigate the relationships between the width of microridges and the separation, function and adaptability of corneal cells.
Data availability statement
The original contributions presented in the study are included in the article/Supplementary Material. Further inquiries can be directed to the corresponding authors.
Ethics statement
The animal study was reviewed and approved by Laboratory Animal Welfare Ethics Review Committee, Institute of Zoology, Chinese Academy of Sciences. The approval number: AEI-04-03-2014.
Author contributions
WH participated in the determination of research and writing ideas, designed and completed the experiment of fish physiology and scanning electron microscopy, completed the data collection and manuscript writing of this part, and completed the manuscript submission. YM participated in the determination of research and writing ideas and completed the data collection, manuscript writing, and paper improvement. JZ provided overall guidance to the research and writing ideas and participated in the writing of the whole manuscript. YG participated in the discussion and paper revision. CW participated in the writing and modification of part of the manuscript. All authors contributed to the article and approved the submitted version.
Funding
Grants supporting this research were received from the Erhai Watershed Ecological Environment Quality Testing Engineering Research Center of Yunnan Provincial Universities to WH and YM (No. DXDGCZX03), from the start-up projects on high-level talent introduction of Dali University to YM (No. KY1916101940), and from the Chinese Academy of Sciences (XDA19050202) to JZ.
Acknowledgments
We thank Shengguo Chen for helping collect specimens and for support in the field. Appreciate for JSPS Invitation Fellowship Programs for Research in Japan to JZ.
Conflict of interest
The authors declare that the research was conducted in the absence of any commercial or financial relationships that could be construed as a potential conflict of interest.
Publisher’s note
All claims expressed in this article are solely those of the authors and do not necessarily represent those of their affiliated organizations, or those of the publisher, the editors and the reviewers. Any product that may be evaluated in this article, or claim that may be made by its manufacturer, is not guaranteed or endorsed by the publisher.
Supplementary material
The Supplementary Material for this article can be found online at: https://www.frontiersin.org/articles/10.3389/fmars.2022.1065358/full#supplementary-material
References
Ansell A., Gibson R., Barnes M., Clayton D. (1993). Mudskippers. Oceanogr. Mar. Biol. Ann. Rev. 31, 507–577.
Avery J. A., Bowmaker J. K. (1982). Visual pigments in the four-eyed fish, Anableps. Nature. 298, 62–63. doi: 10.1038/298062a0
Baeck G. W., Takita T., Yang H. Y. (2008). Lifestyle of Korean mudskipper Periophthalmus magnuspinnatus with reference to a congeneric species Periophthalmus modestus. Ichthyol. Res. 55, 43–52. doi: 10.1007/s10228-007-0009-y
Bates A. E., Helmuth B., Burrows M. T., Duncan M. I., Garrabou J., Guy-Haim T., et al. (2018). Biologists ignore ocean weather at their peril. Nature 560, 299–301. doi: 10.1038/d41586-018-05869-5
Beuerman R. W., Pedroza L. (1996). Ultrastructure of the human cornea. Microsc. Res. Tech. 33, 320–335. doi: 10.1002/(sici)1097-0029(19960301)33:4<320::aid-jemt3>3.0.co;2-t
Bucholtz R. H., Meilvang A. S. (2005). Biology and utilization of the mudskipper pseudapocryptes elongatus in the Mekong river delta, with aspects on other mudskippers in the area (Denmark: Department of Marine Ecology and Centre for Tropical Ecosystem Research, University of Aarhus).
Bucholtz R. H., Meilvang A. S., Cedhagen T., Christensen J. T., Macintosh D. J. (2009). Biological observations on the mudskipper Pseudapocryptes elongatus in the Mekong delta, Vietnam. J. World Aquac. Soc 40, 711–723. doi: 10.1111/j.1749-7345.2009.00291.x
Burggren W. W. (1997). Air-breathing fishes: Evolution, diversity, and adaptation (San Diego, CA: Academic Press).
Collin H. B., Collin S. P. (2000a). The corneal surface of aquatic vertebrates: microstructures with optical and nutritional function? Philos. Trans. R. Soc Lond. B. Biol. Sci. 355, 1171–1176. doi: 10.1098/rstb.2000.0661
Collin S. P., Collin H. B. (2000b). A comparative SEM study of the vertebrate corneal epithelium. Cornea. 19, 218–230. doi: 10.1097/00003226-200003000-00017
Collin S. P., Collin H. B. (2006). The corneal epithelial surface in the eyes of vertebrates: environmental and evolutionary influences on structure and function. J. Morphol. 267, 273–291. doi: 10.1002/jmor.10400
Collin H. B., Ratcliffe J., Collin S. P. (2021). The functional anatomy of the cornea and anterior chamber in lampreys: insights from the pouched lamprey, Geotria australis (Geotriidae, Agnatha). Front. Neuroanat. 15. doi: 10.3389/fnana.2021.786729
DA C. (2017). “Feeding behavior: a review,” in Fishes out of water: Biology and ecology of mudskippers. Eds. Jaafar Z., Murdy E. O. E. (Boca Raton: CRC Press.).
Davies W., Collin S. P., Hunt D. M. (2012). Molecular ecology and adaptation of visual photopigments in craniates. Mol. Ecol. 21, 3121–3158. doi: 10.1111/j.1365-294X.2012.05617.x
Derbalah A., El-Gendy S. A., Alsafy M. A., Elghoul M. (2022). Micro-morphology of the retina of the light-adapted African catfish (Clarias gariepinus). Microsc. Res. Tech., 1–8. doi: 10.1002/jemt.24252
Graham J. B. (1997). Air breathing fishes: Evolution, diversity and adaptation (San Diego, CA: Academic Press).
Hauser F. E., Chang B. S. (2017). ). insights into visual pigment adaptation and diversity from model ecological and evolutionary systems. Curr. Opin. Genet. Dev. 47, 110–120. doi: 10.1016/j.gde.2017.09.005
Hauser F. E., Ilves K. L., Schott R. K., Castiglione G. M., López-Fernández H., Chang B. S. W. (2017). Accelerated evolution and functional divergence of the dim light visual pigment accompanies cichlid colonization of central America. Mol. Biol. Evol. 34, 2650–2664. doi: 10.1093/molbev/msx192
Hora S. L. (1936). Ecology and bionomics of the gobioid fishes of the gangetic delta. Int. Congr. Zool. 12, 841–863.
Hu W. X., Mu Y., Lin F., Li X., Zhang J. (2022). New insight into visual adaptation in the mudskipper cornea: from morphology to the cornea-related COL8A2 gene. Front. Ecol. Evol. 10. doi: 10.3389/fevo.2022.871370
Hu W. X., Zhang J., Kang B. (2016). Structure and function of corneal surface of mudskipper fishes. Fish Physiol. Biochem. 42, 1481–1489. doi: 10.1007/s10695-016-0234-2
Ishimatsu A., Gonzales T. T. (2011). “Mudskippers: front runners in the modern invasion of land,” in The biology of gobies. Eds. Patzner R. A., Tassell J.L.V., Kovačić M., Kapoor B. G. (Enfield, NH: Science Publishers Inc), 609–638.
Jaafar Z., Larson H. K. (2008). A new species of mudskipper, Periophthalmus takita (Gobiidae: Oxudercinae), from Australia, with a key to the genus. Zoolog. Sci. 25, 947. doi: 10.2108/zsj.25.946
Kirkpatrick L. A., Feeney B. C. (2012). A simple guide to IBM SPSS statistics for version 20.0. (Student ed. Wadsworth, Cengage Learning, Belmont).
Kröger R. H. H. (1992). Methods to estimate dispersion in vertebrate ocular media. J. Opt. Soc Am. A. 9, 1486–1490. doi: 10.1364/josaa.9.001486
Mass A. M., Supin A. Y. (2007). Adaptive features of aquatic mammals’ eye. Anat. Rec. (Hoboken) 290, 701–715. doi: 10.1002/ar.20529
Milward N. E. (1974). Studies of the taxonomy, ecology, and physiology of Queensland mudskippers (Australia: University of Queensland).
Murdy E. O. (1989). A taxonomic revision and cladistic analysis of the oxudercine gobies (Gobiidae: Oxudercinae). Rec. Aust. Mus. Suppl. 11, 1–93. doi: 10.3853/j.0812-7387.11.1989.93
Nursall J. R. (1981). Behavior and habitat affecting the distribution of five species of sympatric mudskippers in Queensland. Bull. Mar. Sci. 31, 730–735.
Owens G. L., Windsor D. J., Mui J., Taylor J. S. (2009). A fish eye out of water: ten visual opsins in the four-eyed fish, Anableps anableps. PloS One 4, e5970. doi: 10.1371/journal.pone.0005970
Polgar G., Bartolino V. (2010). Size variation of six species of oxudercine gobies along the intertidal zone in a Malayan coastal swamp. Mar. Ecol. Prog. Ser. 409, 199–212. doi: 10.3354/meps08597
Reuter T., Peichl L. (2008). “Structure and function of the retina in aquatic tetrapods,” in Sensory evolution on the threshold: Adaptations in secondarily aquatic vertebrates. Eds. Thewissen J. G. M., Nummela S. (Berkeley: University of California Press), 149–172. doi: 10.1525/9780520934122-011
Savolainen O., Lascoux M., Merilä J. (2013). Ecological genomics of local adaptation. Nat. Rev. Genet. 14, 807–820. doi: 10.1038/nrg3522
Sayer M. D. J. (2005). Adaptations of amphibious fish for surviving life out of water. Fish Fish. 6, 186–211. doi: 10.1111/jfb.12270
Simmich J., Temple S. E., Collin S. P. (2012). A fish eye out of water: epithelial surface projections on aerial and aquatic corneas of the 'four-eyed fish' Anableps anableps. Clin. Exp. Optom. 95, 140–145. doi: 10.1111/j.1444-0938.2011.00701.x
Steppan S. J., Meyer A. A., Barrow L. N., Alhajeri B. H., Al-Zaidan A. S. Y., Gignac P. M., et al. (2022). Phylogenetics and the evolution of terrestriality in mudskippers (Gobiidae: Oxudercinae). Mol. Phylo. Evol. 169, 107416. doi: 10.1016/j.ympev.2022.107416
Subramanian S., Ross N. W., MacKinnon S. L. (2008). Comparison of antimicrobial activity in the epidermal mucus extracts of fish. Comp. Biochem. Physiol. B. Biochem. Mol. Biol. 150, 85–92. doi: 10.1016/j.cbpb.2008.01.011
Swennen C., Ruttanadakul N., Haver M., Piummongkol S., Prasertsongskum S., Intanai I., et al. (1995). The five sympatric mudskippers (Teleostei: Gobioidea) of pattani area, southern Thailand. Nat. Hist. Bull. Siam. Soc 42, 109–129.
Takegaki T., Fujii T., Ishimatsu A. (2006). Overwintering habitat and low-temperature tolerance of the young mudskipper Boleophthalmus pectinirostris. Nihon-suisan-gakkai-shi 72, 880–885. doi: 10.2331/suisan.72.880
Takita T., Agusnimar, Ali A. B. (1999). Distribution and habitat requirements of oxudercine gobies (Gobiidae: Oxudercinae) along the straits of malacca. Ichthyol. Res. 46, 131–138. doi: 10.1007/BF02675431
Townsend K. A., Tibbetts I. R. (2005). Behaviour and sexual dimorphism of the blue mudskipper, Scartelaos histophorus (Pisces: Gobiidae). P. R. Soc Queensland. 112, 53–62.
Vafeiadou A. M., Bretaña B. L. P., Van Colen C., Dos Santos G. A. P., Moens T. (2018). Global warming-induced temperature effects to intertidal tropical and temperate meiobenthic communities. Mar. Environ. Res. 142, 163–177. doi: 10.1016/j.marenvres.2018.10.005
Vega G. C., Wiens J. J. (2012). Why are there so few fish in the sea? Proc. Biol. Sci. 279, 2323–2329. doi: 10.1098/rspb.2012.0075
Wolfe K., Nguyen H. D., Davey M., Byrne M. (2020). Characterizing biogeochemical fluctuations in a world of extremes: a synthesis for temperate intertidal habitats in the face of global change. Glob. Change Biol. 26 (7), 3858–3879. doi: 10.1111/gcb.15103
Wu H. L., Zhong J. S. (2008). FAUNA SINICA: PISCES, ORDER PERCIFORMES, SUBORDER GOBIOIDEI (Beijing: Science Press).
You X. X., Bian C., Zan Q. J., X. Xu X., Liu J., Chen J. M., et al. (2014). Mudskipper genomes provide insights into the terrestrial adaptation of amphibious fishes. Nat. Commun. 5, 5594. doi: 10.1038/ncomms6594
Keywords: oxudercinae, corneal epithelial cells, amphibian fish adaptation, epithelial cell density, microstructure
Citation: Hu W, Mu Y, Wei C, Gai Y and Zhang J (2022) From morphological to ecological adaptation of the cornea in Oxudercinae fishes. Front. Mar. Sci. 9:1065358. doi: 10.3389/fmars.2022.1065358
Received: 09 October 2022; Accepted: 23 November 2022;
Published: 15 December 2022.
Edited by:
Hongsheng Yang, Institute of Oceanology (CAS), ChinaReviewed by:
Chenguang Feng, Northwestern Polytechnical University, ChinaMohamed Alsafy, Alexandria University, Egypt
Usha Kumari, Banaras Hindu University, India
Copyright © 2022 Hu, Mu, Wei, Gai and Zhang. This is an open-access article distributed under the terms of the Creative Commons Attribution License (CC BY). The use, distribution or reproduction in other forums is permitted, provided the original author(s) and the copyright owner(s) are credited and that the original publication in this journal is cited, in accordance with accepted academic practice. No use, distribution or reproduction is permitted which does not comply with these terms.
*Correspondence: Jie Zhang, emhhbmdqaWVAaW96LmFjLmNu; Yuan Mu, bXV5QGVhc3Rlcm4taGltYWxheWEuY24=