- 1School of Environmental and Civil Engineering, Jiangnan University, Wuxi, China
- 2Jiangsu Collaborative Innovation Center of Technology and Material of Water Treatment Suzhou, University of Science and Technology, Suzhou, China
- 3Freshwater Fisheries Research Centre of Chinese Academy of Fishery Sciences, Ministry of Agriculture, Wuxi, China
Dissolved organic matter (DOM) is likely to coexist with microplastics (MPs) in aquatic environments. However, little is known about the effects of different DOM fractions on the stability and aquatic toxicity of MPs. In this study, we separated humic acid (HA) into three molecular weight (MW) fractions (> 30, 3–30, and < 3 k Da) and examined their effects on the toxicity of polyamide 66 (PA66)-MPs to red tilapia (Oreochromis niloticus). Generally, addition of HA enhanced the stability and thus the exposure of tilapia to MPs, leading to the increased accumulation of MPs in the gut, liver, and brain. However, compared with MPs alone, the accumulation of MPs in the gills of tilapia was reduced. Compared with the presence of high-MW HA, the accumulation of MPs in the fish liver was significantly enhanced by 1.1 times in the presence of medium-MW HA (m-HA) after a 10-day exposure. The protein peroxidation on the liver induced by MPs was alleviated by adding all HA fractions. However, compared with MPs alone, the presence of m-HA resulted in a more severe lipid peroxidation, while the presence of low-MW HA alleviate the lipid peroxidation caused by MPs. In addition, the Integrated Biological Responses version 2 (IBRv2) results suggested that the oxidative stress in the liver tilapia caused by MPs could be alleviated by the presence of all HA fractions, which was largely related to the reduced damage caused by lipid peroxidation and/or protein peroxidation. Collectively, our results suggest that the presence of different MW HA fractions could induce complex changes in the MP toxicity on aquatic organisms.
1 Introduction
Over the last few decades, concern over plastic pollution in aquatic ecosystems has increased owing to the growing global accumulation of plastic products and their resistance to degradation. Plastic particles with sizes less than 5 mm are defined as microplastics (MPs) (Thompson et al., 2004). The presence of MPs in aquatic environments worldwide has been demonstrated in previous studies (de Sá et al., 2018; Wang et al., 2021a; Xu et al., 2020; Bordós et al., 2019). Furthermore, adverse effects of MPs on aquatic organisms, including biochemical perturbations, alterations in gene expression, and malformations in neonates, have been reported (Cole and Galloway, 2015; Ding et al., 2018; Huang et al., 2021; Kokalj et al., 2018; De Marco et al., 2022; Zhang et al., 2019; Eltemsah and Bøhn, 2019). Despite these studies, there are gaps in our knowledge of the toxicity of MPs in aquatic environments. For example, less attention has been paid to the potential influences of environmental factors on the aquatic toxicity of MPs, which may result in a misunderstanding of the risks posed by MPs in aquatic ecosystems.
Dissolved organic matter (DOM) is a ubiquitous component of aquatic systems and consists of complex structured decomposition products from bacteria, animals, algae, or plants (Blewett et al., 2018). DOM can be adsorbed by MPs via ligand exchange, electrostatic interactions, and hydrophobic interactions (Tallec et al., 2019). It is an important factor affecting the existential form and stability of MPs in aquatic environments (Wang et al., 2021b; Sharma et al., 2021), which may alter the aquatic toxicity of MPs. Some studies have reported that the presence of DOM protects against the toxicity of MPs to aquatic organisms. Schür et al. (2021) found a lower mortality of Daphnia magna exposed to wastewater-incubated MPs than those exposed to pristine MPs and hypothesized that the results might be due to the adsorption of DOM. The adsorption of DOM may stabilize the suspension and alleviate the entanglement of plastic particles on Daphnia magna, which consequently reduced the body burden of D. magna (Wu et al., 2019). Similarly, Liu et al. (2019a) found that the addition of 5 mg L-1 humic acid (HA), a typical DOM, significantly alleviated the toxicity of 0.1 μm-MPs to the algae Scenedesmus obliquus after a 48 h exposure, but this alleviation was absent in the case of the 2 μm PS-MP exposure. In contrast, the presence of DOM could exacerbate the toxicity of MPs in some studies, such as increasing the accumulation of MPs in the liver and gut of zebrafish Danio rerio (Qiao et al., 2019) and inducing oxidative stress in S. obliquus and D. rerio (Liu et al., 2019b). Therefore, the impact of DOM on MP toxicity is complex, and further studies on the interactive effects of MPs and DOM are urgently needed.
The molecular weight (MW) of DOM can range from several hundred to tens of thousands in aquatic environments (Piccolo, 2001). Different MW DOM fractions with specific chemical properties may interact with co-existing contaminants in different ways, leading to complicated changes in the aquatic toxicity of co-existing contaminants (Zhu et al., 2020; Wu et al., 2020). Bai et al. (2020) separated HA into four different MW fractions and found that the bioaccumulation of Cd and Pb in earthworm Eisenia fetida and the inhibition rates of earthworm growth both increased with increasing MW of HA. These authors attributed the results mainly to the stronger complexation capacities of lower MW HA fractions with heavy metals. Zhu et al. (2020) found that the addition of HA reduced the aggregation of iron oxide nanoparticles (γ-Fe2O3) in suspension and accelerated the process of nanoparticles entering the cells of the fungus Phanerochaete chrysosporium, which induced the production of reactive oxygen species (ROS) and increased the activities of catalase (CAT) and peroxidase (POD). Furthermore, compared to the higher-MW HA, γ-Fe2O3 could more easily enter the fungal cells and trigger higher toxicity in the presence of lower-MW HA. In analogy to the results from heavy metals and engineered nanomaterials (ENMs), we assumed that the impacts of DOM fractions on the aquatic toxicity of MPs may be MW-dependent.
Therefore, the aim of this study was to clarify the impact of different MW DOM fractions on the stability and aquatic toxicity of MPs. Red tilapia (Oreochromis niloticus) was selected as a model organism for this study. It is an important fish species in aquatic ecosystems, and our previous studies have confirmed the ingestion and accumulation of MPs in different tissues of red tilapia, leading to accompanying perturbations in biochemical systems (Ding et al., 2018; Ding et al., 2020; Zhang et al., 2019; Huang et al., 2021). Polyamide (PA) was one of the most commonly detected MP types in the aquatic environment and the stomach of fish (de Sá et al., 2018; Khosrovyan et al., 2020). However, compared with other common MPs such as polystyrene (PS), polyethylene (PE), and polypropylene (PP), the ecological risk of PA was yet to be assessed at the same extent. Thus, in this study, polyamide 66 (PA66)-MPs, one of the most commonly used PA, was selected as the model MPs. In this study, the influence of DOM fractions with different MWs on the stability of MPs was investigated. The accumulation and distribution of PA66-MPs in fish tissues were assessed in the presence of DOM fractions with different MWs. The antioxidant effects were evaluated by determining several biomarkers in the fish liver, including glutathione-S-transferase (GST), malondialdehyde (MDA), and protein carbonyl (PC).
2 Materials and methods
2.1 HA fractionation
HA containing ≥ 90% fulvic acid (FA) was used as an alternative to DOM in this study. It was purchased from Aladdin (Shanghai, China) and was prepared in water to obtain a 1 g L-1 HA solution. The elemental composition of HA was provided by the manufacturer and presented in Table S1. Based on previous studies (Wu et al, 2020; Zhang et al, 2021), the HA solution was fractionated into three fractions (i.e., < 3, 3–30, and > 30 kDa) using an ultrafiltration device. The low-, medium-, and high-MW HA fractions were denoted as l-HA, m-HA, and h-HA, respectively. The three HA fractions were then stored at 4 °C.
2.2 Characterization of HA
The concentrations of different HA fractions were quantified using a total organic carbon (TOC) analyzer (Shimadzu Corp., Kyoto, Japan). Using Milli-Q water as the blank, UV-Vis spectra of different HA fractions were determined with a UV-Vis spectrophotometer (UV-2600, Shimadzu Corp., Kyoto, Japan) from 185 to 800 nm in 1 nm increments, followed by dilution to 5 mg L-1 dissolved organic carbon (DOC). Specific UV-Vis absorbance (UV254) was used to determine the aromaticity of each HA fraction.
The HA fractions were frozen at −80°C and dried in a freeze dryer (Alpha 1-4 LSCbasic, BMH Instruments Co. Ltd., Beijing, China) to prepare the HA powders. Fourier transform infrared (FTIR, IRTracer-100, Shimadzu Corp., Kyoto, Japan) spectra were measured by pressing 1 mg of dry powdered KBr-HA mixture sample (KBr : HA=200:1) after subtracting the blank of KBr, and the FTIR scan range was set at 525–4000 cm-1 at 1.0 cm-1 intervals.
The different HA fractions were characterized via three-dimensional fluorescence excitation emission matrix (3D-EEM) spectroscopy (F-7000, Hitachi Co. Ltd., Tokyo, Japan) by collecting emission spectra over a range of excitation (Ex) (200–450 nm) and emission (Em) wavelengths (250–550 nm). The main parameters of 3D-EEM spectra were as follows: Photomultiplier (PMT) voltage, 500.0 V; Ex and Em sampling interval, 5.0 nm; Ex and Em slit, 5.0 nm; and scan speed, 1200 nm min-1.
2.3 Stability of MPs under different MW HA fractions
PA66 particles without fluorescent dyes were purchased from the Shengbang Plastic Raw Material Co., Ltd, Shenzhen, China. Particles in size of 75–80 μm were obtained through screens. Subsequently, the particles were photographed using a stereoscopic microscope (PXS8-T, Fenye Photoelectric Instrument Equipment Co., Ltd., Shanghai, China) (Figure S1A). The particle size (76.4 ± 3.2 μm) and distribution were determined by Image J (version 1.53k) (Figure S1B). A suspension of MPs (200 mg L-1) was mixed with different MW HA fractions (0, 5, 10, and 20 mg L-1). The samples were placed in an ultrasonic bath for 5 min before use and transferred to a UV-Vis spectrophotometer (UV-2600, Shimadzu Corp., Kyoto, Japan) for measuring sedimentation. The sedimentation experiments were conducted in a drive time mode for 30 min to monitor the variation in absorbance at 195 nm as a function of time. UV-Vis spectra were recorded at intervals of 1 s. Variations in absorbance (A/A0) were used to represent the variations in the particle concentration in the suspension of MPs (C/C0) (Wu et al., 2020). The solutions of three HA fractions with different concentrations were also processed in the identical manner to ruled out the effects of HA itself.
2.4 Animals and exposure
Healthy red tilapia from the same lineage were kindly provided by the Chinese Academy of Fishery Sciences (Wuxi, China). The fish were maintained in dechlorinated tap water (pH 6.9–7.1 and CaCO3 114.7 ± 5.1 mg L-1) at 25 ± 1 °C under a 12 h/12 h (light/dark) cycle. Before the experiments, the fish were acclimated for 2 weeks. The fish were fed once a day with commercial pellet food (Dayu Aquarium Co., Ltd., Guangzhou, China) in an amount of 3–6% of their body weight, which stopped until four days prior to the beginning of the exposure experiment. Fecal material and uneaten food were removed every day. No aeration was performed throughout the acclimation and subsequent exposure periods, and the dechlorinated tap water (aeration ≥ 24 hours) was replaced every day.
The following experiments were approved by the Animal Care and Use committee of Jiangnan University. The methods of all experiments were carried out in accordance with the National Institutes of Health Guide for the Care and Use of Laboratory Animals of China. After being starved, twelve fish were randomly placed into a 36 L tank containing 30 L of dechlorinated tap water. The fish (54.5 ± 3.5 g wet weight; total standard length 14.6 ± 1.1 cm long) were exposed to six treatments: control (fresh water only), MPs alone, l-HA+MPs, m-HA+MPs, and h-HA+MPs. The exposure concentrations of MPs and HA fractions (l-HA, m-HA, and h-HA) were 100 μg L-1 and 5 mg L-1, respectively. The exposure concentrations of MPs were selected based on the concentrations reported in the water environment and the effective concentrations of MPs on red tilapia reported in previous study (Goldstein et al., 2012; Sul et al., 2014; Ding et al., 2020). The selection of HA concentrations were also based on the concentrations reported in the water environment (Blewett et al., 2018) and the the previous literature on the effect of DOM on MPs toxicity (Liu et al., 2019a; Liu et al., 2019b; Qiao et al., 2019). Each treatment was conducted with three replicates. A total of 15 tanks were applied in this study. All the experiments lasted for 20 d, which included a 10 d uptake period followed by a 10 d depuration period. The fecal pellets in each tank were removed every day, and the exposure media (uptake period) or fresh water (depuration period) were renewed every day. At 0, 10, and 20 d, four fish from each tank were sampled, rinsed with methanol to remove particles from the skin, and then anesthetized with buffered MS-222. The fish were euthanized by cervical transection. The tissues (liver, gut, gills, and brain) were removed immediately, washed in 0.15 M KCl, weighed, and stored at -80 °C for further processing. In the four sampled fish, two of them were mixed to quantify the MPs in tissues, and the remaining two fish were mixed for antioxidant analyses.
2.5 Quantitation of MPs in fish tissues
The MPs in fish tissues (gills, gut, liver and brain) were quantified by the Nile Red (NR) fluorescent staining method (Erni-Cassola et al., 2017; Wang et al., 2020). The tissue samples were digested in 20 mL of 100 g L-1 KOH at 60 °C for 24 h. The digesting solution was filtered through a 0.22 μm polycarbonate track-etched membranes (PCTE, 47 mm diameter, Whatman, USA.) and dried in glass Petri dishes at 40 °C for 12 h. The NR working solution (1 μg L-1) was prepared by dissolving NR dye (98.0%, technical grade, Aladdin, Shanghai, China) in methanol. The PCTE membrane was placed on the microscope slide, then two drops of the NR working solution were carefully added onto the membrane using a glass dropper. The samples were maintained at 60 °C for 15 min in dark. PA66-MPs with NR on PCTE membranes were identified by using a fluorescence microscopy (DSY5000X, Chongqing Photoelectric Instrument Co., Ltd., Chongqing, China). The fluorescence images MPs in fish tissues was tested in bright field and green fluorescence field (Ex: 460 nm, Em: 515–565 nm, 20×). For the determination of the MP concentrations in fish tissues, the NR stained MPs were transferred into 25 mL of Milli-Q water to prepare MP suspensions. Afterward, the fluorescence intensity in green (Ex/Em at 460/525 nm) of different suspensions was measured by using a fluorescence spectrophotometer (F-7000). The green fluorescence was chosen because (1) MPs fluoresced better in green, (2) the fluorescence of HA and natural contaminants after digestion could be ignored (Erni-Cassola et al., 2017; Qiao et al., 2019). The fish tissues of the control group was also processed in the identical manner as exposed fish tissues to obtain the background fluorescence, which was subtracted from that of the treatment samples. The standard curve was generated by serial dilutions of NR stained MP suspensions (Figure S2). The MP concentrations in the fish tissues were calculated from the standard curve.
2.6 Antioxidant analyses
The liver samples of red tilapia were homogenized in nine volumes of cold buffer (0.15 M KCl, 0.1 M Tris-HCl, pH 7.4) and centrifuged for 25 min (10,000 ×g) at 4 °C. The enzyme was extracted from the supernatant, and its activity was measured with a microplate reader (Synergy H4, Biotek, VT., USA). The content of MDA and PC, as well as the activity of GST, were measured with the commercial kits (Comin Biotechnology Co., Ltd., Suzhou, China).
2.7 Data analysis
The data in the study was expressed as the mean ± standard deviation (SD). One-way ANOVA followed by least significant difference (LSD) post hoc test was applied to evaluate the significant differences within or across various treatments and exposure times. All significant differences were considered at p < 0.05. Statistical analyses were performed using the SPSS statistical package (version 26.0, SPSS Company, USA). Moreover, the index of “Integrated Biological Responses version 2” (IBRv2) was applied to combine all the measured biomarker responses into one general “stress index”. According to Sanchez et al. (2013), the method of IBRv2 could be found in the Supplemental Material.
3 Results and discussion
3.1 Characterization of different MW HA fractions
As shown in Figure 1A, the different HA fractions (l-HA, m-HA, and h-HA) displayed strong absorbance in the UV-Vis range. At the same concentration of 5 mg L-1, the absorbance at 254 nm followed the order l-HA < m-HA < h-HA, indicating that the aromaticity of HA increased with increasing MW. This may be attributed to the relatively high amount of aromatic or polyphenolic structures in the high-MW fraction (Wu et al., 2020). As shown in Figure 1B, all HA fractions showed major peaks at 1390 (attributed to the vibrations of symmetric COO- stretching and aliphatic bending), 1600 (attributed to the stretching vibrations of aromatic C=C, olefinic C=C, or asymmetric COO- groups), and 3425 (attributed to the vibrations of O-H stretching for carboxylic and alcoholic groups) cm-1 (Francioso et al., 1996; Ribeiro et al., 2001; Wu et al., 2020; Li et al., 2020). At 1600 cm-1, the intensity of the peak increased with increasing MW of the HA fraction. This was consistent with the UV-Vis spectra, which indicated that the HA fraction with a high MW contained a higher amount of aromatic substances. In addition, the peaks at 1600 and 3425 cm-1 in h-HA were much stronger than those in m-HA and l-HA, suggesting that the high-MW fraction contained more carboxyl, aliphatic, and alcoholic groups.
As shown in Figure S3, peaks of different HA fractions were observed over the range of Ex wavelengths of 265–285 nm and Em wavelengths of 430–500 nm. The strongest peaks were observed at approximately 275/460 nm (Ex/Em), which are classified as HA-like substances (Chen et al., 2003). In addition, the fluorescence intensities of the different HA fractions followed the order l-HA > m-HA > h-HA. This was consistent with the study by Bai et al. (2019), who also found that the fluorescence intensity of the HA fractions decreased with increasing MW. This result may be attributed to the low content of fluorescent chromophores (e.g., aldehydes and quinones) in the high-MW HA fractions per unit of DOC (Ren et al., 2017).
3.2 Stability of MPs under different MW HA fractions
As shown in Figures 2A–C, the C/C0 of three HA fractions with different concentrations were tended to be 1.00, suggesting that the sedimentation of HA fractions could be negligible. The changes in the sedimentation of the MP suspension in the presence of different HA fractions are shown in Figures 2D–F. The C/C0 of the MP suspension without HA decreased after 30 min, implying the sedimentation of MPs. However, the decrease in C/C0 of the MP suspension with HA fractions was reduced, suggesting that the addition of HA fractions could decrease the degree of MP sedimentation and increase the stability of the MP suspension. In addition, the C/C0 of the MP suspension increased with increasing concentrations of different HA fractions (5–20 mg L-1), with an increase rate between 10.3% and 20.3%, revealing that the stability of MPs increased with increasing concentration of HA fractions.
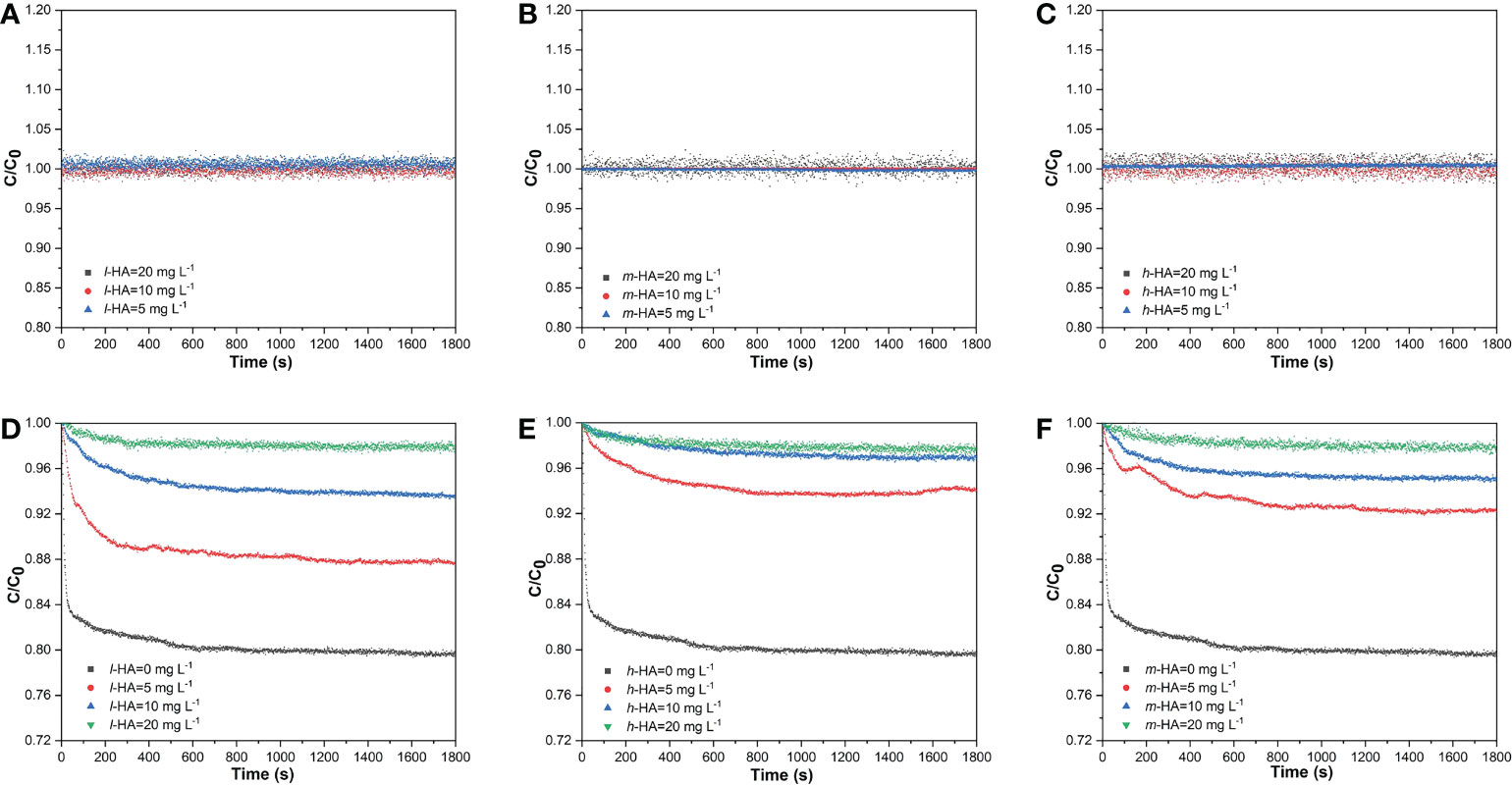
Figure 2 Effects of different HA fractions (l-HA, and h-HA) with various concentration on the sedimentation of MPs as a function of time. (A–C) the sedimentations of different HA fractions (D–F) the sedimentation of MPs in the presence of different HA fractions.
HA can be adsorbed onto the MP surface through ligand exchange, electrostatic interactions, and hydrophobic interactions (Tallec et al., 2019), which may form a coating on the surface of the MPs. The adsorbed HA could carry negative charge onto MPs as pH from 4.0 to 8.0 due to the presence of oxygen-containing functional groups (Zhu et al., 2014; Wu et al., 2021), reduce the sedimentation of MPs by increasing steric hindrance and electrostatic repulsion (Tallec et al., 2019; Wu et al., 2019; Yu et al., 2019), and thus stabilize the suspension. Therefore, in this study, the stabilities of the MP suspensions in the presence of different HA fractions were higher than those without HA. Our results also indicated that the stability of MPs increased with increasing concentrations of the different HA fractions. This finding may be related to the adsorption capacity of the MPs at different HA concentrations. At 5 mg L-1 HA, there was an incomplete saturation of HA adsorbed onto the MP surfaces. As the concentration of HA increased, HA significantly enhanced the stability of MPs, possibly because of the higher adsorption of HA onto the MP surface, which would provide larger steric hindrance and electrostatic repulsion. A similar phenomenon has been observed in a previous study on nanoparticles, in which the stability of nanoscale zero-valent iron (NZVI) particles increased with increasing HA concentrations (0–20 mg L-1) (Wu et al., 2020).
At HA concentrations of 5 and 10 mg L-1, the C/C0 of MPs increased with increasing MW of the HA fraction after 30 min, revealing that the higher-MW HA fraction could inhibit MP sedimentation and better stabilize the MP particles. Previous studies have reported that DOM with higher aromaticity exhibits higher adsorption on the particle surface (Jiang et al., 2017; Wu et al., 2020; Li et al., 2020). In this study, the aromaticity of h-HA was higher than that of the lower MW fractions, which may have led to higher adsorption of HA onto the MP surfaces. In addition, the high MW HA fractions could exert larger steric hindrance due to their higher molecular weights and larger radius of gyration (Li et al., 2020; Louie et al., 2013; Wu et al., 2020). Furthermore, the adsorption of h-HA with more COO- groups onto the MP surfaces might also lead to a higher negative charge compared to those in m-HA and l-HA (Wu et al., 2020). Therefore, compared with l-HA and m-HA, h-HA exerted a larger steric hindrance and caused higher electrostatic repulsion, thus resulting in a higher stability of the MPs. Similarly, Wu et al. (2020) separated HA into four MW fractions (< 10, 10–50, 50–100, and > 100 kDa) and found that the stability of NZVI particles was higher in the presence of higher MW fractions. After increasing the HA concentration, the stability of MPs was enhanced, and the differences between different HA fractions in affecting the stability of MPs were reduced, which was attributed to further adsorption of HA.
3.3 Accumulation of MPs in fish tissues
3.3.1 Accumulation of MPs without HA
During the 20-day period, no mortality or abnormalities were observed in any of the treatments, and no MPs were observed in the control treatment (Figure S4). MPs were observed in the tissues (gills, gut, liver, and brain) of red tilapia (Figure 3), indicating that MPs can be ingested by red tilapia and accumulate in fish tissues. After exposure to MPs alone for 10 days, the accumulation concentrations of MPs were 62.7 ± 19.5, 61.7 ± 3.9, 41.3 ± 13.3, and 37.1 ± 12.4 mg MPs g-1 dry weight (dw) in the gills, gut, liver, and brain, respectively (Figure 4). As the gut and gill tissues are in direct contact with the surrounding environment, MPs can first be adsorbed on the branchial filaments through gill water filtration or stacked in the gut lumen through oral ingestion (Ding et al., 2020). Therefore, the accumulation of MPs in the gut and gills of tilapia could be higher than that in the liver and brain during the exposure period. After a 10-day depuration, the concentrations of MPs in the gills, gut, liver, and brain of tilapia were 66.5 ± 4.4, 41.7 ± 0.5, 37.3 ± 11.1, and 66.0 ± 7.2 mg MPs g-1 dw, respectively. The results showed that the degree of MPs remained in the tissues after 10 days of depuration, which was consistent with our previous research (Huang et al., 2021). Notably, the accumulation of MPs in the fish brain significantly increased (p < 0.05) after a 10-day depuration period, possibly due to internal transport in tilapia.
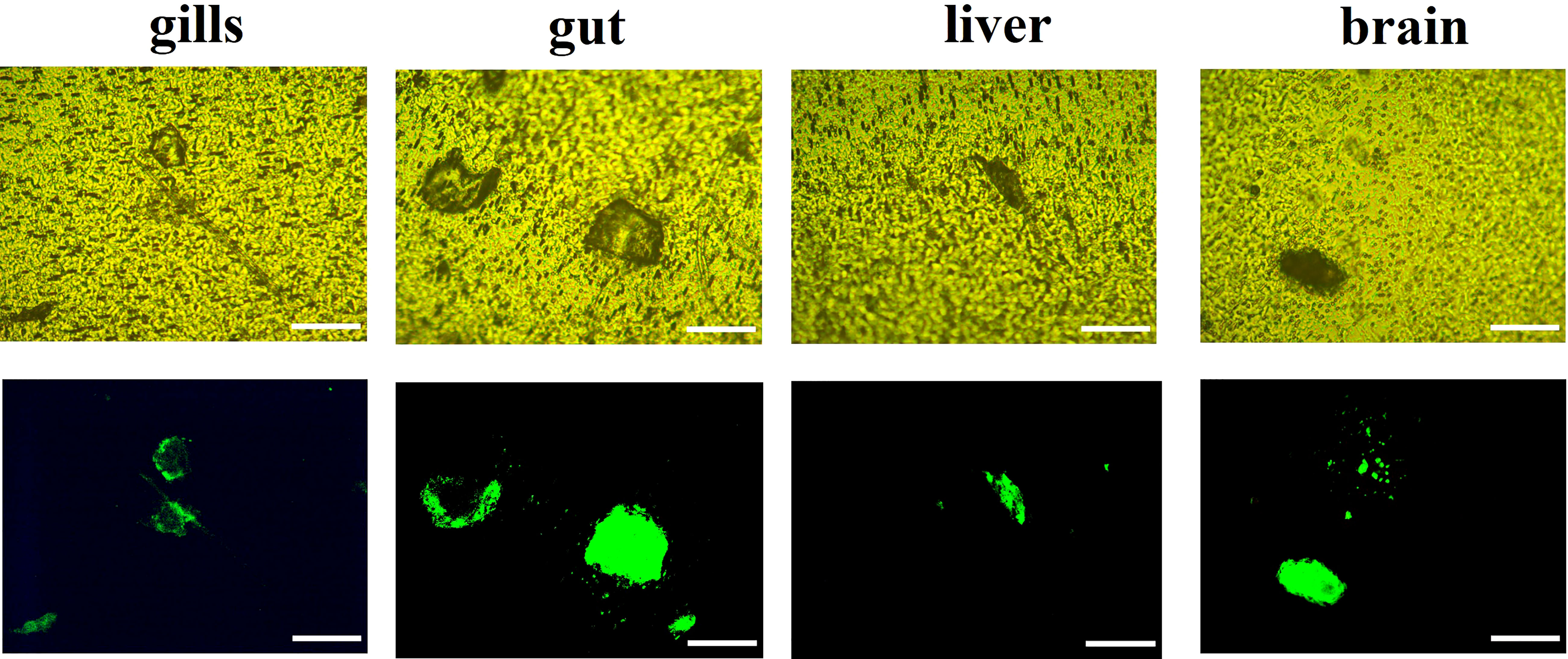
Figure 3 Representative fluorescence iamges of MPs in fish tissues (gills,gut,liver and brain) after 20-day exposure of MPs alone. Fluorescene images of MPs in bright field (up) and in green fluorescene (down). Scale bar= 100µm.
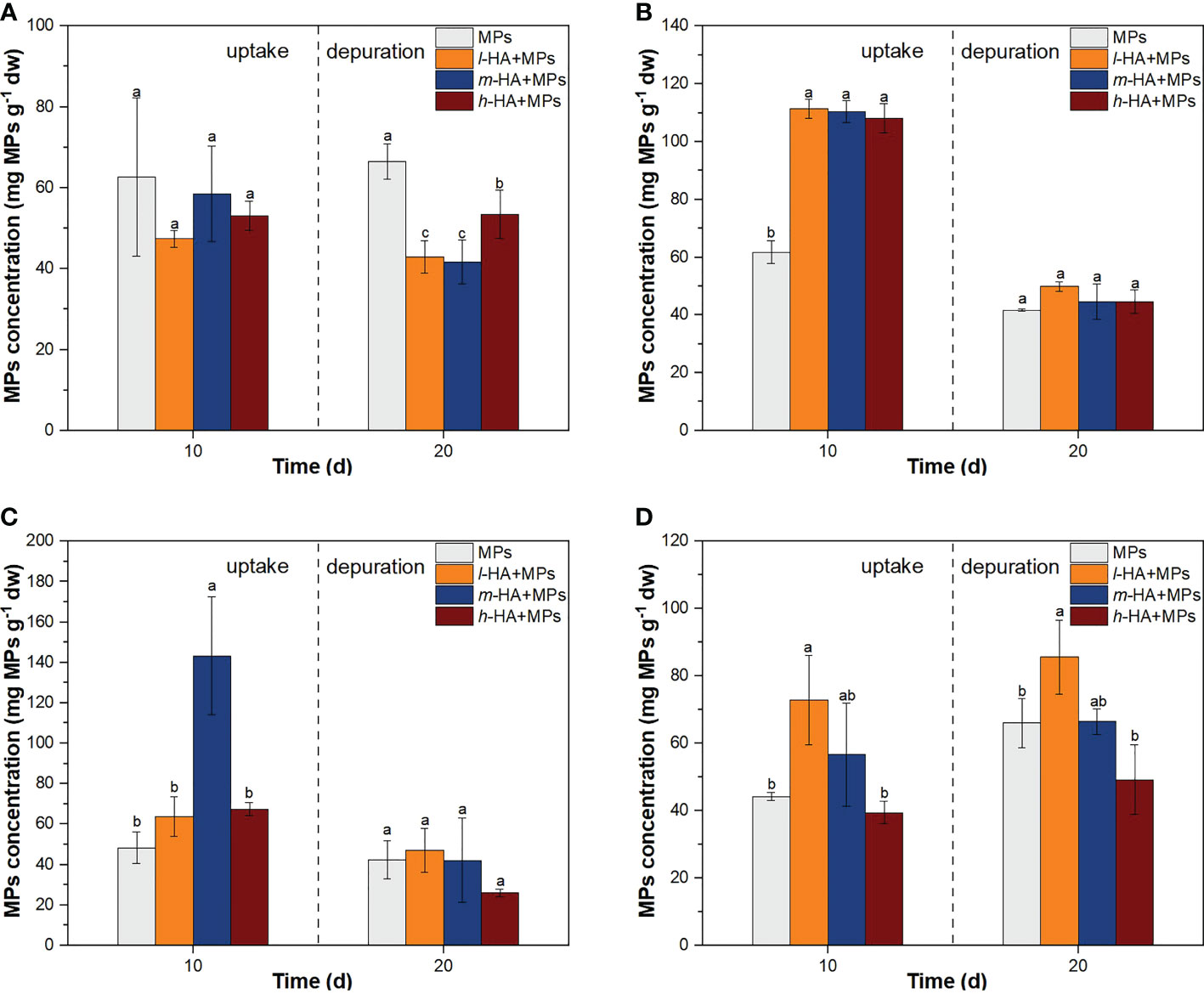
Figure 4 The accumulation of MPs in fish gill (A), gut (B), liver (C), and brain (D) following the treatments of MPs with different HA fractions over 10-day exposure and 10-day depuration. Error bars indicate ± SD (n=3). The different lowercase letter indicate significant differences among different sampling times in each treatment (p<0.05).
3.3.2 Accumulation of MPs with HA in fish external tissues
After a 10-day exposure, the concentrations of MPs in the gills reached 62.7 ± 19.5, 47.4 ± 2.1, 58.5 ± 11.8, and 53.1 ± 3.6 mg g-1 dw after the treatments with MPs, l-HA+MPs, m-HA+MPs, and h-HA+MPs, respectively (Figure 4A). After a 10-day depuration period, the concentrations of MPs in gills of tilapia were 66.5 ± 4.4, 42.9 ± 4.0, 41.7 ± 5.5, and 53.4 ± 6.0 mg MPs g-1 dw in the treatments of MPs, l-HA+MPs, m-HA+MPs, and h-HA+MPs, respectively. The residual concentrations of MPs in the gills treated with different HA fractions were significantly lower than those in the MPs-alone treatment (p < 0.05).
Our results showed that the presence of HA could reduce the accumulation of MPs in fish gills at the end of the depuration period. A recent study reported that Ag accumulation in the gills of the fish Corydoras paleatus decreased when HA was present in the media as compared to that with AgNP exposure alone (Ale et al., 2021), which was consistent with our study. According to the results of the stability experiment, HA adsorbed onto the MP surface could increase the stability of the MP suspensions. It is generally recognized that the increased stability of MP suspensions might increase their residence time in water, resulting in a higher accumulation of MPs in free-swimming organisms (Wang et al., 2016; Eerkes-Medrano et al., 2015; Milne et al., 2017). However, the accumulation of MPs in the gills of tilapia decreased in the presence of HA. This may be related to the pathway of MP transport to the tilapia gills. As mentioned above, MPs accumulate in the gills mainly through adsorption on the branchial filaments of tilapia. The adsorption of HA onto MPs may act as a barrier that hinders direct contact between MPs and gills (Lei et al., 2018; Wang et al., 2016). Additionally, the interaction between HA-adsorbed MPs and cells may be suppressed by increased electrostatic repulsion (Wang et al., 2016). For example, Li et al. (2021) found that the binding of gold nanoparticles (GNPs) to bacteria was suppressed by electrostatic repulsion between negatively charged groups in adsorbed HA and lipopolysaccharides on the bacterial membrane. Collectively, the adsorption of HA may result in less tight binding between MPs and the gill tissue, and consequently cause MPs to be easily removed from the fish gills during the depuration period.
After a 10-day exposure, the concentrations of MPs in the gut of tilapia were 61.7 ± 3.9, 111.3 ± 3.3, 110.3 ± 3.8, and 108.0 ± 5.1 mg g-1 dw after the treatments with MPs, l-HA+MPs, m-HA+MPs, and h-HA+MPs, respectively (Figure 4B). Compared to MPs alone, the presence of three different MW HA fractions (h-HA, m-HA, and l-HA) significantly enhanced the accumulation of MPs in the gut of tilapia (p < 0.05). Qiao et al. (2019) found that NOM enhanced the accumulation of 0.1 and 20 μm PS-MPs in the gut of zebrafish by 1.7 and 2.1 times, respectively, which is consistent with our study. As mentioned above, the adsorption of HA on the MP surface enhances the stability of the MP suspensions. Unlike the uptake route of MPs in fish gills, MPs are stacked in the gut via oral ingestion. Therefore, the accumulation of MPs in the fish gut may increase with increasing MP stability in water. Li et al. (2021) reported that fish can display coughing behavior once the accumulation of MPs in the mouth exceeds a tolerance threshold. The adsorption of HA may reduce the coughing behavior of fish, decrease the clearance of MPs, and thus increase the number of MPs swallowed into the gut.
After a 10-day depuration period, the concentrations of MPs in the gut were 41.7 ± 0.5, 49.9 ± 1.6, 44.7 ± 6.1, and 44.6 ± 4.1 mg g-1 dw after the treatments with MPs, l-HA+MPs, m-HA+MPs, and h-HA+MPs, respectively. Compared with the end of the exposure period, the accumulation of MPs in the fish gut was significantly decreased in all treatments (p < 0.05), suggesting that MPs in the fish guts could be quickly excreted or transferred to other fish tissues through the circulatory system (Huang et al., 2021). The removal rates of the accumulated MPs in the fish gut were 0.3, 0.6, 0.6, and 0.6 after the treatments with MPs, l-HA+MPs, m-HA+MPs, and h-HA+MPs after a 10-day depuration period, respectively. This result suggested that the presence of HA could promote the clearance of MPs in the gut of tilapia. As HA generally contains hydrophobic groups (Li et al., 2021), the adsorption of HA onto the MP surface may result in the higher hydrophobicity of MPs, enhancing the attachment of MPs to the intestine, thereby promoting the transfer of MPs in the gut of tilapia to the internal tissues of fish.
In this study, HA did not show any MW-dependent effect on the accumulation of MPs in the external tissues of fish, although the HA fractions stabilized the MP particles in water in an MW-dependent manner. These results suggest that the stability of the MP suspension is not the only factor influencing the accumulation of MPs in fish tissues. During the uptake and accumulation of MPs, the MW-dependent influence of HA on MP stability may be masked by various factors, such as steric hindrance and electrostatic repulsion in the adsorption onto branchial filaments (Wang et al., 2016) and the complicated environment in the fish intestine (Van Der Zande et al., 2020). The presence and types of extracellular matrices in the gut as well as the innate permeability and tightness of the gut epithelium might also affect the uptake of MPs across the epithelium (Van Der Zande et al., 2020). Moreover, the complex conditions in the gut, such as the constantly changing pH, high ionic strength, and the type and concentration of organic matter, would affect the adsorption and desorption of HA onto MPs, and may further affect the accumulation of MPs in the gut (Zhou et al., 2020; Huang et al., 2021; Wang et al., 2016).
3.3.3 Accumulation of MPs with HA in fish internal tissues
After a 10-day exposure, the concentrations of MPs in the liver of tilapia were 48.3 ± 7.8, 63.7 ± 9.8, 143.2 ± 29.2, and 67.5 ± 3.3 mg g-1 dw after the treatments with MPs, l-HA+MPs, m-HA+MPs, and h-HA+MPs, respectively (Figure 4C). Compared with MPs alone, the accumulation of MPs in the fish liver were enhanced by 0.3, 2.0, and 0.4 times after the treatments with l-HA+MPs, m-HA+MPs, and h-HA+MPs, respectively, although a significant increase was only observed in the treatment with m-HA+MPs (p < 0.05). After a 10-day depuration period, the MPs accumulated in the fish liver significantly decreased under the treatments with l-HA+MPs, m-HA+MPs, and h-HA+MPs (p < 0.05), and there was no significant difference from the MPs-only treatment (p > 0.05).
After a 10-day exposure, the concentrations of MPs in the brain of tilapia were 44.2 ± 1.2, 72.8 ± 13.3, 56.7 ± 15.3, and 39.4 ± 3.4 mg g-1 dw after the treatments with MPs, l-HA+MPs, m-HA+MPs, and h-HA+MPs, respectively (Figure 4D). The accumulation of MPs following treatment with l-HA+MPs was significantly higher than that following treatment with MPs alone (p < 0.05). After a 10-day depuration period, the concentrations of MPs in the brain were 66.0 ± 7.2, 85.6 ± 10.9. 66.5 ± 3.8, and 49.2 ± 10.3 mg g-1 dw after the treatments with MPs, l-HA+MPs, m-HA+MPs, and h-HA+MPs, respectively. A significant increase in the accumulation of MPs was observed only under l-HA+MPs treatment as compared with that in MPs alone (p < 0.05).
The accumulation of MPs in the liver and brain demonstrated that MPs could cross biological barriers and transfer to other internal tissues after being accumulated in the external tissues (gut and gill) of tilapia. MPs could cross the intestinal barrier, enter the bloodstream, translocate to the fish liver, and reach the fish brain after crossing the blood-brain barrier (Vagner et al., 2022; Collard et al., 2017; Lu et al., 2016). Our results showed that the presence of HA fractions generally increased the accumulation of MPs in the liver and brain of tilapia after 10 days of exposure. Similarly, Qiao et al. (2019) found that NOM enhanced the accumulation of 0.1 μm PS-MPs in the liver of zebrafish by a factor of 2. As mentioned above, HA adsorbed onto the MP surface might enhance the attachment of MPs to the intestine and thus increase the transfer of MPs to the internal tissues. This could have also resulted in the increased accumulation of MPs in fish liver and brain. In this regard, previous studies have found that MPs in aquatic environments tend to interact with DOM and form an eco-corona (Fadare et al., 2019; Liu et al., 2019a; Saavedra et al., 2019), which could enhance MP attachment and internalization into cells (Ramsperger et al., 2020).
Compared with the treatment of h-HA+MPs, the accumulation of MPs in the brain with the treatment of l-HA+MPs was significantly enhanced by 0.8 and 0.7 times after a 10-day exposure and a 10-day depuration period (p < 0.05), respectively. Our results suggest that the accumulation of MPs in the brain of tilapia was generally lower in the presence of high-MW HA fractions during the exposure and depuration periods. The adsorption of the high-MW HA fraction onto the MP surface resulted in higher steric hindrance to the cells of fish tissues (Wang et al., 2016), which might make it difficult for MPs to transfer to the brain through the blood brain barrier (Miserocchi et al., 2008; Van Der Zande et al., 2020). However, the accumulation of MPs in the fish liver was significantly higher in the m-HA+MPs treatment after a 10-day exposure (p < 0.05), which was 1.2 and 1.1 times that in the l-HA+MPs and h-HA+MPs treatment, respectively. Unlike the transport route and uptake of MPs in the fish brain, MPs accumulated in the fish liver after crossing the gut barrier. Previous studies have shown that the presence of HA may affect the gut microbiota and permeability, and thus disturb the gut barrier function (Mudroňová et al., 2020; Maguey-Gonzalez et al., 2018; Mirza et al., 2011). Our results suggested that more MPs could cross the gut barrier and translocate to the fish liver in the presence of m-HA. Given the complexity of intestinal environment, it is important to explore the MW-effects and mechanism of HA fractions for MPs crossing the gut barriers and transferring to the fish internal tissues.
3.4 Antioxidative biomarker responses
3.4.1 GST activity
Changes in GST activity in the livers of tilapia subjected to different treatments are shown in Figure 5A. After a 10-day exposure, the GST activity levels in the fish liver were 44.0 ± 9.3, 76.2 ± 4.3, 83.2 ± 2.1, 60.7 ± 3.5, and 76.4 ± 6.6 nmol min-1 mg-1 prot after the treatments with control, MPs, l-HA+MPs, m-HA+MPs, and h-HA+MPs, respectively. Compared with the control, GST activity was significantly higher in the treatments with MPs, l-HA+MPs, m-HA+MPs, and h-HA+MPs (p < 0.05). After a 10-day depuration period, GST activity in all treatments was restored to that of the control levels.
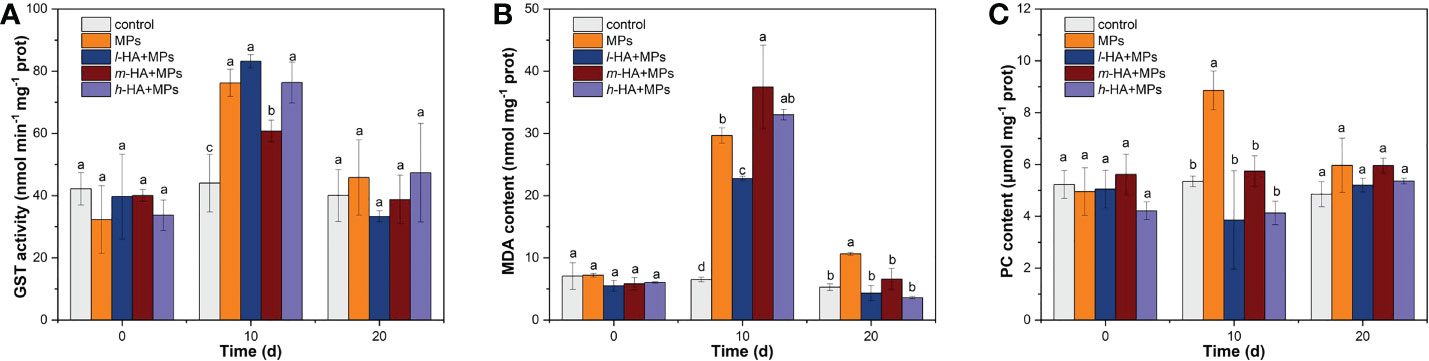
Figure 5 Changes in (A) GST activity, (B) MDA content, and (C) PC content in the liver of red tilapia following the treatments of MPs with different HA fractions at the end of the exposure and depuration periods. Error bars indicate ± SD (n=3). The different lowercase letters indicate significant differences among different treatments on each sampling time (p<0.05).
Compared to the control, GST activity increased by 0.7, 0.9, 0.4, and 0.7 times after the treatments with MPs, l-HA+MPs, m-HA+MPs, and h-HA+MPs, respectively, after a 10-day exposure period. This result implies that MPs, irrespective of the presence or absence of HA, could enhance GST activity in the liver of tilapia. The GST enzyme could act as one of the first biomarkers to activate when aquatic organisms ingested MPs (Rios-Fuster et al., 2021) and play an important role in the cell defense to oxidative damage by catalyzing the binding of glutathione to MPs (Kim and Kang, 2015; Xu et al., 2021). Our results showed that MPs significantly enhanced GST activity in tilapia, possibly mitigating oxidative damage to fish liver cells. Similarly, previous studies have reported that the GST activity of various fish exposed to MPs was significantly increased to act as a detoxification mechanism for alleviating oxidative stress, such as Poecilia reticulata exposed to 100 and 1000 µg L-1 PS-MPs (Huang et al., 2020), C. auratus exposed to 0.5 mg/L polyvinyl chloride (PVC) -MPs (Romano et al., 2020), and Sparus aurata dietary exposed to low-density polyethylene (LDPE) -MPs (Solomando et al., 2020; Rios-Fuster et al., 2021). In this study, compared with the treatment with MPs alone, the addition of m-HA reduced GST activity in fish liver after 10 days of exposure. This may be due to the high accumulation of MPs inhibiting GST activity in the treatment of m-HA+MPs, possibly resulting in more severe oxidative stress in the liver of tilapia.
3.4.2 MDA
After a 10-day exposure, the MDA contents in the fish liver were 6.5 ± 0.4, 29.6 ± 1.2, 22.7 ± 0.3, 37.5 ± 6.7, and 33.0 ± 0.9 nmol mg-1 prot for the treatments of control, MPs, l-HA+MPs, m-HA+MPs, and h-HA+MPs, respectively (Figure 5B). Compared to the control, the MDA contents in MPs, l-HA+MPs, m-HA+MPs, and h-HA+MPs treatments were enhanced by 3.6-, 2.5-, 4.8, and 4.1 times, respectively. Previous studies have confirmed that MPs ingested and accumulated by aquatic organisms cause higher ROS production (Umamaheswari et al., 2021; Yang et al., 2020; Liu et al., 2019b; Hanachi et al., 2022). Increased ROS production in fish tissues may cause oxidative damage (i.e. lipid and protein peroxidation), subsequently resulting in inflammation and cell death (Choi et al., 2018; Ding et al., 2018; Kaloyianni et al., 2021; Kim et al., 2021; Yang et al., 2020). MDA is a terminal product of oxidative damage to cell membranes, lipoproteins, and other lipid-containing structures (Bai et al., 2020), reflects the rate and intensity of lipid peroxidation (LPO) (Huang et al., 2021). In this study, the MDA contents in the treatments of MPs, l-HA+MPs, m-HA+MPs, and h-HA+MPs were significantly higher than those in the control after 10 days of exposure, suggesting that tilapia exposed to MPs experienced severe occurrence of LPO in fish liver. This finding was consistent with our previous study, in which overproduction of MDA was observed in red tilapia exposed to 5 and 70−90 μm PS-MP (Ding et al., 2020). In addition, the MDA content in l-HA+MPs treatment was significantly lower than that in the treatment with MPs after 10 days of exposure, suggesting that the presence of l-HA could alleviate the LPO induced by MPs. Previous studies have reported that HA adsorbed on the particle surface may act as an antioxidant to eliminate a certain amount of ROS generated by particles accumulated in organisms (Lei et al., 2018; Wang et al., 2016). This may result in lower production of MDA in the liver of tilapia exposed to MPs in the presence of HA. However, compared to MPs alone, treatment with m-HA+MPs significantly increased MDA content. As mentioned above, it was convincing that a greater amount of MPs accumulated in the fish liver in the treatment of m-HA+MPs than in the other treatments. Moreover, GST in the treatment with m-HA+MPs was lower than that in the treatment with MPs. Therefore, a more severe LPO in the liver of tilapia may occur when MPs are co-exposed with m-HA.
After a 10-day depuration period, the MDA contents in the fish liver were 5.3 ± 0.5, 10.6 ± 0.0, 4.3 ± 1.2, 6.6 ± 1.7, and 3.6 ± 0.2 nmol mg-1 prot after the treatments with control, MPs, l-HA+MPs, m-HA+MPs, and h-HA+MPs, respectively. The MDA content in the treatments with l-HA+MPs, m-HA+MPs, and h-HA+MPs was restored to that of the control values, but the MDA content in the treatment with MPs was significantly higher than that in the control treatment (p < 0.05). These results revealed that exposure to MPs led to irreversible oxidative damage in the liver of tilapia after a 10-day depuration period, which was avoided by the presence of HA.
3.4.3 Protein peroxidation
After a 10-day exposure, the PC contents in the liver of tilapia were 5.3 ± 0.2, 8.9 ± 0.7, 3.9 ± 1.9, 5.7 ± 0.6, and 4.3 ± 0.5 μmol mg-1 prot after the treatments with control, MPs, l-HA+MPs, m-HA+MPs, and h-HA+MPs, respectively (Figure 5C). PC content in the treatment of MPs was significantly increased compared with other treatments (p < 0.05). After a 10-day depuration period, there were no significant differences between any of the treatments (p > 0.05).
Proteins with a high level of carbonylation are considered dysfunctional, and may cause several adverse effects on cell structure and function (Aryal and Rao, 2018; Kaloyianni et al., 2021). PC content has been proposed as an indicator of protein oxidation, reflecting oxidative damage in various fish species such as D. rerio (Kaloyianni et al., 2021; Bobori et al., 2020), Symphysodon aequifasciatus (Wen et al., 2018), and Rhamdia quelen (Ferreira et al., 2010). In this study, tilapia exposed to MPs without HA had a higher PC content in the liver after 10 days of exposure, which might lead to protein peroxidation. Similarly, Kaloyianni et al. (2021) found that the PC contents in the liver of D. rerio and Perca fluviatilis were significantly increased after a 21-day exposure to PS-MPs compared to the control, with a 1.5 and 10 times increase, respectively. Bobori et al. (2020) found that the PC contents in the liver of D. rerio and Carassius gibelio were significantly increased by 2.3 times after an 8-day exposure to titanium dioxide nanoparticles (TiO2-NPs) compared to those of the control. After a 10-day depuration period, PC content in the treatment of MPs alone was restored to the control values, which might be the result of the proteolysis of high carbonylation proteins and the re-synthesis of normal proteins during the depuration period.
In this study, the presence of the HA fractions significantly reduced the PC content induced by MPs, revealing that HA played a vital role in alleviating protein peroxidation. As mentioned above, HA adsorbed on the MP surface might eliminate a certain amount of ROS induced by MPs (Lei et al., 2018; Wang et al., 2016), and thus relieve protein peroxidation in the fish liver. Moreover, HA coating in MPs might decrease the direct contact between MPs and the cell membrane (Wang et al., 2016), and reduce the damage to membrane proteins.
3.4.4 IBRv2
To visually compare the oxidative stresses among different treatments on red tilapia, IBRv2 was used to integrate three biomarker responses (GST, MDA, and PC) under each exposure condition. Among the biochemical responses, the IBRv2 values of MDA were the highest in response to all exposures, implying that the MDA content was highly sensitive to oxidative stress caused by MPs. The highest IBRv2 value occurred with the treatment of MPs alone (4.5) after 10 days of exposure (Figure 6), suggesting that the exposure of tilapia to MPs without HA could cause more severe oxidative stress in the liver. The IBRv2values were 2.8, 2.4, and 2.6 after the treatments with l-HA+MPs, m-HA+MPs, and h-HA+MPs, respectively. Compared with MPs alone, treatments with l-HA+MPs, m-HA+MPs, and h-HA+MPs resulted in lower IBRv2 values, indicating that the oxidative stress in the liver of tilapia caused by the MPs was indeed alleviated by the presence of different HA fractions. This finding was largely related to the reduced damage of LPO and/or protein peroxidation in the fish liver. Moreover, the IBRv2 value in the treatment of m-HA+MPs was slightly lower than that in the treatments of l-HA+MPs and h-HA+MPs. This result suggested that the presence of m-HA might better alleviate the oxidative stress in the fish liver in some degree compared with other HA fractions, which may be due to the reduced protein damages of fish liver.
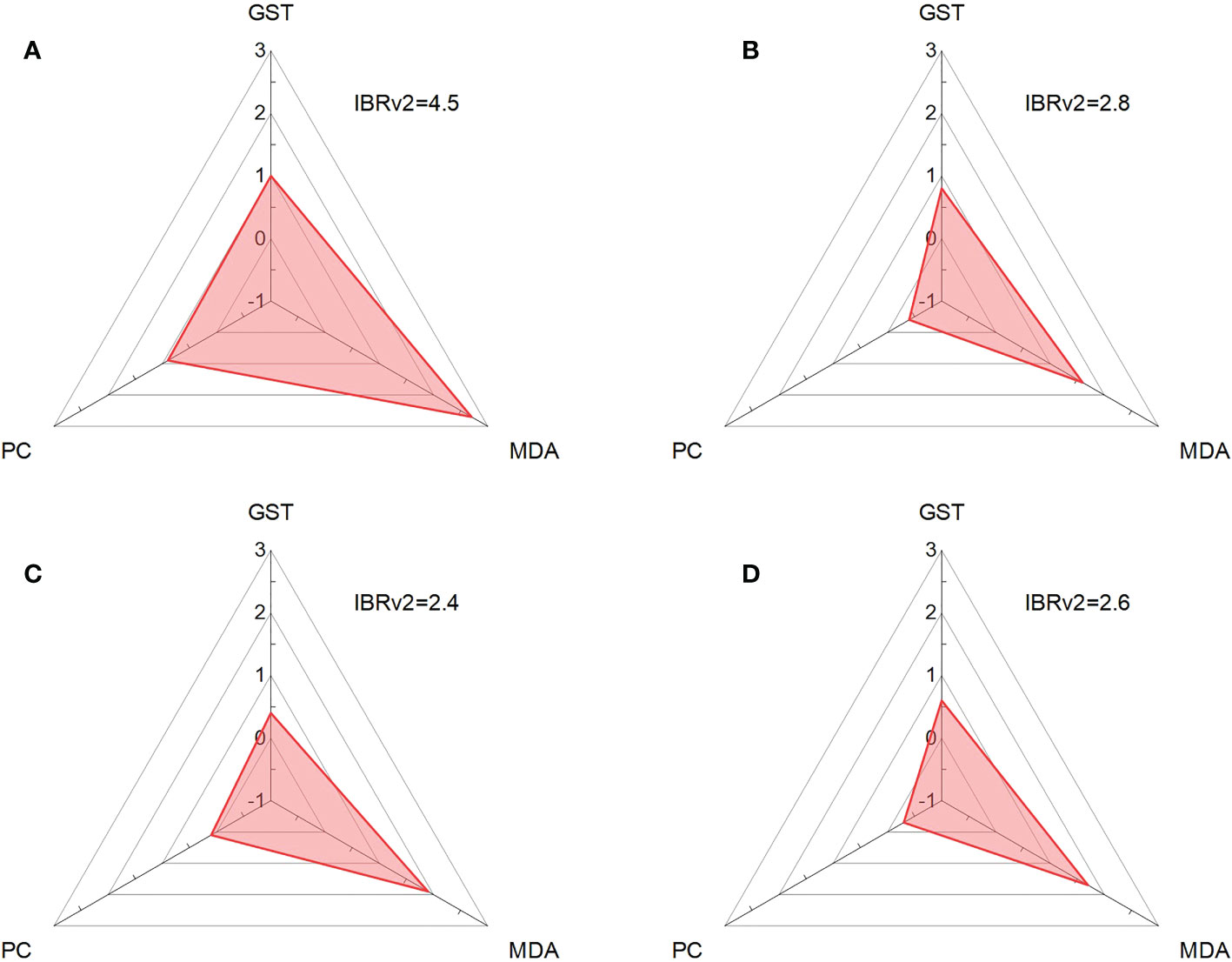
Figure 6 IBRv2 of red tilapia in the treatments of MPs (A), l-HA+MPs (B), m-HA+MPs (C), and h-HA+MPs (D) after a 10-day exposure.
4 Conclusion
To the best of our knowledge, this is the first study on MP toxicity in red tilapia in the presence of different MW fractions of HA. The effects of different HA fractions on MP toxicity were different. Generally, the addition of different HA fractions enhanced the stability and thus the exposure of tilapia to MPs, leading to an increased accumulation of MPs in the gut, liver, and brain. However, the accumulation of MPs in the gills of tilapia was reduced, possibly due to the coating of HA that hinders direct contact between MPs and gills. Moreover, the accumulation of MPs in the gills and gut of tilapia generally showed no difference in the presence of different MW HA fractions, but the accumulation of MPs in the liver and brain of tilapia was generally lower in the presence of the high MW HA fraction than in the low MW HA fraction. Compared to MPs alone, treatment with m-HA+MPs caused inhibition of GST activity and more severe lipid peroxidation. The presence of all HA fractions significantly reduced the PC content induced by MPs. In addition, the IBRv2 results suggested that the oxidative stress (irreversible LPO and reversible protein peroxidation) in the liver tilapia caused by MPs could be alleviated by the presence of all HA fractions, which was largely related to the reduced damage caused by LPO and/or protein peroxidation. Notably, the IBRv2 value in the treatment of m-HA+MPs was lower than that in the treatments of l-HA+MPs and h-HA+MPs, suggesting that the presence of m-HA may better alleviate the oxidative stress in the fish liver compared with other HA fractions possibly due to the reduced protein damages of fish liver. Collectively, our results suggest that the presence of different MW HA fractions could induce complex changes in the MP toxicity on aquatic organisms.
Data availability statement
The original contributions presented in the study are included in the article/Supplementary Material. Further inquiries can be directed to the corresponding author.
Ethics statement
The animal study was reviewed and approved by the Animal Care and Use committee of Jiangnan University.
Author contributions
LZ: Investigation, Writing-Original Draft. JD: Conceptualization, Writing-Review & Editing, Funding acquisition. HX: Formal analysis, Data Curation. WT: Methodology. JX: Validation. HZ: Supervision. WZ: Methodology, Resources. All authors contributed to the article and approved the submitted version.
Funding
This study was supported by the Postdoctoral Science Foundation of China (No. 2019M651704) and the Natural Science Foundation of Jiangsu Province, China (No. BK20221542).
Conflict of interest
The authors declare that the research was conducted in the absence of any commercial or financial relationships that could be construed as a potential conflict of interest.
Publisher’s note
All claims expressed in this article are solely those of the authors and do not necessarily represent those of their affiliated organizations, or those of the publisher, the editors and the reviewers. Any product that may be evaluated in this article, or claim that may be made by its manufacturer, is not guaranteed or endorsed by the publisher.
Supplementary material
The Supplementary Material for this article can be found online at: https://www.frontiersin.org/articles/10.3389/fmars.2022.1060582/full#supplementary-material
References
Ale A., Galdopórpora J. M., Desimone M. F., de la Torre F. R., Cazenave J. (2021). Nanosilver and silver nitrate toxicity in ex vivo-exposed gills of fish and mitigation by humic acids Bull. Environ. Contam. Toxicol. 107 (3), 421–426. doi: 10.1007/s00128-021-03257-w
Aryal B., Rao V. A. (2018). Specific protein carbonylation in human breast cancer tissue compared to adjacent healthy epithelial tissue PloS One 13 (3), e0194164. doi: 10.1371/journal.pone.0194164
Bai H., Luo M., Wei S., Jiang Z., He M. (2020). The vital function of humic acid with different molecular weight in controlling cd and Pb bioavailability and toxicity to earthworm (Eisenia fetida) in soil Environ. pollut. 261, 114222. doi: 10.1016/j.envpol.2020.114222
Bai H., Wei S., Jiang Z., He M., Ye B., Liu G. (2019). Pb (II) bioavailability to algae (Chlorella pyrenoidosa) in relation to its complexation with humic acids of different molecular weight Ecotoxicol. Environ. Saf. 167, 1–9. doi: 10.1016/j.ecoenv.2018.09.114
Blewett T. A., Dow E. M., Wood C. M., McGeer J. C., Smith D. S. (2018). The role of dissolved organic carbon concentration and composition on nickel toxicity to early life-stages of the blue mussel Mytilus edulis and purple sea urchin Strongylocentrotus purpuratus. Ecotoxicol Environ. Saf. 160, 162–170. doi: 10.1016/j.ecoenv.2018.05.029
Bobori D., Dimitriadi A., Karasiali S., Tsoumaki-Tsouroufli P., Mastora M., Kastrinaki G., et al. (2020). Common mechanisms activated in the tissues of aquatic and terrestrial animal models after TiO2 nanoparticles exposure Environ. Int. 138, 105611. doi: 10.1016/j.envint.2020.105611
Bordós G., Urbányi B., Micsinai A., Kriszt B., Palotai Z., Szabó I., et al. (2019). Identification of microplastics in fish ponds and natural freshwater environments of the Carpathian basin, europe Chemosphere. 216, 110–116. doi: 10.1016/j.chemosphere.2018.10.110
Chen W., Westerhoff P., Leenheer J. A., Booksh K. (2003). Fluorescence excitation-emission matrix regional integration to quantify spectra for dissolved organic matter Environ. Sci. Technol. 37 (24), 5701–5710. doi: 10.1021/es034354c
Choi J. S., Jung Y. J., Hong N. H., Hong S. H., Park J. W. (2018). Toxicological effects of irregularly shaped and spherical microplastics in a marine teleost, the sheepshead minnow (Cyprinodon variegatus). Mar pollut. Bull. 129 (1), 231–240. doi: 10.1016/j.marpolbul.2018.02.039
Cole M., Galloway T. S. (2015). Ingestion of nanoplastics and microplastics by pacific oyster larvae Environ. Sci. Technol. 49 (24), 14625–14632. doi: 10.1021/acs.est.5b04099
Collard F., Gilbert B., Compère P., Eppe G., Das K., Jauniaux T., et al. (2017). Microplastics in livers of European anchovies (Engraulis encrasicolus, l.) Environ. pollut. 229, 1000–1005. doi: 10.1016/j.envpol.2017.07.089
De Marco G., Conti G. O., Giannetto A., Cappello T., Galati M., Iaria C., et al. (2022). Embryotoxicity of polystyrene microplastics in zebrafish danio rerio Environ. Res. 208, 112552. doi: 10.1016/j.envres.2021.112552
de Sá L. C., Oliveira M., Ribeiro F., Rocha T. L., Futter M. N. (2018). Studies of the effects of microplastics on aquatic organisms: what do we know and where should we focus our efforts in the future? Sci. Total Environ. 645, 1029–1039. doi: 10.1016/j.scitotenv.2018.07.207
Ding J., Huang Y., Liu S., Zhang S., Zou H., Wang Z., et al. (2020). Toxicological effects of nano-and micro-polystyrene plastics on red tilapia: are larger plastic particles more harmless? J. Hazard. Mater. 396, 122693. doi: 10.1016/j.jhazmat.2020.122693
Ding J., Zhang S., Razanajatovo R. M., Zou H., Zhu W. (2018). Accumulation, tissue distribution, and biochemical effects of polystyrene microplastics in the freshwater fish red tilapia (Oreochromis niloticus) Environ. pollut. 238, 1–9. doi: 10.1016/j.envpol.2018.03.001
Eerkes-Medrano D., Thompson R. C., Aldridge D. C. (2015). Microplastics in freshwater systems: A review of the emerging threats, identification of knowledge gaps and prioritisation of research needs Water. Res. 75, 63–82. doi: 10.1016/j.watres.2015.02.012
Eltemsah Y. S., Bøhn T. (2019). Acute and chronic effects of polystyrene microplastics on juvenile and adult Daphnia magna. Environ Pollut. 254, 112919. doi: 10.1016/j.envpol.2019.07.087
Erni-Cassola G., Gibson M. I., Thompson R. C., Christie-Oleza J. A. (2017). Lost, but found with Nile red: a novel method for detecting and quantifying small microplastics (1 mm to 20 μm) in environmental samples Environ. Sci. Technol. 51 (23), 13641–13648. doi: 10.1021/acs.est.7b04512
Fadare O. O., Wan B., Guo L. H., Xin Y., Qin W., Yang Y. (2019). Humic acid alleviates the toxicity of polystyrene nanoplastic particles to Daphnia magna. Environ Sci. Nano. 6 (5), 1466–1477. doi: 10.1039/C8EN01457D
Ferreira D., Motta A., Kreutz L. C., Toni C., Loro V. L., Barcellos L. J. G. (2010). Assessment of oxidative stress in Rhamdia quelen exposed to agrichemicals Chemosphere. 79 (9), 914–921. doi: 10.1016/j.chemosphere.2010.03.024
Francioso O., Sanchez-Cortes S., Tugnoli V., Ciavatta C., Sitti L., Gessa C. (1996). Infrared, raman, and nuclear magnetic resonance (1 h, 13 c, and 31 p) spectroscopy in the study of fractions of peat humic acids Appl. Spectrosc. 50 (9), 1165–1174. doi: 10.1366/0003702963905169
Goldstein M. C., Rosenberg M., Cheng L. (2012). Increased oceanic microplastic debris enhances oviposition in an endemic pelagic insect Biol. Lett. 8, 817–820. doi: 10.1098/rsbl.2012.0298
Hanachi P., Khoshnamvand M., Walker T. R., Hamidian A. H. (2022). Nano-sized polystyrene plastics toxicity to microalgae Chlorella vulgaris: Toxicity mitigation using humic acid Aquat. Toxicol. 245, 106123. doi: 10.1016/j.aquatox.2022.106123
Huang Y., Ding J., Zhang G., Liu S., Zou H., Wang Z., et al. (2021). Interactive effects of microplastics and selected pharmaceuticals on red tilapia: Role of microplastic aging Sci. Total Environ. 752, 142256. doi: 10.1016/j.scitotenv.2020.142256
Huang J. N., Wen B., Meng L. J., Li X. X., Wang M. H., Gao J. Z., et al. (2020). Integrated response of growth, antioxidant defense and isotopic composition to microplastics in juvenile guppy (Poecilia reticulata) J. Hazard. Mater. 399, 123044. doi: 10.1016/j.jhazmat.2020.123044
Jiang Y., Raliya R., Peng L., Biswas P., Fortner J. D. (2017). Graphene oxides in water: assessing stability as a function of material and natural organic matter properties Environ. Sci. Nano. 4, 1484–1493. doi: 10.1039/C7EN00220C
Kaloyianni M., Bobori D. C., Xanthopoulou D., Malioufa G., Sampsonidis I., Kalogiannis S., et al. (2021). Toxicity and functional tissue responses of two freshwater fish after exposure to polystyrene microplastics Toxics. 9 (11), 289. doi: 10.3390/toxics9110289
Khosrovyan A., Gabrielyan B., Kahru A. (2020). Ingestion and effects of virgin polyamide microplastics on Chironomus riparius adult larvae and adult zebrafish Danio rerio Chemosphere 259, 127456. doi: 10.1016/j.chemosphere.2020.127456
Kim J. H., Kang J. C. (2015). Oxidative stress, neurotoxicity, and non-specific immune responses in juvenile red sea bream, Pagrus major, exposed to different waterborne selenium concentrations Chemosphere. 135, 46–52. doi: 10.1016/j.chemosphere.2015.03.062
Kim J. H., Yu Y. B., Choi J. H. (2021). Toxic effects on bioaccumulation, hematological parameters, oxidative stress, immune responses and neurotoxicity in fish exposed to microplastics: A review J. Hazard. Mater. 413, 125423. doi: 10.1016/j.jhazmat.2021.125423
Kokalj A. J., Horvat P., Skalar T., Kržan A. (2018). . plastic bag and facial cleanser derived microplastic do not affect feeding behaviour and energy reserves of terrestrial isopods Sci. Total Environ. 615, 761–766. doi: 10.1016/j.scitotenv.2017.10.020
Lei C., Sun Y., Daniel C. W. T., Lin D. (2018). Environmental transformations and ecological effects of iron-based nanoparticles Environ. pollut. 232, 10–30. doi: 10.1016/j.envpol.2017.09.052
Li Z., Shakiba S., Deng N., Chen J., Louie S. M., Hu Y. (2020). Natural organic matter (NOM) imparts molecular-weight-dependent steric stabilization or electrostatic destabilization to ferrihydrite nanoparticles Environ. Sci. Technol. 54 (11), 6761–6770. doi: 10.1021/acs.est.0c01189
Liu G., Jiang R., You J., Muir D. C., Zeng E. Y. (2019a). Microplastic impacts on microalgae growth: effects of size and humic acid Environ. Sci. Technol. 54 (3), 1782–1789. doi: 10.1021/acs.est.9b06187
Liu Y., Wang Z., Wang S., Fang H., Ye N., Wang D. (2019b). Ecotoxicological effects on Scenedesmus obliquus and Danio rerio co-exposed to polystyrene nano-plastic particles and natural acidic organic polymer Environ. Toxicol. Pharmacol. 67, 21–28. doi: 10.1016/j.etap.2019.01.007
Li S., Wang S., Yan B., Yue T. (2021). Surface properties of nanoparticles dictate their toxicity by regulating adsorption of humic acid molecules ACS Sustain. Chem. Eng. 9 (41), 13705–13716. doi: 10.1021/acssuschemeng.1c02795
Louie S. M., Tilton R. D., Lowry G. V. (2013). Effects of molecular weight distribution and chemical properties of natural organic matter on gold nanoparticle aggregation Environ. Sci. Technol. 47 (9), 4245–4254. doi: 10.1021/es400137x
Lu Y., Zhang Y., Deng Y., Jiang W., Zhao Y., Geng J., et al. (2016). Uptake and accumulation of polystyrene microplastics in zebrafish (Danio rerio) and toxic effects in liver Environ. Sci. Technol. 50 (7), 4054–4060. doi: 10.1021/acs.est.6b00183
Maguey-Gonzalez J. A., Michel M. A., Baxter M. F., Tellez J., Moore P. A. Jr., Solis-Cruz B., et al (2018). Effect of humic acids on intestinal viscosity, leaky gut and ammonia excretion in a 24 hr feed restriction model to induce intestinal permeability in broiler chickens Anim. Sci. J. 89 (7), 1002–1010. doi: 10.1111/asj.13011
Milne C. J., Lapworth D. J., Gooddy D. C., Elgy C. N., Valsami-Jones É. (2017). Role of humic acid in the stability of Ag nanoparticles in suboxic conditions Environ. Sci. Technol. 51 (11), 6063–6070. doi: 10.1021/acs.est.6b06054
Mirza M. A., Agarwal S. P., Rahman M. A., Rauf A., Ahmad N., Alam A., et al (2011). . role of humic acid on oral drug delivery of an antiepileptic drug Drug Dev. Ind. Pharm. 37 (3), 310–319. doi: 10.3109/03639045.2010.512011
Miserocchi G., Sancini G., Mantegazza F., Chiappino G. (2008). Translocation pathways for inhaled asbestos fibers Environ. Health 7 (1), 1–8. doi: 10.1186/1476-069X-7-4
Mudroňová D., Karaffová V., Pešulová T., Koščová J., Maruščáková I. C., Bartkovský M., et al. (2020). The effect of humic substances on gut microbiota and immune response of broilers Food Agric. Immunol. 31 (1), 137–149. doi: 10.1080/09540105.2019.1707780
Piccolo A.. (2001). The supramolecular structure of humic substances. Soil Sci. 166 (11), 810–832. doi: 10.1097/00010694-200111000-00007
Qiao R., Lu K., Deng Y., Ren H., Zhang Y. (2019). Combined effects of polystyrene microplastics and natural organic matter on the accumulation and toxicity of copper in zebrafish Sci. Total Environ. 682, 128–137. doi: 10.1016/j.scitotenv.2019.05.163
Ramsperger A. F. R. M., Narayana V. K. B., Gross W., Mohanraj J., Thelakkat M., Greiner A., et al. (2020). Environmental exposure enhances the internalization of microplastic particles into cells Sci. Adv. 6 (50), eabd1211. doi: 10.1126/sciadv.abd1211
Ren J., Fan W., Wang X., Ma Q., Li X., Xu Z., et al. (2017). Influences of size-fractionated humic acids on arsenite and arsenate complexation and toxicity to Daphnia magna Water. Res. 108, 68–77. doi: 10.1016/j.watres.2016.10.052
Ribeiro J. S., Ok S. S., Garrigues S., de la Guardia M. (2001). FTIR tentative characterization of humic acids extracted from organic materials Spectrosc. Lett. 34 (2), 179–190. doi: 10.1081/SL-100002007
Rios-Fuster B., Arechavala-Lopez P., García-Marcos K., Alomar C., Compa M., Álvarez E., et al. (2021). Experimental evidence of physiological and behavioral effects of microplastic ingestion in Sparus aurata. Aquat Toxicol. 231, 105737. doi: 10.1016/j.aquatox.2020.105737
Romano N., Renukdas N., Fischer H., Shrivastava J., Baruah K., Egnew N., et al. (2020). Differential modulation of oxidative stress, antioxidant defense, histomorphology, ion-regulation and growth marker gene expression in goldfish (Carassius auratus) following exposure to different dose of virgin microplastics Comp. Biochem. Physiol. Part C Toxicol. Pharmacol. 238, 108862. doi: 10.1016/j.cbpc.2020.108862
Saavedra J., Stoll S., Slaveykova V. I. (2019). Influence of nanoplastic surface charge on eco-corona formation, aggregation and toxicity to freshwater zooplankton Environ. pollut. 252, 715–722. doi: 10.1016/j.envpol.2019.05.135
Sanchez W., Burgeot T., Porcher J. M. (2013). A novel “integrated biomarker response” calculation based on reference deviation concept Environ. Sci. pollut. Res. 20, 2721–2725. doi: 10.1007/s11356-012-1359-1
Schür C., Weil C., Baum M., Wallraff J., Schreier M., Oehlmann J., et al (2021). . incubation in wastewater reduces the multigenerational effects of microplastics in Daphnia magna Environ. Sci. Technol. 55 (4), 2491–2499. doi: 10.1021/acs.est.0c07911
Sharma V. K., Ma X., Guo B., Zhang K. (2021). Environmental factors-mediated behavior of microplastics and nanoplastics in water: A review Chemosphere. 271, 129597. doi: 10.1016/j.chemosphere.2021.129597
Solomando A., Capó X., Alomar C., Álvarez E., Compa M., Valencia J. M., et al. (2020). Long-term exposure to microplastics induces oxidative stress and a pro-inflammatory response in the gut of Sparus aurata Linnaeus 1758 Environ. pollut. 266, 115295. doi: 10.1016/j.envpol.2020.115295
Sul J. A. I., Costa M. F., Fillmann G. (2014). . microplastics in the pelagic environment around oceanic islands of the Western tropical Atlantic ocean Water Air Soil pollut. 225, (2004). doi: 10.1007/s11270-014-2004-z
Tallec K., Blard O., González-Fernández C., Brotons G., Berchel M., Soudant P., et al. (2019). Surface functionalization determines behavior of nanoplastic solutions in model aquatic environments Chemosphere. 225, 639–646. doi: 10.1016/j.chemosphere.2019.03.077
Thompson R. C., Olsen Y., Mitchell R. P., Davis A., Rowland S. J., John A. W., et al. (2004). Lost at sea: where is all the plastic? Science. 304 (5672), 838–838. doi: 10.1126/science.1094559
Umamaheswari S., Priyadarshinee S., Bhattacharjee M., Kadirvelu K., Ramesh M. (2021). Exposure to polystyrene microplastics induced gene modulated biological responses in zebrafish (Danio rerio) Chemosphere. 281, 128592. doi: 10.1016/j.chemosphere.2020.128592
Vagner M., Boudry G., Courcot L., Vincent D., Dehaut A., Duflos G., et al. (2022). Experimental evidence that polystyrene nanoplastics cross the intestinal barrier of European seabass Environ. Int. 166, 107340. doi: 10.1016/j.envint.2022.107340
Van Der Zande M., Kokalj A. J., Spurgeon D. J., Loureiro S., Silva P. V., Khodaparast Z., et al. (2020). The gut barrier and the fate of engineered nanomaterials: a view from comparative physiology Environ. Sci. Nano. 7 (7), 1874–1898. doi: 10.1039/D0EN00174K
Wang X., Bolan N., Tsang D. C., Sarkar B., Bradney L., Li Y. (2021b). A review of microplastics aggregation in aquatic environment: Influence factors, analytical methods, and environmental implications J. Hazard. Mater. 402, 123496. doi: 10.1016/j.jhazmat.2020.123496
Wang Z., Zhang Y., Kang S., Yang L., Shi H., Tripathee L., et al. (2021a). Research progresses of microplastic pollution in freshwater systems Sci. Total Environ. 795, 148888. doi: 10.1016/j.scitotenv.2021.148888
Wang Z., Zhang L., Zhao J., Xing B. (2016). Environmental processes and toxicity of metallic nanoparticles in aquatic systems as affected by natural organic matter Environ. Sci. Nano. 3, 240–255. doi: 10.1039/c5en00230c
Wang X., Zheng H., Zhao J., Luo X., Wang Z., Xing B. (2020). Photodegradation elevated the toxicity of polystyrene microplastics to grouper (Epinephelus moara) through disrupting hepatic lipid homeostasis Environ. Sci. Technol. 54 (10), 6202–6212. doi: 10.1021/acs.est.9b07016
Wen B., Jin S. R., Chen Z. Z., Gao J. Z., Liu Y. N., Liu J. H., et al. (2018). Single and combined effects of microplastics and cadmium on the cadmium accumulation, antioxidant defence and innate immunity of the discus fish (Symphysodon aequifasciatus) Environ. pollut. 243, 462–471. doi: 10.1016/j.envpol.2018.09.029
Wu X., Liu P., Gong Z., Wang H., Huang H., Shi Y., et al. (2021). Humic acid and fulvic acid hinder long-term weathering of microplastics in lake water Environ. Sci. Technol. 55(23), 15810–15820. doi: 10.1021/acs.est.1c04501
Wu Y., Dong H., Tang L., Li L., Wang Y., Ning Q., et al. (2020). Influence of humic acid and its different molecular weight fractions on sedimentation of nanoscale zero-valent iron Environ. Sci. pollut. Res. 27 (3), 2786–2796. doi: 10.1007/s11356-019-07140-4
Wu J., Jiang R., Lin W., Ouyang G. (2019). Effect of salinity and humic acid on the aggregation and toxicity of polystyrene nanoplastics with different functional groups and charges Environ. pollut. 245, 836–843. doi: 10.1016/j.envpol.2018.11.055
Xu Z., Sui Q., Li A., Sun M., Zhang L., Lyu S., et al. (2020). How to detect small microplastics (20–100 μm) in freshwater, municipal wastewaters and landfill leachates? a trial from sampling to identification Sci. Total Environ. 733, 139218. doi: 10.1016/j.scitotenv.2020.139218
Xu K., Zhang Y., Huang Y., Wang J. (2021). Toxicological effects of microplastics and phenanthrene to zebrafish (Danio rerio) Sci. Total Environ. 757, 143730. doi: 10.1016/j.scitotenv.2020.143730
Yang H., Xiong H., Mi K., Xue W., Wei W., Zhang Y. (2020). Toxicity comparison of nano-sized and micron-sized microplastics to goldfish Carassius auratus Larvae J. Hazard. Mater. 388, 122058. doi: 10.1016/j.jhazmat.2020.122058
Yu S., Shen M., Li S., Fu Y., Zhang D., Liu H., et al. (2019). Aggregation kinetics of different surface-modified polystyrene nanoparticles in monovalent and divalent electrolytes Environ. pollut. 255, 113302. doi: 10.1016/j.envpol.2019.113302
Zhang S., Ding J., Razanajatovo R. M., Jiang H., Zou H., Zhu W. (2019). Interactive effects of polystyrene microplastics and roxithromycin on bioaccumulation and biochemical status in the freshwater fish red tilapia (Oreochromis niloticus) Sci. Total Environ. 648, 1431–1439. doi: 10.1016/j.scitotenv.2018.08.266
Zhang S., Su J., Ali A., Zheng Z., Sun Y. (2021). Enhanced denitrification performance of strain YSF15 by different molecular weight of humic acid: Mechanism based on the biological products and activity Bioresour. Technol. 325, 124709. doi: 10.1016/j.biortech.2021.124709
Zhou Y., Yang Y., Liu G., He G., Liu W. (2020). Adsorption mechanism of cadmium on microplastics and their desorption behavior in sediment and gut environments: the roles of water pH, lead ions, natural organic matter and phenanthrene Water. Res. 184, 116209. doi: 10.1016/j.watres.2020.116209
Zhu Q., Li N., Wang C., Zhang Q., Sun H. (2020). Effect of interactions between various humic acid fractions and iron nanoparticles on the toxicity to white rot fungus Chemosphere. 247, 125895. doi: 10.1016/j.chemosphere.2020.125895
Keywords: microplastics, stability, MW-dependent toxicity, accumulation, oxidative stress
Citation: Zheng L, Ding J, Xu H, Tian W, Xu J, Zou H and Zhu W (2022) Influences of molecular weight fractionated humic acids on polyamide 66 microplastic stability and toxicity in red tilapia (Oreochromis niloticus). Front. Mar. Sci. 9:1060582. doi: 10.3389/fmars.2022.1060582
Received: 03 October 2022; Accepted: 31 October 2022;
Published: 14 November 2022.
Edited by:
Miguel Oliveira, University of Aveiro, PortugalReviewed by:
Jie Ma, Shenzhen University, ChinaXinguo Zhao, Yellow Sea Fisheries Research Institute (CAFS), China
Copyright © 2022 Zheng, Ding, Xu, Tian, Xu, Zou and Zhu. This is an open-access article distributed under the terms of the Creative Commons Attribution License (CC BY). The use, distribution or reproduction in other forums is permitted, provided the original author(s) and the copyright owner(s) are credited and that the original publication in this journal is cited, in accordance with accepted academic practice. No use, distribution or reproduction is permitted which does not comply with these terms.
*Correspondence: Jiannan Ding, ZGpuQGppYW5nbmFuLmVkdS5jbg==