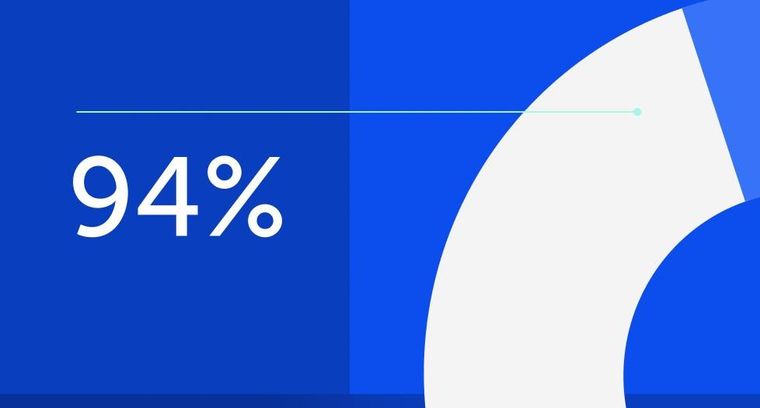
94% of researchers rate our articles as excellent or good
Learn more about the work of our research integrity team to safeguard the quality of each article we publish.
Find out more
ORIGINAL RESEARCH article
Front. Mar. Sci., 24 January 2023
Sec. Deep-Sea Environments and Ecology
Volume 9 - 2022 | https://doi.org/10.3389/fmars.2022.1059616
This article is part of the Research Topic16th Deep-Sea Biology SymposiumView all 27 articles
Benthic foraminifera cannot be sampled adequately using a single device. Smaller taxa are best collected using multicorers, the larger with box corers, but towed devices (dredges, trawls and epibenthic sledges) also retain many larger species. Here, we describe macrofaunal (>300 µm) foraminiferal assemblages obtained using an epibenthic sledge (EBS) in the Clarion-Clipperton Zone (eastern equatorial Pacific), a region hosting seafloor deposits of polymetallic nodules. Twelve EBS samples were collected in four areas licenced for exploration by the International Seabed Authority (ISA) to German, IOM, Belgium and French contractors, and to APEI-3, one of the protected Areas of Special Scientific Interest designated by the ISA. We recognised 280 morphospecies among 1954 specimens, with between 74 (IOM) and 121 (Belgium) in particular areas. Most (92.7%) were single-chambered monothalamids, of which 75 species (26.8%) belonged to the Komokioidea (‘komoki’), 47 (16.8%) to branched and unbranched tubes, 33 (11.8%) to chain-like and 32 (11.4%) to various ‘komoki-like’ forms. Fragments of megafaunal xenophyophores represented 21 species (7.50%), including Spiculammina delicata, previously reported only from the Russian area. Rarefaction curves and sample coverage completeness curves suggest that only a fraction of the macrofaunal foraminiferal diversity had been sampled. The occurrence of 71.8% of species in 1-2 of the 12 samples and 84.9% in 1-3 of the samples was a likely result of substantial undersampling. Dissimilarity in species composition between areas was very high: 64.2% (German vs IOM area) to 86.9% (German area vs APEI-3). Similarity within a single area was quite low: 29.1% (German) to 45.1% (IOM). In multidimensional scaling (MDS) plots, the APEI-3 area was clearly distinct in terms of faunal composition from all other areas, the French area somewhat separated from the German, IOM and Belgium areas, with the German and IOM samples being the most similar. These patterns may reflect the geographical separation of the French and APEI-3 areas and their location in deeper, more oligotrophic waters. Our study demonstrates that EBS samples from the eastern CCZ are a rich source of novel foraminiferal taxa, particularly light, easily resuspended komoki, providing a valuable perspective on foraminiferal biodiversity.
Until the middle of the last century, much of our knowledge of deep-sea benthic invertebrates was based on relatively coarse-meshed dredges that are towed across the seafloor. These devices have continued to be used for sampling larger fauna, including some foraminifera, in the deep sea, but they are not quantitative and do not collect smaller organisms (macro- and meiofauna). The advent of a more quantitative approach to the study of deep-sea faunas during the 1960s saw the deployment of new coring devices that obtained largely undisturbed sediment containing smaller organisms from a defined area of the seafloor. For the macrofauna, the introduction of the USNEL box corer by Hessler and Jumars (1974) ushered in a new age of quantitative research and provided the basis for studies that revealed the huge abundance and diversity of novel foraminifera larger than 300 µm at abyssal depths (Bernstein et al., 1978). For the meiofauna, different versions of hydraulically-dampened multicorers, first developed by Barnett et al. (1984), have become the instruments of choice in the deep sea.
The second half of the 20th century saw the development of various new devices that obtain much larger numbers of benthic organisms than core samples, as well as collecting the smaller organisms that pass through the meshes of traditional dredges (reviewed by Gage and Tyler, 1991; Kaiser and Brenke, 2016). These include towed epibenthic sledges and sleds (EBSs) fitted with one or more nets and runners to smooth their passage across the seafloor. The work of Hessler and Sanders (1967), who were the first to use epibenthic sledges, and earlier of Sanders et al. (1965) using an anchor dredge, together with studies by Russian and Danish biologists in the 1950s and 1960s based on grab samples (cited in Sanders et al., 1965), mark the beginnings of modern quantitative deep-sea biology and revealed high levels of diversity among smaller-sized organisms on the ocean floor. Since then, many different types of EBSs have been developed, some able to operate on rough seafloors, others able to sample organisms living just above the seabed, as well as on and below the sediment surface (Kaiser and Brenke, 2016). One of the most widely used was designed by Brenke (2005). It includes a lower epibenthic net and an upper suprabenthic net, both with a mesh size of 500 µm and fitted with a cod-end bucket equipped with a 300-µm-mesh filter. Although at best only semi-quantitative (Rice et al., 1979; Rice et al., 1982), EBSs have provided a rich source of material for taxonomic and biogeographic studies of deep-sea macrofaunal metazoans (e.g., Brandt et al., 2007; Brandt et al., 2014).
The major contribution that foraminifera make to deep-sea sediment communities across all size categories is well established (e.g., Thiel, 1975; Tendal and Hessler, 1977; Bernstein et al., 1978; Thiel, 1983 Gooday et al., 2020a). All the sampling gears mentioned above will collect benthic foraminifera, albeit with different biases and degrees of efficiency. The earliest observations on benthic species from depths below a few hundreds of meters were based on small sediment samples recovered with sounding devices, but bottom samples collected using a dredge also yielded rich collections of foraminifera, including larger-sized species, during the pioneering campaigns of H.M.S. Lightning (1868) and Porcupine (1869) in the NE Atlantic and Mediterranean, those of the U.S. Coast Survey in the NW Atlantic (briefly reviewed by Douglas and Woodruff, 1981; Gooday, 1990; Gooday et al., 2020a), and particularly during the global expedition of H.M.S. Challenger (1873-1876) (Brady, 1884), which laid the foundations for the science of oceanography. Dredges continued to provide material for important papers and monographs on foraminifera published during the first half of the 20th Century (Douglas and Woodruff, 1981 and publications cited therein). More recently, epibenthic sledge samples have proved to be an excellent source for macrofauna-sized species (e.g., Gooday, 1983; Gooday, 1986; Gooday et al., 2007; Cedhagen et al., 2009; Lejzerowicz et al., 2015). However, foraminifera have usually been picked from these samples selectively, and few if any studies describe entire assemblages.
Here, we provide an account of macrofauna-sized (>300 µm) foraminiferal species in samples collected using a Brenke epibenthic sledge (EBS) in four areas of the eastern Clarion-Clipperton Zone (CCZ) licensed for polymetallic nodule prospecting by the International Seabed Authority, and in a nearby Area of Particular Environment Interest (APEI-3), a protected area (Wedding et al., 2013) also located in the eastern CCZ. Our overall objective is to characterise the foraminiferal assemblages in these samples. Specifically, we explore 1) their taxonomic composition and species richness, 2) the distribution of species in relation to their abundance, and 3) patterns of assemblage composition across the study area. The majority of species are undescribed. We illustrate many of the abundant species, together with a selection of those that are interesting but less common. It is hoped that this study will increase awareness of the value of deep-sea samples obtained by towed devices, and particularly epibenthic sledges, in studies of deep-sea foraminiferal diversity.
The CCZ covers a total area of around 6 million km2, extending for some 4,650 km across the equatorial Pacific (McQuaid et al., 2021). Water depth generally increases from east to west, while surface productivity and Particulate Organic Matter (POM) fluxes increase from south to north and west to east and surface productivity from south to north (Morgan, 2012). Washburn et al. (2021b) present a comprehensive compilation of environmental data from across the CCZ, with mean values for different parameters given for each of nine subregions and the nine APEIs. Our samples were collected at twelve stations within the areas assigned to the contractors BGR (German), Interoceanmetal Joint Organization (IOM), DEME/GSR (Belgium) and IFREMER (France) as well as APEI-3 (Figure 1; Table 1). The French and Belgium stations lie within the Central East (CE), the IOM and German stations lie, respectively, to the north and the south of the boundary between the CE and South East (SE) subregions (Washburn et al., 2021b).
Figure 1 Map of the Clarion-Clipperton Zone showing the general location of sampling sites in the German, IOM, Belgium, and French areas and APEI-3.
Table 1 Station data, based on Table 7.2.6.7 in Martínez Arbizu and Haeckel (2015).
Distances between the mean positions of the 2-3 EBS sites in each of our five areas vary from 256 km (German to IOM) to 1440 km (German to APEI-3). APEI-3 shows the greatest separation from the other sites, with the exception of the French, which is located closer to APEI-3 (578 km) than it is to the other sites (minimum 740 km from French to Belgium).
Data for some key environmental parameters obtained during cruise SO239 from close to our sampling sites are summarised in Table 2. The more northerly APEI-3 site is clearly more oligotrophic than the four license areas, as indicated by lower estimated POC flux to the seafloor, sediment TOC values, and chloroplastic pigment concentrations. The highest values for these parameters were in the German area. Actual POC flux values at APEI-3 may have been overestimated since increased degradation of organic matter resulting from the weaker oxygen minimum zone in the overlying water column probably decreased the amount of organic matter reaching the seafloor (Volz et al., 2018). Sedimentation rates at APEI-3 are also lower and the sediments different, with a higher proportion of clay. Nodule density is very variable across the CCZ at scales varying from less than 1 km to 100 km (Washburn et al., 2021b). At our sites they are high at the German-PA, Belgium and French sites, much lower at the German-RA and APEI-3 sites, and particularly the IOM site. Bottom-water oxygen concentrations are fully oxic, although somewhat higher in the deeper French area and APEI-3.
Table 2 Environmental data based on samples obtained during RV Sonne Cruise SO239 from sites close to EBS deployments. Sed. rate = Sedimentation rate.
Samples were collected in the four license areas and APEI-3 using an epibenthic sledge (Brenke, 2005). Those used for foraminiferal analyses originated from 12 deployments (2-3 in each area) ranging between 4093 and 5028 m in depth (Table 1). As soon as possible after the sledge arrived on deck, the supra- and epi-net cod ends were removed. In the cold room, the samples were carefully elutriated with cold (+4°C) sea water, then sieved through a 300-µm mesh and immediately transferred to pre-cooled (-20°C) 96% ethanol (EtOH). These samples were stored at -20°C for at least 48 h, and during the first 12 hours, they were gently rotated every three hours to ensure thorough preservation and avoid freezing. After 12-24 hours the EtOH was replaced and samples again stored at -20° until further processing.
The samples for foraminiferal analysis was obtained opportunistically as follows. First, part of the material collected during each EBS deployment (‘German 20’, ‘IOM 81’ etc.) was sorted on the ship for metazoan macrofauna by other cruise participants. Several subsamples of the sorted residue where then taken at random from each deployment for foraminiferal analyses and preserved in separate vials in 96% EtOH. These subsamples cannot be considered as replicates because they come from one EBS deployment. The combined residues in these vials constituted our working material and are referred to hereafter as ‘samples’. Their volumes (the sum of the residues in each vial) are given in Table 1. Either two or three such samples were obtained from separate EBS deployments in each of the five study areas, 12 samples in total (Table 1). Since the samples from within one area came from separate EBS deployments they are regarded as replicates for that area.
In the laboratory at the University of Szczecin, the residues were sorted for benthic foraminifera under either a Nikon SMZ 1500 or Nikon AZ100 stereo microscope and each complete and fragmentary specimen photographed using a Nikon DS-Fi2 camera. In principal, all specimens were picked, but in practice it was not possible to take every small fragment, particularly of abundant tubular taxa, notably Rhizammina and some Komokioidea, for example, the tangles of fine Lana tubules that were often emeshed with detritus. Many abyssal monothalamids have sparse cytoplasm do not stain well with Rose Bengal (Kuhnt and Collins, 1995). As a result, no attempt was made to distinguish specimens that were living when collected from those that were dead. An effort was made to distinguish intact specimens from fragments, although this was not always easy. Based on their morphological characteristics, specimens are either assigned to know species or to working morphotypes.
As explained above, the sampling at each station (German 20 etc.) was conducted without replication since the material originated from a single EBS deployment. It was not possible, therefore, to perform reliable statistical tests based on samples from one station. However, data from separate stations within one area (e.g., APEI-3 192, 197, 210) could be treated as replicates from that area. These area-level data were suitable for non-metric multi-dimensional scaling (nMDS) in order to identify similarities/dissimilarities in the distribution of foraminifera within the area, based on their taxonomic structure (presence/absence) or specimen counts (number of specimens per species). For this purpose, nMDS was performed with PRIMER v. 7 (Plymouth Routines In Multivariate Ecological Research; Clarke et al., 2014; Clarke and Gorley, 2015). Software modules that performed ordination in the form of similarity/dissimilarity analysis (SIMPER), as well as MDS, were applied. Results were based on all data (complete specimens plus fragments) in order to ensure the maximum geographical coverage, as well as on species presence/absence, since this is most appropriate for our semi-quantitative data. SIMPER implements a ‘similarity percentage’ routine that decomposes average Bray-Curtis dissimilarities between all pairs of samples (one from each group), or all similarities among samples within each group, into the percentage contributions of each species; these are then listed in decreasing order of their contributions. In order to limit the list of species that contributed to similarity or dissimilarity (i.e., within stations and between areas, respectively), the list was confined to those accounting for more than 50% of intragroup similarity and more than 50% of dissimilarity between groups. The significance of differences in species composition across the twelve stations was tested using the analysis of similarity, implemented in the PRIMER module ANOSIM.
A major limitation in deep-sea foraminiferal studies that include the highly diverse monothalamids is the number and size of the samples required to capture their entire taxonomic richness. We used rarefaction curves, specifically sample-size-based rarefaction and extrapolation (R/E) sampling curves, in order to estimate sampling completeness (the differences between the observed richness and the estimated asymptotic richness). This approach estimates diversity with confidence intervals as a function of the sample size up to double the reference sample size (Chao et al., 2014). By extrapolating the estimated rarefaction curves, the additional number of sampled individuals needed to detect the total estimated species richness in the sampling areas can be estimated. Sample completeness curves were also constructed by taking the known species richness at actual sample sizes and then determining how much the species richness would increase if entire foraminiferal assemblages were sampled. The Shannon-Wiener index was calculated assuming full sample coverage. These analyses were performed using the R package iNEXT for the interpolation (rarefaction) and extrapolation of the Hill numbers, representing an intuitive and statistically rigorous diversity measure (Hill, 1973; Chao et al., 2014; Hsieh et al., 2016).
We picked a total of 1954 foraminiferal specimens from the 12 samples, of which 1147 were considered to be complete and 811 were considered to be fragments (Table 3). These spanned a wide range of morphotypes that we grouped into a pragmatic mixture of formal taxa and informal, morphology-based groupings (Table 3). Only 27 specimens (1.38%) were multichambered members of the classes Globothalamea, Tubulothalamea and Nodosariata (‘lagenids’), the remaining 1931 (98.6%) being single-chambered Monothalamea (‘monothalamids’). The most common group was the Komokiidae (586 specimens = 29.9%), a member of the superfamily Komokioidea (‘komoki’), followed by fragments of the tubular genus Rhizammina (368 = 18.8%, all fragments), the komoki family Baculellidae (326 = 16.6%), and finally other tubular forms, the majority of them branched (218 = 11.1%). Among this material we recognised 280 morphospecies and morphotypes (hereafter, ‘species’), some based on fragments rather than complete specimens (Table 3). A selection of the more important or interesting species are illustrated in Figures 2-6 and Supplementary Figures S1–16, with brief descriptions and taxonomic notes being given in the taxonomic appendix (Supplementary Material). Only 33 (11.9%) species have been scientifically described. These include many of the komoki species established by Tendal and Hessler (1977), based on material from the central North Pacific, but also some species that have been reported from other oceans.
Figure 2 Komoki. (A) Edgertonia argillispherula Tendal & Hessler, 1977; Belgium area, sample 118. (B) Edgertonia tolerans Tendal & Hessler, 1977; IOM area, sample 99. (C) Reticulum conical; Belgium area, sample 118. (D) Reticulum pad-like; IOM area, sample 99. (E) Septuma brachyramosa Kamenskaya, 1993; Belgium area, sample 118. (F) Mudball with dotted surface; German area, sample 50.
Twenty (7.14%) of the 280 species were multichambered (Table 3). These comprised 11 members of the Globothalamea (mainly agglutinated textulariids), 7 Tubulothalamea (miliolids) and 2 ‘lagenids’ (Supplementary Figures S1C–F). All other species were monothalamids. The main groups were the komoki (Figures 2, 3F, 4, 5; Supplementary Figures S5E, F; S8–S14), which included 37 species assigned to the family Baculellidae, 36 to the Komokiidae, and 2 to the Normaninidae (13.2%, 12.9%, 0.71%, respectively, total 26.8%), a variety of chains-like (33 = 11.9%) (Figure 3E; Supplementary Figures S2D–G), and ‘komoki-like’ (32 = 11.4%) (Figures 3A, B) forms, and branched and unbranched tubes (33 = 11.8%, 14 = 5.0%, respectively, excluding Rhizammina) (Figure 3C; Supplementary Figures S2C, S4C–F, S5A–C, S6, S7C–F).
Figure 3 Various monothalamids. (A) Cerebrum-like form; French area, sample 158. (B) Cluster of wide tubes; Belgium area, sample 133. (C) Dichotomously branching tree A; APEI-3, sample 192. (D) Mudball with big chambers; German area, sample 50. (E) Chain with prominent tubercles; APEI-3, sample 192. (F) ? Edgertonia sp. 5; French area, sample 171.
Figure 4 Komokia-like komoki from APEI-3. (A) Sample 210. (B Sample, D–F) Sample 192. (C) Sample 197.
Figure 5 Indeterminate Komokiidae from APEI-3. (A–D) Sample 210. (E, F) Same species from Sample 197.
The remaining 73 monothalamid species were distributed among different agglutinated groups (Table 3). They included various astrorhiziids with mud-walled tests, assigned to Pelosina spp., Globipelorhiza spp., and a branched stellate form somewhat similar to the genus Radicula (Supplementary Figures S3A–D,G), species with spherical tests identified as Crithionina, Storthosphaera, and Thurammina (Supplementary Figures S1A,B, S2A, B), three species of Rhizammina (Supplementary Figure S4A, B), fragments of Saccorhiza (Supplementary Figures S5D) and Hyperammina species, and two species of Vanhoeffenella. A variety of forms that are difficult to classify morphologically are grouped together as ‘other monothalamids’. Finally, the samples yielded a small but diverse collection of xenophyophores, comprising a total of 21 (7.50%) morphologically distinct forms, all of them represented by one or a few fragments (Figure 6; Supplementary Figures S15, S16). The only xenophyophore species that could be confidently identified were Aschemonella ramuliformis Brady, 1884 and Spiculammina delicata Kamenskaya, 2005, but another is morphologically identical to Occultammina sp. of Gooday et al. (2017a), while two others are possibly the same as Psammina multiloculata Kamenskaya, 2005 and Xenophyophore sp. 2 of Gooday et al. (2017a). The others appear to be previously unreported.
Figure 6 Xenophyophores; all specimens are fragments. (A) Stannoma-like species; French area, sample 171. (B) Spiculammina delicata; French area, sample 158. (C) 'Mud xenophyophore'; IOM area, sample 99. (D) Psammina-like form with internal compartments; IOM area, sample 81. (E) Galatheammina-like form with poorly defined internal compartments; German area; sample 20. (F, G) Abyssalia-like species; IOM area, sample 99.
Corresponding data based on complete specimens only and fragments only are summarised in Supplementary Tables S1 and S2, respectively. Komoki (families Baculellidae, Komokiidae, Normaninidae) account for the majority (68.2%) of complete specimens and a substantial minority (30.7%) of complete species. A somewhat lower proportion of both fragments (64.4%) and fragmented species (28.0%) were assigned to branched and unbranched tubes and Rhizammina.
Between 74 (IOM) and 121 (Belgium) species occurred in particular areas (Table 3) and 38 (German 20) to 84 (Belgium 118) in individual samples. The Belgium samples also yielded the largest numbers of specimens, 2.00 and 1.14 times more than the German and APEI-3 samples, respectively (Table 3). We used rarefaction curves and sample coverage completeness curves (Figures 7,8) in order to estimate the completeness of sampling (differences between the observed and the estimated asymptotic richness). Considering the current levels of coverage achieved based on existing sample sizes (German area 84%, French area 86%, IOM area 88%, Belgium area 90%, APEI-3 94%), it would require an increase in sample size by a factor ranging from 3.5 (Belgium area) to approximately 7 (German area) in order to achieve 100% coverage (Figure 8). Table 4 summaries the species richness and diversity values based on existing samples (actual data) compared to predicted values based on ‘complete’ sampling. Increasing the sample to approximately 2000 specimens would result in an increase in species richness by up to almost threefold (1.74 to 2.94), but lower increases in diversity indices, particularly the Simpson index.
Figure 7 Sample-size-based rarefaction and extrapolation curves, estimating foraminiferal species richness as a function of the sample size (specimen number) up to double the reference sample size (Chao et al., 2014).
Figure 8 Sample completeness curves based on actual species richness, extrapolated to estimate how species richness would increase if entire foraminiferal assemblages were sampled.
Table 4 Observed (actual data) and predicted (assuming full sample representativeness) values of species richness and diversity in the five areas.
The majority of species in our samples are rare. A total of 117 are singletons represented by one complete specimen or fragment, and 49 are doubletons represented by two specimens or fragments. The singletons are, by definition, confined to one sample, while 33 doubletons also occur in one sample, with the remaining 16 occurring in 2 samples. These 166 species represent 59.3% of all species but only 11.0% of all specimens and fragments. The total number of species occurring in only one or two samples (i.e., those represented by ≥3 specimens as well as singletons and doubletons) is 201 (71.8% of total), and these account for 385 specimens (19.7% of total). In contrast, only a few species are widely distributed in our samples (Table 5). Two (0.71%) were found in 11 samples, five (1.79%) in 10-11 samples, seven (2.50%) in 9-11 samples and nine (3.21%) in 8-11 samples. However, although these widely distributed species represent only a small fraction of all species, they include a much greater proportion of all specimens, respectively 13.0%, 18.4%, 22.6% and 36.2%. A similar pattern emerges at the area level. Twelve species (4.29%) that occur in all five areas and 20 (7.27%) that occur in 4-5 areas account for 25.0% and 38.4%, respectively, of specimens, whereas the much larger number of species (188 = 67.1%) that are confined to one area account for 28.0% of specimens. Of those confined to one area, 150 (53.6% of total species numbers) are singletons and doubletons that represent only 9.37% of all specimens.
Table 5 Distribution in samples and areas of 35 top-ranked species represented by 10 or more complete or fragmentary specimens.
Clearly, species that are widely distributed across our samples represent a disproportionally high number of specimens, compared to those that are confined to one or a few samples. However, not all common species are widely distributed (Table 5). Of the 79 species represented by 5 or more specimens, 17 (21.5%) are found in only one area, and 16 of these, including Komokia-like sp. (107 specimens, ranked 4th) and Indeterminate Komokiidae (43 specimens, ranked 6th), are restricted to APEI-3 (Supplementary Table S3).
SIMPER results based on the presence/absence of species, including those represented by fragments as well as complete specimens, show that the overall Bray-Curtis dissimilarity in species composition between areas was quite high, ranging from 86.9% (German area vs APEI-3) to 64.2% (German vs IOM area), while similarity between samples within an area ranged from 29.1% (German) to 45.1% (APEI-3) (Table 6). Relatively few species are responsible for the taxonomic similarity of samples from the same area, while the dissimilarity between areas or groups of areas reflects the influence of a larger suite of species, many of them rare. The corresponding MDS plot (Figure 9) reveals a close relationship between the IOM and German areas with the Belgium and French area being progressively more distinct from the German/IOM grouping. The APEI-3 area is clearly well separated from these other four areas.
Table 6 Results of the SIMPER analysis for the similarity/dissimilarity of the taxonomic structure (presence/absence of species) of macrofauna-sized foraminifera based on complete specimens and fragments combined.
Figure 9 MDS plot based on the presence/absence of species, including those represented by complete specimens and fragments.
SIMPER analysis, based again on all data but incorporating the numbers of complete specimens and fragments, yielded a similar result; dissimilarity between areas ranged from 91.9% (German vs APEI-3) to 64.7% (IOM vs Belgium) while similarity within areas ranged from 28.1% (French) to 56.5% (Belgium) (Supplementary Table S4). The corresponding MDS plot (Supplementary Figure S17) shows a similar pattern to that based on presence/absence. In order to check the consistency of these patterns we obtained additional MDS plots using different databases (complete specimens only, complete + 1 fragment, fragments only, all data combined) and based on either presence/absence or specimens counts. These are again broadly comparable (Supplementary Figures S18–20). The APEI-3 area is always well separated from other areas. The French area is always somewhat distinct, but much closer to the German, IOM and Belgium areas than to APEI-3. The German and IOM samples are usually the most closely related and the Belgium area usually located nearby (but see Supplementary Figure S18A). In general, all areas except APEI-3 tend to be more closely related when species counts are included.
The general faunal pattern revealed by the MDS analyses is consistent with the fact that 63.8% of the species present in the APEI-3 samples are not recorded elsewhere (Supplementary Table S3), compared with 41.8% (French), 40.8% (Belgium), 30.5% (German), and 27.0% (IOM) in other areas. Similarly, the number of species shared between the German, IOM and Belgium areas is generally higher than the number shared with the French area: either 31 (German/IOM; Belgium/IOM) or 34 (German/Belgium) compared to 24 (French/German, French/APEI-3), 28 (French/IOM), and 32 (French/Belgium). The number of morphospecies shared with APEI-3 is consistently lower than for any other area: 18 (APEI/German; APEI/IOM), 24 (APEI/French), 28 (APEI/Belgium). The differences in the species composition of assemblages between different areas were very significant, as indicated by the results of ANOSIM (sample statistic, Global R: 0.727, significance level of sample statistic: 0.2%).
There was much greater consistency in the composition of assemblages at the higher grouping/taxon level (Table 3). However, members of the Komokiidae tend to be more common in the APEI-3 area, where they represent 22.7% of species compared to between 12.2% (French) and 21.6% (IOM) elsewhere. Three delicate komokiids accounted for >50% of the similarity between the samples from APEI-3 and much of its distinctive character (Supplementary Table S4). As mentioned above, a Komokia-like species (Figure 4) and Indeterminate Komokiidae (Figure 5) are confined to this area, while species of Ipoa (Supplementary Figures S12A–C) are notably more abundant. Several distinctive but uncommon species, ‘dichotomously branching tree’ (Figure 3C), Normanina saidovae (Supplementary Figure S12F), and ? Edgertonia sp. 5 (Supplementary Figures S9E, F), were also found only in the APEI-3 samples (Supplementary Table S3). Conversely, tubular fragments of Rhizammina spp. (Supplementary Figures S4A, B) were absent at APEI-3 but common elsewhere, notably in the German and Belgium areas.
Epibenthic sledge samples are either qualitative or at best semiquantitative, although the samples they collect generally reflect the relative abundance of different species (Brenke, 2005; Kaiser and Brenke, 2016). An additional and more serious issue in the case of foraminifera is that of fragmentation, combined with the difficulty of distinguishing fragments from complete specimens. Tubular species are particularly prone to fragmentation and a single specimen, for example of Rhizammina, could potentially generate many hundreds of fragments (Bubik, 2019). Obvious fragments made up 40.9% of the specimens in our material, while specimens that we were fairly confident were complete made up 45.5%. The remaining 13.6% were considered to be possibly complete. For our analyses, the ‘complete’ and ‘possibly complete’ specimens were combined. The ‘possibly complete’ category represented 29.9% of this combined total, giving some idea of the potential error in differentiating fragments and complete tests.
Given these possible sources of error in specimen counts and the semiquantitative nature of the samples, we have placed the greatest emphasis on analyses (MDS, SIMPER and ANOSIM) based on the presence or absence of species. However, MDS plots that incorporate species abundances and use different databases show essentially the same relationships between assemblages in the five areas. This even applies when complete specimens and fragments are combined and specimen counts are included, a somewhat questionable procedure. This consistency encourages us to believe that the patterns are robust.
Although foraminifera typically account for a large proportion of macrofaunal organisms in abyssal box cores (Tendal and Hessler, 1977; Bernstein et al., 1978), entire assemblages, including delicate monothalamids, are rarely evaluated. In one of the few such studies, Shires (1994) described monothalamid-dominated (>94%) assemblages >500 µm from the Porcupine and Madeira abyssal plains (NE Atlantic) and from the Arabian Sea, where komoki accounted for 40.1%, 60.0% and 45.7%, respectively, of the complete plus fragmented specimens with xenophyophore fragments also being very common, particularly at the Porcupine Abyssal Plain site (data summarised in Gooday et al., 1997). Rhizammina fragments, agglutinated spheres, and at the two Atlantic sites chain-like forms, were also common elements. More than 100 species were recognised in each of the Atlantic samples, again mainly komoki, together with spheres and chains (Supplementary Table S5; data summarised in Gooday et al., 1998). The only other study to provide some quantitative data is that of Bernstein et al. (1978), which was based on five central North Pacific box cores (>297 µm fraction). Komoki represented a quarter of a subset of 7984 specimens and fragments picked from five subcores of box core H-153 that were sliced into layers down to 10-cm depth. These included 56 komoki species compared to 85 species belonging to other foraminiferal taxa, a proportion that is higher than in our samples (42.7% compared to 25.5%), although the number of species is lower than the 75 species that we recognised. In a broad sense, therefore, the macrofaunal fractions of these box cores provide a view of foraminiferal assemblages that is similar to that of our epibenthic sledge samples, although box cores are quantitative samplers whereas the EBS is not.
Smaller multicores provide a rather different perspective. Data from different size fractions of 10-cm diameter cores (‘megacores’) from the eastern CCZ are summarised in Supplementary Table S5: >250 µm (Stachowska-Kamiǹska et al., 2022), >150 µm (Goineau and Gooday, 2019), and >63 µm (Gooday and Goineau (2019). The most striking difference is that, while monothalamids represent the majority of species in all cases, they dominate to a greater extent (92.7%) in the EBS samples compared to the megacore samples, where they account for 78.5%, 76.6% and 75.5% of species in progressively smaller size fractions. The majority of the multichambered species in the core residues are textulariids, and to a lesser extent rotaliids (at least in the >150 µm and >63 µm fractions). All of the core data are based on the 0-1 cm layer and on combined live and dead tests. If deeper sediment layers had been included, then the proportion of multichambered taxa may have been higher. Among the monothalamids, komoki, komoki-like, and chain-like species are all better represented in the EBS samples, and xenophyophores and large mud-walled astrorhiziids (mainly Pelosina and Globipelorhiza) are largely confined to the EBS samples. The komoki genus Septuma is common in our material but rare (only 6 specimens from 9 samples) in the multicorer residues (>250 µm) of Stachowska-Kamiǹska et al (2022) from the IOM area. This genus was also not recorded in a qualitative study by Kamenskaya et al. (2012) of monothalamids in multicore samples, also from the IOM area. On the other hand, Nodellum-like forms, Lagenammina spp. and flasks, saccamminids (mainly soft-walled forms), organic-walled ‘allogromiids’, and spheres (except for Crithionina, Storthosphaera, and Thurammina) are too small to be routinely retained by the EBS and were found only in megacore samples, particularly in the finer fractions.
Clearly, the Brenke epibenthic sledge and different size fractions of megacores give contrasting views of benthic foraminiferal assemblages in the eastern CCZ. The megacorer is a quantitative device that collects sediments down to at least 10 cm depth and provides a much better representation of smaller foraminifera than the EBS. The EBS is basically a qualitative sampler that concentrates mainly larger macrofauna and smaller megafaunal organisms from a much larger area of seafloor than that sampled by the megacorer (Kaiser and Brenke, 2016). Another point to consider is that the EBS stirs up sediment during its passage across the seafloor and therefore has a bias, at least in the eastern CCZ, towards lighter, easily resuspended foraminifera, such komoki and delicate tubes, compared to heavier, macrofauna-sized, multi-chambered tests. Komoki occur mainly in the upper 0-2 cm layer of sediment (Tendal and Hessler, 1977; Kuhnt and Collins, 1995; Kamenskaya et al., 2012) and are therefore easily sampled by the EBS, which is well suited to collecting organisms associated with the sediment-water interface (Kaiser and Brenke, 2016).
Our samples yielded a core group of common, widely distributed foraminiferal species found in 4 or 5 areas and a much larger number of rare species found only in 1 or 2 areas. This is a common pattern in the deep sea, seen, for example, among abyssal polychaetes in the NE Atlantic (Glover et al., 2001) and polychaetes, tanaids and isopods in the eastern equatorial Pacific (Glover et al., 2002; Washburn et al., 2021a). Singletons and doubletons included well over a half (166 = 59.3%) of the 280 species in our samples but a much smaller proportion (11.0%) of specimens. Such species are inevitably confined to one or in some cases two samples and sites. In a synthesis of macrofaunal biodiversity data from the CCZ, Washburn et al. (2021a) concluded that, overall, >49% of polychaete species and >45% of isopod and tanaid species, are represented by one or two specimens (based on 473 box cores from which a total surface area of 109.2656 m-2 was sampled for macrofauna; Table 1 in Washburn et al., 2021a). Among meiofaunal taxa, Hauquier et al. (2019) recognised 156 nematode genera in multicore samples taken at the same five sites during the same cruise (SO239) as our EBS samples. Almost half (72 = 46.2%) were confined to one area but these were rare, contributing only 1.82-4.25% towards the nematode densities, while the minority of genera (23 = 14.7%) found in all areas accounted for 42.5-60.5%. This pattern was mirrored by 24 species of the nematode genus Halalaimus, which was analysed in more detail (Hauquier et al., 2019). Similarly, a study of megafaunal ophiuroids in EBS samples from six areas in the eastern CCZ and one (DISCOL) in the SW Pacific reported that 31 of the 43 species recognised were confined to one or two sites, compared to three species (ranked 1st, 2nd and 5th in abundance) that were found in six of the seven areas (Christodoulou et al., 2020).
Twenty common, widely distributed foraminiferal species (7.14% of species, 38.4% of specimens) occurred in 4 or 5 areas. Although only about 12% of our 280 species can be confidently identified, many of these named species were among those widely distributed in our samples. They include Baculella hirsuta, Edgertonia argillispherula, E. floccula, Septuma ocotillo + komokiformis (found in all 5 areas), B. globofera, Ipoa fragila, Septuma brachyramosa, and Normanina conferta (4 areas). These are all common komoki species, most of them described from the central North Pacific (Tendal and Hessler, 1977) and also reported from the Atlantic Ocean (Schröder et al., 1989; Kamenskaya 1993). Several less common species (Hormosinella distans, Nodosinum gaussicum, Storthosphaera alba) found in 3 areas are also well known and widely reported from different oceans (e.g., Brady, 1884; Cushman, 1910; Schröder et al., 1988). Indeed, many deep-sea foraminiferal species, notably those living on abyssal plains, are said to be ‘cosmopolitan’ (e.g., Holbourn et al., 2013), albeit usually without genetic confirmation. This is consistent with a general perception that species are often widely distributed across abyssal plains and other deep-sea habitats (McClain and Mincks Hardy, 2010). In the CCZ, some more common macrofaunal species span distances of 1000-3000 km (Washburn et al., 2021a), and some megafaunal xenophyophores have similar ranges that are genetically supported (Gooday et al., 2020b).
A few of our more common species, however, are confined to one area, notably the relatively oligotrophic APEI-3 area (Supplementary Table S3). The two prime examples are ? Komokia sp. and ‘Indeterminate Komokiidae’ (Figures 4, 5). Conversely, species that are rare in our samples do not necessarily have restricted distributions more generally. For example, our samples yielded a single specimen of Cribrostomoides subglobosa, one of the most common and widely reported multichambered species in the North Pacific (Cushman, 1910), including the eastern CCZ (Gooday et al., 2021). Several other multichambered species, including Hormosina ovicula, Karreriella bradyi, Pyrgo depressa, that are well known and widely distributed in the deep sea (Murray, 1991; Holbourn et al, 2013), are also represented by only one or two specimens. Clearly, these relatively small species are either not well sampled by the EBS or lost during subsequent sieving. However, these are exceptions and many of our rare species are undescribed and have never been recorded before. In such cases, it is impossible to determine whether they have restricted distributions or whether they occur elsewhere but remain undiscovered. Neither of these scenarios can be assumed in any particular case (Washburn et al., 2021a). The commonness of rarity is a pervasive problem in the deep sea (McClain, 2021) and severely hinders the detection of endemic distribution, even for a relatively well-studied group such as the foraminifera (Gooday and Jorissen, 2012). Although many well-known deep-sea foraminifera are widely distributed, genetic support for large ranges is often lacking, particularly for agglutinated species. It is therefore difficult to exclude the possibility that apparently cosmopolitan deep-sea foraminiferal species encompass genetically distinct cryptic species with more restricted distributions, as has been shown for some polychaetes (Washburn et al., 2021a). There are counterexamples of abyssal species, for example, the protobranch bivalve Ledella ultima, that show only modest genetic differentiation over large distances (Etter et al., 2011). Unfortunately, attempts to sequence the komoki that are common in our EBS samples have not yielded convincing results (Lecroq et al., 2009), making them a particular challenge in this regard.
Foraminiferal assemblages from the German, IOM and Belgium license areas are fairly similar, whereas those from APEI-3 are clearly distinct in MDS plots. This is reflected in the larger proportion of species that are unique to APEI-3 (48.9%), compared to the German (33.7%), Belgium (33.0%) and IOM (25.4%) areas. Of the 17 species represented by six or more specimens that are confined to one area, 16 are found in APEI-3 (Supplementary Table S3), including the two ranked 4th and 6th overall (Figures 4, 5). The French area is also somewhat separated from these three in the MDS plots and has a rather higher proportion of unique species (38.1%), although still far less than for APEI-3. The number of shared species reflects the same pattern: APEI-3 18-28 and French 24-32, compared to 31-34 between the German, IOM and Belgium and other areas. These results are consistent with the above-mentioned observations on meiofaunal nematodes and megafaunal ophiuroids. Compared to other areas, APEI-3 had lower nematode densities and higher numbers of Halalaimus species (Hauquier et al, 2019). For ophiuroids, only one of the 10 most abundant species was present in APEI-3, five of the top 10 species found in APEI-3 were confined to that area, while three of the most widely distributed species occurred in five of the six CCZ areas but not APEI-3 (Table 2 in Christodoulou et al., 2020). The number of shared ophiuroid species in APEI-3 was lower, and the number of unshared species higher, than in any other area. This pattern was reflected in a nMDS plot that also revealed a close similarity between the Belgium and IOM area although, in contrast to our results, the German area was the most distant (Figure 24 in Christodoulou et al., 2020).
The APEI-3 area has a number of environmental characteristics that tend to set it apart from our other study areas, the main ones being the lower concentrations of phytopigments and total organic carbon (TOC), finer sediments and lower nodule density (Table 2; Hauquier et al, 2019; Volz et al., 2020). The French area is the deepest, followed by APEI-3, which is considerably further north that the other areas and located under less productive surface waters. Phytopigment concentrations and TOC values reflect the flux of particulate organic matter (POC) to the seafloor, which is believed to exert an important influence on regional patterns of abundance, diversity and other sediment community attributes (Levin et al., 2001; Rex and Etter, 2012), particularly on food-limited abyssal plains (Smith et al., 2008), including the CCZ (Washburn et al., 2021a), where many other parameters are relatively stable. In the eastern Pacific, productivity is enhanced as a result of upwelling in the equatorial region and decreases in a northerly direction towards APEI-3, as well as to the south (Smith et al., 1997). The species richness and diversity of foraminifera in our APEI-3 samples are not obviously depressed compared to other areas (Table 4) and given the semi-quantitative nature of our material, we cannot conclude anything regarding foraminiferal abundance. However, the clear differences in assemblage composition between APEI-3 and other areas, is likely to be real and may be plausibly linked to a reduced POC flux to the seafloor.
Many attributes of foraminiferal assemblages appear to be strongly linked to the POC flux (e.g., Murray, 2006; Gooday et al., 2012). These include the distribution of individual species and the overall taxonomic composition of assemblages (Altenbach et al., 1999; Loubere and Fariduddin, 1999). A series of studies by Loubere, (1991, 1994, 1996) used multivariate regression and principal component analyses to analyse benthic foraminifera in gravity and piston core-top samples from lower bathyal depths (2,200 to 3,200 m) in the eastern equatorial Pacific, the SE Pacific along the East Pacific Rise, and at scattered sites in the North Pacific. He found relationships between foraminiferal assemblages and a combination of bottom-water oxygenation and surface productivity, but with productivity being the dominant driver of assemblage composition. Oxygen concentrations at Loubere’s sites ranged from <2 to about 3.5 ml/L (Figure 3 in Loubere, 1996), a much wider range that across our study areas (144 to 156 µM = about 3.2 to 3.5 ml/L; Table 2), suggesting that reduced POC flux to the seafloor as a consequence of lower surface productivity and greater water depth probably exerts the strongest influence on APEI assemblage composition.
Komoki are an important component of our assemblages. Historically, the best and most diverse collections of these organisms have come from abyssal depths below 5000 m, notably in the central North Pacific (Tendal and Hessler, 1977; Bernstein et al., 1978) and the NW Atlantic Nares abyssal plain (Schröder et al., 1989). They are also common and diverse in EBS samples from a 5432-m deep site in the NE Atlantic, where they include 23 (29.5%) of the 78 foraminiferal species collected (Gooday, 1987). It is perhaps not surprising that komoki are slightly more common in absolute terms in the APEI-3 samples (33 species compared to 22-31 elsewhere), rather more clearly so in relative terms (37.5% of species compared to 25.6-32.2%). These assemblages from the deeper parts of the abyss are notably rich in delicate taxa such as those illustrated in Figure 4, 5 and Ipoa spp. In the NW Atlantic, Kuhnt and Collins (1995) reported differences in komoki assemblages at two abyssal sites in the NW Atlantic. One was relatively oligotrophic and characterised by small, finely-agglutinated specimens, the other relatively eutrophic and characterised by thicker-walled, more coarsely-agglutinated specimens. However, the relationship with productivity was confounded by the more tranquil nature of the oligotrophic site compared to the current-influenced eutophic site. Currents are unlikely to be a factor in the eastern CCZ, a region where seabed velocities are very low.
The small but interesting collection of fragmentary xenophyophores obtained from the EBS samples during the SO329 cruise adds to our knowledge of these large monothalamids in the CCZ. One fragment from the French license area (Station 158) can be confidently identified as Spiculammina delicata, the first record of this species from outside the Russian licence area (Kamenskaya, 2005; Kamenskaya et al., 2015; Kamenskaya et al., 2017). It was found in an EBS sample from 4946-4977 m, consistent with its depth range from 4716 to 5400 m in the Russian area. Despite extensive sampling, S. delicata has not been found at sites shallower than about 4500 m in the eastern CCZ (this study; Gooday et al., 2017a,b), suggesting that it is restricted to deeper areas. Another fragment, from the German area, is clearly the same as Occultammina sp. from the adjacent UK-1 license area (Gooday et al., 2017a). Several other forms resemble known species but are too fragmentary for their identifications to be confirmed. These include the most common xenophyophore in our material, a mud-walled species found in the Belgium, French and German license areas, which resembles Xenophyophore sp. 2 of Gooday et al. (2017a) from the UK-1 area. A fragment from the German area (Figure 6E) may represent Galatheammina interstincta from the UK-1 area (Gooday et al., 2017c), while another comprising a granellare system emeshed with sponge spicules exhibits features characteristic of the newly described genus Abyssalia (Figures 6F, G). Finally, a mass of stercomare and granellare tubes interwoven with organic fibres (linellae) is probably a damaged specimen of Stannophyllum.
The remaining five fragmentary xenophyophore morphotypes in our EBS material do not appear to have been reported previously. The most interesting of these has an elongated, flexible branched test, held together by fine, organic fibres (Figure 6A). The fibres resemble the linellae that are characteristic of the family Stannomidae (Tendal, 1972), suggesting that this form may represent a new genus of stannomid xenophyophores. The branched but linear test contrasts with the tree-like morphology of Stannoma, in which branches arise from a basal trunk. Gooday et al. (2020b, Table 1 therein) list a total of 63 described and undescribed xenophyophore species known to occur within the CCZ. Assuming that these five forms are hitherto unknown morphospecies, they increase the number of xenophyophores from the CCZ to 68, of which 44 are undescribed.
Our analyses of macrofaunal foraminifera in epibenthic sledge samples from the eastern Clarion Clipperton Zone has led to the following main results and conclusions.
1) Twelve EBS samples from four licence areas (German, IOM, Belgium, French) and one protected area (APEI-3), spanning the depth range 4093-5030 m, yielded rich collections of larger-sized (macrofaunal) foraminifera. Single-chambered monothalamids overwhelmingly predominated and represent the vast majority of the 280 morphospecies recognised. Members of the Komokioidea account for almost half of specimens and more than a quarter of species, with tubes, chain-like forms, and ‘komoki-like’ forms also making important contributions. On the other hand, multichambered taxa (classes Globothalamea, Tubulothalamea, Nodosariata), which are generally smaller with heavier tests, were rare. Only a small proportion (~12%) of all species are described.
2) The majority of species (201) were confined to 1-2 samples. Many were rare and despite representing 71.8% of all species they contributed only a relatively small proportion (19.7%) of specimens. Among them were 166 singletons and doubletons (59.2% of species but <10% of specimens). In contrast, a few species were distributed across the five study areas. Only twelve were found in all five areas and nine in eight or more samples, but these included 25.0% and 36.2%, respectively, of all specimens. Some of these widely-distributed species were komoki that had been described from the central North Pacific and subsequently reported from the Atlantic Ocean.
3) While the higher-level faunal composition was similar across the study areas, multidimensional scaling (MDS) analyses reveals differences in assemblage composition at the species level. The German and IOM areas were very similar and showed some overlap in MDS plots with the Belgium area, while the French area, which is the deepest, was somewhat distinct. APEI-3, however, was clearly separated from the other areas on MDS plots and characterised by the largest number of unique species, consistent with previous studies in the same areas based on other faunal components (Hauquier et al., 2019; Christodoulou et al., 2020). The distinctive faunal character of APEI-3 can be plausibly attributed to its location to the north of the other sites under a more oligotrophic water column.
4) In contrast to the EBS, box corers and multicorers are quantitative samplers that collect relatively undisturbed sediment from a much smaller area of seafloor. Multicore samples from the eastern CCZ yield a smaller proportion of monothalamids and more of the smaller but heavier multichambered taxa, particularly agglutinated textulariids, that were rare in our EBS samples.
Although epibenthic sledges are qualitative (Kaiser and Brenke, 2016), or at best semi-quantitative (Rice et al., 1982) devices, they are able to collect large quantities of material from a much larger area than box corers or multi-corers and are therefore well-suited to taxonomic and biogeographic studies. While our EBS samples from the abyssal CCZ have proved to be a valuable source of komoki and other delicate monothalamids, these devices can also collect many heavier and more robust species. For example, EBS samples from the NE Atlantic yielded thousands of large tubes assigned to Bathysiphon rusticus and Rhabdamina species (Gooday, 1983; Gooday, 1986), while Hyperammina crassatina tubes dominated an Agassiz trawl catch on the Oman margin (3,400 m; Gooday et al., 1997). If macrofauna-sized benthic foraminifera are present on the seafloor, epibenthic sledges will concentrate them, sometimes in huge numbers although often fragmented. They are a good source of species that are absent or uncommon in core samples, as well as large collections of taxonomically valuable specimens belonging to more common species. In this way, EBS samples can complement the multi-cores and box-cores that form the basis for many deep-sea foraminiferal studies, provide abundant material for taxonomic and genetic studies, and a more complete view of the composition of larger abyssal foraminiferal assemblages.
The raw data supporting the conclusions of this article will be made available by the authors, without undue reservation.
BW-W collected the samples during Sonne cruise SO239. The paper was jointly conceived by both authors who sorted and analysed the samples and photographed specimens together. Data treatment was undertaken by BW-W and taxonomic assignments by AG. Photographic figures were compiled by AG and final version prepared by BW-W, who also prepared the remaining figures. The paper was largely written by AG with additions from BW-W. The taxonomic appendix was prepared by AG. All authors contributed to the article and approved the submitted version.
Cruise SO239 was financed by the German Ministry of Education and Science (BMBF) as a contribution to the European project JPI Oceans “Ecological Aspects of Deep-Sea Mining”. BW-W acknowledge support provided by the Polish National Science Center Grant No. 2014/13/B/ST10/02996 and by the JPI Oceans Pilot Action “Ecological Effects of Deep-Sea Mining”. Additional support was provided by the Institute of Marine and Environmental Sciences of the University of Szczecin, Poland.
We are grateful to Magdalena Błażewicz for her help with sampling the EBS during the SO239 cruise. BW-W thanks Teresa Radziejewska for facilitating her participation in the cruise. We would like to thank Przemysław Śmietana for advice on statistical analyses. We greatly appreciate the comments of the two reviewers, which helped to improve the paper considerably.
The authors declare that the research was conducted in the absence of any commercial or financial relationships that could be construed as a potential conflict of interest.
All claims expressed in this article are solely those of the authors and do not necessarily represent those of their affiliated organizations, or those of the publisher, the editors and the reviewers. Any product that may be evaluated in this article, or claim that may be made by its manufacturer, is not guaranteed or endorsed by the publisher.
The Supplementary Material for this article can be found online at: https://www.frontiersin.org/articles/10.3389/fmars.2022.1059616/full#supplementary-material
Altenbach A. V., Pflaumann U., Schiebel R., Thies A., Timm S., Trauth M. (1999). Scaling percentages and distributional patterns of benthic foraminifera with flux rates of organic carbon. J. Foram. Res. 29, 173–185.
Barnett P. R. O., Watson J., Connelly D. (1984). A multiple corer for taking virtually undisturbed samples from shelf, bathyal and abyssal sediments. Oceanol. Acta 7, 401–408.
Bernstain B. B., Hessler R. R., Smith R., Jumars P. A. (1978). Spatial dispersion of benthic foraminifera in the abyssal central North Pacific. Limnol. Oceanogr. 23, 401–416. doi: 10.4319/lo.1978.23.3.0401
Brady H. B. (1884). “Report on the foraminifer dredged by H.M.S. Challenger during the years 1873–1876,” in Report of the scientific results of the voyage of H.M.S. Challenger, vol. 9. (London, England), 1873–1876. Zoology.
Brandt A., Gooday A. J., Brandão S. N., Brix S., Brökeland W., Cedhagen T., et al. (2007). First insights into biodiversity and biogeography of the Southern Ocean deep sea. Nature 447, 307–311. doi: 10.1038/nature05827
Brandt A., Havermans C., Janussen D., Jörger K. M., Meyer-Löbbecke A., Schnurr S., et al. (2014). Composition of epibenthic sledge catches in the south polar front of the Atlantic. Deep-Sea Res. II 108, 69–75. doi: 10.1016/j.dsr2.2014.08.017
Brenke N. (2005). An epibenthic sledge for operations on marine soft bottom and bedrock. Mar. Technol. Soc J. 39, 10–19. doi: 10.4031/002533205787444015
Bubik M. (2019). Remarks on the quantitative analysis of deep-sea agglutinated foraminiferal taphocoenoses with special attention to tubular astrorhizids. Micropaleontology 65, 63–74.
Cedhagen T., Gooday A. J., Pawlowski J. (2009). A new genus and two new species of saccamminid foraminiferans (Protista, Rhizaria) from the deep Southern Ocean. Zootaxa 2096, 9–22.
Chao A., Gotelli N. J., Hsieh T. C., Sander E. L., Ma K. H., Colwell R. K., et al. (2014). Rarefaction and extrapolation with Hill numbers: A framework for sampling and estimation in species diversity studies. Ecol. Monogr. 84, 45–67. doi: 10.1890/13-0133.1
Christodoulou M., O’Hara T., Hugall A. F., Khodami S., Rodrigues C. F., Hilario A., et al. (2020). Unexpected high abyssal ophiuroid diversity in poly:metallic nodule fields of the northeast Pacific Ocean and implications for conservation. Biogeosciences 17, 1845–1876. doi: 10.5194/bg-17-1845-2020
Clarke K. R., Gorley R. N., Somerfield P. J., Warwick R. M. (2014). Change in marine communities: an approach to statistical analysis and interpretation. 3rd edition (Plymouth: PRIMER-E).
Cushman J. A. (1910). A monograph of the foraminifera of the North Pacific Ocean. Part 1. Astrorhizidae and Lituolidae. Bull. U. S. Nat. Mus. 71, 1–134.
Douglas R. G., Woodruff F. (1981). ‘Deep-sea benthic foraminifera’, in The oceanic lithosphere. The Sea, vol. 7 . Ed. Emiliani E. (New York: John Wiley & Sons), 1233–1327.
Etter R. J., Boyle E. E., Glazier A., Jennings R. M., Dutra E., Chase M. R. (2011). Phylogeography of a pan-Atlantic abyssal protobranch bivalve: implications for evolution in the deep Atlantic. Mol. Ecol. 20, 829–843. doi: 10.1111/j.1365-294X.2010.04978.x
Gage J. D., Tyler P. A. (1991). Deep-Sea biology: A natural history of organisms at the deep-sea floor (Cambridge: Cambridge University Press, Cambridge).
Glover A. G., Paterson G., Bett B., Gage J., Sibuet M., et al. (2001). Patterns in polychaete abundance and diversity from the Madeira abyssal plain, northeast Atlantic. Deep-Sea Res. I 48, 217–236. doi: 10.1016/S0967-0637(00)00053-4
Glover A. G., Smith C. R., Paterson G. L. J., Wilson G. D. F., Hawkins L., Sheader M. (2002). Polychaete species diversity in the central Pacific abyss: local and regional patterns, and relationships with productivity. Mar. Ecol. Prog. Ser. 240, 157–170. doi: 10.3354/meps240157
Goineau A., Gooday A. J. (2019). Diversity and spatial patterns of foraminiferal assemblages in the eastern Clarion-Clipperton Zone (abyssal eastern equatorial Pacific). Deep-Sea Res. I 149, 103036. doi: 10.1016/j.dsr.2019.04.014
Gooday A. J. (1983). Bathysiphon rusticus de Folin and B. folini sp. nov. two large agglutinated foraminifers abundant in abyssal NE Atlantic epibenthic sledge samples. J. Foram. Res. 13, 262–276. doi: 10.2113/gsjfr.13.4.262
Gooday A. J. (1986). The genus Rhabdammina in the north-east Atlantic: A new species, a redescription of R. major de Folin, and some speculations on species relationships. J. Foram. Res. 16, 150–160. doi: 10.2113/gsjfr.16.2.150
Gooday A. J. (1987). ‘Rhizopoda’ in Great Meteor East: A biological characterisation, ed. Roe H. S. J.. Institute of Oceanographic Sciences Deacon Laboratory Report No. 248 (Natural Environment Research Council, Swidon), pp.153–156.
Gooday A. J. (1990). “Recent deep-sea agglutinated foraminifera: A brief review,” in Paleoecology, biostratigraphy, paleoceanography and taxonomy of agglutinated foraminifera, vol. 327 . eds. Hemleben C., Kaminski M. A., Kuhnt W., Scott D. B. (Dordrecht: Kluwer Academic Publishers), 271–304.
Gooday A. J., Bett B. J., Jones D. O. B., Kitazato H. (2012). The influence of productivity on abyssal foraminiferal biodiversity. Mar. Biodiv. 42, 415–431. doi: 10.1007/s12526-012-0121-8
Gooday A. J., Bett B. J., Shires R., Lambshead P. J. D. (1998). Deep-sea benthic foraminiferal diversity in the NE Atlantic and NW Arabian sea: a synthesis. Deep-Sea Res. II 45, 165–201. doi: 10.2113/gsjfr.27.4.278
Gooday A. J., Durden J. M., Smith C. R. (2020b). Giant, highly diverse protists in the abyssal Pacific: vulnerability to impacts from seabed mining and potential for recovery. Commun. Integr. Biol. 13, 189–197. doi: 10.1080/19420889.2020.1843818
Gooday A. J., Goineau A. (2019). The contribution of fine sieve fractions (63–150 µm) to foraminiferal abundance and diversity in an area of the eastern Pacific Ocean licensed for polymetallic nodule exploration. Front. Mar. Sci. 6. doi: 10.3389/fmars.2019.00114
Gooday A. J., Holzmann M., Caulle C., Goineau A., Kamenskaya O. E., Weber A. A. T., et al. (2017a). Giant foraminifera (xenophyophores) are exceptionally diverse in parts of the abyssal eastern Pacific where seabed mining is likely to occur. Biol. Conserv. 207, 106–116. doi: 10.1016/j.biocon.2017.01.006
Gooday A. J., Holzmann M., Caulle C., Goineau A., Pearce R. B., Voltski I., et al. (2017c). Five new species and two new genera of xenophyophores (Foraminifera: Rhizaria) from part of the abyssal equatorial Pacific licensed for polymetallic nodule exploration. Zool. J. Linn. Soc 183, 723–748. doi: 10.1093/zoolinnean/zlx093
Gooday A. J., Jorissen F. J. (2012). Benthic foraminiferal biogeography: controls on global distribution patterns in deep-water settings. Ann. Rev. Mar. Sci. 4, 237–262. doi: 10.1146/annurev-marine-120709-142737
Gooday A. J., Kamenskaya O. E., Cedhagen T. (2007) New and little-known Komokiacea (Foraminifera) from the bathyal and abyssal Weddell Sea and adjacent areas. Zool. J. Linn. Soc 151, 219–251. doi: 10.1111/j.1096-3642.2007.00326.x
Gooday A. J., Lejzerowicz F. J., Goineau A., Holzmann M., Kamenskaya O., Kitazato H., et al. (2021). The biodiversity and distribution of abyssal benthic foraminifera and their possible ecological roles: a synthesis across the Clarion-Clipperton Zone. Front. Mar. Sci. 8. doi: 10.3389/fmars.2021.634726
Gooday A. J., Schoenle A., Dolan J. R., Arndt H. (2020a). Protist diversity and function in the dark ocean – challenging the paradigms of deep-sea ecology with special emphasis on foraminiferans and naked protists. Eur. J. Protistol. 75, 125721. doi: 10.1016/j.ejop.2020.125721
Gooday A. J., Shires R., Jones A. R. (1997). Large deep-sea agglutinated foraminifera; two differing kinds of organization and their possible ecological significance. J. foram. Res. 27, 278–291. doi: 10.2113/gsjfr.27.4.278
Hauquier F., Macheriotou L., Bezerra T. N., Egho G., Martínez Arbizu P., Vanreusel A. (2019). Distribution of free-living marine nematodes in the Clarion-Clipperton Zone: implications for future deep-sea mining scenarios. Biogeosciences 16, 3475–3489. doi: 10.5194/bg-16-3475-2019
Hessler R. R., Jumars P. A. (1974). Abyssal community analysis from replicate box cores in the central North Pacific. Deep-Sea Res. 21, 185–209. doi: 10.1016/0011-7471(74)90058-8
Hessler R. R., Sanders H. L. (1867). Faunal diversity in the deep sea. Deep-Sea Res. 14, 65–78. doi: 10.1016/0011-7471(67)90029-0
Hill M. (1973). Diversity and evenness: A unifying notation and its consequences. Ecology 54, 427–432. doi: 10.2307/1934352
Holbourn A., Henderson A., McLeod N. (2013). Atlas of benthic foraminifera (Chichester: Wiley-Blackwell).
Hsieh T. C., Ma K. H., Chao A. (2016). iNEXT: An r package for interpolation and extrapolation of species diversity (Hill numbers). Methods Ecol. Evol. 7, 1451–1456. doi: 10.1111/2041-210X.12613
Kaiser S., Brenke N. (2016). ‘Epibenthic sledges,’, in Biological sampling in the deep sea. Eds. Clark M. R., M. C., Rowden A. A. (John Wiley and Sons), 184–208.
Kamenskaya O. E. (1993). Komokiacea from the region of the underwater seamount Valdivia (South-Eastern Atlantic). Transactions of the P.P. Shirshov Institute of Oceanology 127, 72–81.
Kamenskaya O. E. (2005). Spiculammina delicata gen. et sp. n., a new xenophyophore from the eastern Pacific (Psamminidae). Invertebr. Zool. 2, 23–27. doi: 10.15298/invertzool.02.1.03
Kamenskaya O. E., Gooday A. J., Radziejewska T., Wawrzyniak-Wydrowska B. (2012). Large, enigmatic foraminiferan-like protists in the eastern part of the Clarion-Clipperton Fracture Zone (abyssal eastern equatorial Pacific): biodiversity and vertical distribution in the sediment. Mar. Biodiv. 42, 311–327. doi: 10.1007/s12526-012-0114-7
Kamenskaya O. E., Gooday A. J., Tendal O. S., Melnik V. P. (2015). Xenophyophores (Protista, Foraminifera) from the Clarion-Clipperton fracture zone with description of three new species. Mar. Biodiv. 45, 581–593. doi: 10.1007/s12526-015-0330-z
Kamenskaya O. E., Gooday A. J., Tendal O. S., Melnik V. F. (2017). Xenophyophores (Rhizaria, Foraminifera) from the Russian license area of the Clarion-Clipperton Zone (eastern equatorial Pacific), with the description of three new species. Mar. Biodiv. 47, 299–306. doi: 10.1007/s12526-016-0595-x
Kuhnt W., Collins W. (1995). ‘Fragile abyssal foraminifera from the northwestern Sargasso Sea: distribution, ecology and paleoceanographic significance’ in Proceedings of the Fourth International Workshop on Agglutinated Foraminifera. Grzybowski Foundation Special Publication no. 3. Eds. Kaminski M. A., Geroch S. S., Gasinski M. A. (Grzybowski Foundation, Krakow, Poland), 159–172.
Lecroq B., Gooday A. J., Cedhagen T., Sabbatini A., Pawlowski J. (2009). Molecular analyses reveal high levels of eukaryotic richness associated with enigmatic deep-sea protists (Komokiacea). Mar. Biodiv. 39, 45–55. doi: 10.1007/s12526-009-0006-7
Lejzerowicz F., Voltski I., Pawlowski J. (2015). Foraminifera of the Kuril-Kamchatka trench area: the prospects of molecular study. Deep Sea Res II 111, 19–25.
Levin L. A., Etter R. J., Rex M. A., Gooday A. J., Smith C. R., Pineda J., et al. (2001). Environmental influences on regional deep-sea species diversity. Annu. Rev. Ecol. Evol. Syst. 32, 51–93.
Loubere P. (1991). Deep-sea benthic foraminiferal assemblage response to a surface ocean productivity gradient: a test. Paleoceanography 6, 193–204. doi: 10.1029/90PA02612
Loubere P. (1994). Quantitative estimation of surface ocean productivity and bottom water oxygen concentration using benthic foraminifera. Paleoceanography 9, 727–737. doi: 10.1029/94PA01624
Loubere P. (1996). The surface ocean productivity and bottom water oxygen signals in deep water benthic foraminiferal assemblages. Mar. Micropal. 28, 247–261. doi: 10.1016/0377-8398(96)00004-7
Loubere P., Fariduddin M. (1999). ‘Benthic foraminifera and the flux of organic carbon to the seabed’, in Modern foraminifera. Ed. Gupta B. K. S. (Dordrecht, The Netherlands: Kluwer Academic Publishers), 181–199.
Martínez Arbizu P., Haeckel M. (2015). RV Sonne SO239 Cruise Report. Berichte aus dem GEOMAR Helmholtz-Zentrum für Ozeanforschung Kiel, New Series, nr. 25 (Kiel, Germany: GEOMAR Helmholtz-Zentrum für Ozeanforschung), 204 pp. doi: 10.3289/GEOMAR.rep.ns.25.2015.
McClain C. R. (2021). The commonness of rarity in a deep-sea taxon. Oikos 130, 863–878. doi: 10.1111/oik.07602
McClain C. R., Mincks Hardy S. (2010). The dynamics of biogeographic ranges in the deep sea. Proc. R. Soc. B 277, 3533–3546. doi: 10.1098/rspb.2010.1057
McQuaid K. L., Attrill M. J., Clark M. R., Cobley A., Glover A. G., Smith C. R., et al. (2021). Using habitat classification to assess representativity of a protected area network in a large, data-poor area targeted for deep-sea mining. Front. Mar. Sci. 7. doi: 10.3389/fmars.2020.558860
Morgan C. (2012). A geological model of polymetallic nodule deposits in the Clarion-Clipperton Fracture Zone (Kingston, Jamaica: International Seabed Authority Briefing Paper 01/12), 12 p.
Murray J. W. (1991). Ecology and palaeoecology of benthic foraminifera (Harlow, Essex, U.K: Longman Scientific & Technical).
Murray J. W. (2006). Ecology and applications of benthic foraminifera (Cambridge University Press, Cambridge, New York, Melbourne, Madrid, Cape Town, Singapore, São Paulo: Cambridge University Press).
Rex M. A., Etter R. J. (2010). Deep-Sea Biodiversity: Pattern and Scale. Harvard University Press, Cambridge, MA
Rice A. L., Aldred R. G., Billett D. M. S., Thurston M. H. (1979). The combined use of an epibenthic sledge and deep-sea camera to give quantitative relevance to macro-benthic samples. Ambio Special Rep. 6, 59–72. Available at: https://www.jstor.org/stable/25099610'
Rice A. L., Aldred R. G., Darlington E., Wild R. A. (1982). The quantitative estimation of the deep-sea benthos; a new approach to an old problem. Oceanol. Acta. 5, 63–72.
Sanders H. L., Hessler R. R., Hampson G. R. (1965). An introduction to the study of the deep-sea benthic faunal assemblages along the Gay Head, Bermuda transect. Deep-Sea Res. 12, 845–867. doi: 10.1016/0011-7471(65)90808-9
Schröder C. J., Medioli F. S., Scott D. B. (1989). Fragile abyssal foraminifera (including new Komokiacea) from the Nares abyssal plain. Micropaleontology 35, 10¬48.
Schröder C. J., Scott D. B., Medioli F. S., Bernstein B. B., Hessler R. R. (1988). Larger agglutinated foraminifera: comparison of assemblages from central Pacific Ocean and western North Atlantic (Nares abyssal plain). J. Foram. Res. 18, 25–41. doi: 10.2113/gsjfr.18.1.25
Shires R. (1994). The taxonomy, morphology and ecology of novel deep-sea agglutinated foraminifera in the northeast Atlantic. (PhD Thesis). (University of Reading).
Smith C. R., Berelson W., Demaster D. J., Dobbs F. C., Hammond D., Hoover D. J., et al. (1997). Latitudinal variations in benthic processes in the abyssal equatorial Pacific: control by biogenic particle flux. Deep-Sea Res. II 44, 2295–2317. doi: 10.1016/S0967-0645(97)00022-2
Smith C. R., De Leo F. C., Bernardino A. F., Sweetman A. K., Martinez Arbizu P. (2008). Abyssal food limitation, ecosystem structure and climate change. Trends Ecol. Evol. 23, 518–528. doi: 10.1016/j.tree.2008.05.002
Stachowska-Kamiǹska Z., Gooday A. J., Radziejewska T. (2022). Macrofaunal foraminifera from a former benthic impact experiment site (IOM contract area) in the abyssal eastern Clarion-Clipperton Zone. Deep–Sea Res. I 188, 103848. doi: 10.1016/S0967-0645(97)00022-2
Tendal O. S., Hessler R. R. (1977). An introduction to the biology and systematics of Komokiacea. Galathea Rep. 14, 165–194.
Thiel H. (1983). “Meiobenthos and nanobenthos of the deep sea” in The Sea, Vol. 8 . Ed. Rowe G. T. (New York: Wiley Interscience), 167–230.
Volz J. B., Haffert L., Haeckel M., Koschinsky A., Kasten S. (2020). Impact of small-scale disturbances on geochemical conditions, biogeochemical processes and element fluxes in surface sediments of the eastern Clarion-Clipperton Zone, Pacific Ocean. Biogeosciences 17, 1113–1131. doi: 10.5194/bg-17-1113-2020
Volz J. B., Mogollón J. M., Geibert W., Martínez Arbizu P., Koschinsky A., Kasten S. (2018). Natural spatial variability of depositional conditions, biogeochemical processes and element fluxes in sediments of the eastern Clarion-Clipperton Zone, Pacific Ocean. Deep-Sea Res. I 140, 159–172. doi: 10.1016/j.dsr.2018.08.006
Washburn T. W., Jones D. O. B., Wei C.-L., Smith C. R. (2021b). Environmental heterogeneity throughout the Clarion-Clipperton Zone and the potential representativity of the APEI network. Front. Mar. Sci. 8. doi: 10.3389/fmars.2021.661685
Washburn T. W., Menot L., Bonifácio P., Pape E., Błażewicz M., Bribiesca-Contreras G., et al. (2021a). Patterns of macrofaunal biodiversity across the Clarion-Clipperton Zone: an area targeted for seabed mining. Front. Mar. Sci. 8. doi: 10.3389/fmars.2021.626571
Keywords: eastern equatorial Pacific, monothalamids, xenophyophores, biogeography, APEI-3
Citation: Gooday AJ and Wawrzyniak-Wydrowska B (2023) Macrofauna-sized foraminifera in epibenthic sledge samples from five areas in the eastern Clarion-Clipperton Zone (equatorial Pacific). Front. Mar. Sci. 9:1059616. doi: 10.3389/fmars.2022.1059616
Received: 01 October 2022; Accepted: 14 December 2022;
Published: 24 January 2023.
Edited by:
Eleonora Puccinelli, Université de Bretagne Occidentale, FranceReviewed by:
Hidetaka Nomaki, Japan Agency for Marine-Earth Science and Technology (JAMSTEC), JapanCopyright © 2023 Gooday and Wawrzyniak-Wydrowska. This is an open-access article distributed under the terms of the Creative Commons Attribution License (CC BY). The use, distribution or reproduction in other forums is permitted, provided the original author(s) and the copyright owner(s) are credited and that the original publication in this journal is cited, in accordance with accepted academic practice. No use, distribution or reproduction is permitted which does not comply with these terms.
*Correspondence: Andrew J. Gooday, YW5nQG5vYy5hYy51aw==
Disclaimer: All claims expressed in this article are solely those of the authors and do not necessarily represent those of their affiliated organizations, or those of the publisher, the editors and the reviewers. Any product that may be evaluated in this article or claim that may be made by its manufacturer is not guaranteed or endorsed by the publisher.
Research integrity at Frontiers
Learn more about the work of our research integrity team to safeguard the quality of each article we publish.