- 1Department of Biology, University of Oxford, Oxford, United Kingdom
- 2Nekton Foundation, Begbroke Science Park, Oxford, United Kingdom
- 3Department of Oceanography, University of Cape Town, Cape Town, South Africa
- 4SAEON (Elwandle Coastal Node) and Coastal and Marine Research Institute, Nelson Mandela University, Pretoria, South Africa
- 5Marine and Antarctic Centre for Research and Innovation (MARIS), University of Cape Town, Cape Town, South Africa
- 6School of Justice, Security and Sustainability, Staffordshire University, The Science Centre, Stoke-on-Trent, United Kingdom
Understanding the transport and accumulation of microplastics is useful to determine the relative risk they pose to global biodiversity. The exact contribution of microplastic sources is hard to elucidate; therefore, investigating the Antarctic Weddell Sea, an area known for its remoteness and little human presence (i.e. limited pollution sources), will help us to better understand microplastic transportation. Here, we investigate the presence of microplastics in a range of Antarctic sample media including air, seawater, and sediment. We hypothesised that multiple transportation processes including atmospheric and oceanic vectors determine the presence of microplastics in the Antarctic. Using techniques including Polarised Light Microscopy and Raman Spectrometry, we identified mostly fibres and categorised them based on their optical and chemical properties. A total of 47 individual microplastic categories (45 of which were fibres) were identified in the air, seawater, and sediment samples. The majority of categories did not overlap multiple media (42/47); however, four fibre categories were present in both air and water samples, and another fibre category was found in all three media (category 27). We suggest that the large variety of fibres identified and the overlap of fibre categories among media indicates that the pollution may result from multiple diffuse sources and transportation pathways. Additionally, our Air Mass Back Trajectory analyses demonstrates that microplastic fibres are being transported by air masses or wind, and strongly suggests that they are transported to the Antarctic from southern South America. We also propose that fibres may be transported into the Antarctic in subsurface waters, and as pollution was identified in our sediment and additional sea ice samples, we suggest that the coastal and Antarctic deep sea may be a sink for microplastic fibres. The results shown here from a remote, near-pristine system, further highlight the need for a global response to the plastic pollution crisis.
1. Introduction
Anthropogenic stressors on the environment are pervasive and increasing on a global scale (O’Hara et al., 2021), with microplastic pollution at the forefront of recent interest and the focus of a rapidly growing number of studies. Microplastic pollution is now considered ubiquitous, occurring from the tropics to the polar regions (Curren & Leong, 2019; Mishra et al., 2021) and from the peak of Mount Everest to the deep sea (Woodall et al., 2014; Napper et al., 2020). Given the persistence and microscopic size of these plastics, attempting to remove them from marine systems would be time intensive, expensive, and highly inefficient (Beaumont et al., 2019). Instead, understanding the sources and where and how microplastic pollutants accumulate is useful to determine the relative risk they pose to global biodiversity (Jones et al., 2021). From here, steps to prevent microplastic pollution at its source and measures to mitigate the impact on the ecosystem can be implemented.
The majority of microplastics are thought to originate from land-based sources such as fabric fibres or tyre dust (Landon-Lane, 2018; O'Brien et al., 2020; Materić et al., 2022) and enter marine systems either through point sources such as wastewater treatment plants and sewer overflows, or through diffuse pathways such as rainfall, surface runoff, and wind (Siegfried et al., 2017). The exact contribution of sources or specific plastic products is hard to elucidate (Napper et al., 2022); therefore, reducing the contribution of point sources by investigating a remote marine system such as the Antarctic Weddell Sea will help us to better understand the transport of microplastic pollution.
The Weddell Sea is one of the most isolated regions of the Antarctic with very limited access for human activities because of its year-round sea-ice cover (Leistenschneider et al., 2021). In addition, the eastward-flowing Antarctic Circumpolar Current (ACC), extending to 2000-4000 m deep, is thought to broadly determine the abiotic and biotic conditions of the region, isolates the Weddell Sea and the surrounding open and ice-free Southern Ocean (Vedenin et al., 2020). Despite this, Antarctica and the Southern Ocean south of the ACC are under increasing threat from the consequences of anthropogenic activities (Waller et al., 2017) including plastic pollution (Perold et al., 2020; Tirelli et al., 2022). To date, microplastic pollution including fibres has been described in various Antarctic sample media, such as seawater from coastal areas off the Antarctic Peninsula (Absher et al., 2019; Lacerda et al., 2019), and the Weddell Sea (Leistenschneider et al., 2021), benthic sediment from the Ross Sea (Munari et al., 2017) and the Scotia Sea (Cunningham et al., 2020), and ice from the Eastern Antarctic Peninsula (Kelly et al., 2020). Microplastics have also been found in the digestive tract of Antarctic benthic invertebrates (Sfriso et al., 2020) and the scat of penguins (Bessa et al., 2019; Fragão et al., 2021).
Microplastics including fibres are known to travel in air masses and dust clouds from land to sea (Allen et al., 2019; Bergmann et al., 2019; Liu et al., 2019) and are believed to move large distances from highly populated areas to remote polar regions (Obbard, 2018). Once in the marine environment, microplastics can be transported in sea ice (Peeken et al., 2018) or by oceanic currents over long distances before sinking to the benthos through both physical and biological processes (Lusher et al., 2015; Kaiser et al., 2017). The horizontal and vertical transport of microplastics in marine environments varies depending on microplastic type (i.e., fibres vs fragments), polymer type, and degree of biofouling (Zhang, 2017; Szewc et al., 2021); however, the relative contribution of multiple oceanic and atmospheric processes (e.g., wind and currents) to the transportation of microplastics is understudied.
In this study, we investigate the presence of microplastic pollution in a range of Antarctic sample media including air, subsurface seawater, benthic sediment, and sea ice collected during the ‘Weddell Sea Expedition 2019’, to explore the transportation pathways of microplastics. Through a series of optical and chemical identification techniques, including Polarised Light Microscopy and Raman Spectrometry (Woodall et al., 2015a; Gwinnett and Miller, 2021), we categorised microplastic particles to determine the nature of the present microplastic pollution. We hypothesise that multiple transportation processes including atmospheric and oceanic vectors determine the presence of microplastics in the Antarctic. We also attempt to answer a series of questions in order to support our hypothesis, including (i) is there evidence of a major source of microplastic pollution within the Weddell Sea?; (ii) are microplastics transported by air and, if so, from where? (iii); are microplastics transported in seawater to the Antarctic by ocean currents?; and (iv) is the coastal and Antarctic deep sea a sink for microplastic pollution? As the shape profile of microplastics is known to affect their transport through the environment (Zhang, 2017), we expect to find fibres in the air and fragments/films in the water. Therefore, we also hypothesise that microplastic type (i.e., fibre/fragment/film) will differ among sample media.
2. Methodology
2.1. Sample collection and preparation
During the ‘Weddell Sea Expedition 2019’, a range of sample media including air, subsurface seawater, benthic sediment, and sea ice were collected from the Weddell Sea, Antarctica, onboard the R/V SA Agulhas II between December 2018 and March 2019 (Figure 1; Supplementary Table 1).
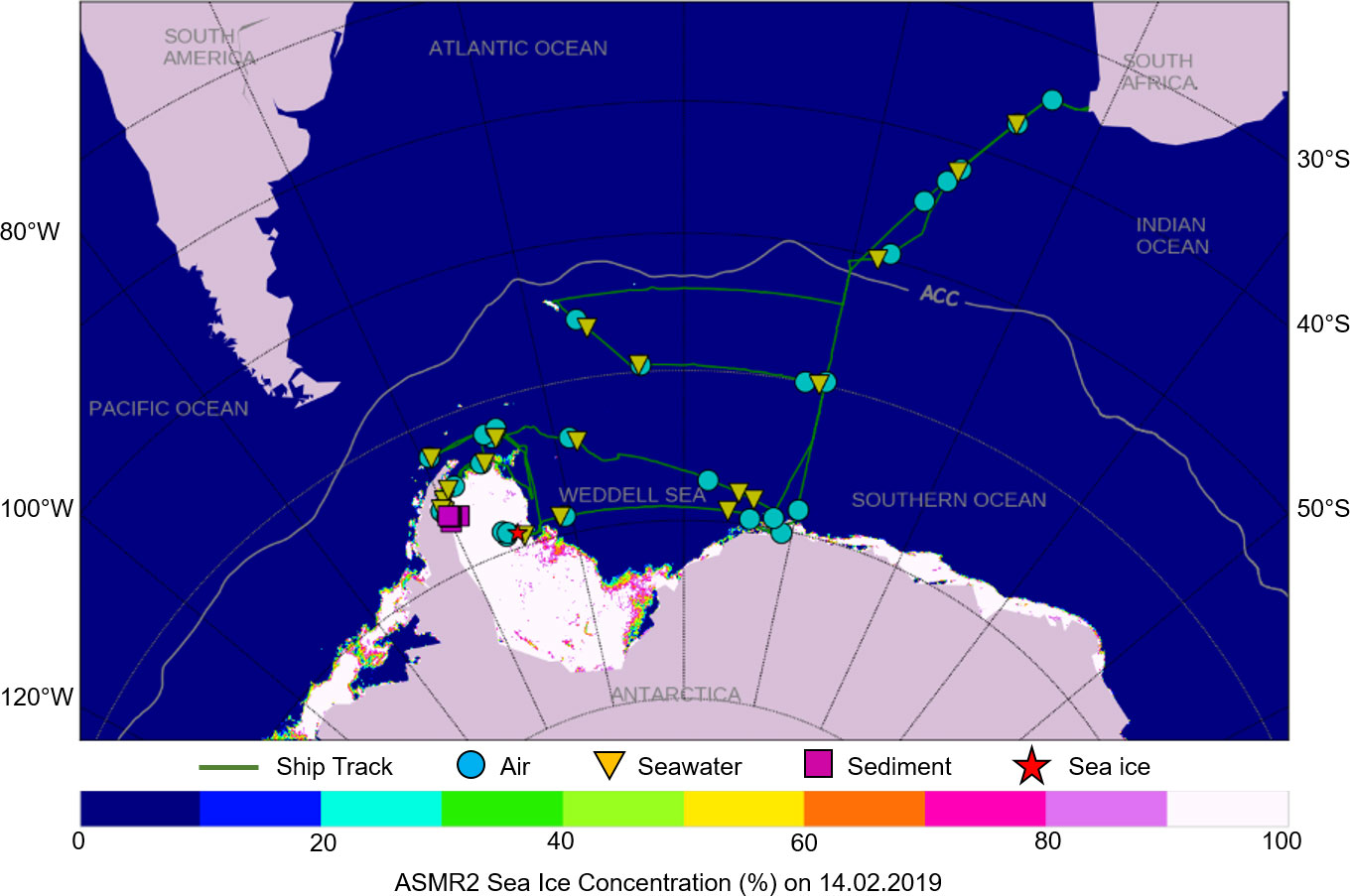
Figure 1 The ship track of the R/V SA Agulhas II and sampling locations for air, seawater, sediment, and sea ice during the ‘Weddell Sea Expedition 2019’.
Atmospheric air samples (n = 31) and air field blanks (n = 2) were collected above the vessel’s bridge (~20 m above sea level) using a high-volume air sampler (HV-AS; Tisch Environmental) (Supplementary Table 2). Air was pumped at an average flow rate of 0.82 m3/min through a five-stage cascade impactor (TE-235; Tisch Environmental) loaded with pre-combusted glass fibre filters (400 °C for 4 hr). Stage 1 filters were used in this study (TE-230-GF; Tisch Environmental; total surface area ~119 cm2), where the aerodynamical diameter of particles collected was > 7 μm. A sector sampling system (Campbell Scientific Africa) was installed with the HV-AS to avoid contamination from ship stack emissions.
The sector sampling system included a 03002 wind sentry anemometer and vane, manufactured by R.M Young, and a CR300 data logger located next to the HV-AS. The wind sentry has a range of 0 – 50 m/s ± 0.5 m/s wind speed and 360˚ mechanical and 352˚ electrical (8˚ open) azimuth with ± 5˚ accuracy. The signal wires from the wind sentry were wired into the data logger, which was connected to a relay switch that was wired to feed power to the HV-AS. The data logger was programmed in such a way that it would only switch the relay and power the pump when the wind was within a specified angle range, i.e., blowing from the front of the ship to avoid contamination by ship stack emissions.
An attempt was made to ensure that at least 24 hours of in-sector sampling had passed before the filters were removed from the cascade impactor. However, this was not always possible, on occasion the filters had to be removed early to avoid contamination due to unusual ship manoeuvres or stagnant conditions. Therefore, filter deployment times ranged between 22 and 88 hours.
The filters were removed from the cascade impactor inside a laminar flow cabinet (Air Science), placed in individual zip-sealed plastic bags, and stored at -20°C until analysis. For analysis, each sample filter and field blank were subsampled into three replicates. During the research voyage, air field blanks were collected by fitting the cascade impactor with a set of filters and walking the cascade impactor from the laboratory to the HV-AS in the same way as when atmospheric samples were deployed. The cascade impactor was placed into the HV-AS and then immediately removed without the HV-AS turning on, after which the filters were removed and stored in the same manner as the atmospheric samples.
Subsurface seawater samples (n = 20) were collected using the vessel’s underway system (Supplementary Table 3). At each sampling station, several 5 L HDPE bottles were filled with seawater and vacuum filtered through nylon mesh filters (Millipore NY20; 47mm; pore size 20 μm) until clogged. The mean (± SD) volume of water filtered was 13.15 ± 4.28 L. Filters were then folded in half using cleanedforceps and stored in 50 ml centrifuge tubes (see section 2.4 for a description of how all equipment was cleaned). Ashore at SAEON/CEFAS Commonwealth Litter Programme laboratory on the Ocean Sciences Campus of the Nelson Mandela University, the filters were rinsed with ultra-pure distilled water (Milli-Q Direct Water Purification System - Type 1 Ultra-pure) together with the contents of the centrifuge tubes through nylon mesh filter in a fume hood. The filters were then folded in half using forceps and sealed in aluminium foil envelopes until analysis.
Sediment cores (n = 6) were collected using a multicorer (Craib-type; 10 cm diameter) at depths ranging from 323 - 530 m (Supplementary Table 4). Once cut, the top two horizons of the core used for this study (0 - 1 cm and 1 - 2 cm) were double wrapped in aluminium foil and frozen inside cardboard boxes. In the lab ashore, the horizons were added to a glass beaker and homogenized by stirring with a metal spatula and adding filtered deionised water. Following this, the solution was then evenly divided into three representative subsamples by volume (n = 36 total). The subsamples were then wrapped in aluminium foil and dried at ~65 °. Each subsample was then weighed (~2-4 g) and transferred to a 50ml centrifuge tube with a high-density solution of zinc chloride (ZnCl 2; ~1.38 g/cm3) (Hidalgo-Ruz et al., 2012; Maes et al., 2017). To separate potential microplastics from the sediment, the centrifuge tubes were agitated for 1 minute and then centrifuged (Fisher Scientific©; AccuSpin 400) at 3900 rpm for 10 minutes. The supernatant was then removed via pipette and the process was repeated four times (Maes et al., 2017). The solution was then vacuum filtered onto cellulose filters (Cytiva Whatman™; Grade 1; 47 mm; pore size > 11 μm) and stored in a petri dish.
Multi-year sea-ice cores (n = 2) were sampled along the Weddell Sea ice floe edge using a Kovacs Mark II coring system (Supplementary Table 5). The ice core was placed in a sealed bag and stored horizontally at -21°C before being segmented along its length (core 1 - 128 cm; core 2 - 126 cm) into 5 cm slices at -10°C in the University of Cape Town Mobile Polar Laboratory. The slices were then left to melt in glass beakers covered with aluminium foil, after which the liquid was weighed and then vacuum filtered through cellulose filters (Cytiva Whatman™; Grade 1; 47 mm; pore size > 11 μm) in a fume hood. The filters were folded and stored in aluminium foil until analysis.
2.2. Laboratory processing
Each media required specific extraction and visual identification protocols to identify prospective microplastics (Figure 2).
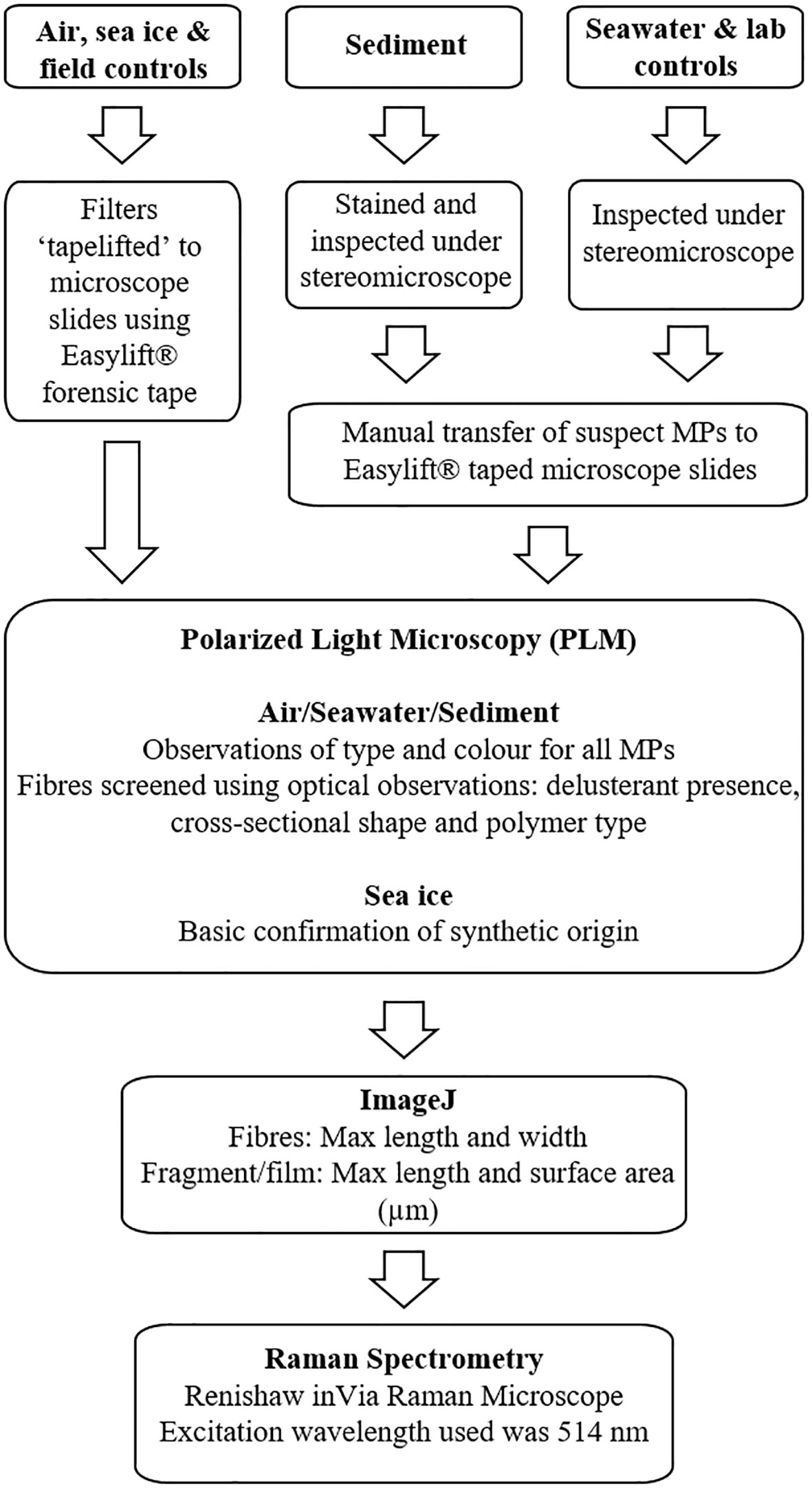
Figure 2 A workflow diagram illustrating the extraction of prospective microplastics from each sample medium and the subsequent optical observations and chemical identification processes.
The air and sea-ice filters were “tape lifted” using the Easylift® forensic tape method (Gwinnett et al., 2021). The filters were encased in a glass petri dish then checked under the stereomicroscope (Motic® SMZ-171), and any remaining prospective microplastics were transferred manually to the taped slide. Sediment filters were stained using a Nile Red staining protocol (Maes et al., 2017) and inspected for prospective microplastics under the stereomicroscope using ultraviolet (360 – 380 nm), royal blue (440 – 460 nm), and white light (normal light). The prospective microplastics were then manually transferred to a taped slide. Water filters were visually inspected under the stereomicroscope and again, prospective microplastics were manually transferred to a taped slide.
2.3. Optical observations and chemical identification
All prospective microplastics on the taped slides were screened with a Polarised Light Microscope (PLM; Brunel SP250P) to identify possible microplastics using morphological and optical properties (Gwinnett et al., 2021). The PLM analysis provided enough information to distinguish between natural and synthetic particles and to later determine the polymer types (Gwinnett et al., 2021). By assessing morphological and optical properties of microplastics using PLM, the polymer type can be easily identified even after extended periods of ageing, as properties such as delusterant remain fixed in the polymer matrix. The particles were then photographed under the PLM (Tucsen Photonics©; TrueChrome Metrics) and the longest length (fibres) or longest length and width (fragments/films) measured using ImageJ software v1.53. Only prospective microplastic fibres, films, and fragments within the 100 μm - 5 mm size range were analysed in this study. Any particulates whose polymer type were not confirmed during the PLM analysis, this includes all fragments and films, were analysed using Raman Spectrometry (Figure 2).
Spectra of prospective microplastics were collected using a Renishaw inVia Raman Microscope with a Leica microscope, and a ×20 objective lens was used for simultaneous illumination and data collection. To ensure the best possible spectra were generated, the following settings were used; 514 nm excitation wavelength with the laser intensity at 1%, 5%, 10%, and 50% and an integration time of 4 - 10 seconds (Gwinnett & Miller, 2021). Polymer identification was confirmed by comparing spectra using the Microtrace Forensic Fibre Reference at Staffordshire University, and previously published Raman spectra from the literature.
2.4. QA/QC procedures
Care was taken to reduce the likelihood of procedural contamination throughout the sample and analyses process on the R/V SA Agulhas II. Sample collection and processing during the expedition was conducted in a contained laboratory with minimal personnel. All sample containers and any other equipment that was in direct contact with the sample were cleaned prior to use with either filtered ethanol or deionised water. A sample of the expedition clothing and each plastic item present in the labs or at the sample collection locations were collected as controls and cross-referenced during the analysis. Atmospheric controls consisting of damp filters were used during sample collection and processing (e.g., cutting sediment cores and filtering seawater) and checked under a microscope for contamination after each procedure (see Supplementary Material). The laboratories were cleaned prior to sample processing, limited access to only the microplastic team and all researchers wore 100% cotton lab coats and nitrile gloves.
During the subsequent processing of samples at the on-land laboratories, care was taken at all times to only expose the samples to the atmosphere under strict conditions. All sources of air movement were removed when possible, including windows sealed, air conditioning units turned off, and fume hood used without an airflow. The laboratory where the microplastics were analysed was enclosed by a cotton curtain to further reduce the likelihood of contamination. In both sections of the laboratory, the floors were lint rolled, and all surfaces wiped clean with wet bamboo paper towels every day. All work on the samples was conducted in a cleaned fume hood, except when the prospective microplastics were manually extracted onto a taped slide on the stereomicroscope. All solutions used during the laboratory processes were filtered (Fisherbrand™; Grade 263; 47mm; pore size 0.7um), atmospheric controls (dampened filter paper) were implemented at all times, and researchers wore regularly cleaned nitrile gloves, and 100% cotton uniforms and lab coats. In addition, no synthetic clothing was worn during analysis at any time and hair was covered with 100% cotton covers. Samples of all plastic equipment used in the lab were also collected and cross-referenced during the analysis. In line with the QA/QC procedures from the R/V, atmospheric controls consisting of damp filters were used during processing and checked for contamination after each procedure. In the case where contamination was found, the PLM was used to determine if it was of natural or synthetic origin. All fibres found during processing at on-land laboratories were identified as natural and removed from the analysis.
2.5. Data analysis
We analysed three media (air, seawater, and sediment) for microplastic abundance and composition. Our analysis focused on the presence/absence of different categories of microplastics within these media. As each sample medium was collected and processed using different methods, it is not possible to compare microplastic abundance across the different media. Microplastic particles were grouped into categories based on specific characteristics; media (air, seawater, and sediment), type (i.e., fibre/fragment/film), colour, delusterant presence, cross-sectional shape (length/width dimensions), and polymer. The richness of microplastic categories can be found in Supplementary Table 1.
Separately, we determined the presence of microplastics across 12 and six horizons from ice core 1 and 2, respectively. We did not have the capacity to identify all of these microplastics to polymer type, but we did confirm synthetic origin using PLM (Supplementary Table 5).
To determine air mass origins, air mass back trajectories (AMBTs) were computed hourly for each sample. Only hours during which the HV-AS pump was operational for at least 45 min were used in the analyses. Given that the ship was travelling during sample collections, a different date, time, and starting location was used to compute each AMBT. An altitude of 20 m was chosen to represent the height at which samples were collected above sea-level. 72 h AMBTs were computed to account for the lifetime of plastics in the atmosphere using NOAA’s Hybrid Single - Particle Lagrangian Integrated Trajectory model (HYSPLIT v 4) with NCEP Global Data Assimilation System (GDAS) output, which can be accessed at: https://www.arl.noaa.gov/hysplit/(NOAA Air Resources Laboratory, Silver Spring, Maryland) (Stein et al., 2015; Rolph, 2016).
3. Results
A total of 82 microplastic particles were identified across the air (53), seawater (18), and sediment (11) samples. The majority of these microplastics were fibres (n = 80), followed by a single fragment and a piece of film. Microplastics consisted of four polymer types: polyester (49; 60%), nylon (12; 14%), polypropylene (8; 10%), and acrylic (4; 5%). In addition, a small number of microplastics within these media were identified as synthetic (9; 11%) during the PLM stage, but no specific polymer could be determined using Raman Spectrometry due to sample size and/or quality. Therefore, 89% of the microplastics from the air, seawater, and sediment were identified to polymer level, with the remaining 11% classified as synthetic. Microplastic particles had a mean (± SE) length of 780 ± 72 µm and a width of 18 ± 4 µm (Supplementary Table 1).
A total of 47 individual microplastic categories were identified in the air, seawater, and sediment samples. At least one microplastic particle was found in 13 of the 47 categories. Category richness of sample media decreased from the air to the seabed, with the highest diversity present in the air samples, followed by the seawater, and finally the sediment (Supplementary Table 1). The majority of these microplastic categories did not overlap between or among multiple media (42/47); however, four fibre categories were present in both the air and water samples, and another fibre category was found in all three sample media (category 27). Category 27, which consisted of colourless cylindrical polyester fibres with low amounts of delusterant, was identified as the second most common polymer type (Figure 3; Figure S1; Table 1). The most common fibre type found was category 18 (blue-grey cylindrical polyester with low amounts of delusterant); however, it was only identified in the air samples. Four of the identified microplastics categories were present in samples from the same media that were collected north and south of the ACC, including air and water. Fibres in categories 10, 23, and 27 were also present in samples taken from different media collected from both sides of the ACC (Supplementary Table 1).
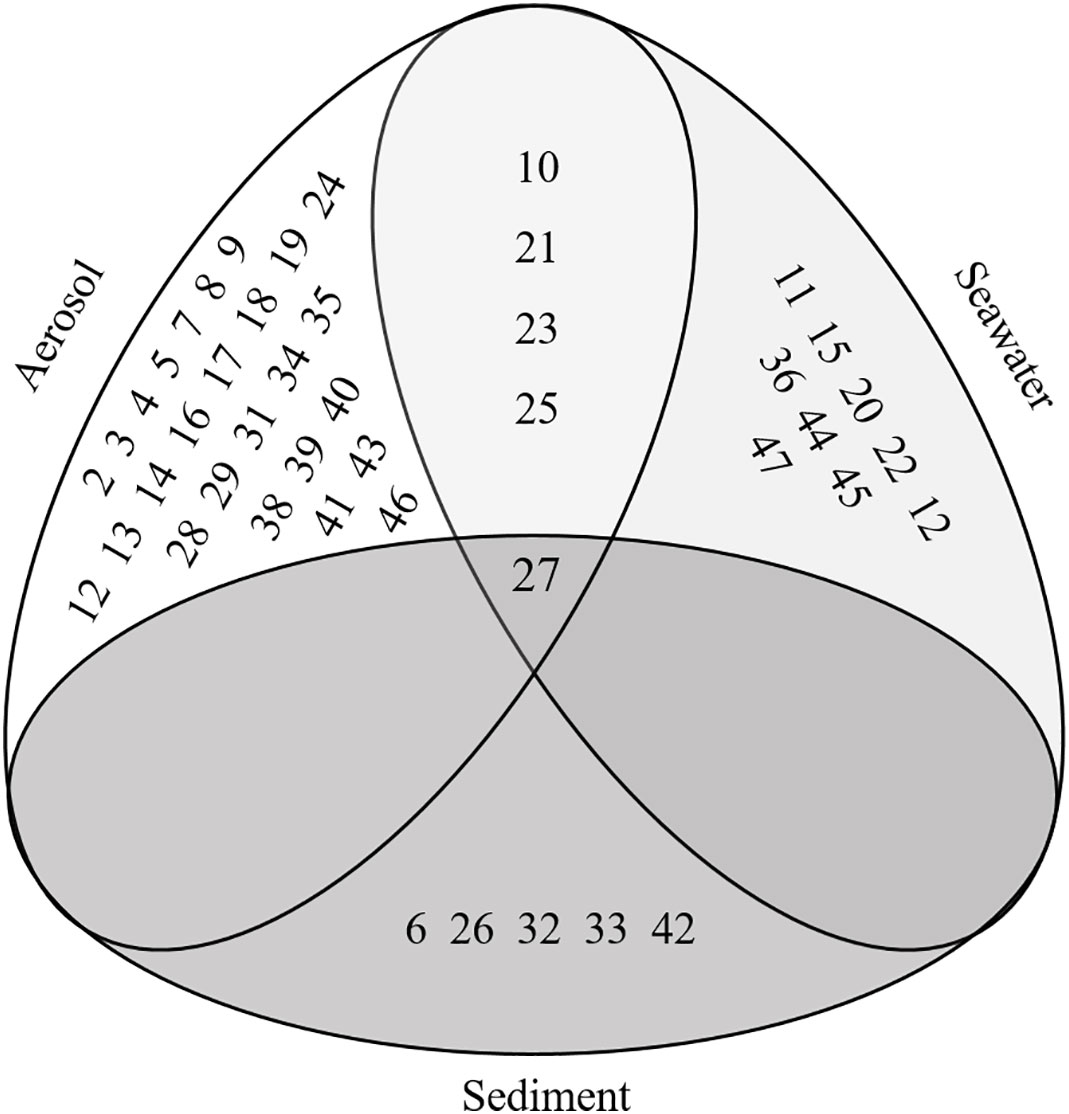
Figure 3 A Euler diagram illustrating the categories of microplastics identified in the air, seawater, and sediment samples, and those that overlap in two or more of the sample media. Full descriptions of the characteristics defining each category of microplastics are available in Supplementary Table 1.

Table 1 The characteristics and number of microplastic fibre categories present in two or more sample media.
Four fibres corresponding to categories 1, 10, 30, and 37 were identified as contamination from the air field blanks. As a result, all particles from categories 1, 30, and 37 were removed from the analysis (n = 4), and a number of particles from category 10, that were of similar width and optical characteristics to the contaminated fibres were also removed (n = 2). No further adjustments to the results were made, as no more microplastics were identified in the expedition or laboratory atmospheric controls, and all natural particles had already been removed from the analysis.
In sediment samples, microplastics were found in the surface horizon (0 - 1 cm) at all sample locations. All the surface horizons contained one fibre except core 979 that had three fibres from different categories. In the 1 - 2 cm horizon, just three cores contained a single fibre each (Supplementary Table 4).
Synthetic microplastics (fibres and fragments) were ubiquitous across all the sea-ice core horizons screened, from the bottom layer in the seawater to the top layer in contact with the snow and air.
The highest number of airborne microplastics were present closest to the eastern point of the Antarctic Peninsula (orange; Figure 4A). The 72-hour AMBT analyses shows that the high number of particles within these samples (5 - 7 per sample) derived from air masses that originated over South America and travelled southward towards the Weddell Sea (Figure 4B). Fewer microplastics (2 – 4 per sample), identified on the eastern point of the Peninsula and on the eastern side of the Weddell Sea (closest to the Larsen C Ice Shelf). These microplastics were observed in air masses that travelled over the Antarctica continent, at least in part, 72h prior to sampling. The fewest microplastics (0 - 1 per sample) were observed in air samples originating predominantly from over the centre of the Weddell Sea (Figure 4).
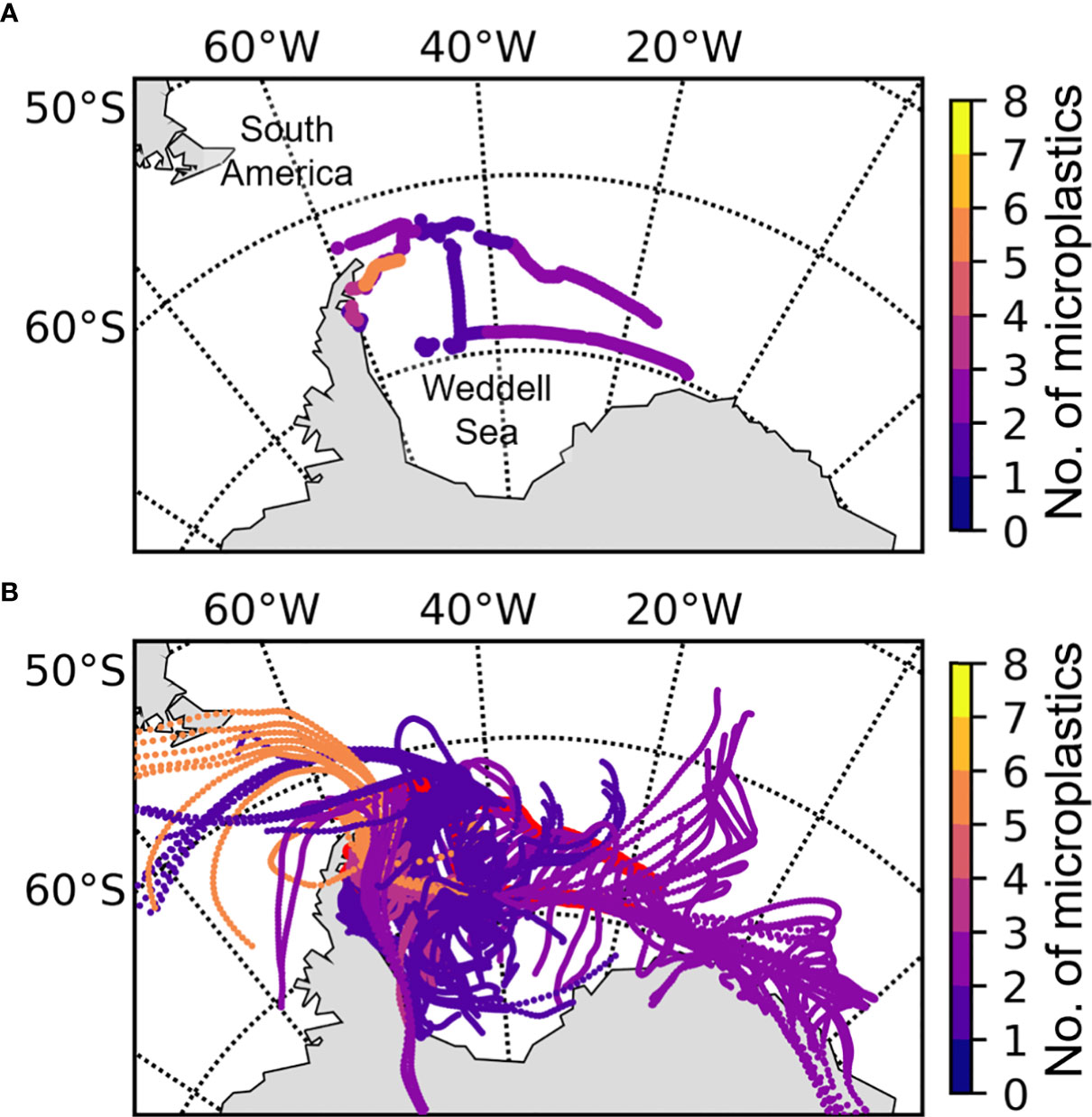
Figure 4 Cruise track across the northern edge of the Weddell Sea (A), overlayed by 72 hr AMBTs (B) computed for each hour of every air sample (the red line highlights the ship’s track). Both subplots are colour coded by the number of microplastics recovered from the air samples.
4. Discussion
To our knowledge, this is the first study that assesses the presence of microplastic pollution in multiple media in the Antarctic region, and with these data we reveal that global processes act differentially on the transportation of microplastics.
Microplastics are considered ubiquitous and therefore, as expected, were present in all of the sample media, despite the remote setting of our study sites and very few contamination point sources. All but two of the microplastics identified in the samples were fibres; consequently, we can reject our hypothesis that microplastic type differs among sample media. However, the large variety of fibres identified and the fact that fibre categories were shared among the media suggest that multiple diffuse sources and transportation pathways are contributing to the pollution that we observed.
We identified four fibre types that were present in both the air and subsurface seawater, and although this relationship was limited overall, it may indicate that atmospheric and oceanic processes influence the presence of pollutants in tandem. The coupling of these global processes was proposed in a recent study that quantified microplastic transport from sea to air in sea-splash (Allen et al., 2020). Although we assume that microplastics travel predominantly from air to sea (Weber et al., 2012; Obbard, 2018), it is possible that sea to air transport is also occurring in the remote Weddell Sea. For example spindrift during storms could aid the transport of microplastics to surrounding marine systems, ice sheets, or onto land (Allen et al., 2020). In addition, microplastic fibre category 27, which consisted of the second most common fibre type (colourless, cylindrical, polyester; Table 1) was found in the air, seawater, and sediment (Figure S1). Their presence in multiple sample media may demonstrate not only the transport of these pollutants to and among marine systems and the coupling of global processes, but the eventual fate of microplastics, which is deposition to the seabed (Woodall et al., 2014). However, as the overlapping of microplastic fibre categories between or among multiple media was scarce, it is also possible that certain fibre categories are preferentially sequestered in different environments by different global processes (Clark et al., 2016). To further investigate the role of multiple transportation processes in the distribution of microplastic pollution, we explore the following questions illustrated and outlined below (Figure 5).
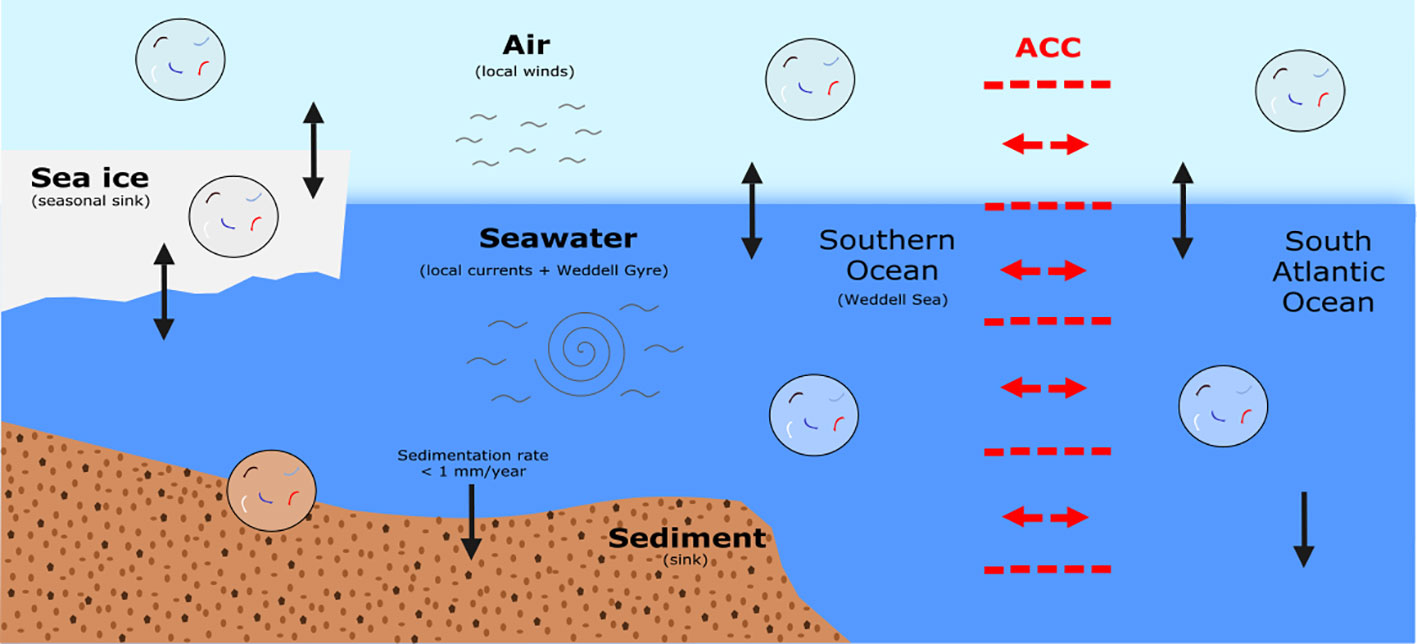
Figure 5 An infographic illustrating the multiple transportation processes that impact upon the presence and fate of microplastic fibre pollution in multiple media including air, seawater, sediment, and sea ice. In red, we show the ACC, and with black arrows the transfer of fibres between the different media. In grey, we show winds/currents and the cyclonic Weddell Gyre. The sedimentation rate value was taken from Dowdeswell et al. (2020).
4.1. Is there evidence for a major source of microplastic pollution in the Weddell Sea?
Although it is hard to identify the exact sources of the microplastic pollution from environmental samples (Auta et al., 2017), we are confident that the fibrous polyesters, which make up the majority of microplastics identified in this study, derive from synthetic fabric (O'Brien et al., 2020). Other possible sources include fishing gear, as fishing fleets within the neighbouring Scotia Sea are known to use polyester longlines (Cunningham et al., 2020). The small number of nylon fibres identified in our samples may originate from the degrading ropes and fishing gear that has been found in the Antarctic (do Sul et al., 2011) and Southern Ocean (Woodall et al., 2015b) in the past, as synthetic fishing gear is known to supply high numbers of fibres to the marine environment over time (Welden & Cowie, 2017). Additionally, point sources of microplastics to Antarctica and the surrounding areas, including research bases and vessels, have been identified in the past (Waller et al., 2017; Reed et al., 2018; Leistenschneider et al., 2021); however, an incremental analysis of microplastics emitted from the Rothera research station showed that none were detectable at a distance of more than 7 km from the sewage outflow (Reed et al., 2018). While these are all possible sources of microplastics to the Antarctic, it is unlikely they are the major contributor to the pollution we documented, given the remote location of the Weddell Sea. It is more likely that the pollution found in this study is derived from diffuse sources, as the nearest human activity to our study sites, aside from the research vessel, was ~200 km away.
4.2. Are microplastics transported to the Antarctic by air and, if so, from where?
As microplastics were present in our air samples, we conclude that these pollutants are being transported by diffuse sources, consistent with the findings of other recent studies (Obbard, 2018; Bergmann et al., 2019). However, our AMBT analyses not only demonstrate that microplastics are being transported by diffuse sources such as air masses or wind, but also strongly suggest that microplastics are being transported to the Antarctic from southern South America. Although the transport of microplastics between continents has been alluded to in the past (Allen et al., 2020), it was previously concluded that the ACC and the associated polar fronts would prevent microplastics from the surrounding continents and Southern Ocean reaching Antarctica (Waller et al., 2017; Yamazaki et al., 2021). We identified four microplastic categories in multiple media that were found on either side of the ACC, further suggesting that microplastics could enter Antarctica from the surrounding regions (do Sul et al., 2011). We also found that microplastic category 27, present in our air and seawater samples, as well as in the ‘sink’ media (sediment), may suggest that microplastics can cross the ACC and become trapped and remain in Antarctica (Isobe et al., 2017). Similarly, deep-sea cores from the Scotia Sea and East Antarctica were found to contain historical dust sediments from Patagonia (Weber et al., 2012), corroborating the notion that Antarctic sediment in the Weddell Sea may be a sink for particles transported by air from South America. In our air samples, we initially reported the presence of one acetate fibre (formerly category 41), however we decided to remove this from the results as acetate is classified as a natural fibre (Smyth et al., 2021). Our AMBT analyses further suggest that a high number of microplastics (7 - 8 per sample) travelled to the Weddell Sea from further east on the Antarctic continent (Figure S2). It is possible that this contamination came from the research facilities located within this area such as Halley Research Base (UK) or the South African National Antarctic Expedition IV. During this time, the ship was right next to the ice shelf and was moving back and forth through the ice as researchers walked above the bridge to see the view (Pers. Obs.). Additional sources at this location could include the use of helicopters, a field excursion undertaken by the researchers, or the ship stack may have contaminated the sample because of the back and forth movement.
4.3. Are microplastics being transported to the Antarctic in seawater by ocean currents?
Our results show that microplastic particles are indeed present in the subsurface waters of the Antarctic, despite their absence in Antarctic surface waters (Kuklinski et al., 2019). Previous studies have shown natural origin fibres to dominate seawater samples as opposed to microplastics (Suaria et al., 2020), therefore our PLM methodology of screening prospective microplastics for synthetic origin has shown to be an efficient method for removing natural fibres at an early stage of the analysis (Gwinnett & Miller, 2021). Microplastic category 10 was identified in the water samples on both sides of the ACC, suggesting that microplastics may be transported into the Antarctic via ocean currents (Suaria et al., 2020). It is thought that the high frequency variability in the ACC, evident as eddies, jets, and filaments, can provide pathways for debris to cross the fronts southward into the Antarctic (Waller et al., 2017). ACC-derived eddies are known to provide a mechanism for floating kelp to cross the ACC (Fraser et al., 2016) and they could similarly be facilitating the transport of plastics into the Antarctic, particularly as kelp is a documented bio-transporter of plastic pollution (Turner & Williams, 2021). Although the transport of plastic debris into the Antarctic through water pathways is yet to be documented, the aforementioned studies highlight that the ACC is not impenetrable. In addition, our results did not show an increase in the number of microplastics in water samples collected north of the ACC, which one may expect if there was a physical barrier stopping microplastics from entering the Antarctic. The microplastics that cross into the Antarctic will eventually lose buoyancy and be transported vertically through the water column to the seabed (Woodall et al., 2014; Figure 5); thereby, acting as a sink for most ocean-bound and transported fibres. Also, the presence of synthetic fibres throughout all the layers of the ice cores is an indication that microplastics are present and being trapped during the creation of the sea-ice layer every year. The accumulation of microplastics in the sea ice prior to their release during the summer season when the ice melts could be thought of as a seasonal sink for pollution before they are transported to the seabed or to a new area of the world (Peeken et al., 2018; Mountford & Morales Maqueda, 2021).
4.4. Is the coastal and Antarctic deep sea a sink for microplastic pollution?
Our air and subsurface seawater samples demonstrate the transportation pathways for microplastic pollution, but what happens subsequently? In our sediment samples, we found the same fibre category identified in the mobile media types (air, seawater) and fibres were also documented in all horizons of the sea-ice cores. Ocean sedimentation is suggested to be <1 mm/year in the Weddell Sea (Dowdeswell et al., 2020) but can fallto between 0.011 and 0.02 mm/year at depths of over 1000 m in areas such as Kapp Norvegia (Grobe, 1986).The discovery of microplastics in the 1 - 2 cm horizon may suggest that the sedimentation rate in our collection area, where the seafloor is <500 m deep, is higher than that documented in Kapp Norvegia and closer to the suggested rate by Dowdeswell et al. (2020) or that bioturbation is occurring (Näkki et al., 2017), with the latter idea consistent with the observed high abundance of benthic organisms mixing the layers (Pers. Obs.). It is also possible that the surface layer was more unconsolidated than the deeper layers, or a slight layer mixture occurred during the coring or the wet processing of the sediment, which can result in a loss of the core surface material into the lower layers (Pudsey, 2000). The presence of microplastics in sea ice indicates that pollution in the surrounding environment gets trapped when the ice forms (e.g., microplastics in air and/or precipitation may be deposited on the forming ice from above, with microplastics in seawaterand/or marine plankton possibly integrated into the forming ice from below). That sea ice, which is mobile and can travel vast distances over a single season (Vichi et al., 2019), apparently traps and concentrates microplastics, which suggests that such pollution might have reached other regions deep in the Antarctic that have yet to be explored. The sea ice analysed here is considered a seasonal (at times even a multi-seasonal) sink for microplastics, similar to the East Antarctic (Kelly et al., 2020), but over time, the pollution may reach the permanent ice shelves on the Antarctic continent where it can become trapped indefinitely.
We are confident that we avoided self-contamination of our air (with the exception of one filter which was removed from this study), seawater, and sediment media, although it is common for researchers to contaminate their own samples (Gwinnett and Miller, 2021). This could be especially problematic in polar microplastics studies where warm synthetic clothing is needed for field sampling. As a very high abundance of microplastics was found in our ice-core horizons (three times that of all other media combined), we cannot rule out the possibility of self-contamination. Therefore, a full analysis of the ice cores collected for this study was not completed. However, Antarctic sea ice is known to concentrate nutrients and biological particles during its formation (e.g., Janssens et al., 2016), such that it may do the same to microplastics, especially given that they could be concentrated from both the atmosphere above the ice and the seawater below the ice.
In conclusion, our study provides preliminary evidence that multiple transportation processes affect the presence and distribution of microplastic pollution in the Antarctic environment. Indeed, preferential sequestration of certain pollutants by differing global processes may also be possible. By examining multiple media types, we show that microplastic pollution is entering the Antarctic in air masses travelling from the South American continent, and also provide evidence for microplastic transport via seawater from the surrounding Southern Ocean. These results highlight that the ACC is not the physical barrier for microplastic pollution that it has previously been concluded to be. Additionally, as pollution was found in our sediment and sea ice samples, we suggest that microplastics may become trapped in the Antarctic once they have crossed the ACC. By examining a range of media from the same system, we propose a series of hypotheses that cover the transportation and accumulation processes of microplastic pollutants that could be applied to other systems in future studies. Air and seawater are vectors for plastic pollution to travel across the globe from continent to continent. Given this transboundary transportation, it is imperative that we reduce our reliance on plastic products at a global scale. The recent resolution at the United Nations Environment Assembly to establish an Intergovernmental Negotiating Committee for a plastic pollution treaty is therefore a critically important step for the conservation of marine systems, and the results shown here from a remote, arguably near-pristine system, further highlight the need for a global response to the plastic pollution crisis.
Data availability statement
The original contributions presented in the study are included in the article/Supplementary Material. Further inquiries can be directed to the corresponding author.
Author contributions
LW conceptualised the study. NS, EC, AO, and CG extracted and identified the microplastic pollution. KA, RA, JMB, TB, SF, and LW collected sample media in the field. All authors contributed to and approved the manuscript, which was led by EC, NS, and LW.
Acknowledgments
We thank Captain Knowledge Bengu, Captain Freddie Ligthelm, the crew of the R/V SA Agulhas II, and the South African Department of Forestry, Fisheries and the Environment, as well as the Weddell Sea Expedition 2019. This research was supported by the Flotilla Foundation through a grant to LW, TB, and SF, the South African National Antarctic Program through grants 117035 and 129232 to SF and 110732 to KA, the South African National Research Foundation through Competitive Support for Rated Researchers grant 111716 to KA, and postgraduate fellowships to JB and RA, the University of Cape Town through Vice-Chancellor Future Leaders 2030 awards to SF and KA and a University Research Council Launching Grant to KA, the African Academy of Sciences/Royal Society through a FLAIR Fellowship to SF, and the Nekton Foundation. The authors also acknowledge the South African Department of Science and Innovation’s Biogeochemistry Research Infrastructure Platform (BIOGRIP) and Shallow Marine and Coastal Research Infrastructure (SMCRI), as well as the joint SAEON/CEFAS Commonwealth Litter Programme (CLiP) laboratory on the Ocean Sciences Campus of the Nelson Mandela University. Finally, we thank Annie Bekker, Mark Hambrock, Siobhan Johnson, Lu Liangliang, James-John Matthee, Wolfgang Rack, Mishka Rawatlal, and Christof van Zijl for technical support during the expedition and in the laboratory, and Cashifa Karriem and Hazel Leighton-Little for administrative assistance. The authors also thank the technical team at Staffordshire University for supporting the analysis of the microplastic samples and Nobya Sakai and Joseph Taylor from Helio Display Materials for their technical support and equipment. This is Nekton publication 32.
Conflict of interest
The intellectual property that underpins EasyliftⓇ is owned by Staffordshire University where this work was completed and one of the authors of this paper CG are named as the inventors in the relevant patents. One of the authors CG is a professor at Staffordshire University and one AO holds a funded PhD research position paid for by that University. Staffordshire University is interested in licencing the production and sale of EasyliftⓇ .
The remaining authors declare that the research was conducted in the absence of any commercial or financial relationships that could be construed as a potential conflict of interest.
Outside the support listed in the Acknowledgements, this research has received no external funding.
Publisher’s note
All claims expressed in this article are solely those of the authors and do not necessarily represent those of their affiliated organizations, or those of the publisher, the editors and the reviewers. Any product that may be evaluated in this article, or claim that may be made by its manufacturer, is not guaranteed or endorsed by the publisher.
Supplementary material
The Supplementary Material for this article can be found online at: https://www.frontiersin.org/articles/10.3389/fmars.2022.1056081/full#supplementary-material
References
Absher T. M., Ferreira S. L., Kern Y., Ferreira A. L., Christo S. W., Ando R. A. (2019). Incidence and identification of microfibers in ocean waters in admiralty bay, Antarctica. Environ. Sci. pollut. Res. 26, 292–298. doi: 10.1007/s11356-018-3509-6
Allen S., Allen D., Moss K., Le Roux G., Phoenix V. R., Sonke J. E. (2020). Examination of the ocean as a source for atmospheric microplastics. PloS One 15, e0232746. doi: 10.1371/journal.pone.0232746
Allen S., Allen D., Phoenix V. R., Le Roux G., Jiménez P. D., Simonneau A., et al. (2019). Atmospheric transport and deposition of microplastics in a remote mountain catchment. Nat. Geosci. 12, 339–344. doi: 10.1038/s41561-019-0335-5
Auta H. S., Emenike C. U., Fauziah S. H. (2017). Distribution and importance of microplastics in the marine environment: a review of the sources, fate, effects, and potential solutions. Environ. Int. 102, 165–176. doi: 10.1016/j.envint.2017.02.013
Beaumont N. J., Aanesen M., Austen M. C., Börger T., Clark J. R., Cole M., et al. (2019). Global ecological, social and economic impacts of marine plastic. Mar. pollut. Bull. 142, 189–195. doi: 10.1016/j.marpolbul.2019.03.022
Bergmann M., Mützel S., Primpke S., Tekman M. B., Trachsel J., Gerdts G. (2019). White and wonderful? microplastics prevail in snow from the Alps to the Arctic. Sci. Adv. 5, eaax1157. doi: 10.1126/sciadv.aax1157
Bessa F., Ratcliffe N., Otero V., Sobral P., Marques J. C., Waluda C. M., et al. (2019). Microplastics in gentoo penguins from the Antarctic region. Sci. Rep. 9, 1–7. doi: 10.1038/s41598-019-50621-2
Clark J. R., Cole M., Lindeque P. K., Fileman E., Blackford J., Lewis C., et al. (2016). Marine microplastic debris: a targeted plan for understanding and quantifying interactions with marine life. Front. Ecol. Environ. 14, 317–324. doi: 10.1002/fee.1297
Cunningham E. M., Ehlers S. M., Dick J. T., Sigwart J. D., Linse K., Dick J. J., et al. (2020). High abundances of microplastic pollution in deep-sea sediments: evidence from Antarctica and the southern ocean. Environ. Sci. Technol. 54, 13661–13671. doi: 10.1021/acs.est.0c03441
Curren E., Leong S. C. Y. (2019). Profiles of bacterial assemblages from microplastics of tropical coastal environments. Sci. Totl. Environ. 655, 313–320. doi: 10.1016/j.scitotenv.2018.11.250
do Sul J. A. I., Barnes D. K., Costa M. F., Convey P., Costa E. S., Campos L. S. (2011). Plastics in the Antarctic environment: are we looking only at the tip of the iceberg? Oecol. 15, 150–170. doi: 10.4257/oeco.2011.1501.11
Dowdeswell J. A., Batchelor C. L., Dorschel B., Benham T. J., Christie F. D., Dowdeswell E. K., et al. (2020). Sea-Floor and sea-ice conditions in the western weddell Sea, Antarctica, around the wreck of sir Ernest shackleton's endurance. Antarct. Sci. 32, 301–313. doi: 10.1017/S0954102020000103
Fragão J., Bessa F., Otero V., Barbosa A., Sobral P., Waluda C. M., et al. (2021). Microplastics and other anthropogenic particles in Antarctica: Using penguins as biological samplers. Sci. Totl. Environ. 788, 147698. doi: 10.1016/j.scitotenv.2021.147698
Fraser C., Kay G. M., Plessis M. D., Ryan P. G. (2016). Breaking down the barrier: dispersal across the Antarctic polar front. Ecography 40, 235–237. doi: 10.1111/ecog.02449
Grobe H. (1986). Sedimentation processes on the Antarctic continental margin at kapp norvegia during the late pleistocene. Geol. Rundsch. 75, 97–104. doi: 10.1007/BF01770181
Gwinnett C., Miller R. Z. (2021). Are we contaminating our samples? a preliminary study to investigate procedural contamination during field sampling and processing for microplastic and anthropogenic microparticles. Mar. pollut. Bull. 173, 113095. doi: 10.1016/j.marpolbul.2021.113095
Gwinnett C. M., Osborne A. O., Jackson A. R. (2021). The application of tape lifting for microplastic pollution monitoring. Environ. Adv. 5, 100066. doi: 10.1016/j.envadv.2021.100066
Hidalgo-Ruz V., Gutow L., Thompson R. C., Thiel M. (2012). Microplastics in the marine environment: a review of the methods used for identification and quantification. Environ. Sci. Technol. 46, 3060–3075. doi: 10.1021/es2031505
Isobe A., Uchiyama-Matsumoto K., Uchida K., Tokai T. (2017). Microplastics in the southern ocean. Mar. pollut. Bull. 114, 623–626. doi: 10.1016/j.marpolbul.2016.09.037
Janssens J., Meiners K. M., Tison J. L., Dieckmann G., Delille B., Lannuzel D., et al. (2016). Incorporation of iron and organic matter into young Antarctic sea ice during its initial growth stagesIncorporation of iron and organic matter into sea ice. Elementa 4, 000123. doi: 10.12952/journal.elementa.000123
Jones J. S., Porter A., Muñoz-Pérez J. P., Alarcón-Ruales D., Galloway T. S., Godley B. J., et al. (2021). Plastic contamination of a Galapagos island (Ecuador) and the relative risks to native marine species. Sci. Totl. Environ. 789, 147704. doi: 10.1016/j.scitotenv.2021.147704
Kaiser D., Kowalski N., Waniek J. J. (2017). Effects of biofouling on the sinking behavior of microplastics. Environ. Res. Lett. 12, 124003. doi: 10.1088/1748-9326/aa8e8b
Kelly A., Lannuzel D., Rodemann T., Meiners K. M., Auman H. J. (2020). Microplastic contamination in east Antarctic sea ice. Mar. pollut. Bull. 154, 111130. doi: 10.1016/j.marpolbul.2020.111130
Kuklinski P., Wicikowski L., Koper M., Grala T., Leniec-Koper H., Barasiński M., et al. (2019). Offshore surface waters of Antarctica are free of microplastics, as revealed by a circum-Antarctic study. Mar. pollut. Bull. 149, 110573. doi: 10.1016/j.marpolbul.2019.110573
Lacerda A. L. D. F., Rodrigues L. D. S., Van Sebille E., Rodrigues F. L., Ribeiro L., Secchi E. R., et al. (2019). Plastics in sea surface waters around the Antarctic peninsula. Sci. Rep. 9, 1–12. doi: 10.1038/s41598-019-40311-4
Landon-Lane M. (2018). Corporate social responsibility in marine plastic debris governance. Mar. pollut. Bull. 127, 310–319. doi: 10.1016/j.marpolbul.2017.11.054
Leistenschneider C., Burkhardt-Holm P., Mani T., Primpke S., Taubner H., Gerdts G. (2021). Microplastics in the weddell Sea (Antarctica): A forensic approach for discrimination between environmental and vessel-induced microplastics. Environ. Sci. Technol. 23, 15900–15911. doi: 10.1021/acs.est.1c05207
Liu K., Wu T., Wang X., Song Z., Zong C., Wei N., et al. (2019). Consistent transport of terrestrial microplastics to the ocean through atmosphere. Environ. Sci. Technol. 53, 10612–10619. doi: 10.1021/acs.est.9b03427
Lusher A. L., Tirelli V., O’Connor I., Officer R. (2015). Microplastics in Arctic polar waters: the first reported values of particles in surface and sub-surface samples. Sci. Rep. 5, 1–9. doi: 10.1038/srep14947
Maes T., Jessop R., Wellner N., Haupt K., Mayes A. G. (2017). A rapid-screening approach to detect and quantify microplastics based on fluorescent tagging with Nile red. Sci. Rep. 7, 1–10. doi: 10.1038/srep44501
Materić D., Kjær H. A., Vallelonga P., Tison J. L., Röckmann T., Holzinger R. (2022). Nanoplastics measurements in northern and southern polar ice. Environ. Res. 208, 112741. doi: 10.1016/j.envres.2022.112741
Mishra A. K., Singh J., Mishra P. P. (2021). Microplastics in polar regions: An early warning to the world's pristine ecosystem. Sci. Totl. Environ. 784, 147149. doi: 10.1016/j.scitotenv.2021.147149
Mountford A. S., Morales Maqueda M. A. (2021). Modeling the accumulation and transport of microplastics by Sea ice. J. Geophys. Res. Oceans. 126, e2020JC016826. doi: 10.1029/2020JC016826
Munari C., Infantini V., Scoponi M., Rastelli E., Corinaldesi C., Mistri M. (2017). Microplastics in the sediments of terra nova bay (ross sea, Antarctica). Mar. pollut. Bull. 122, 161–165. doi: 10.1016/j.marpolbul.2017.06.039
Näkki P., Setälä O., Lehtiniemi M. (2017). Bioturbation transports secondary microplastics to deeper layers in soft marine sediments of the northern Baltic Sea. Mar. pollut. Bull. 119, 255–261. doi: 10.1016/j.marpolbul.2017.03.065
Napper I. E., Davies B. F., Clifford H., Elvin S., Koldewey H. J., Mayewski P. A., et al. (2020). Reaching new heights in plastic pollution–preliminary findings of microplastics on mount Everest. One Earth 3, 621–630. doi: 10.1016/j.oneear.2020.10.020
Napper I. E., Parker-Jurd F. N. F., Wright S. L., Thompson R. C. (2022). Examining the release of synthetic microfibres to the environment via two major pathways: Atmospheric deposition and treated wastewater effluent. Sci. Totl. Environ. 159317, 159317. doi: 10.1016/j.scitotenv.2022.159317
O'Brien S., Okoffo E. D., O'Brien J. W., Ribeiro F., Wang X., Wright S. L., et al. (2020). Airborne emissions of microplastic fibres from domestic laundry dryers. Sci. Totl. Environ. 747, 141175. doi: 10.1016/j.scitotenv.2020.141175
Obbard R. W. (2018). Microplastics in polar regions: the role of long range transport. Curr. Opin. Environ. Sci. Health 1, 24–29. doi: 10.1016/j.coesh.2017.10.004
O’Hara C. C., Frazier M., Halpern B. S. (2021). At-Risk marine biodiversity faces extensive, expanding, and intensifying human impacts. Sci 372, 84–87. doi: 10.1126/science.abe6731
Peeken I., Primpke S., Beyer B., Gütermann J., Katlein C., Krumpen T., et al. (2018). Arctic Sea ice is an important temporal sink and means of transport for microplastic. Nat. Commun. 9, 1–12. doi: 10.1038/s41467-018-03825-5
Perold V., Schoombie S., Ryan P. G. (2020). Decadal changes in plastic litter regurgitated by albatrosses and giant petrels at sub-Antarctic Marion island. Mar. pollut. Bull. 159, 111471. doi: 10.1016/j.marpolbul.2020.111471
Pudsey C. J. (2000). Sedimentation on the continental rise west of the Antarctic peninsula over the last three glacial cycles. Mar. Geol. 167, 313–338. doi: 10.1016/S0025-3227(00)00039-6
Reed S., Clark M., Thompson R., Hughes K. A. (2018). Microplastics in marine sediments near rothera research station, Antarctica. Mar. pollut. Bull. 133, 460–463. doi: 10.1016/j.marpolbul.2018.05.068
Rolph G. D. (2016) Real-time environmental applications and display system (READY) website (NOAA Air Resources Laboratory). Available at: https://www.ready.noaa.gov/index.php (Accessed 12 January 2022).
Sfriso A. A., Tomio Y., Rosso B., Gambaro A., Sfriso A., Corami F., et al. (2020). Microplastic accumulation in benthic invertebrates in Terra Nova bay (Ross Sea, Antarctica). Environ. Int. 137, 105587. doi: 10.1016/j.envint.2020.105587
Siegfried M., Koelmans A. A., Besseling E., Kroeze C. (2017). Export of microplastics from land to sea. a modelling approach. Water. Res. 127, 249–257. doi: 10.1016/j.watres.2017.10.011
Smyth K., Drake J., Li Y., Rochman C., Van Seters T., Passeport E. (2021). Bioretention cells remove microplastics from urban stormwater. Wat. Res. 191, 116785. doi: 10.1016/j.watres.2020.116785
Stein A. F., Draxler R. R., Rolph G. D., Stunder B. J. B., Cohen M. D., Ngan F. (2015). NOAA’s HYSPLIT atmospheric transport and dispersion modelling system. Bull. Amer. Meteor. Soc 96, 2059–2077. doi: 10.1175/BAMS-D-14-00110.1
Suaria G., Achtypi A., Perold V., Lee J. R., Pierucci A., Bornman T. G., et al. (2020). Microfibers in oceanic surface waters: A global characterization. Sci. Adv. 6, eaay8493. doi: 10.4257/oeco.2011.1501.11
Szewc K., Graca B., Dołęga A. (2021). Atmospheric deposition of microplastics in the coastal zone: Characteristics and relationship with meteorological factors. Sci. Totl. Environ. 761, 143272. doi: 10.1016/j.scitotenv.2020.143272
Tirelli V., Suaria G., Lusher A. L. (2022). “Microplastics in polar samples,” in Handbook of microplastics in the environment (Springer Nature Switzerland: Cham: Springer International Publishing), 281–322. doi: 10.1007/978-3-030-39041-9_4
Turner A., Williams T. (2021). The role of kelp in the transport and fate of negatively buoyant marine plastic. J. Sea Res. 175, 102087. doi: 10.1016/j.seares.2021.102087
Vedenin A., Kulagin D., Musaeva E., Vereshchaka A. (2020). The southern polar front as a key to mesoplankton migratory behavior. Sci. Rep. 10, 1–14. doi: 10.1038/s41598-020-70720-9
Vichi M., Eayrs C., Alberello A., Bekker A., Bennetts L., Holland D., et al. (2019). Effects of an explosive polar cyclone crossing the Antarctic marginal ice zone. Geophys. Res. Lett. 46, 5948–5958. doi: 10.1029/2019GL082457
Waller C. L., Griffiths H. J., Waluda C. M., Thorpe S. E., Loaiza I., Moreno B., et al. (2017). Microplastics in the Antarctic marine system: an emerging area of research. Sci. Totl. Environ. 598, 220–227. doi: 10.1016/j.scitotenv.2017.03.283
Weber M. E., Kuhn G., Sprenk D., Rolf C., Ohlwein C., Ricken W. (2012). Dust transport from Patagonia to Antarctica–a new stratigraphic approach from the Scotia Sea and its implications for the last glacial cycle. Quat. Sci. Rev. 36, 177–188. doi: 10.1016/j.quascirev.2012.01.016
Welden N. A., Cowie P. R. (2017). Degradation of common polymer ropes in a sublittoral marine environment. Mar. pollut. Bull. 118, 248–253. doi: 10.1016/j.marpolbul.2017.02.072
Woodall L. C., Gwinnett C., Packer M., Thompson R. C., Robinson L. F., Paterson G. L. (2015a). Using a forensic science approach to minimize environmental contamination and to identify microfibres in marine sediments. Mar. pollut. Bull. 95, 40–46. doi: 10.1016/j.marpolbul.2015.04.044
Woodall L. C., Robinson L. F., Rogers A. D., Narayanaswamy B. E., Paterson G. L. (2015b). Deep-sea litter: a comparison of seamounts, banks and a ridge in the Atlantic and Indian oceans reveals both environmental and anthropogenic factors impact accumulation and composition. Front. Mar. Sci. 2. doi: 10.3389/fmars.2015.00003
Woodall L. C., Sanchez-Vidal A., Canals M., Paterson G. L., Coppock R., Sleight V., et al. (2014). The deep sea is a major sink for microplastic debris. R. Soc Open Sci. 1, 140317. doi: 10.1098/rsos.140317
Yamazaki K., Aoki S., Katsumata K., Hirano D., Nakayama Y. (2021). Multidecadal poleward shift of the southern boundary of the Antarctic circumpolar current off East Antarctica. Sci. Adv. 7, eabf8755. doi: 10.1126/sciadv.abf8755
Keywords: AMBT, Antarctica, atmospheric, fibres, forensics, oceanic, PLM, pollution
Citation: Cunningham EM, Rico Seijo N, Altieri KE, Audh RR, Burger JM, Bornman TG, Fawcett S, Gwinnett CMB, Osborne AO and Woodall LC (2022) The transport and fate of microplastic fibres in the Antarctic: The role of multiple global processes. Front. Mar. Sci. 9:1056081. doi: 10.3389/fmars.2022.1056081
Received: 28 September 2022; Accepted: 31 October 2022;
Published: 21 November 2022.
Edited by:
Fabiana Corami, Department of Earth System Sciences and Technologies for the Environment, National Research Council (CNR), ItalyReviewed by:
Giuseppe Suaria, Department of Earth System Sciences and Technologies for the Environment, National Research Council (CNR), ItalyChristina Zeri, Hellenic Centre for Marine Research (HCMR), Greece
Copyright © 2022 Cunningham, Rico Seijo, Altieri, Audh, Burger, Bornman, Fawcett, Gwinnett, Osborne and Woodall. This is an open-access article distributed under the terms of the Creative Commons Attribution License (CC BY). The use, distribution or reproduction in other forums is permitted, provided the original author(s) and the copyright owner(s) are credited and that the original publication in this journal is cited, in accordance with accepted academic practice. No use, distribution or reproduction is permitted which does not comply with these terms.
*Correspondence: Eoghan M. Cunningham, ZW9naGFuLmN1bm5pbmdoYW1AYmlvbG9neS5veC5hYy51aw==
†These authors share first authorship