- 1Faculty of Agriculture, Institute of Animal Sciences, University of Belgrade, Belgrade, Serbia
- 2Department of Microscopy, Laboratory of Histology and Embryology, ICBAS-School of Medicine and Biomedical Sciences, University of Porto, Porto, Portugal
- 3Faculty of Fisheries and Protection of Waters, South Bohemian Research Center of Aquaculture and Biodiversity of Hydrocenoses, University of South Bohemia in České Budějovice, České Budějovice, Czechia
Aquaponics is a food production system that combines aquaculture with hydroponics. The simultaneous existence of fish, beneficial bacteria and plants in the same water loop predisposes the fish and bacteria to a possible detrimental effect of plant protection products. Additionally, there is an inadequate exploration of scientific studies on the impact of pesticides on fish and bacteria in aquaponics systems. This study investigated the effects of three commercial insecticides based on the following active ingredients: pyrethrum, azadirachtin, and spinosad, on aquaponics systems. Due to ethical concerns in animal testing, applying insecticides directly to aquaponics setup was impossible. Therefore, three separate experiments were conducted: (1) Pesticide runoff rate – in which insecticides were applied to basil (Ocimum basilicum) plants grown in two hydroponic systems: media bed and floating raft. The concentrations of applied insecticides were measured in the water of nutrient solutions of the hydroponics after 1, 3, 6, 12, 24, 36, 48, 72 and 96h to establish a pattern of degradation of insecticides. The result from this experiment showed that pyrethrum and spinosad were detected in unquantifiable concentrations in the nutrient solutions. Hence, further experiments were conducted only with azadirachtin. In a biofilter trial (2) – azadirachtin, at three concentrations (1.5 µg L-1; 7.5 µg L-1; and 15 µg L-1), was added to a running biofilter to investigate the effects on nitrifying bacteria. Mild effects were recorded in the nitrification and bacteria microbiome. In the third trial (3) – Nile tilapia (Oreochromis niloticus) were exposed to similar concentrations of azadirachtin for seven days (and the same period for recovery) to investigate effects on fish hematology, blood biochemistry, antioxidative enzymes in the brain, gills, muscle, liver and intestine and histopathology of gills and liver. Results showed mild effects in hematology and biochemistry profile in fish and higher levels of lipid peroxidation in the liver during the exposure. The results indicate a safe use of pyrethrum and spinosad in aquaponics setup, while azadirachtin has to be used with care, especially in coupled aquaponics systems.
1 Introduction
Aquaponics is a sustainable food production system that integrates the simultaneous culture of plants and fish. Modern aquaponics started in the 1970s mainly as a hobby and backyard activity, but it advanced in recent years, and it is “on the brink of commercialization” (Palm et al., 2018). Increased interest in production was accompanied by the growth of published research papers covering this topic, which increased almost exponentially until 2019 (Yep & Zheng, 2019). Aquaponics combines well-established practices transferred from both plant and animal sciences. This includes plant pest management, as the main recommendation is to use integrated pest management (IPM) for pest control in aquaponics (Bittsanszky et al., 2017). This is because aquaponics is regarded as eco-friendly food production, so chemical plant protection products must be considered the last resort for treating pests. A comprehensive review paper was recently published by Folorunso et al. (2021) in which several recommendations were given prior to the use of chemical agents: (a) culture control; (b) physical and mechanical control measures (such as UV irradiation, ozonation, and filtration) and (c) biological control measures. Chemical control practices were mainly used from the knowledge gained in hydroponic systems (Stouvenakers et al., 2019), with the major difference being that in aquaponics, fish (or other aquatic organisms) is added to the hydroponics system. This means that fish is regarded as a “non-targeted organism” in aquaponics, as unwished effects of plant chemical treatment could have adverse effects on fish (Yavuzcan Yildiz et al., 2019; Folorunso et al., 2021).
Moreover, in aquaponics, between plant and animal components of the system, there is also a microbial component populating the biofilter, which acts as biological water treatment (Yang et al., 2012). Using chemical treatment in aquaponics can also modulate the bacterial population in the biofilter, which could subsequently lower the water nitrification rate (Rašković et al., 2021). The easiest way to avoid the risk of applied chemicals affecting fish is to adopt decoupled aquaponic systems, which can physically separate water from the plant and fish components of the system (Stouvenakers et al., 2019; Baganz et al., 2022), but this is not always feasible. Moreover, coupled (one-loop) systems in which water flows in all compartments of aquaponics are the most frequently utilized by practitioners around the globe (Palm et al., 2019).
In aquaponic and hydroponic setups, plants are susceptible to different kinds of pests and diseases. Greenhouses carry even higher risks due to the specific environment in which plants are grown, characterized by high humidity, temperature, and plant density (Reddy, 2016). Plant pests include various groups of organisms such as fungi, viruses, bacteria, insects, and nematodes, among others (Jensen, 1997), but the presence of pest insects is of particular importance because, apart from the direct impact they will have on plant, they can also serve as a vector for other types of diseases (Wisler & Norris, 2005). As already mentioned, insects in aquaponics are usually treated with biological and biodegradable insecticides. These natural products are shown to be effective against various insects in a range of hydroponic facilities and setups across the World, such as in Egypt, Greece, and Thailand (Saleem et al., 2019; Lykogianni et al., 2021; Thaochan et al., 2021) or even Antarctica (Bergstrom et al., 2018), while similar scientific studies in aquaponics are lacking. To the authors` knowledge, there is not a single research paper or grey literature findings that focus on effects of insecticides to biofilter bacteria and fish. There is also a lack of information on the specific amount of biological insecticides used in aquaponics or hydroponics (Isman, 2020), as the Food and Agriculture Organization of the United Nations does not provide detailed statistics. Ujváry (2010) recognized three large groups of natural agents used worldwide for insect control: botanical insecticides, microbial insecticides, and semiochemicals. The present study aimed to test the impact of three insecticides: two botanical insecticides (pyrethrum and azadirachtin) and one microbial (spinosad). They were chosen due to their availability and presence in stores, primarily in the Czech Republic, where this study was conducted. Pyrethrum and azadirachtin are extracts from chrysanthemum (Chrysanthemum cinerariifolium) plant and neem tree (Azadirachta indica) seeds, respectively, while spinosad is the fermentation product of aerobic soil bacterium (Saccharopolyspora spinosa). All three insecticides have different properties and modes of action: spinosad is a systemic insecticide (van Leeuwen et al., 2005), meaning that it is soluble in water and has fast access to the plant vascular system; azadirachtin is weakly systemic through the leaves and systemic in the root (Kreutzweiser et al., 2011), while pyrethrum is non-systemic insecticide. These properties of insecticides are essential since, together with accidental drift, they can enter the water in which fish are reared. The concentrations of insecticides in the water are extremely important due to the possible acute or chronic effect on fish, and one of the measurements is lethal concentration LC50 – the concentration of toxicant which will lead to death of 50% of exposed fish within certain time frame (usually 96h). The review of LC50 for all three insecticides and several important aquatic species can be found elsewhere (Ujváry, 2010; Rašković et al., 2021).
The present study aimed to: (1) assess the risk of commercial formulations of insecticides mentioned above by applying them to basil plants (Ocimum basilicum) and monitoring their concentrations in the water for 3-4 days; (2) apply detected concentrations of the selected insecticides on working biofilter in order to assess possible effects on nitrification; (3) apply measured concentrations of the selected insecticide on Nile tilapia (Oreochromis niloticus) in order to investigate possible subacute toxicity to fish. The study is conceived as a simulation of a real-life scenario that can later be extrapolated to small aquaponics units and more comprehensive production systems.
2 Material and methods
2.1 Pesticide formulations
In order to investigate a real-life scenario, commercial products of insecticides were purchased from a local shop in České Budějovice (Czech Republic), and the following formulations were used: ND Spruzit AF (W Neudorff, Germany), with pyrethrum as an active ingredient (1.8% of Pyrethrum); Neem Azal - T/S (Biocont Laboratory, Czech Republic), with azadirachtin as an active ingredient (1.2% of azadirachtin); Spintor (AgroBio Opava, Czech Republic), with spinosad as an active ingredient (22.8% of spinosyn A and D). Neem Azal was applied at the rate of 0.3 mL m-2 of plant area. Spruzit, on the other hand, was applied at the rate of 60 mL m-2 while Spintor was applied at the rate of 0.04 mL m-2 area. These application doses were manufacturers’ recommended dosages for greenhouse vegetables. 300 mL of spraying solutions were prepared per each treatment, hence, each experimental unit was sprayed with 100 mL of insecticide solution.
2.2 Study design
The major obstacle during the planning phase of the study was that it was not possible to obtain ethical permission to assess the toxicity of insecticides to fish in an aquaponics setup. Fish toxicity tests are allowed only in laboratory conditions in line with specific guidelines provided by OECD, US EPA or similar national guidelines. Moreover, due to the complexity of the aquaponics system and the fact that it is challenging to manage fish, plants, water quality and biofilter at the same time, the authors decided that instead of investigating the effects of the insecticides in one aquaponics setup, it would be more precise and methodologically exact to conduct the experiments in three phases. The first experiment was conducted to investigate the concentrations of insecticides ending up in different aquaponics systems following foliar application on the basil plant. Using the basis of information on concentrations obtained from the first experiment, a potential risk assessment for biofilter bacteria and fish was investigated only with azadirachtin. The decision to exclude pyrethrum and spinosad from these experiments was because we could not detect quantifiable concentrations of the insecticides in the hydroponics solution (more data and rationale for this decision can be found in the result section); Therefore, the second experiment was conducted with three chosen concentrations of azadirachtin (1.5 µg L-1, 7.5 µg L-1, 15 µg L-1), in a dose-response manner. These concentrations were chosen considering the multiple application of the insecticide in hydroponic/aquaponics practices. In order to test the possible effects of the insecticide runoff on the nitrifying bacteria, these three concentrations were subsequently applied to the water of a working biofilter; the third experiment was a subacute toxicity test of azadirachtin to the Nile tilapia using the same concentrations as in the second experiment with a biofilter.
2.3 Experiment 1 – pesticide runoff in water
Two hydroponic systems were assembled at the experimental facility based at the Faculty of Fisheries and Protection of Waters, University of South Bohemia in České Budějovice (Czech Republic): media bed and floating raft systems. Both systems were assembled inside an experimental greenhouse equipped with automatic temperature, lighting and humidity regulation. (1) each experimental unit consisted of 12 polyethylene grow boxes (60 cm× 40 cm × 28 cm) filled with commercially available expanded clay pebbles (hydroton), filled up to 85% (51 cm) of the grow box and 12 polyethylene sump buckets with the volume of 40 L that served as a reservoir of water for each container (Figure 1). Water was pumped to grow box using an electric pump (6000 SOBO, 85W), regulated with the sensor, and the water returned to the plastic tank via the force of gravity through a bell siphon outlet. 8 plants of basil with an average height of 15.1 ± 2.4 cm were transplanted to each grow box at 15 cm spacing. The plants were placed in the net pots so that the tips of the roots pass through the perforated net pots filled with the same hydroton pebbles to ensure an easy passage of water between the plant root and the expanded clay pebbles. Plants were left to acclimatize in the experimental units for one week, during which the nutrients solutions were prepared from commercial Flora (General Hydroponics, California, USA) using the manufacturer’s guidelines for leafy vegetables (FloraGro 2.5 mL L-1, FloraMicro 2 mL L-1, and Florabloom 1 mL L-1). The trial commenced at 18:00 hr on the 16th of July, 2019. A foliar application of the three insecticides was carried out by spraying each unit with 100 mL of the prepared insecticide solution, while three control replicates were sprayed with water of same volume. During the foliar application, precautions were taken to avoid contamination of different treatments by demarcating each experimental unit with cardboard before the application. Before the foliar application, pesticides were thoroughly dissolved in water by mixing, using the manual provided by the manufacturer. Water samples for determining the concentration of insecticides were taken after 3, 6, 12, 24, 48, 72, and 96h post application. The plant growth was monitored for adverse effects of insecticides 7 and 14 days after the beginning of the trial, while basic water parameters were measured using a multimeter, HI9849 (Hanna, Romania). Dissolve oxygen (DO), pH, and electrical conductivity (EC) were kept at >5 mg L-1, 5.5-6.5, and >1, respectively, throughout the entire duration of the experiment (Table 1).
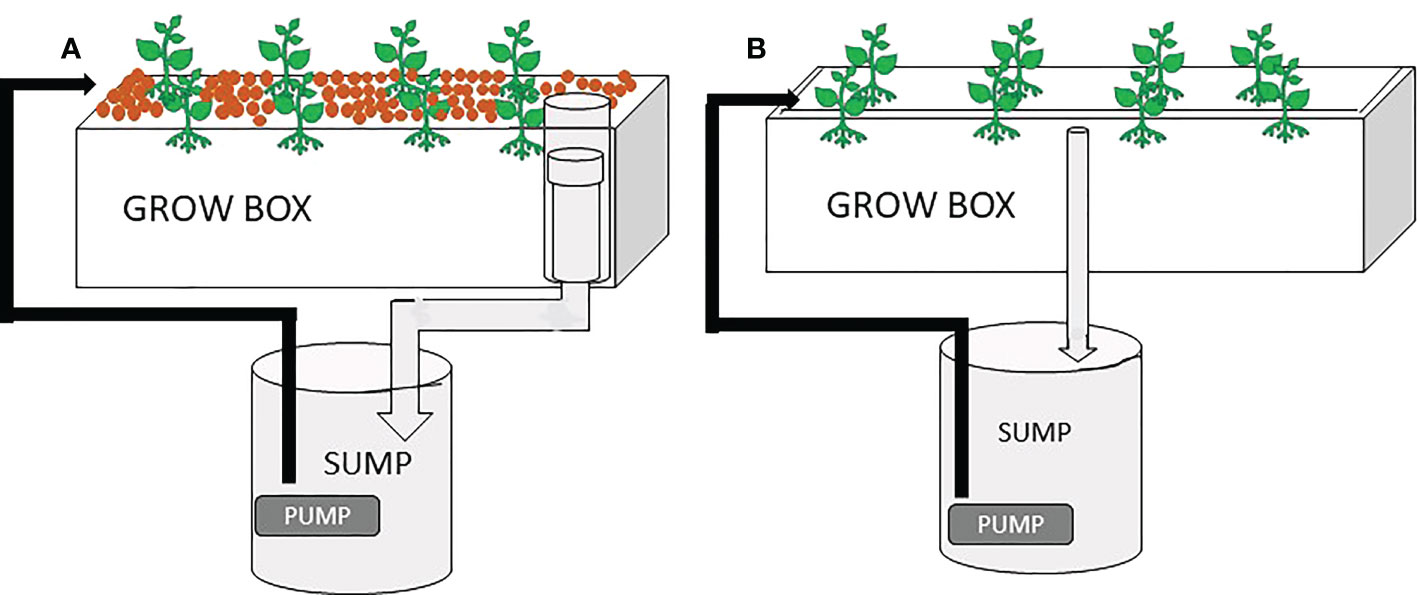
Figure 1 The schematic design showing the sample media bed system (A), consisting of grow pebbles, bell siphon outlet, 0.2 m2 grow box, 40 L sump bucket, waterflow pipes, submersible pump and basil plants; and the raft system (B), consisting of 0.2 m2 grow box, polystyrene grow foam, 40 L sump bucket, submersible pump, waterflow pipes, and basil plants.
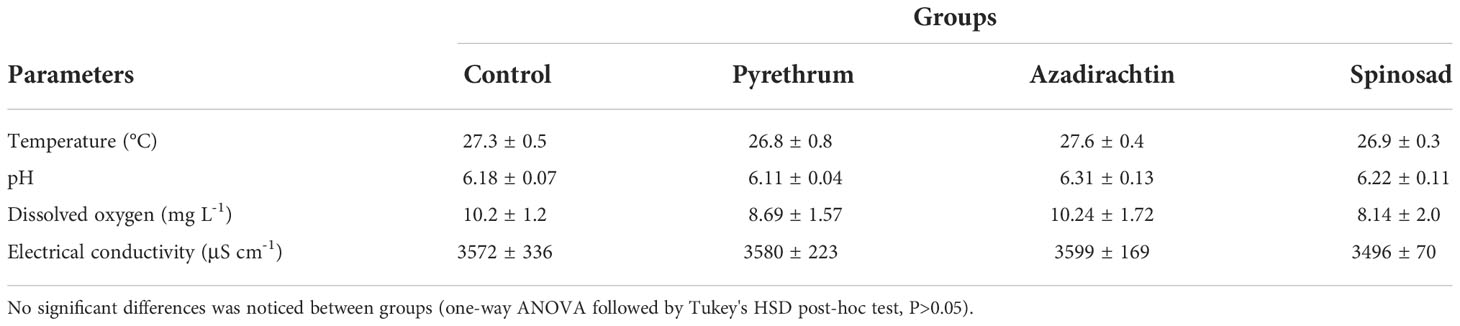
Table 1 The physico-chemical parameters (mean values ± SD) of the nutrient solutions during the raft and the media bed experiment.
(2) In the experiment above, pyrethrum was not detected at a quantifiable concentration in the water samples. This forms a basis for conducting a similar trial in raft systems to attest to the hypothesis that runoff concentrations might defer in different hydroponics systems. The floating raft system was assembled in 12 identical plastic grow box connected to a sump (Figure 1B). At the top of each grow box was a 3’’ thick polystyrene foam sheet having 8 holes in which 4”-diameter net pots can fit in. Plants were placed in the net pots in a way that the roots were always in contact with water on which polystyrene foam was floating, while stem and leaves were above the sheet. The same protocol was followed in the media bed for the application of insecticides and trial started at 18:00 hr on the 10th of August, 2019. The only difference was that water samples were taken after 1, 3, 6, 12, 24, 48 and 72h, because in the media bed trial, pyrethrum concentration was below the limit of quantification at all sample times (see the chapter “Results”), so we hypothesized that it would be found in the water of hydroponic system after 1h. The average basil plant height ( ± SD) used in this experimental setup at the start of the experiment was 30.0 ± 0.8 cm.
2.3.1 Determination of the pesticides in water
A combination of online solid-phase extraction, liquid chromatography, and mass spectrometer (LC-LC-MS) was used to quantify pesticide concentrations in water samples. The developed analytical method was based on a work by Khan et al. (2012). HTS XT-CTC auto-sampler (CTC Analytics AG, Zwingen, Switzerland), Accela 1250, and Accela 600 LC pumps (Thermo Fisher Scientific, San Jose, CA, USA) were components of the LC-LC system together with Hypersil Gold aQ column 20 x 2.1 mm, 12 µm (SPE) and Hypersil Gold Phenyl 50 x 2.1 mm, 5 µm as analytical column (Thermo Fisher Scientific, San Jose, CA, USA). Gradient elution for sample extraction (injected sample volume 1 mL) and chromatographic separation is further described in Table 1 of the Supplementary Material. Solvent A represents ultra-pure water prepared with AquaMax Basic 360 Series and Ultra 370 Series (Young Lin Instruments, purchased from Labicom, Czech Republic) and Solvent B methanol (Merck, Germany, LC grade).
Compounds were detected with triple quadrupole mass spectrometer TSQ Quantiva and HESI ion source (Thermo Fisher Scientific, San Jose, CA, USA). The instrument operated in negative and positive ion mode and selected reaction monitoring data acquisition mode. Detailed instrument setup is concluded in Table 3 of the Supplementary Material. The methods of evaluating parameters such as linearity of a calibration curve, the limit of quantification (LOQ) (Solliec et al., 2014), accuracy, and precision (Kruve et al., 2015) were evaluated prior to sample analysis. The results are summarized in Table 4 of the Supplementary Material. Sample matrix used for all evaluation parameters was identical to the experimental samples.
The results were calculated with an average response factor with internal standard calibration when each sample was spiked with 20 ng of isotopically labeled standard (Borik et al., 2020). Analytical data postprocessing and reporting were performed with TraceFinder 4.1 (Thermo Fisher Scientific, San Jose, CA, USA). Analytical standard of azadirachtin used for method evaluation and preparation of calibration curve was purchased from Sigma-Aldrich (Czech Republic). Stock solutions of native and internal standards were prepared at 1 mg mL-1 in methanol (Merck, Germany, LC grade) and stored at −20°C.
2.4 Experiment II - biofilter trial
The biofilter study is conceived on the design of an already published trial study (Rašković et al., 2021). The biofilter trial was run in 12 polyethylene circular tanks with a net volume of 35 L each. The tanks were filled with 12 L of dechlorinated tap water, 3 L of RAS water, and 3 L of biofilter media BT10 (Ratz Aqua and Polymer Technik, Germany) from a running RAS. The system was placed indoors in an air-conditioned room. All buckets were equipped with two round air stones (5 cm diameter, Hailea, China), delivering air and mixing the bio media-water solution to mimic the conditions of a biofilter. Air was supplied with a central air blower (Secoh JDK-50, Japan). During the stabilization period, the bacteria consortium was fed 10 mg L-1 of NH4-N twice daily using NH4Cl (Penta, Czech Republic) stock solution (1.5 mg L-1 of NH4-N). Temperature, oxygen saturation, and pH were measured twice daily (mean ± SD; pooled data for all buckets, no significant differences p > 0.05; t = 22.4 ± 0.1°C; O2 = 90.4 ± 4.0% and pH was kept between 7 and 8 using 10% NaHCO3 solution (Penta, Czech Republic) with a handheld multimeter (HI9829, Hanna Instruments, Romania). Before the experiment’s commencement, the water was well mixed between treatments and control to ensure homogeneity of the water parameters.
After the stabilization period, the azadirachtin-based pesticide (10.6 g L-1 of azadirachtin; Neem Azal T/S, Biocont, Czech Republic) was applied from a stock solution with a concentration of 1.5 µg L-1 azadirachtin. The stock solution was prepared right before the application by mixing 2.1226 mL of the pesticide and 997.9 of ultra-pure water and shook vigorously to ensure proper mixing (calculation based on declared azadirachtin concentration in the pesticide and its density of 0.98 g mL-1). The azadirachtin was applied in three concentrations, while the control was left untreated. The concentrations were as follows: 1.5 µg L-1; 7.5 µg L-1; and 15 µg L-1. The lowest concentration (1.5 µg L-1) mimicked the highest concentration detected in the plant trial, while the others were 5 and 10 times higher, respectively, mimicking possible accumulations in other hydroponic systems such as nutrient film technique and drip irrigation which use lower water volumes compared to rafts systems used in this experiment. To measure the azadirachtin concentrations, 15 minutes after the application, 10 mL of water was sampled from each unit, filtered into a glass vials through 0.2 µm PVDF syringe filter (Whatman, Germany). The water samples were frozen until further analyses (described below). Samples of the stock solution and tap water were also taken (Table 1 in the Supplementary Material).
2.4.1 Determination of nitrogen species in water
During the next 72 hours, the NH4-N, NO2-N and NO3-N were measured twice daily using standard spectrophotometric method (APHA, 1989). Temperature, oxygen saturation and pH were measured twice daily (HI9829, Hanna Instruments, Romania). pH was maintained in a range of 7 to 8 using 10% NaHCO3 solution (Penta, Czech Republic). The systems were fed with 10 mg L-1 and 15 mg L-1 of NH4-N daily using NH4Cl (Penta, Czech Republic) in the morning and evening, respectively. In order to investigate the possible effects of these pesticide concentrations on the nitrifying bacteria, 25 media elements were collected from each unit after 6 hours of application for DNA analysis.
The bacteria load in the elements was extracted 6 hours after application of the pesticide. Bacteria were obtained by adding 50 mL of ultrapure water to a 100 mL falcon tube containing the elements and vigorously vortexed for two minutes. This was followed by placing the tube in an ultrasonic bath (Sonorex; Baudelin) for 5 minutes. Afterwards, the media were removed and the biofilm was centrifuged (5000 rpm, 10 min). The pellet was used for DNA extraction (DNEasy, Quiagen, Germany). Sequencing on an Illumina MiSeq (2 × 300 bp) was done by GATC AG (Konstanz, D) according to the InView™ Microbiome Profiling protocol (see Schmautz et al., 2017 for details). Data have been made available under the study accession PRJEB56899 at EBI.
2.5 Experiment III – fish exposure trial
2.5.1 Description of semi-static exposure assay
180 individuals of Nile tilapia (Oreochromis niloticus) with average body mass of 141 ± 23 g (mean ± SD) were purchased from Kirschauer Aquakulturen GmbH fish farm (Schirgiswalde - Kirschau, Germany). Fish were transported to the Laboratory of Nutrition (Institute of Aquaculture and Protection of Waters, University of South Bohemia in České Budějovice), where they were kept in plastic tanks and fed daily with Skretting T3 tilapia feed (3 mm floating pellets; 44% crude protein, 10% lipid, 25% carbohydrate and 11.5% ash; Skretting, Czech Republic), at a ratio of 2.5% body weight. Prior to the beginning of the experimental trial, fish were transferred to Laboratory of Aquatic Toxicology and Ichtyopathology (Research Institute of Fish Culture and Hydrobiology, University of South Bohemia in České Budějovice) and randomly allocated to 12 glass aquaria (15 fish in each aquaria) with following dimensions (L x W x H): 65 x 45 x 40 cm, and total volume of 100 L. Fish were placed in the aquaria for 10 days of acclimatization period, while subsequent semi-static exposure assay was conducted for 7 days. In addition, recovery period of another 7 days was given to the same batch of fish. Fish were exposed to the following nominal concentrations of azadirachtin-based commercial product AZA in triplicates: 1.5 µg L-1, 7.5 µg L-1, 15 µg L-1 and control group, which contained only water. During the course of the experiment, actual concentration of azadirachtin was determined in the water using spektrometr TSQ Quantiva Triple-Stage Quadrupole (Thermo Scientific) on day 1 (after addition of water), 3, 5 (both before and after exchange of water) and 7 (before termination of the experiment) of the trial. Actual concentrations differed more than 20% comparing to nominal concentrations, so we decided to use actual concentrations in the text of this manuscript, as recommended by OECD guidelines (OECD, 2019). Concentrations were measured as following: group A (nominal - 1.5 µg L-1): 2.04 ± 0.99 µg L-1; group B (nominal 7.5 µg L-1): 6.52 ± 2.83 µg L-1; group C (nominal 15 µg L-1): 7.93 ± 2.91 µg L-1, while in control group, no trace of AZA was discovered in the sampled water; thus, experimental groups will be named as Group 0, Group 2, Group 6.5 and Group 8 µg L-1. During semi-static exposure and recovery period, total volume of dechlorinated tap water was exchanged on every second day (days 1, 3 and 5), while basic water parameters were measured using HI 98194 (Hanna Instruments) device. Following values are recorded during (1) exposure assay: temperature: 26 ± 1°C, pH value: 7.8 ± 0.5, oxygen saturation: 90-99%; total ammonium 0.02 mg L-1 and (2) recovery period: temperature: 26 ± 1°C; pH value: 7.8 ± 0.5; oxygen saturation: 90-99%; total ammonium: 0.02 mg L-1.
2.5.2 Fish sampling
At the end of the exposure period (day 7) and at the end of the recovery period (day 14), three fish per aquarium was randomly picked and anaesthetized with a solution of buffered ethyl 3-aminobenzoatemethanesulfonic acid (MS 222) (Sigma-Aldrich, Czech Republic). Blood was sampled using heparinized syringe and needle (5000 IU heparin sodium salt in 1 mL), with insertion of needle in the caudal vein. The blood samples were later stabilized with an aqueous solution of heparin sodium salt in the rate of 0.01 mL L-1 and were immediately processed. Second portion of blood was used for biochemical analyses and was centrifuged at 1500 ×g for 10 min in a microcentrifuge (MPW 55, MPW Instruments, Poland). Supernatant, containing blood plasma was collected, transferred into tubes on ice and stored at -80°C until subsequent analysis. After blood sampling, fish were carefully dissected and second gill arch from the right side of every fish, and part of liver were taken for histological assessment and placed in 4% formalin (Sigma-Aldrich, Czech Republic) for fixation, while samples of gills, brain, kidney, muscle, intestine and liver were taken for determination of concentrations of antioxidative enzymes in mentioned fish organs. These samples were snap frozen in liquid nitrogen and kept at -80°C until further processing. Frozen tissues were later weighted and homogenized in 50 mM potassium phosphate buffer, pH 7.0, containing 0.5 mM EDTA (1:10, w/v) using an Ultra Turrax homogenizer (Ika, Germany) and divided in two parts – one for measuring TBARS and other was subjected to centrifugation at 12,000×g for 30 min at 4°C and supernatant is used for determining of antioxidant parameters (SOD, GPx and GR).
2.5.2.1 Heamatological and biochemical blood plasma parameters
Several heamatological parameters were determined from sampled blood and analyzed using protocol by Svobodova et al. (1991): number of red blood cells (RBC), concentrations of heamatocrit (Ht) and haemoglobin (Hb), mean corpuscular volume (MCV), mean corpuscular haemoglobin (MCH), mean corpuscular haemoglobin concentration (MCHC), number of leukocytes and leukogram (lymphocytes (%), monocytes (%), neutrophil segments (%), neutrophil bands (%), myeloid sequence (%)).
Concerning plasma biochemical parameters, following one were measured: total protein (TP), albumin (ALB), globulin (GLB), glucose (GLU), triglyceride (TG), phosphorous (P), magnesium (Mg), creatinine (CREA), lactate (LACT) and ammonia (NH3) concentrations, and activities of alanine transaminase (ALT), aspartate aminotransferase (AST), alkaline phosphatase (ALP), lactate dehydrogenase (LDH) and creatinine kinase (CK). They were determined using a blood analyser VETTEST 8008 (IDEXX Laboratories Inc., USA) according to already established protocol (Kolarova and Velisek, 2012).
2.5.2.2 The antioxidant parameters and lipid peroxidation of tissues
Three antioxidant parameters were determined spectrophotometrically from the tissues of sampled fish: (1) glutathione peroxidase (GPx; EC 1.11.1.9), by determining the rate of NADPH oxidation at 340 nm by the reaction with glutathione reductase (GR; EC 1.6.4.2) The specific activity was determined using the extinction coefficient 6.22 mM cm−1 (Lawrence and Burk, 1976); (2) GR, by measuring rate of NADPH oxidation at 340 nm (Carlberg and Mannervik, 1975). For both GPx and GR activity - one unit was defined as the quantity of enzyme that consumes 1 mol mL-1 of substrate or generates 1 mol mL-1 of product per minute and is expressed in IU per mg of protein; (3) total superoxide dismutase (SOD; EC 1.15.1.1) activity was detected using the method developed by Marklund and Marklund (1974), based on autoxidation of pyrogallol. SOD activity was assessed at 420 nm and expressed as the amount of enzyme per milligram of protein. For lipid peroxidation of sampled tissues, the TBARS assay was employed using methodology described by Luschak et al. (2005). The TBARS concentration was calculated by the absorption at 535 nm and a molar extinction coefficient of 156 mM cm-1. The value was expressed as nmol of TBARS g-1 wet weight tissue.
2.5.2.3 Histological processing and assessment
After 24 hours of fixation samples were transferred to 70% ethanol and stored for further processing. Later, they were placed in tissue processor (Leica TP 1020, Nussloch, Germany), dehydrated, cleared using xylene and embedded in paraffin. Paraffin blocks were cut on 5 µm thickness using microtome and mounted on glass slides, which are further stained using automated staining device (Leica ST 4040, Nussloch, Germany). At the end, cover slides are mounted and slides were assessed for the presence of histopathological alterations using semiquantitative scoring system. Each alteration that was present in the slides of gills and liver was given one of the following scores: 1 (mild), 2 (moderate) or 3 (severe), depending on amount of altered tissue.
2.6 Statistics
Prior to statistical analysis, all data sets were tested for normality and homoscedasticity using Shapiro-Wilk’s and Levene’s test, respectively. If data set passed both assumptions, then ANOVA followed by Tukey’s HSD post-hoc test was used, and if not, non-parametric Kruskal-Wallis H test was used, while difference between experimental groups was tested using Mann Whitney U test. The significance level (α) was set at 5% while all values are presented as means ± standard deviation (SD). For all statistical analysis PAST software, version 4.06b (Hammer et al., 2001) was used.
3 Results
3.1 Experiment I – pesticide runoff and degradation rate
Measurements of concentration of insecticides in the water after application on plants showed distinctive patterns in both tested systems during time points (Figure 2). Concentrations of pyrethrum were lower than the limit of quantification (50 ng L-1) in all sampling points in both systems. Spinosad showed lower concentration in the water when applied to plants in media bed system, comparing to floating raft system.
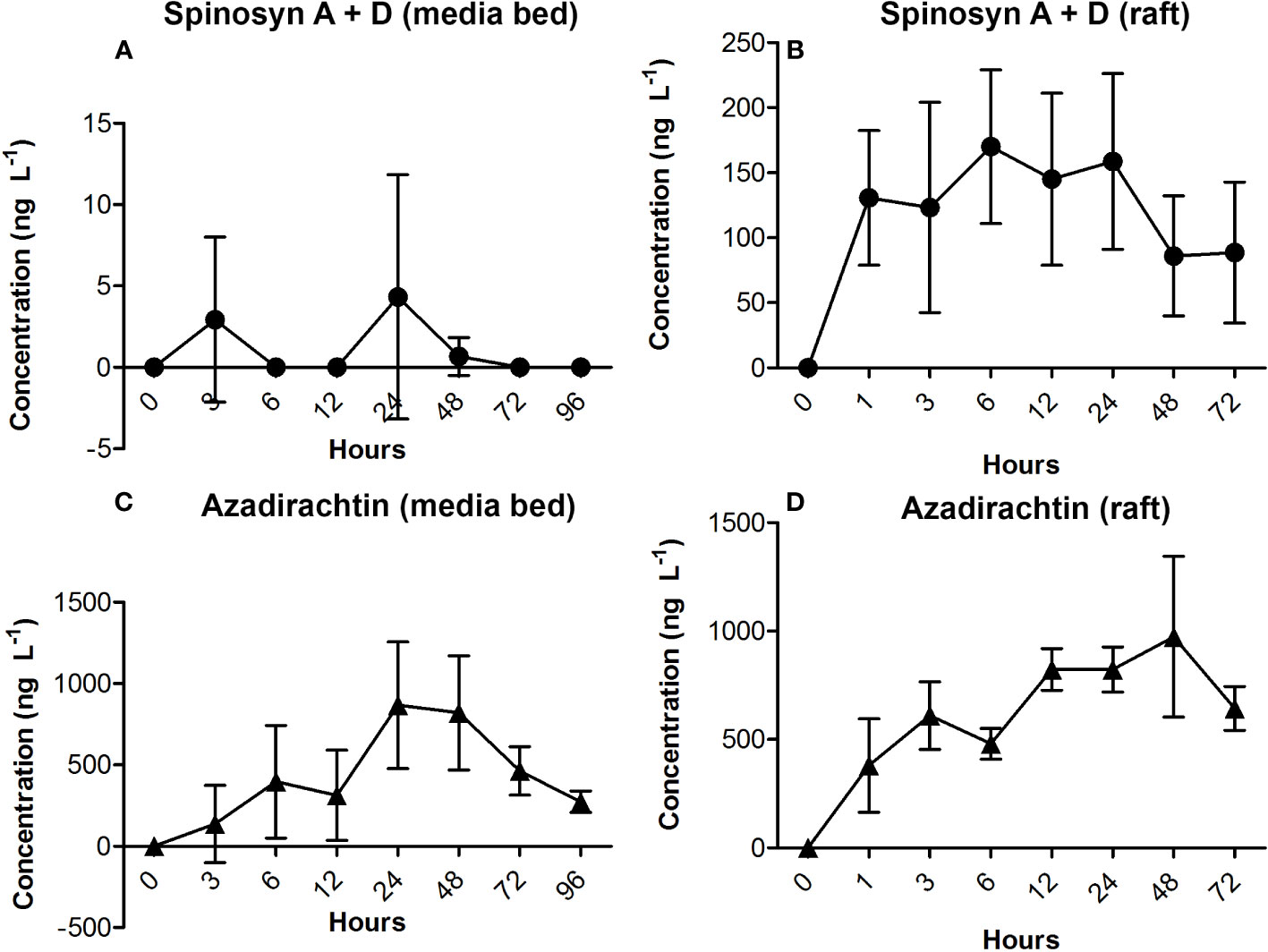
Figure 2 Aqueous concentrations of two insecticides in relation to time points at two different systems for growing basil in hydroponics: (A) concentrations of spinosyn A + spinosyn D at media bed system during 96h; (B) concentration of spinosyn A + spinosyn D at floating raft system during 96h; (C) concentration of azadirachtin at media bed system during 96h; (D) concentration of azadirachtin at floating raft system during 96h.
The highest concentration of spinosad sampled in the water from single replicate was 13 ng L-1 at media bed system and 230 ng L-1 at floating raft system, while mean concentrations of spinosad in both systems peaked early, between 6 and 24 h after application and gradually declined afterwards. Mean concentrations of azadirachtin were also higher in floating raft system at each sampling point, similar to spinosad. Maximal concentration in single replicate was 1.3 µg L-1 at media bed system and 1.4 µg L-1 at floating raft system, but peaks were measured at different sampling points: when applied at media bed system, peak concentrations were established after 24 h and were gradually lowered after 48 h, while concentration in the water from floating raft system peaked later, after 48 h and started to decline afterwards. The percentage of these detected concentrations were <0.01% of the applied concentration of the active ingredient per treatment.
3.2 Experiment II - biofilter trial
Biofilter trial showed no significant differences between control and buckets supplemented with azadirachtin at any concentration for all nitrogen compounds (NH4-N, NO2-N, and NO3-N). However, mean values of NH4-N in group AZA 15 was significantly higher comparing to AZA 1.5 after 12 hours of the trial (Figure 3). From the DNA result of the bacteria consortium in the biofilter to show community compositions, the largest bacteria phylum in all the treatments and control was Proteobacteria, with a percentage proportion >65% in all the treatments. Other major phyla were group after the end; Bacteroidetes (7-10%), Gemmatimonadetes (1-3%), Nitrospirae (4-16%), and Acidobacteria (2-3%) (Figure 4). Temporal community changes were found in the percentage proportions of Nitrospirae in the treatments and control. The average percentage proportion of Nitrospirae in control (16%) is significantly higher than the proportion in the 1.5 µg L-1 (4.1%), 7.5 µg L-1 (6.5%), and 15 µg L-1 (5.8%).
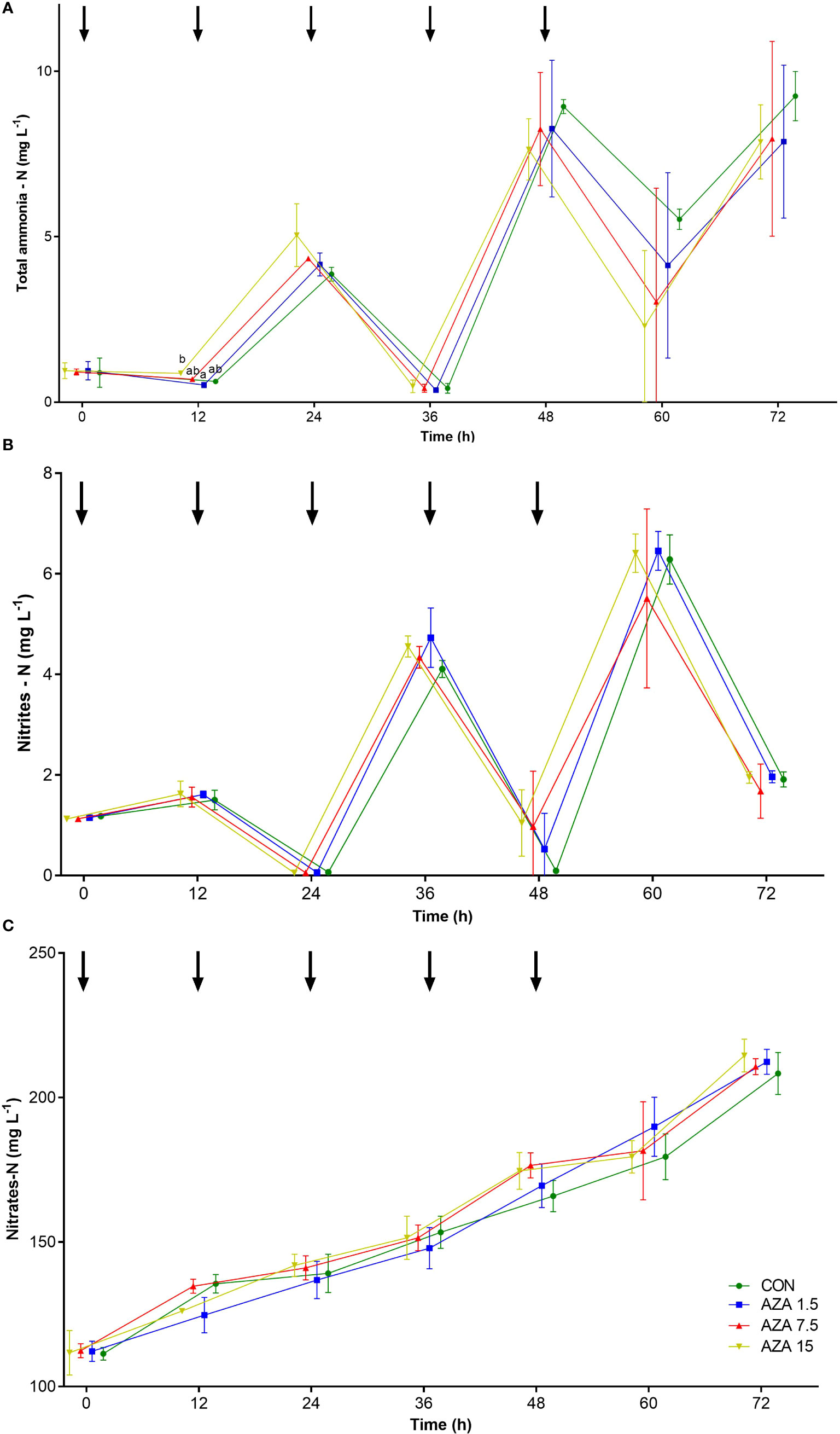
Figure 3 Concentrations (mean ± SD) of nitrogen compounds in the water from working biofilter exposed to varying concentrations of azadirachtin during 72h: (A) total ammonia – nitrogen; (B) nitrites – nitrogen; (C) nitrates – nitrogen; lines with different colors and symbols represent control group (CON), and concentration of azadirachtin insecticide (AZA 1.5; AZA 7.5 and AZA 15 µg L-1) added to the working biofilter; points are shifted to the left or to the right in order for easier comparison among groups; arrows show time when ammonium chloride was added to the buckets; different letters represent significant differences between groups within the same sampling point (one-way ANOVA followed by Tukeyˈs HSD posthoc test, P<0.05).
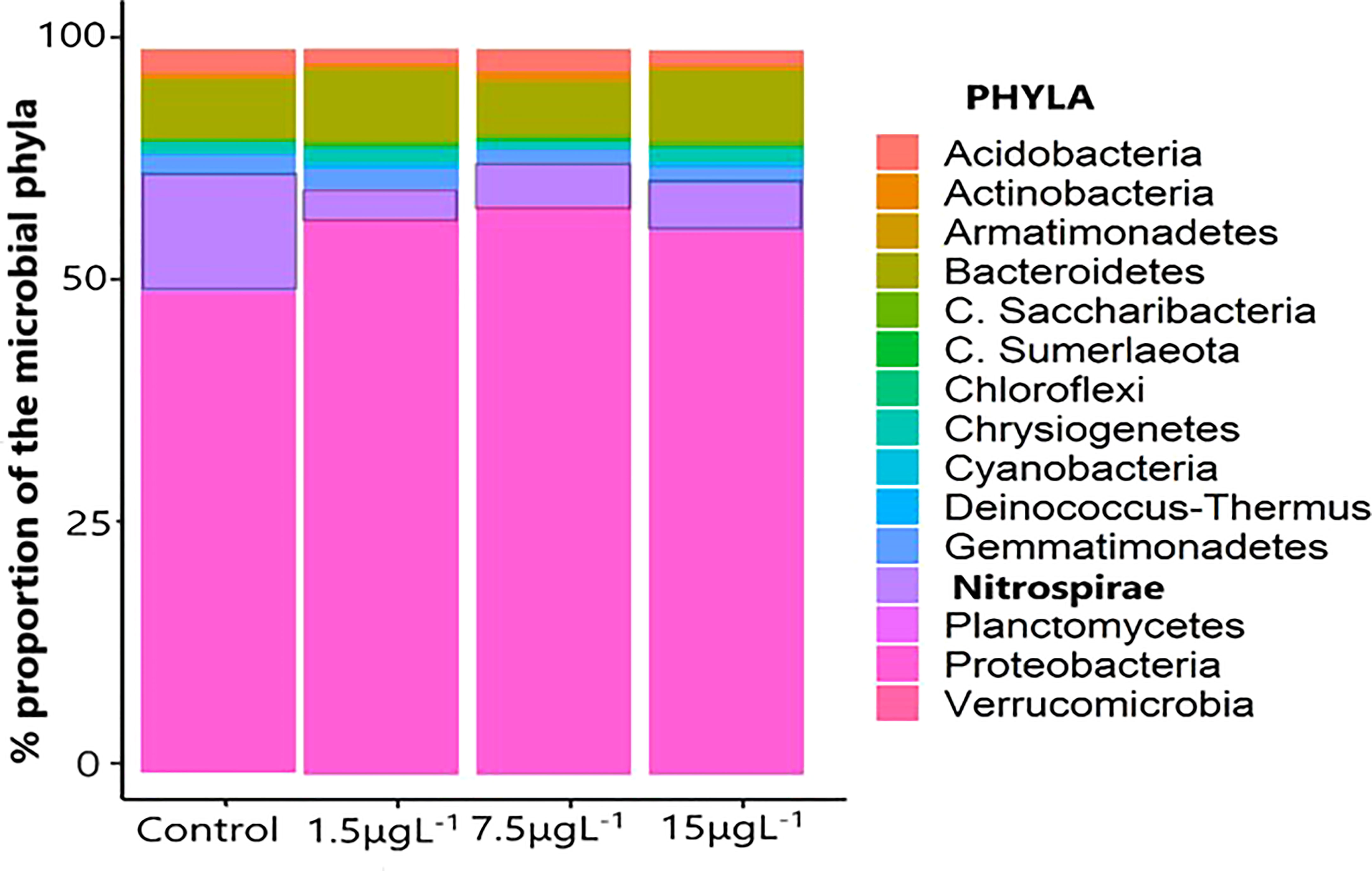
Figure 4 The percentage proportion of the bacteria consortium in the biomedia samples (n=3) from the biofilter treatments, 1.5 µgL-1, 7.5 µgL-1, 15 µgL-1, and control. Samples were taken 6 hours after the application of azadirachtin in the biofilter.
3.3 Experiment III – fish exposure trial
The average body mass and length of experimental fish in each treatment did not show a statistical difference from the control in both sampling points (7 and 14 days; Table 2). Hematological analysis revealed that majority of parameters did not significantly differ from the control group (Table 3). However, lower values were established between group AZA 8 compared to control for MCV after the exposure period (P < 0.05), but the same parameter was very similar among the groups at the end of the recovery period. MCHC showed a contrasting pattern, as groups AZA 6.5 and AZA 8 were higher compared to the control (P < 0.05) only at the end of the recovery period and not after the exposure. A Higher number of erythrocytes was recorded in fish from AZA 8 group compared for AZA 2 group after the end of exposure (and not recovery) period (P <0.05). On the other hand, the number of leukocytes was lower in groups AZA 2 and AZA 8 compared to the control after the exposure period and remained lower in groups AZA 8 and AZA 6.5 after recovery.

Table 2 Mean ± SD body mass (g) and total length (mm) of experimental fish measured after exposure (7 days) and recovery period (14 days).
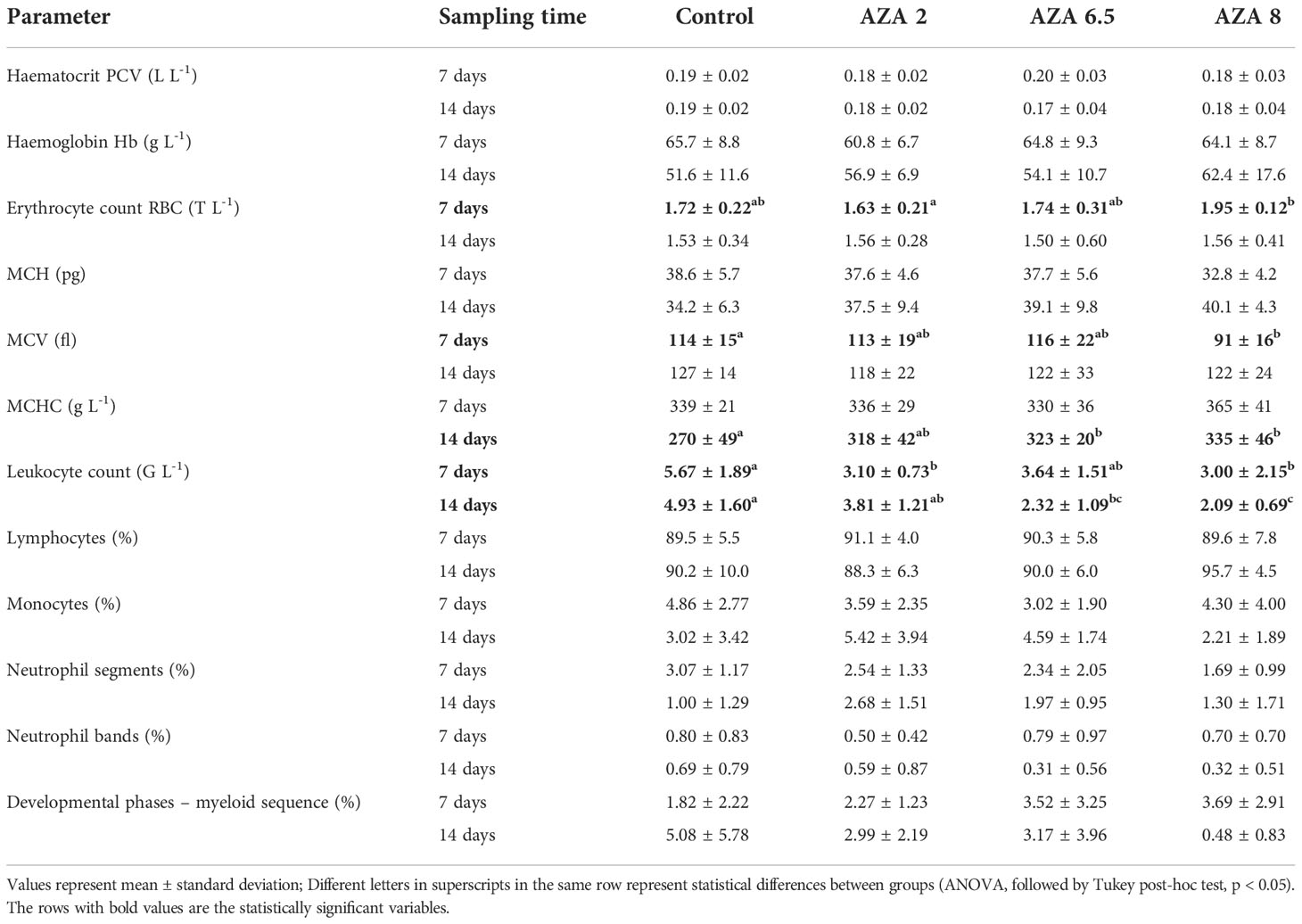
Table 3 Hematology values and leukogram (mean ± SD) measured from the blood of Nile tilapia kept in control and exposed to different concentrations of azadirachtin (AZA 2 µg L-1; AZA 6.5 µg L-1 and AZA 8 µg L-1) for 7 days and after recovery period (14 days).
Similar to the hematology results, only a few parameters were significantly altered in the blood biochemistry of the experimental animals after the exposure period (Table 4), but those changes ameliorated and no differences were established after the recovery period. The concentration of glucose and ammonia in the blood of animals from groups AZA 6.5 and AZA 8 were increased compared to both the control group and AZA 2 (P < 0.05), while creatine kinase was increased in group AZA 8, compared to all groups (P < 0.05). The dose-dependent response was established for the lactate concentration, as it increased in AZA 6.5 and AZA 8 groups (P < 0.05).
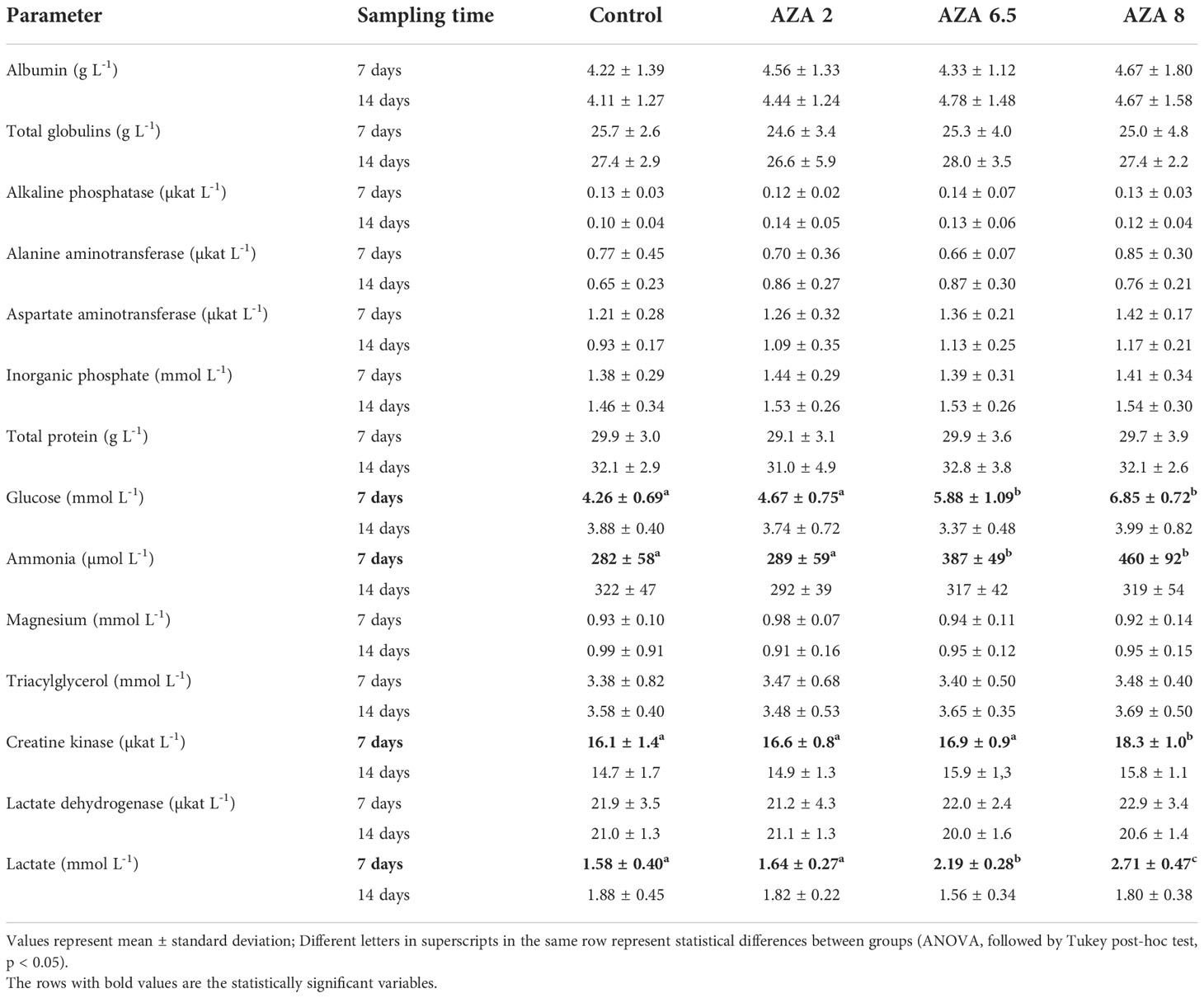
Table 4 Blood biochemistry values (mean ± SD) measured from the blood of Nile tilapia kept in control and exposed to different concentrations of azadirachtin (AZA 2 µg L-1; AZA 6.5 µg L-1 and AZA 8 µg L-1) for 7 days and after recovery period (14 days).
Concentrations of three antioxidant enzymes (GR, GPx, SOD) from five different tissues showed a mild effect from azadirachtin (Figures 5A–C). The concentrations of GR in any tissue did not differ between groups, while in GPx, only AZA 6.5 was lower compared to control in the sampled intestine (P < 0.05) after the exposure period. SOD concentration in the fish brain from the highest exposure group (AZA 8) was significantly increased after the recovery period compared to control fish (P < 0.05). On the other hand, lipid peroxidation measured by malondialdehyde concentration in the liver (TBARS assay) showed that all three exposed groups had higher values compared to the control (P < 0.05) after the exposure period. However, all values dropped after the recovery period (Figure 5D).
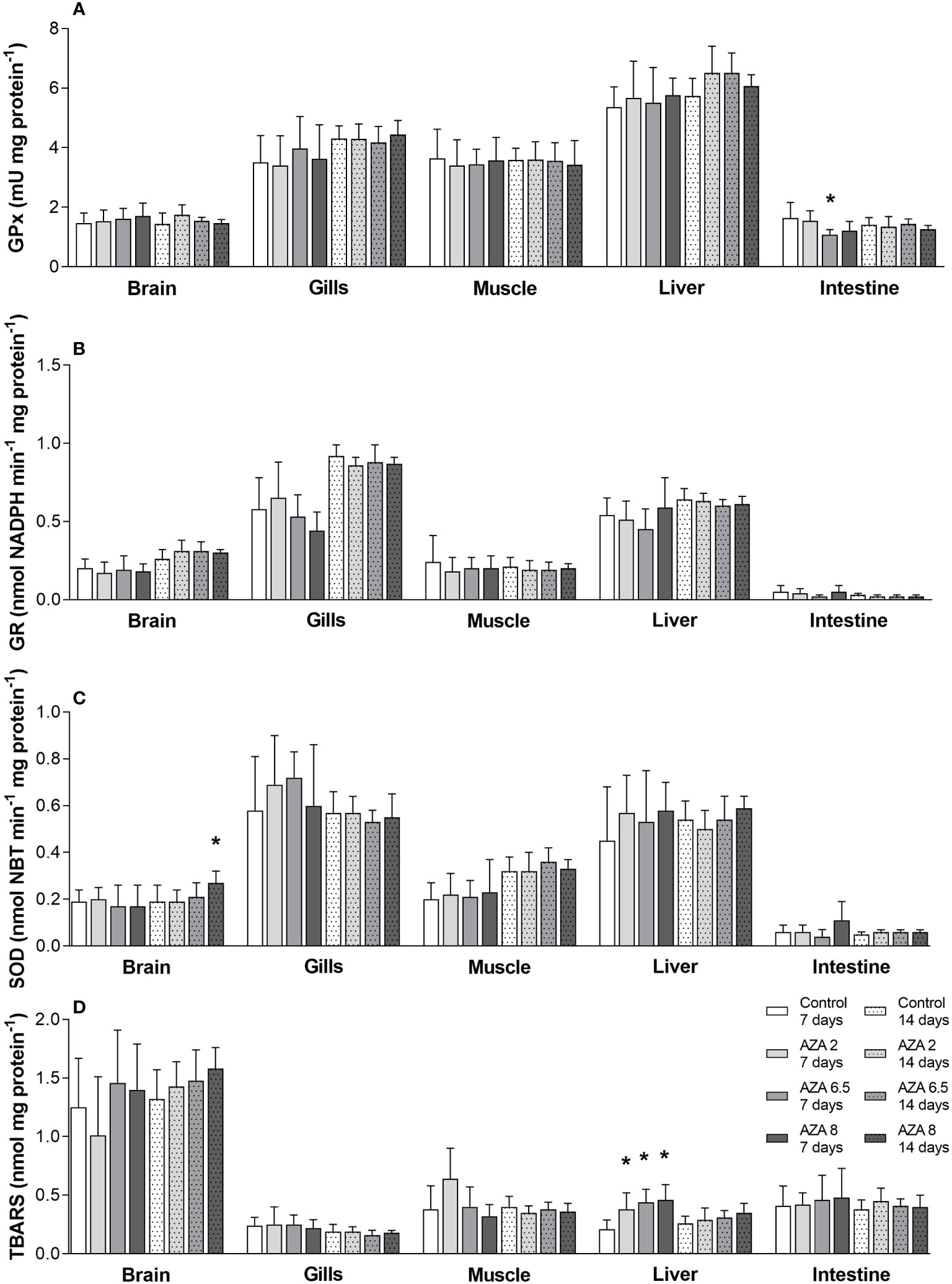
Figure 5 The mean ± SD concentrations of: (A) glutathione peroxidase; (B) glutathione reductase; (C) superoxide dismutase; (D) thiobarbituric acid reactive substances in the brain, gills, muscle, liver, and intestine of Nile tilapia exposed to 0, 2, 6.5, and 8 mg L-1 after 7 days and after recovery period (at the end of 14 days); asterisk denotes statistically significant difference (one way ANOVA followed by Tukey’s post hoc test; P < 0.05) between control and exposed group.
Histology of hepatopancreas showed no signs of significant alterations (Supplementary Table 2) after 7 days of exposure and 7 days of recovery. The majority of fish in all groups had some mild changes in the pancreas and they included degranulation of eosinophilic granulocytes, cellular inclusions, and vacuolated cytoplasm of hepatocytes. However, they were present in all groups and did not show statistical significance among groups (P > 0.05). Similar results were recorded in the gills (Supplementary Table 2), where higher semiquantitative scores were noted for infiltration of leukocytes in branchial tissue and for the proliferation of interlamellar cell mass in the primary epithelium. However, neither histopathological alteration in gills showed statistical significance among groups (P > 0.05).
4 Discussion
4.1 Insecticides runoff and degradation in nutrient solution
After applying three different insecticides to basil plants, it was obvious that pyrethrum possessed the fastest degradable properties, as there were no pyrethrum concentrations above the quantification limit (50 ng L-1) in any tested water sample, even one hour after application. Pyrethrum is composed of six esters, commonly known as pyrethrins which act as active substances (Zhu et al., 2020), but pyrethrin I and pyrethrin II account for approximately 73% of total amount of natural esters in pyrethrum (Ujváry, 2010). Moreover, in studies where concentrations of pesticides were assessed in soil and runoff water, pyrethrin II was determined in 10 to 100 times higher concentrations compared to pyrethrin I (Antonious et al., 1997) and this is the main reason why pyrethrin II was chosen as a focus molecule in testing water samples from the present study. The biodegradation of pyrethrum is extremely fast and the half-life of this compound strongly depends on sunlight, with a half-life ranging between 10-12 minutes (Ujváry, 2010). Since the foliar application of the insecticides was conducted in July and August, a reaction with the sun could be the probable reason why the pyrethrin was not detected at a quantifiable concentration in the nutrient solution.
In contrast to pyrethrum, two other insecticides were detected in the water in both tested systems (media bed and floating raft) (Table 5). The fact that water from the media bed system contained lower concentrations of insecticides is probably due to the adsorbent properties of expanded clay pebbles. Some types of clay show excellent removal efficiency of pesticides in the water (Cosgrove et al., 2019), which are, for particular substances, even comparable with active carbon. For example, for pesticide diuron, removal efficiency of expanded clay is 98%, surpassing 92% when active carbon is used (Tahar et al., 2014), but percentages are highly dependent on the type of adsorbent and pesticide/toxicant. Mean and maximal concentrations of spinosad in the water sampled from both tested systems should not be considered as a risk for fish in the aquaponics system. NOEC (No Observed Effect Concentration) of spinosad for fish such as Common carp (Cyprinus carpio), Common minnow (Phoxinus phoxinu) and rainbow trout (Oncorhynchus mykiss) have been reported to range between 0.7 and 5.2 mg L-1 (Barden, 1998). Also, while chronic NOEC for aquatic invertebrates is 0.0012 mg L-1, the compound is considered unharmful to microorganisms. In addition, 96h LC50 concentrations for spinosad are reported as > 202 mg L-1 for guppies (Poecilia reticulata) and platys (Xiphophorus maculatus) (Pereira et al., 2016) and > 500 mg L-1 for juvenile Coho salmon (Oncorhynchus kisutch) (Deardorff & Stark, 2009). These concentrations are thousand times higher than the highest concentration (1.3 ng L-1) reported in the present study, hence, no risk is perceived. Moreover, it was impossible to determine the exact analyte concentration presented in the sample during sampling time due to the high degradation rates of spinosyn in the water matrix under low temperature (4°C) and complete dark conditions. Although it was unable to properly quantify concentrations of spinosyn, the estimated concentration values also seem to be orders of magnitude lower than reported LOEC. In contrast, the persistence of azadirachtin in the water was longer compared to both pyrethrum and spinosad and could be considered a risk, as it is common practice to use it in multiple applications (Pavela & Benelli, 2016).

Table 5 The calculated percentage runoffs of the insecticides generated from the detected maximum concentrations of the insecticides in the nutrient solution and the concentrations of the applied active ingredients (AI) per treatment.
It is noteworthy to state that, the low percentage runoff (< 0.001%) of pyrethrum and azadirachtin detected in the nutrient solution is an indication of the mildness of their could-be-effects when applied in aquaponics systems. This result is in line with the meta-analysis based review by Folorunso et al. (2021). In the study, using the established NOEC and LC50 of commonly used pesticides, the authors found that only a runoff >10% of these pesticides could cause detrimental effects in aquaponics systems.
4.2 Effects of Azadirachtin on biofilter bacteria
The proper functioning of biofilter, inhabited by bacteria, is essential for aquaponic setup (Wongkiew et al., 2018). Bacteria in the biofilter are transforming ammonia (a toxic excretion towards fish) to nitrates in one step (COMMAMOX) or in two-step reaction (first, ammonia-oxidizing bacteria (AOB) are transforming ammonia to nitrites, and then nitrite-oxidizing bacteria (NOB) are transforming nitrites to nitrates) (van Kessel et al., 2015; Kasozi et al., 2021). The monitoring of ammonia concentration is essential for fish production in recirculating aquaculture systems (Becke et al., 2019), and the high concentrations could be detrimental to fish (Becke et al., 2019), so it is of utmost importance not to disrupt nitrifying processes that are ongoing in the biofilter. The addition of azadirachtin to buckets with fully operating biofilter did not show any adverse effects on the nitrification process of biofilter bacteria, except at the sampling point after 6h of exposure. This is in contrast with the pilot study that a similar group of authors already published using the same experimental design, in which buckets supplied with azadirachtin constantly showed higher concentrations of ammonia during the first step of nitrification compared to control (Rašković et al., 2021). The probable reason for the conflicting result is the concentration of the azadirachtin since, in the mentioned study; the nominal concentration was 20 µg L-1, higher than in the present study. However, a slight reduction in Nitrospirae levels (an essential bacteria phylum in the nitrite-oxidizing process) was noted six hours after azadirachtin application in all the treatments. Thus, azadirachtin reduced nitrifying bacteria but not enough to stop nitrification and collapse the system. There are currently no studies on the influence of azadirachtin on nitrifying bacteria in water or nutrient solutions; however, similar effects have been reported in soil nitrification and nitrogen fixation studies. Singh et al. (2015) identified azadirachtin as the major contributor to the suppression of the growth of 49-99% of the nitrogen fixers in the soil after treating 1kg of soil with 1.13 mg of azadirachtin for 30, 50, and 80 days.
Neem tree seeds (from which azadirachtin is obtained by extraction) are known for their bactericidal properties, and it is already shown that in a culture of rohu carp (Labeo rohita) fed with neem seed cake, the concentrations of ammonia, nitrites and nitrates in the water increased compared to control (Das et al., 2018). This is confirmed by quantifying the number of nitrifying bacteria, which also dropped, at least during the first 60 days of feeding fish with neem seed cake. In the same study, the dose-dependent effect of toxicity to bacteria is shown since fish fed with higher incorporation of neem seed in the diet lived in water with increased concentrations of ammonia, nitrites, and nitrates (Das et al., 2018), and this is the probable answer for no effects shown in the present study. To the authors’ knowledge, there were no more studies on the effect of azadirachtin on the biofilter bacteria, but the effects of azadirachtin were tested to nitrifying soil bacteria, and published results were also in discrepancy. Some reported a robust inhibitory effect of azadirachtin on an abundance of AOB soil communities (Gopal et al., 2007; Singh et al., 2015), while others are even reporting the stimulatory effect of azadirachtin on both microbial abundance and diversity for AOA and AOB soil microflora (Suciu et al., 2019). The differences in soil were explained mostly by different soil niches (Suciu et al., 2019), which is not applicable to biofilter bacterial communities. Moreover, biofilter communities in aquaponics are well known and described (Eck et al., 2019; Kasozi et al., 2021); and the microbial diversities vary in different compartments of the aquaponics system (Schmautz et al., 2017; Schmautz et al., 2022).
4.3 Effects of azadirachtin on Nile tilapia
One of the known adverse effects of pesticides during chronic exposures is in reducing weight of fish (Stanley & Preetha, 2016). Even though the aim of exposure was not to evaluate weight gain during 14 days of the fish trial, we have to emphasize that fish exposed to insecticide experienced reduced average body mass by approximately 20% compared to the control group after a recovery period. Average body mass did not differ significantly from the control group due to the large variability, but future studies should be focused on this fact since aquaponics aim to obtain optimal fish growth for commercial reasons.
The mechanism of toxicity of azadirachtin in animals is well established: it reduces RNA synthesis and cell proliferation by blocking the formation of microtubules in insects and mammals (Salehzadeh et al., 2003; Morgan, 2009). It is also proven that azadirachtin have genotoxic effects in the Mozambique tilapia (Oreochromis mossambicus) (Chandra & Khuda-Bukhsh, 2004) and that it modulates hormonal status in common carp (Korkmaz & Örün, 2022). Concerning effects on the hematological status of different fish exposed to azadirachtin in the present study, increased number of RBC and decreased values of MCV and leukocytes after exposure in the highest concentration (AZA 8) is mostly in line with other studies. Common carp exposed in acute (96h) test to 40 and 60 µL L−1 of azadirachtin showed decreased values of MCV (Murussi et al., 2016a), and the same was shown in goldfish (Carassius auratus) after using high concentrations of azadirachtin solution (10-20 mg L-1) as antiparasitic agent (Kumar et al., 2013). A decrease in MCV and higher levels of RBC indicate impaired oxygen transport functions. However, the levels of MCV and RBC were back to normal after the 14 days of recovery, indicating the mildness of the effect. On the other hand, leukocyte levels showed decreased values even in the recovery period, which can impair the immune system in the long-term period (Yang et al., 2021), even after exposure to 6.5 µg L−1 of azadirachtin.
In the blood biochemistry analysis, blood glucose, plasma ammonia, creatine kinase and lactate levels were significantly elevated compared to the control group after 7 days of exposure, but no significant differences were recorded after recovery. This result is in line with Oyoo-Okoth et al. (2011), where authors exposed Nile tilapia (Oreochromis niloticus) to high concentrations of azadirachtin ranging from 500 to 3600 mg L-1. Blood glucose and plasma ammonia increased throughout the entire duration of the experiment (96h). This phenomenon is a typical response to pesticide exposure in fish, such as common carp exposure to simazine (Velisek et al., 2009), metribuzin (Velisek et al., 2009) or formulation containing a mixture of terbuthylazine and S-metolachlor (Dobšíková et al., 2012). Creatine kinase is already proposed as an alternative biomarker of pesticide toxicity in mammals (Bhattacharyya et al., 2011) and is known to be involved in the metabolism of nitrogen, more exact, in the excretion of nitrogen waste, apart from dominant ammonia (Randall, 2011). Hyperammonemia in fish blood results from altered physiological processes in the liver, which fails to metabolize ammonia (Dobšíková et al., 2012)
Concentrations of antioxidant enzymes are typically altered upon fish exposure to azadirachtin, and they depend on fish species, used concentration, and trial duration (Winkaler et al., 2007; Plhalova et al., 2018). However, in the present study, the response was minimal and the only significant and consistent parameter was lipid peroxidation in liver in all three used insecticide concentrations after 7 days of exposure. This points out to cell injury in hepatocytes caused by free radicals and toxicity of azadirachtin, which is already showed in acute and chronic exposures to rainbow trout (Alak et al., 2017) and neotropical fish - piava (Megaleporinus obtusidens) (Glusczak et al., 2011).
Studies on effects of azadirachtin on fish has shown that it induces histopathological alterations in liver of the stinging catfish (Heteropneustes fossilis) (Kumar et al., 2013), as well as in gills of the common carp (Murussi et al., 2016b) and Prochilodus lineatus (Winkaler et al., 2007), which is in contrast with findings in the present study. However, in all mentioned studies, concentrations azadirachtin used for exposure were higher: (1) 10.47 mg L−1 in chronic, 4 weeks trial; (2) 40-60 µL L−1 in acute, 96h test; (3) 2.5-7.5 g L-1 in acute, 24h trial, which can explain observed histopathology in target organs.
5 Conclusion
To the author’s knowledge, this is the first study that aimed to assess risk of use of botanical/microbial insecticides to all three components of an aquaponics system. Although these insecticides are commonly used, it is not easy to find any guidance to aquaponics setup, even though there is a substantial difference between hydroponic and aquaponics systems. Evaluation from the present study showed that the use of pyrethrum and spinosad do not pose a risk affecting the fish in the aquaponics setup, at least not in the chosen combination of plant/fish species (basil/Nile tilapia). However, slight caution has to be taken into consideration, as both insecticides are very unstable and it is very hard to measure their concentrations in the water properly. On the other hand, azadirachtin showed mild adverse effects on fish at the highest measured concentration after one foliar application. Even on the lowest tested concentration (relevant concentration, determined in the real-life scenario), lipid peroxidation in liver and drop of leukocytes in the fish blood were detected. Therefore, caution when azadirachtin is used and more studies on this topic are recommended in the future.
Data availability statement
The data presented in the study are deposited in the EBI repository, accession number PRJEB56899.
Ethics statement
The animal study was reviewed and approved by Institutional Animal Care and Use Committee (IACUC) of the University of South Bohemia based on the EU harmonized Animal Welfare Act of the Czech Republic and approved by the Departmental Expert Committee for Authorization of Experimental Projects of the Ministry of Education, Youth, and Sports of the Czech Republic (permit MSMT-6744/2018-4).
Author contributions
Conceptualization – BR, JM; Formal analysis – BR, RGe, JV, AB, RGr, JM; Funding acquisition – BR, JM; Investigation – BR, RGe, EF, GB, JV, PD, AB, RGr; Project administration – JM; Resources – JV, RGr, JM; Visualization – BR, RGe, EF; Writing – BR, EF; Writing - review & editing – JV, RGe, JM. All authors contributed to the article and approved the submitted version.
Funding
This research has received funding from the European Union’s Horizon 2020 research and innovation programme under grant agreement No. 652831 (AQUAEXCEL2020). This output reflects only the author’s view and the European Union cannot be held responsible for any use that may be made of the information contained therein. Additional support for the research was received by the agreement between University of Belgrade - Faculty of Agriculture and Ministry of Education, Science and Technological Development of Serbia [grant number 451-03-68/2022-14/200116] and CENAKVA VVI Research Infrastructure (ID LM2018099, Ministry of Education, Youth and Sports of the Czech Republic, 2019−2022).
Conflict of interest
The authors declare that the research was conducted in the absence of any commercial or financial relationships that could be construed as a potential conflict of interest.
Publisher’s note
All claims expressed in this article are solely those of the authors and do not necessarily represent those of their affiliated organizations, or those of the publisher, the editors and the reviewers. Any product that may be evaluated in this article, or claim that may be made by its manufacturer, is not guaranteed or endorsed by the publisher.
Supplementary Material
The Supplementary Material for this article can be found online at: https://www.frontiersin.org/articles/10.3389/fmars.2022.1055560/full#supplementary-material
References
Alak G., Ucar A., Parlak V., Yeltekin A.Ç., Taş I. H., Ölmez D., et al. (2017). Assessment of 8-hydroxy-2-deoxyguanosine activity, gene expression and antioxidant enzyme activity on rainbow trout (Oncorhynchus mykiss) tissues exposed to biopesticide. Comp. Biochem. Physiol. Part C: Toxicol. Pharmacol. 203, 51–58. doi: 10.1016/j.cbpc.2017.10.007
Antonious G. F., Byers M. E., Kerst W. C. (1997). Residue levels of pyrethrins and piperonyl butoxide in soil and runoff water. J. Environ. Sci. Health Part B 32 (5), 621–644. doi: 10.1080/03601239709373106
APHA (1989). WPCF, standard Methods for the examination of water and wastewater (17th edit.). Washington DC, USA.
Baganz G. F. M., Junge R., Portella M. C., Goddek S., Keesman K. J., Baganz D., et al. (2022). The aquaponic principle–it is all about coupling. Rev. Aquaculture 14 (1), 252–264. doi: 10.1111/raq.12596
Barden G. (1998). Evaluation of the new active spinosad in the products laser naturalyte insect control and tracer naturalyte insect control (Canberra., Australia: NRA (National Registration Authority for Agricultural and Veterinary Chemical).
Becke C., Schumann M., Steinhagen D., Rojas-Tirado P., Geist J., Brinker A. (2019). Effects of unionized ammonia and suspended solids on rainbow trout (Oncorhynchus mykiss) in recirculating aquaculture systems. Aquaculture 499, 348–357. doi: 10.1016/j.aquaculture.2018.09.048
Bergstrom D. M., Sharman A., Shaw J. D., Houghton M., Janion-Scheepers C., Achurch H., et al. (2018). Detection and eradication of a non-native collembola incursion in a hydroponics facility in East Antarctica. Biol. Invasions 20 (2), 293–298. doi: 10.1007/s10530-017-1551-9
Bhattacharyya K., Phaujdar S., Sarkar R., Mullick O. S. (2011). Serum creatine phosphokinase: A probable marker of severity in organophosphorus poisoning. Toxicol. Int. 18 (2), 117. doi: 10.4103/0971-6580.84263
Bittsanszky A., Gyulai G., Junge R., Schmautz Z., Komives T. (2017). Plant protection in ecocycle-based agricultural systems: Aquaponics as an example. Int. Plant Prot. Congress (IPPC) 1, 2–3.
Borik A., Staňová A. V., Brooks B. W., Grabicová K., Randák T., Grabic R. (2020). Determination of citalopram in fish brain tissue: Benefits of coupling laser diode thermal desorption with low-and high-resolution mass spectrometry. Analytical Bioanalytical Chem. 412 (18), 4353–4361. doi: 10.1007/s00216-020-02672-y
Carlberg I., Mannervik B. (1975). Purification and characterization of the flavoenzyme glutathione reductase from rat liver. J. Biol. Chem. 250 (14), 5475–5480. doi: 10.1016/S0021-9258(19)41206-4
Chandra P., Khuda-Bukhsh A. R. (2004). Genotoxic effects of cadmium chloride and azadirachtin treated singly and in combination in fish. Ecotoxicology Environ. Saf. 58 (2), 194–201. doi: 10.1016/j.ecoenv.2004.01.010
Cosgrove S., Jefferson B., Jarvis P. (2019). Pesticide removal from drinking water sources by adsorption: A review. Environ. Technol. Rev. 8 (1), 1–24. doi: 10.1080/21622515.2019.1593514
Das S. K., Das S., Mandal A., Dinda R. (2018). Impact of neem ( Azadirachta indica a. juss) seed cake upon selective nutrient cycling bacterial population in fish culture. J. Appl. Aquaculture 30 (4), 353–365. doi: 10.1080/10454438.2018.1513887
Deardorff A. D., Stark J. D. (2009). Acute toxicity and hazard assessment of spinosad and r-11 to three cladoceran species and coho salmon. Bull. Environ. Contamination Toxicol. 82 (5), 549–553. doi: 10.1007/s00128-009-9643-6
Dobšíková R., Blahová J., Modrá H., Škorič M., Svobodová Z. (2012). The effect of acute exposure to herbicide gardoprim plus gold 500 SC on haematological and biochemical indicators and histopathological changes in common carp (Cyprinus carpio l.). Acta Veterinaria Brno 80 (4), 359–363. doi: 10.2754/avb201180040359
Eck M., Sare A. R., Massart S., Schmautz Z., Junge R., Smits T. H. M., et al. (2019). Exploring bacterial communities in aquaponic systems. Water 11 (2), 260. doi: 10.3390/w11020260
Folorunso E. A., Roy K., Gebauer R., Bohatá A., Mraz J. (2021). Integrated pest and disease management in aquaponics: A metadata-based review. Rev. Aquaculture 13 (2), 971–995. doi: 10.1111/raq.12508
Glusczak L., Loro V. L., Pretto A., Moraes B. S., Raabe A., Duarte M. F., et al. (2011). Acute exposure to glyphosate herbicide affects oxidative parameters in piava (Leporinus obtusidens). Arch. Environ. Contamination Toxicol. 61 (4), 624–630. doi: 10.1007/s00244-011-9652-4
Gopal M., Gupta A., Arunachalam V., Magu S. P. (2007). Impact of azadirachtin, an insecticidal allelochemical from neem on soil microflora, enzyme and respiratory activities. Bioresource Technol. 98 (16), 3154–3158. doi: 10.1016/j.biortech.2006.10.010
Hammer Ø., Harper D. A., Ryan P. D. (2001). PAST: Paleontological statistics software package for education and data analysis. Palaeontologia Electronica 4 (1), 9.
Isman M. B. (2020). Botanical insecticides in the twenty-first century–fulfilling their promise? Annu. Rev. Entomology 65 (1), 233–249. doi: 10.1146/annurev-ento-011019-025010
Kasozi N., Abraham B., Kaiser H., Wilhelmi B. (2021). The complex microbiome in aquaponics: Significance of the bacterial ecosystem. Ann. Microbiol. 71 (1), 1. doi: 10.1186/s13213-020-01613-5
Khan G. A., Lindberg R., Grabic R., Fick J. (2012). The development and application of a system for simultaneously determining anti-infectives and nasal decongestants using on-line solid-phase extraction and liquid chromatography–tandem mass spectrometry. J. Pharm. Biomed. Anal. 66, 24–32. doi: 10.1016/j.jpba.2012.02.011
Kolarova J., Velisek J. (2012). Determination and assessment of the biochemical profile of fish blood Vodňany, Czech Republic. (Czech: Faculty of Fisheries and Protection of Waters).
Korkmaz N., Örün İ. (2022). Effects of pesticide NeemAzal-T/S on thyroid, stress hormone and some cytokines levels in freshwater common carp, cyprinus carpio l. Toxin Rev. 41 (2), 496–505. doi: 10.1080/15569543.2021.1895841
Kreutzweiser D., Thompson D., Grimalt S., Chartrand D., Good K., Scarr T. (2011). Environmental safety to decomposer invertebrates of azadirachtin (neem) as a systemic insecticide in trees to control emerald ash borer. Ecotoxicology Environ. Saf. 74 (6), 1734–1741. doi: 10.1016/j.ecoenv.2011.04.021
Kruve A., Rebane R., Kipper K., Oldekop M.-L., Evard H., Herodes K., et al. (2015). Tutorial review on validation of liquid chromatography–mass spectrometry methods: Part I. Analytica Chimica Acta 870, 29–44. doi: 10.1016/j.aca.2015.02.017
Kumar S., Raman R. P., Kumar K., Pandey P. K., Kumar N., Mallesh B., et al. (2013). Effect of azadirachtin on haematological and biochemical parameters of argulus-infested goldfish carassius auratus (Linn. 1758). Fish Physiol. Biochem. 39 (4), 733–747. doi: 10.1007/s10695-012-9736-8
Lawrence R. A., Burk R. F. (1976). Glutathione peroxidase activity in selenium-deficient rat liver. Biochem. Biophys. Res. Commun. 71 (4), 952–958. doi: 10.1016/0006-291X(76)90747-6
Lushchak V. I., Bagnyukova T. V., Lushchak O. V., Storey J. M., Storey K. B. (2005). Hypoxia and recovery perturb free radical processes and antioxidant potential in common carp (Cyprinus carpio) tissues. Int. J. Biochem. Cell Biol. 37 (6), 1319–1330. doi: 10.1016/j.biocel.2005.01.006
Lykogianni M., Bempelou E., Karamaouna F., Aliferis K. A. (2021). Do pesticides promote or hinder sustainability in agriculture? the challenge of sustainable use of pesticides in modern agriculture. Sci. Total Environ. 795, 148625. doi: 10.1016/j.scitotenv.2021.148625
Marklund S., Marklund G. (1974). Involvement of the superoxide anion radical in the autoxidation of pyrogallol and a convenient assay for superoxide dismutase. Eur. J. Biochem. 47 (3), 469–474. doi: 10.1111/j.1432-1033.1974.tb03714.x
Morgan E. D. (2009). Azadirachtin, a scientific gold mine. Bioorganic Medicinal Chem. 17 (12), 4096–4105. doi: 10.1016/j.bmc.2008.11.081
Murussi C. R., Menezes C. C., Nunes M. E. M., Araújo M., do C. S., Quadros V. A., et al. (2016a). Azadirachtin, a neem-derived biopesticide, impairs behavioral and hematological parameters in carp (Cyprinus carpio). Environ. Toxicol. 31 (11), 1381–1388. doi: 10.1002/tox.22143
Murussi C. R., Costa M. D., Leitemperger J. W., Flores-Lopes F., Menezes C. C., Loebens L, et al. (2016b). Acute exposure to the biopesticide azadirachtin affects parameters in the gills of common carp (Cyprinus carpio). Comp. Biochem. Physiol. C Toxicol. Pharmacol. 180 , 49–55. doi: 10.1016/j.cbpc.2015.12.003
OECD (2019). Test no. 203: Fish, acute toxicity test, OECD guidelines for the testing of chemicals, section 2 (Paris: OECD Publishing). doi: 10.1787/9789264069961-en
Oyoo-Okoth E., Ngugi C. C., Chepkirui-Boit V. (2011). Physiological and biochemical responses of Nile tilapia (Oreochromis niloticus) exposed to aqueous extracts of neem (Azadirachta indica). J. Appl. Aquaculture 23 (2), 177–186. doi: 10.1080/10454438.2011.581588
Palm H. W., Knaus U., Appelbaum S., Goddek S., Strauch S. M., Vermeulen T., et al. (2018). Towards commercial aquaponics: A review of systems, designs, scales and nomenclature. Aquaculture Int. 26 (3), 813–842. doi: 10.1007/s10499-018-0249-z
Palm H. W., Knaus U., Appelbaum S., Strauch S. M., Kotzen B. (2019). “Coupled aquaponics systems,” in Aquaponics food production systems (Cham: Springer International Publishing), 163–199.
Pavela R., Benelli G. (2016). Essential oils as ecofriendly biopesticides? challenges and constraints. Trends Plant Sci. 21 (12), 1000–1007. doi: 10.1016/j.tplants.2016.10.005
Pereira B. B., Caixeta E. S., Freitas P. C., Santos V. S. V., Limongi J. E., de Campos Júnior E. O., et al. (2016). Toxicological assessment of spinosad: Implications for integrated control of aedes aegypti using larvicides and larvivorous fish. J. Toxicol. Environ. Health Part A 79 (12), 477–481. doi: 10.1080/15287394.2016.1176974
Plhalova L., Blahova J., Divisova L., Enevova V., Casuscelli di Tocco F., Faggio C., et al. (2018). The effects of subchronic exposure to NeemAzal T/S on zebrafish (Danio rerio). Chem. Ecol. 34 (3), 199–210. doi: 10.1080/02757540.2017.1420176
Randall D. J. (2011). “NITROGENOUS-WASTE BALANCE | excretion of ammonia,” in Encyclopedia of fish physiology. Ed. Farrell A. P. (San Diego: Academic Press), 1437–1443. doi: 10.1016/B978-0-12-374553-8.00032-0
Rašković B., Dvořák P., Mráz J. (2021). Effects of biodegradable insecticides on biofilter bacteria: Implications for aquaponics. Turkish J. Fisheries Aquat. Sci. 21 (04), 169–177. doi: 10.4194/1303-2712-v21_4_02
Reddy P. P. (2016). “Insects pests and their management,” in Sustainable crop protection under protected cultivation. Ed. Reddy P. P. (Singapore: Springer), 187–206. doi: 10.1007/978-981-287-952-3_16
Saleem M. S., Batool T. S., Akbar M. F., Raza S., Shahzad S. (2019). Efficiency of botanical pesticides against some pests infesting hydroponic cucumber, cultivated under greenhouse conditions. Egyptian J. Biol. Pest Control 29 (1), 37. doi: 10.1186/s41938-019-0138-4
Salehzadeh A., Akhkha A., Cushley W., Adams R. L. P., Kusel J. R., Strang R. H. C. (2003). The antimitotic effect of the neem terpenoid azadirachtin on cultured insect cells. Insect Biochem. Mol. Biol. 33 (7), 681–689. doi: 10.1016/S0965-1748(03)00057-2
Schmautz Z., Graber A., Jaenicke S., Goesmann A., Junge R., Smits T. H. M. (2017). Microbial diversity in different compartments of an aquaponics system. Arch. Microbiol. 199 (4), 613–620. doi: 10.1007/s00203-016-1334-1
Schmautz Z., Walser J.-C., Espinal C. A., Gartmann F., Scott B., Pothier J. F., et al. (2022). Microbial diversity across compartments in an aquaponic system and its connection to the nitrogen cycle. Sci. Total Environ. 852, 158426. doi: 10.1016/j.scitotenv.2022.158426
Singh S., Gupta R., Kumari M., Sharma S. (2015). Nontarget effects of chemical pesticides and biological pesticide on rhizospheric microbial community structure and function in vigna radiata. Environ. Sci. pollut. Res. 22 (15), 11290–11300. doi: 10.1007/s11356-015-4341-x
Solliec M., Massé D., Sauvé S. (2014). Analysis of trimethoprim, lincomycin, sulfadoxin and tylosin in swine manure using laser diode thermal desorption-atmospheric pressure chemical ionization-tandem mass spectrometry. Talanta 128, 23–30. doi: 10.1016/j.talanta.2014.04.023
Stanley J., Preetha G. (2016). “Pesticide toxicity to fishes: Exposure, toxicity and risk assessment methodologies,” in Pesticide toxicity to non-target organisms. Eds. Stanley J., Preetha G. (Netherlands: Springer), 411–497. doi: 10.1007/978-94-017-7752-0_7
Stouvenakers G., Dapprich P., Massart S., Jijakli M. H. (2019). “Plant pathogens and control strategies in aquaponics,” in Aquaponics food production systems: Combined aquaculture and hydroponic production technologies for the future. Eds. Goddek S., Joyce A., Kotzen B., Burnell G. M. (Cham, Switzerland: Springer International Publishing), 353–378. doi: 10.1007/978-3-030-15943-6_14
Suciu N., Vasileiadis S., Puglisi E., Pertile G., Tourna M., Karas P.A., et al. (2019). Azadirachtin and trifloxystrobin had no inhibitory effects on key soil microbial functions even at high dose rates. Appl. Soil Ecol. 137, 29–38. doi: 10.1016/j.apsoil.2019.01.016
Svobodova Z., Pravda D., Palackova J. (1991). “Unified methods of haematological examination of fish,” in Vodnany: Research institute of fish culture and hydrobiology; edition methods, vol. 20. , 31.
Tahar A., Choubert J. M., Miège C., Esperanza M., Le Menach K., Budzinski H., et al. (2014). Removal of xenobiotics from effluent discharge by adsorption on zeolite and expanded clay: An alternative to activated carbon? Environ. Sci. pollut. Res. 21 (8), 5660–5668. doi: 10.1007/s11356-013-2439-6
Thaochan N., Ngampongsai A., Prabhakar C. S., Hu Q. (2021). Beauveria bassiana PSUB01 simultaneously displays biocontrol activity against lipaphis erysimi (Kalt.)(Hemiptera: Aphididae) and promotes plant growth in Chinese kale under hydroponic growing conditions. Biocontrol Sci. Technol. 31 (10), 997–1015.
Ujváry I. (2010). “Pest control agents from natural products,” in Handbook of pesticide toxicology (New York, United State: Academic Press), vol. 111. doi: 10.1080/09583157.2021.1917512
van Kessel M. A. H. J., Speth D. R., Albertsen M., Nielsen P. H., Op den Camp H. J. M., Kartal B., et al. (2015). Complete nitrification by a single microorganism. Nature 528 (7583), 555–559. doi: 10.1038/nature16459
van Leeuwen T., Dermauw W., Van De Veire M., Tirry L. (2005). Systemic use of spinosad to control the two-spotted spider mite (Acari: Tetranychidae) on tomatoes grown in rockwool. Exp. Appl. Acarology 37 (1–2), 93–105. doi: 10.1007/s10493-005-0139-8
Velisek J., Stastna K., Sudova E., Turek J., Svobodova Z. (2009). Effects of subchronic simazine exposure on some biometric, biochemical, hematological and histopathological parameters of common carp (Cyprinus carpio l.). Neuro Endocrinol. Lett. 30 Suppl 1, 236–241. doi: 10.1007/s00128-009-9648-1
Velisek J., Svobodova Z., Piackova V., Sudova E. (2009). Effects of acute exposure to metribuzin on some hematological, biochemical and histopathological parameters of common carp (Cyprinus carpio l.). Bull. Environ. Contamination Toxicol. 82 (4), 492–495. doi: 10.1007/s00128-009-9648-1
Winkaler E. U., Santos T. R. M., Machado-Neto J. G., Martinez C. B. R. (2007). Acute lethal and sublethal effects of neem leaf extract on the neotropical freshwater fish prochilodus lineatus. Comp. Biochem. Physiol. Part C: Toxicol. Pharmacol. 145 (2), 236–244. doi: 10.1016/j.cbpc.2006.12.009
Wisler G. C., Norris R. F. (2005). Interactions between weeds and cultivated plants as related to management of plant pathogens. Weed Sci. 53 (6), 914–917. doi: 10.1614/WS-04-051R.1
Wongkiew S., Park M.-R., Chandran K., Khanal S. K. (2018). Aquaponic systems for sustainable resource recovery: Linking nitrogen transformations to microbial communities. Environ. Sci. Technol. 52 (21), 12728–12739. doi: 10.1021/acs.est.8b04177
Yang C., Lim W., Song G. (2021). Immunotoxicological effects of insecticides in exposed fishes. Comp. Biochem. Physiol. Part C: Toxicol. Pharmacol. 247, 109064. doi: 10.1016/j.cbpc.2021.109064
Yang T., Stoopen G., Wiegers G., Mao J., Wang C., Dicke M., et al. (2012). Pyrethrins protect pyrethrum leaves against attack by Western flower thrips, frankliniella occidentalis. J. Chem. Ecol. 38 (4), 370–377. doi: 10.1007/s10886-012-0097-7
Yavuzcan Yildiz H., Radosavljevic V., Parisi G., Cvetkovikj A. (2019). “Insight into risks in aquatic animal health in aquaponics,” in Aquaponics food production systems: Combined aquaculture and hydroponic production technologies for the future. Eds. Goddek S., Joyce A., Kotzen B., Burnel G. M. (Cham, Switzerland: Springer International Publishing), 435–452. doi: 10.1007/978-3-030-15943-6_17
Yep B., Zheng Y. (2019). Aquaponic trends and challenges – a review. J. Cleaner Production 228, 1586–1599. doi: 10.1016/j.jclepro.2019.04.290
Keywords: azadirachtin, spinosad, pyrethrum, Nile tilapia, biofilter bacteria, fish exposure, degradation rate
Citation: Rašković B, Gebauer R, Folorunso EA, Božić G, Velíšek J, Dvořák P, Bořík A, Grabic R and Mráz J (2022) Botanical and microbial insecticides application in aquaponics - is there a risk for biofilter bacteria and fish? Front. Mar. Sci. 9:1055560. doi: 10.3389/fmars.2022.1055560
Received: 28 September 2022; Accepted: 31 October 2022;
Published: 22 November 2022.
Edited by:
Marcel Martinez-Porchas, Centro de Investigación en Alimentación y Desarrollo, Consejo Nacional de Ciencia y Tecnología (CONACYT), MexicoCopyright © 2022 Rašković, Gebauer, Folorunso, Božić, Velíšek, Dvořák, Bořík, Grabic and Mráz. This is an open-access article distributed under the terms of the Creative Commons Attribution License (CC BY). The use, distribution or reproduction in other forums is permitted, provided the original author(s) and the copyright owner(s) are credited and that the original publication in this journal is cited, in accordance with accepted academic practice. No use, distribution or reproduction is permitted which does not comply with these terms.
*Correspondence: Jan Mráz, am1yYXpAZnJvdi5qY3UuY3o=