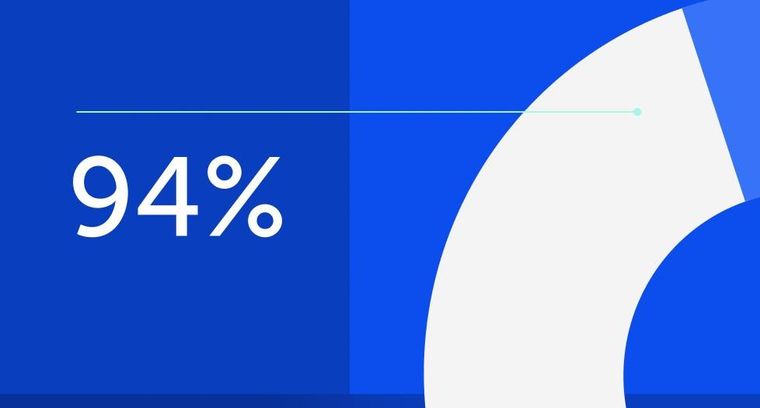
94% of researchers rate our articles as excellent or good
Learn more about the work of our research integrity team to safeguard the quality of each article we publish.
Find out more
ORIGINAL RESEARCH article
Front. Mar. Sci., 24 November 2022
Sec. Coastal Ocean Processes
Volume 9 - 2022 | https://doi.org/10.3389/fmars.2022.1052373
This article is part of the Research TopicAdapting and Building Local Resilience to Sea Level Rise Impacts on CoastlinesView all 9 articles
The increased risk of coastal flooding associated with climate-change driven sea level rise threatens to displace communities and cause substantial damage to infrastructure. Site-specific adaptation planning is necessary to mitigate the negative impacts of flooding on coastal residents and the built environment. Cost-benefit analyses used to evaluate coastal adaption strategies have traditionally focused on economic considerations, often overlooking potential demographic impacts that can directly influence vulnerability in coastal communities. Here, we present a transferable framework that couples hydrodynamic modeling of flooding driven by sea level rise and storm scenarios with site-specific building stock and census block-level demographic data. We assess the efficacy of multiple coastal adaptation strategies at reducing flooding, economic damages, and impacts to the local population. We apply this framework to evaluate a range of engineered, nature-based, and hybrid adaptation strategies for a portion of Santa Monica Bay, California. Overall, we find that dual approaches that provide protection along beaches using dunes or seawalls and along inlets using sluice gates perform best at reducing or eliminating flooding, damages, and population impacts. Adaptation strategies that include a sluice gate and partial or no protection along the beach are effective at reducing flooding around inlets but can exacerbate flooding elsewhere, leading to unintended impacts on residents. Our results also indicate trade-offs between economic and social risk-reduction priorities. The proposed framework allows for a comprehensive evaluation of coastal protection strategies across multiple objectives. Understanding how coastal adaptation strategies affect hydrodynamic, economic, and social factors at a local scale can enable more effective and equitable planning approaches.
Coastal flooding due to sea level rise (SLR) and storm surge poses a significant threat to coastal communities worldwide (Tebaldi et al., 2012), leading to inundation of low-lying areas, displacement of residents, and substantial damage to infrastructure (FitzGerald et al., 2008; Hinkel et al., 2014). While the drivers of coastal flooding may manifest over large scales, the extent and severity of impacts are experienced locally and can differ greatly among locations depending on the local hydrodynamics and the exposure, vulnerability, and resilience of communities and assets (Nastev and Todorov, 2013; Cazenave and Cozannet, 2014). Thus, site-specific planning and preparation are necessary to predict the impacts of coastal flooding and to identify and evaluate effective adaptation approaches. Stakeholder-informed modeling efforts that incorporate local needs, capacities, and practical constraints in the development and evaluation of adaptation options can facilitate decision-making by promoting understanding between the research team and end-users and ensuring that model outputs are accepted, trusted, and relevant (Guerry et al., 2012; Koenigstein et al., 2016).
Historically, coastal flood adaptation has focused on the use of gray infrastructure, including engineered structures such as seawalls, bulkheads, and dikes (Hatheway, 2008). Such built infrastructure provides substantial flood-reduction benefits but is usually costly to build, lacks flexibility to adapt to change, and requires maintenance, repair, and replacement over time (Sovacool, 2011; Powell et al., 2019). Growing populations in coastal regions put stress on already deteriorating infrastructure that has reached the end of its design life, presenting a financial and logistical challenge for communities (Rosenbloom, 2018). Furthermore, gray infrastructure can lead to unintended negative environmental impacts such as the loss of beaches and other natural habitats and alterations in natural sediment dynamics (Chapman and Underwood, 2011). Gray infrastructure can also alter nearshore hydrodynamics, causing tidal amplification and inadvertently shifting coastal flood hazards to other localities (Holleman and Stacey, 2014; Wang et al., 2018; Hummel and Stacey, 2021; Hummel et al., 2021). These unintended consequences may increase the vulnerability of communities to the impacts of SLR (Griffis, 2007; Van Slobbe et al., 2013).
The use of natural and nature-based features (NNBF) has become increasingly popular as a sustainable complement or alternative to gray infrastructure (Sutton-Grier et al., 2018; Storlazzi et al., 2019). NNBF approaches use the features of natural ecosystems like coastal dunes, reefs, and wetlands as effective infrastructure that provides benefits to local communities. Natural features encompass the environmental characteristics of an ecosystem as is, while nature-based features incorporate ecosystem components into man-made designs (Bridges et al., 2015). Like gray infrastructure, NNBF can provide important flood risk reduction benefits (Storlazzi et al., 2019) by reducing the impacts of extreme flow discharges, waves, tides, and coastal surge (Beck et al., 2018; Foster-Martinez et al., 2018; Hartmann et al., 2019). The features have the advantage of often requiring less maintenance than gray infrastructure, and some have proven to naturally recover after disasters with little to no intervention (Adger et al., 2005; Temmerman and Kirwan, 2015; Powell et al., 2019). NNBF can also provide multiple secondary benefits such as water purification, provision of raw materials, and maintenance of biodiversity (Barbier et al., 2011; Sutton-Grier et al., 2015).
Despite the apparent benefits of NNBF, there are also many challenges to their effective implementation. Compared to gray strategies, which begin functioning as soon as they are constructed, habitats and ecosystem services of NNBF usually take longer to become established and effective (Powell et al., 2019). The optimal design and implementation of NNBF for coastal protection are uncertain, and the long-term effectiveness with climate change is unknown (Bridges et al., 2013). NNBF approaches also require site-specific knowledge of the ecological conditions needed to ensure that the infrastructure successfully mitigates coastal flooding (Sutton-Grier et al., 2018).
The evaluation of coastal adaptation strategies often relies on economic cost-benefit analysis that accounts for construction costs and avoided damages to structures (Reguero et al., 2018; Palinkas et al., 2022). However, the vulnerability of different demographic groups and the ability of communities to adapt in response to disasters are also important considerations when deciding how to respond to SLR and coastal flooding (Nastev and Todorov, 2013). Various social factors influence the ability of communities to prepare for, respond to, and recover from flooding. These factors may be demographic (e.g., age, gender, and ethnicity), socioeconomic (e.g., education and employment), and health-related, among other factors like land ownership and risk perception (Rufat et al., 2015). Past studies have found that vulnerable groups experience disproportionate impacts due to flood events (Zahran et al., 2008; Alderman et al., 2012; Rolfe et al., 2020), and increased coastal flooding due to SLR is expected to disproportionately impact low-income communities and people of color (Heberger et al., 2009). Given the disparity in flood vulnerability across populations, demographic factors have been increasingly recognized as a vital part of the decision-making and policymaking surrounding coastal adaptation, and some have recommended consideration of demographics in the funding, design, and implementation stages (McGuire, 2021). However, despite the recognized importance of demographic considerations, their inclusion in formal flood risk assessments, cost-benefit analyses, and other evaluation tools for coastal adaptation remain limited (Martinich et al., 2013; Sutton-Grier et al., 2015). Further research on the interactions between environmental, economic, and social dynamics is imperative and will enable informed decision-making that accounts for multiple priorities.
This study aims to develop an integrated, transferable framework for evaluating the physical, economic, and demographic impacts and benefits of gray and NNBF adaptation strategies in the context of coastal flooding. We apply this framework to the neighboring communities of Marina del Rey and Venice on Santa Monica Bay in Los Angeles County, California, where intensifying coastal flood hazards and growing stakeholder involvement in coastal adaptation provide an illustrative case study to demonstrate the applicability of our approach for local-scale planning. Across Los Angeles County, 60,000 residents and $22 billion in property are at risk of flooding due to SLR and storms over the next century, assuming no interventions (USGS, 2017; Barnard et al., 2019). Our framework consists of a physics-based numerical model, the Coastal Storm Modeling System (CoSMoS) (Barnard et al., 2019), that simulates coastal hydrodynamics and shoreline flooding associated with a range of SLR and storm scenarios and adaptation alternatives identified through stakeholder workshops. The hydrodynamic outputs are then coupled with site-specific building stock data and demographic information to determine the economic and social impacts of each alternative. Finally, the trade-offs and synergies between different alternatives are evaluated to inform local and regional coastal adaptation planning. Overall, this study advances the research on holistic evaluation of coastal adaptation strategies that satisfy stakeholder priorities.
The following sections offer more detail on the case study site, the stakeholder engagement process, the coastal adaptation strategies considered, and the approach for hydrodynamic, economic, and demographic evaluation of the identified strategies.
Santa Monica Bay is located along the Southern California Bight and stretches from the Palo Verdes Peninsula to Point Dume near Malibu. The bay experiences mixed semi-diurnal tides with a mean range of 1.1 m (National Oceanic and Atmospheric Administration, 2022). Ocean waves driven by swell and local winds are the primary contributors to coastal flooding (O’Neill et al., 2018). The coastline surrounding the bay lies within Los Angeles County, the most populous county in the US, and includes miles of engineered, wide sandy beaches that serve as an initial line of defense against rising seas and wave events.
Marina del Rey, an unincorporated community in Los Angeles County, is adjacent to Venice, a neighborhood in the City of Los Angeles. Both are situated within reclaimed tidal marsh in the central part of Santa Monica Bay near the mouth of Ballona Creek (Figure 1). Past studies have found that SLR could significantly impact the residents and infrastructure in these communities (Heberger et al., 2009; Aerts et al., 2018). This is especially true in low-lying Venice, which has an older housing stock, a high percentage of renters, and a network of canals protected from high tides by a tide gate (Grifman et al., 2013; Moffatt & Nichol, 2018). A study by King et al. (2011) on the economic impacts of a 100-year flood with current and future sea levels estimated that with SLR of 1.4 m, the economic damages for Venice would more than double from $7 million to $15.1 million. With sea level in Santa Monica Bay projected to rise by up to 1.96 m under a high emission scenario in the next century and 3.51 m by 2150 (Sweet et al., 2022), additional strategies are needed to protect the area’s coastal infrastructure and residents.
Figure 1 Map of the full hydrodynamic model domain covering Santa Monica Bay, California, and including Marina del Rey and the Venice Canals. The full study area considered for the physical, economic, and demographic impact analysis is outlined by a dashed line.
To inform the development of our modeling framework, we held two workshops with 24 stakeholders with an interest in coastal management in Santa Monica Bay. Workshop participants included three representatives from non-profit organizations, ten from city and county governments, nine from state agencies, and two from federal agencies. In the first workshop, participants identified coastal values that should be considered in adaptation planning, including critical infrastructure such as residences, businesses, transportation, and piers; environmental values such as habitat and natural resource protection and restoration; and underrepresented and underserved communities. Based on these priorities, we identified a need to integrate physical modeling with economic and demographic data to address local stakeholders’ concerns. During the second workshop, stakeholders assisted with the selection and prioritization of locations where the developed framework should be applied, one of which was the Marina del Rey and Venice area.
Based on input from the workshops, we identified and implemented nine stakeholder-prioritized coastal adaptation strategies (Figure 2) within our modeling framework to assess their effectiveness and response to SLR and coastal storms as compared to a no-action scenario. These strategies largely overlapped with approaches outlined in a recent study by Aerts et al. (2018) that developed a collection of adaption pathways for the same study area. Gray adaptation strategies consisted of a seawall along the beach in Venice, a sluice gate at the mouth of the harbor in Marina del Rey, and a combination of the seawall and sluice gate. Aerts et al. (2018) described sluice gates suitable to protect the ports of Los Angeles as a type of tide lock system with movable parts allowing seafarers access to the sea across the entrance while protecting the ports from flooding by maintaining a low water level inside the gates. NNBF approaches included three dune scenarios. The first consisted of a series of targeted sand dunes, ranging in length from 225 to 290 m. These targeted dunes were located where seasonal berms are constructed each winter by the Los Angeles County Department of Beaches and Harbors to reduce winter wave impacts and were modeled with similar dimensions (4.6 m high) (Noble Consultants-G.E.C. Inc, 2016). Two more extensive dune scenarios were also considered, including the construction of sand dunes (4.6 m high) at all beach locations below 4 and 5 m in elevation relative to the NAVD88 vertical datum. The 4-m dune scenario was the lowest elevation where implementing dunes provided any substantial flood reduction benefits. The 5-m dune scenario extended along the entire beach and thus provided the maximum potential reduction in flooding. Finally, the nature-based dune approaches were also combined with the sluice gate strategy to evaluate three additional hybrid alternatives.
Figure 2 Coastal adaptation strategies implemented in the hydrodynamic model, including (A) targeted dunes, (B) dunes in beach locations under 4 m in elevation, (C) dunes in beach locations under 5 m in elevation, and (D) seawall and sluice gate.
We utilized CoSMoS to investigate how a range of gray and NNBF shoreline adaptation approaches influence coastal flooding in the study area. CoSMoS is a physics-based numerical model developed by the US Geological Survey (USGS) using the Delft3D hydrodynamic software to assess coastal flooding exposure as a result of tides, surge, and waves for various SLR and storm scenarios (Barnard et al., 2014; Erikson et al., 2018; O’Neill et al., 2018; Barnard et al., 2019). CoSMoS simulates future storms by deterministically downscaling the WaveWatchIII wave model, TOPEX/Poseidon satellite altimetry-based global tide model, and atmospheric pressure forcing data from global climate model projections. The model resolves hydrodynamics around local topographic features using multiple nested structured grids that allow for local grid refinement where higher resolution is desired (Erikson et al., 2018). Grid resolutions range from 100 m in the outer domain to 8 m in the nested inner domains (O’Neill et al., 2018).
For each adaptation strategy, we modeled daily conditionsand 1-, 20-, and 100-year storm events in combination with a 200 cm SLR scenario based on current projections for an upper estimate of SLR by 2100 (Sweet et al., 2022). The storm event simulations included a 120-hour tide-only spin-up period followed by a 24-hour storm event driven by wind and waves. An existing hydraulic structure at the entrance to the Venice Canals, near the mouth of the harbor, was assumed to be closed during the storm events. Discharge for Ballona Creek, which parallels the harbor entrance channel at its downstream end, was included as a point source at the upper boundary of the modeling domain. A hydrograph derived from regional USGS river gauge data and implemented by CoSMoS was utilized for the discharge time series [O’Neill et al. (2018)]. The seawall and sluice gate strategies were implemented in the model as infinitely high walls at defined velocity points to prevent flow exchange between adjacent grid cells. Dune strategies were modeled by adjusting the topography in specified areas. Hybrid alternatives used a combination of the above approaches.
The magnitude of flooding (i.e., flood volume and area) associated with each scenario was calculated using the maximum flood level in each cell to evaluate the effectiveness of the respective adaptation strategies at reducing flooding. Results are presented for the full study area, which includes the entire extent of the nested inner domains outlined by a dashed line in Figure 1, as well as for the Venice Canals alone.
We applied and advanced the Federal Emergency Management Agency’s (FEMA) Hazus framework to analyze the economic and demographic impacts of flooding and coastal adaptation strategies in our study area. Hazus presents a standardized best management practice that allows hazard mitigation planners to assess the risk of different disasters, including floods, hurricanes, earthquakes, and tsunamis (Schneider and Schauer, 2006), by predicting physical damage as well as economic and social impacts (Nastev and Todorov, 2013). Hazus methodology has been used to estimate flood risk in various areas of North America (Cummings et al., 2012; Nastev and Todorov, 2013; Ghanbari et al., 2020).
For the economic evaluation, we applied FEMA’s Flood Assessment Structure Tool (FAST), which was recently developed under the Hazus program, to calculate building-level flood damages (FEMA, 2018). FAST combines user-provided flood depth and building stock data with depth-damage functions from the Hazus flood model methodology to calculate estimated costs of building loss, content loss, and inventory loss. We used peak water level outputs from the CoSMoS model in raster format to characterize flood depths. For the building data, we used the Nationwide Structure Inventory version 1 dataset (NSI v1) (USACE, 2019), which provides building-specific data on foundation types, total number of stories, first-floor height, building type, occupancy type, and replacement costs derived from features that are observable with remote sensing (Mostafiz et al., 2021). Building locations are not exact but are distributed approximately evenly across a given census block. Building, content, and inventory loss values (in 2021 dollars) from the NSI dataset were summed to estimate the total flood damage cost across storm intensities for each adaptation configuration and were compared to the no-action scenario to quantify the reduction in damages. We also divided the damage results into residential and nonresidential structures to evaluate differential impacts to households and other facilities such as commercial, governmental, educational, religious, industrial, and agricultural classes.
To complement the evaluation of damage reduction benefits provided by each adaptation strategy, we also estimated a range of construction costs for each strategy. This was accomplished by combining high and low unit cost estimates (in 2021 dollars) from previous studies (Dijkman, 2007; Heberger et al., 2009; Aerts et al., 2013; Aerts, 2018) with length or volume estimates derived from the adaptation strategy configurations implemented in the CoSMoS model (Table 1). Seawall costs were calculated per unit length and dune costs per unit volume based on estimates from other California projects (Heberger et al., 2009; Aerts et al., 2018). Recognizing that the cost of a sluice gate will largely depend on the dimensions and complexity of its movable parts (Aerts et al., 2013), we separately estimated the cost of the movable gate and fixed components. The fixed components are levee-type structures that permanently span across waterways and block water discharge. Movable gates are typically constructed with steel or concrete and are closed during extreme coastal flooding events. A site-specific estimate for the movable gate width (25 m) was derived based on the width of largest docked vessel in the harbor together with the need for bi-directional passage through the gate. The remaining channel width (255 m) was assumed to consist of fixed components on either side of the gate. For each adaptation strategy, we then calculated the benefit-cost ratio (BCR) for a single event at each storm intensity level (daily conditions and 1-, 20-, and 100-year storm events) by dividing the avoided economic damages from FAST by the construction cost. We assumed that the event occurred in the year that construction was completed and thus did not apply any dollar value adjustments. This approach is not a formal cost-benefit analysis but still provides an initial idea of the relationship between construction costs and associated damage reduction benefits across the considered strategies.
To assess the demographic impacts and benefits of each adaptation strategy, we expanded upon the Hazus flood model methodology, which only included the total shelter needs and displaced households, by incorporating demographic data from the 2010 Census. We evaluated total population impacts as well as differential impacts to groups that are typically considered vulnerable, including children under the age of 16, seniors over the age of 65, minorities, and households earning less than $20,000 in annual income (Martinich et al., 2013; Rufat et al., 2015; Martin et al., 2022). Minority groups consisted of Black, Native American, Asian, Hispanic, Pacific Islander, and Other races. Using the CoSMoS model outputs, we calculated the percent flooded area of each census block and then applied that percentage to the demographic data for each census block. This approach assumed that the population was distributed evenly across each census block, a reasonable assumption given the small average size of census blocks in the study area (0.02 km2).
Figure 3 shows the total flood area (panels A-B) and flood volume (panels C-D) modeled for each adaptation approach across the range of storm scenarios. Results are presented for the full study area in the left column and for the refined area surrounding the Venice Canals in the right column. Flood maps displaying the maximum floodwater depth and flooding extent under each adaptation strategy are presented in the Supplementary Material (Figures S1-S4). Gray and NNBF approaches targeted along the beach, including the seawall and dune scenarios, are more effective at reducing flood area than flood volume (Figures 3A, C) because flooding along the beachfront is generally shallow but extensive, while flooding around the harbor in Marina del Rey is deeper but confined to a smaller spatial footprint. In fact, flood area increases by over 1,200% in the Venice Canals from the no-storm scenario to the 100-year storm scenario, while it increases by only 39% across the rest of the study domain. Beachfront strategies such as the seawall can reduce flood area by up to 52% but only provide a maximum flood volume reduction of 29% for a 100-year storm. In contrast, the sluice gate option targeted at preventing flooding in the harbor is more effective at reducing flood volume, with a maximum reduction of 95% under the no-storm scenario compared to an 83% reduction in flood area.
Figure 3 Simulated flood area (A, B) and volume (C, D) for each adaptation approach across the range of storm scenarios. Results for the full study area are shown in the left column and the Venice Canals in the right column with bar colors represented by the adaptation approaches. Where bars are not visible, minimal or no flooding was simulated.
For the NNBF strategies, increasing the dune footprint results in greater flood-reduction benefits. For the 100-year storm scenario, the targeted dunes, which have the smallest footprint, reduce the flooded area from the no-action scenario by 10%, while the 4-m dune and 5-m dune strategies reduce the flooded area by 45 and 52%, respectively. The NNBF 5-m dune strategy provides similar flood volume and area reduction benefits as the gray seawall strategy across all storm events. Strategies that combine beachfront protection with sluice gate protection at the entrance to the harbor provide greater flood reduction benefits than their individual components. In fact, the combined seawall plus sluice gate and 5-m dune plus sluice gate strategies essentially eliminate flooding in the study area for all scenarios.
Important contrasts are noted when considering the effects of the adaptation strategies in the Venice Canals area alone (Figures 3B, D), as compared to the entire study area. For example, although the sluice gate provides flood-reduction benefits across all scenarios when aggregated across the entire domain, it is far less effective in the Venice Canals area and actually increases flood area and volume by 9 and 25%, respectively, under the 100-year storm scenario. This occurs because the sluice gate blocks flow into the harbor and redirects floodwaters toward the beach, leading to more extensive flooding. The negative effect of the sluice gate in the Venice Canals is also notable when comparing the targeted dune strategy to the hybrid strategy consisting of the targeted dunes plus the sluice gate. For the 100-year storm event, the addition of the sluice gate increases flood area by 153% and flood volume by 261% compared to the targeted dunes alone. The seawall, 4-m dune, and 5-m dune scenarios and other hybrid approaches are all effective at reducing flooding in the Venice Canals across all storm intensities.
Figure 4 shows the total economic damages for each adaptation approach across the range of storm scenarios (panels A-B) and the percentage deviation in residential and nonresidential damages as compared to the total damages (panels C-F). Results are presented for the full study area in the left column and the Venice Canals area in the right column. Strategies implemented along the beach are less effective at reducing flood damages than strategies that include the sluice gate when viewed across the full study area (Figure 4A). The sluice gate alone reduces economic damages by more than 75% across all storm intensities, over three times as much as the beach-targeted alternatives. Hybrid strategies that include the sluice gate in combination with dunes or a seawall provide a minimum of an 85% reduction in damages across all storm scenarios. Among the beachfront-only strategies, the seawall and 5-m dune scenarios perform best, reducing total economic damages by 20% for the 100-year storm event. The 4-m dune scenario reduces total damages by 18%. The least effective individual strategy is the targeted dune scenario, which only provides a 13% reduction in damages.
Figure 4 Simulated total damages (A, B) and percent differential for residential (C, D) and nonresidential (E, F) structures for each adaptation approach across the range of storm scenarios. Positive bars indicate a higher level of protection for the specified structure type as compared to the entire structure inventory, while negative bars represent a lower level of protection. Results for the full study area are shown in the left column and the Venice Canals in the right column.
In contrast to the results for the full study area, economic damages in the Venice Canals are reduced the most when beach-front adaptation strategies are implemented (Figure 4B). The seawall, 4-m dune, and 5-m dune scenarios reduce damages completely across all storm events. The targeted dune strategy also provides substantial benefits, reducing damages completely for the no-storm event (i.e., daily conditions) and by a minimum of 87% for the storm scenarios. The sluice gate scenario, on the other hand, provides minimal damage reduction in the Venice Canals and actually increases damages by 24% for the 100-year storm event. Hybrid strategies including the targeted dunes plus the sluice gate and the 4-m dunes plus the sluice gate are less effective at reducing economic damages than their beach-focused single feature counterparts for the 100-year storm event. Thus, consistent with the flood results, the sluice gate can exacerbate damages in the Venice Canals.
Damage to residential structures is approximately an order of magnitude higher than damage to nonresidential structures across all storm intensities for the no-action scenario. In the full study area, damage to residential structures accounts for ~ 90% of the total economic damage. Overall, the economic damages to residential and nonresidential structures generally mirror the total economic damage trend across all adaptation and storm scenarios (Figures 4C, E). Most deviations are within 10% of the total damages. However, in the Venice Canals, there are some notable differences (Figures 4D, F). For example, the targeted dunes plus the sluice gate provide 18% less protection against nonresidential damages as compared to total damages for the 100-year storm, while the sluice gate alone provides 12% more protection against nonresidential damages.
Figure 5 shows the total exposed population for each adaptation approach across the range of storm scenarios (panels A-B) and the percentage deviation in exposed residents for each vulnerable group as compared to the total exposed population (panels C-J). Results are presented for the full study area in the left column and for the area surrounding the Venice Canals in the right column. Under the no-action scenario, the total exposed population is 3,544 for the no-storm scenario and increases to 7,437 for the 100-year storm (Figure 5A). Strategies that protect the entire beachfront, including the seawall and the 5-m dune scenario, reduce population impacts by 21% (740 people) for the no-storm scenario. The protective benefits of these strategies increase with increasing storm intensity for a maximum reduction of 55% (4,098 people) for the 100-year storm event. Other beachfront strategies are less effective. Although the 4-m dune scenario performs similarly to the 5-m dune scenario for the no-storm and 1-year storm events, its protective benefits diminish for the more extreme storms (20- and 100-year). The targeted dunes perform the worst of all strategies, reducing population impacts by only 26% for the 100-year storm. The sluice gate strategy targeted at the harbor provides the greatest proportional benefits (80% reduction in exposed population) across all single-strategy options for the no-storm scenario. However, its effectiveness decreases with increasing storm intensity, providing only a 40% reduction in exposed population for the 100-year storm. Hybrid strategies are more effective than their individual counterparts because they target both the beachfront and the harbor. The combined seawall plus sluice gate and 5-m dune plus sluice gate strategies provide complete flood protection for residents. The 4-m dune plus sluice gate scenario also provides substantial benefits, reducing the exposed population by over 97% for the no-storm, 1-year, and 20-year storm scenarios, but it is slightly less effective for the 100-year storm (85% reduction in exposed population). The targeted dune plus sluice gate scenario provides a greater than 80% reduction in exposed population for all storm events except the 100-year storm, for which it only provides a 52% reduction.
Figure 5 Simulated total exposed population (A, B) and percent differential for child (C, D), senior (E, F), minority (G, H), and low-income (I, J) populations for each adaptation approach across the range of storm scenarios. Positive bars indicate a higher level of protection for the specified demographic group as compared to the entire population, while negative bars represent a lower level of protection. Results for the full study area are shown in the left column and the Venice Canals in the right column.
For the Venice Canals area, we observe that the sluice gate performs the worst of the modeled strategies and has the potential to exacerbate population impacts. When implemented alone, the sluice gate increases the exposed population by 10% (219 people) compared to the no-action scenario for a 100-year storm event. When the targeted dunes are implemented in combination with the sluice gate, the protective benefits for the 100-year storm decline from a 72% reduction in exposed population due to the targeted dunes alone to only a 28% reduction for the hybrid strategy, representing exposure of 984 more people. Other beachfront strategies, including the seawall, 4-m dune, and 5-m dune scenarios, provide almost complete protection for residents in the Venice Canals.
Evaluation of the demographic composition of the affected population highlights potential disparate impacts on vulnerable groups within the study area (Figures 5C–J). Across the entire study area, we observe some cases where the relative benefits of an adaptation strategy vary more substantially across demographic groups. For example, the sluice gate strategy provides less benefit (14% less for the 100-year storm) to children as compared to all other demographic groups because children comprise a lower percentage of the population in census blocks that surround the marina versus those along the beach that are not protected by the sluice gate (Figure S5). On the other hand, strategies that provide partial or complete protection along the beach, including the seawall and all dune scenarios, provide higher percentage reductions in exposed children compared to the total population for the 1-, 20-, and 100-year storm scenarios. The largest difference occurs for the targeted dune scenario with a 20-year storm event, which benefits the population of exposed children 18% more than the total population. Although some variations exist among the other demographic groups, none vary by more than 9% from the total population results. In the Venice Canals, the trends seen in the total population are generally mirrored by all four vulnerable demographic groups, although some exceptions are apparent. For example, the population of exposed children benefits 8% more from the sluice gate strategy compared to the overall exposed population in the no-storm scenario, while the population of exposed low-income households benefits 5% less from this strategy.
The previous sections highlighted the hydrodynamic, economic, and demographic impacts between individual strategies. Recognizing the complexity and variation between impacts and strategies, this section aims to assist stakeholders in their decision-making process by providing a combined analysis of the impacts associated with each strategy across storm intensities. Figure 6 and Tables S1-S4 show the percentage reduction in hydrodynamic, economic, and demographic impacts compared to the no-action scenario across all storm intensities. Negative values represent an increase in impacts compared to the no-action scenario. Viewing performance in percentages rather than absolute impact numbers facilitates a comparison of trade-offs between hydrodynamic, economic, and demographic attributes with different magnitudes.
Figure 6 Percentage reduction in hydrodynamic, economic, and demographic impacts of strategies compared to the no-action scenario for (A, B) no storm, (C, D) a 1-year storm, (E, F) a 20-year storm, and (G, H) a 100-year storm. Results for the full study area are shown in the left column and the Venice Canals in the right column. Results above 0% indicate a reduction in flood impact from implementing the adaptation strategy, while results below 0% indicate an increase in flood impact. FA, flood area; FV, flood volume; ET, total economic damages; ER, residential economic damages; EN, nonresidential economic damages; PT, total population; PC, child population; PS, senior population; PM, minority population; PI, low-income population.
The economic performance of each strategy generally follows the pattern of flood volume. For example, the sluice gate strategy reduces flood volume by over 64% across all storm scenarios and results in a corresponding reduction in total economic damages of over 75%. The beach-focused strategies, on the other hand, reduce flood volume by a maximum of only 29% and economic damages by only 20%. In contrast, the demographic performance of the modeled strategies is closely aligned with the trends in flood area. In this case, beach-focused strategies are more effective, as the 5-m dune and seawall scenarios reduce the flood area by over 52% and the number of exposed people by over 55% in the 100-year storm event. In contrast, the sluice gate reduces the flooded area by only 44% and the exposed population by only 40% for the 100-year storm. The hybrid strategies, which generally perform better at reducing both flood volume and area, also provide the greatest benefits in reducing corresponding economic and demographic impacts.
For most individual strategies, performance relative to the no-action scenario increases with increasing storm intensity, leading to greater reductions in impacts across the range of attributes. A notable exception is the sluice gate strategy, which performs worse as storm intensity increases. For example, the sluice gate performance is reduced by more than 31% across demographic attributes, 9% across economic attributes, 39% in flood area impacts, and 31% in flood volume impacts between the no-storm event and the 100-year storm event. The effect of the sluice gate strategy is also visible in the reduced performance of the hybrid strategies with increasing storm intensity.
In the Venice Canals, the beach-front strategies generally reduce both flood area and flood volume and thus provide substantial economic and demographic benefits (>97% reduction in impacts) across all storm events. However, the performance of the targeted dune scenario decreases for the 100-year storm event. Under this scenario, flood volume and economic damage impacts are reduced by only 85 and 87%, respectively, and flood area and total exposed population impacts are reduced by only 71 and 72%, respectively. The sluice gate strategy provides minimal benefits across hydrodynamic, economic, and demographic attributes, and in some scenarios exacerbates damages above those observed for the no-action scenario. The targeted dune plus sluice gate scenario also leads to poorer performance across all attributes.
Using the unit cost ranges and modeled dimensions from Table 1, we estimated the range of total costs associated with constructing each strategy (Figure 7A). The gray infrastructure strategies have higher construction costs than the NNBF strategies. This is particularly prevalent when comparing the cost of the 5-m dune and seawall strategies, which are similar in extent and performance. The low range construction cost of the seawall is approximately 15 times that of the 5-m dune strategy, while the high range cost of the seawall is over 9 times that of the 5-m dune strategy. Hybrid strategies that combine NNBF strategies with the sluice gate are still less expensive than the upper-range estimate for the seawall strategy alone.
Figure 7 Estimated (A) cost ranges and (B) benefit-cost ratios across storm intensities for adaptation scenarios, as explained in the text. The dashed gray lines in panel (B) show a benefit-cost ratio of one.
The ranges of BCRs for the no-storm and 1-, 20-, and 100-year storm scenarios are presented in Figure 7B. As expected, BCRs increase with increasing storm intensity due to the higher level of avoided damages provided. For the seawall and seawall plus sluice gate scenarios, the BCR never exceeds one, indicating that the cost of these strategies cannot be recovered with a single event. When considering low-end construction cost estimates, the 4-m dune and 5-m dune scenarios and dune plus sluice scenarios provide BCRs slightly above one for higher storm intensities. The targeted dune scenario generally provides the highest BCRs (up to 9.3) due to its low construction cost and moderate flood reduction benefits. In fact, if high-end cost estimates are assumed across all strategies, only the targeted dune scenario provides benefits that exceed the cost of construction, with BCRs of 1.3, 1.6, and 2.5 for the 1-, 20-, and 100-year storms, respectively.
Our analysis of adaptation alternatives demonstrates the potential for certain strategies (i.e., the sluice gate) to shift flood hazards to other areas, particularly at higher storm intensities. This highlights two important requirements for adaptation planning and analysis: (1) careful delineation of the study region considered for cost-benefit analysis based on the potential extent of impacts and (2) quantification of variations in impacts (e.g., at the neighborhood scale) within the study region. If the study region and associated modeling domain is too narrowly defined, impacts to neighboring areas may not be accounted for. On the other hand, if the study region is broadly defined but impacts are aggregated only across the entire area, disparate effects on certain neighborhoods or populations may be overlooked. By modeling the hydrodynamic feedbacks between Marina del Rey and the Venice Canals and calculating impacts at a refined scale, our approach identifies undesirable impacts in the Venice Canals due to the sluice gate strategy that cannot be observed when considering the full study area. Further extension and refinement of the modeling domain could reveal additional impacts beyond the current study site, as observed in other embayments (Hummel et al., 2021), and could thus inform how adaptation planning in Marina del Rey and the Venice Canals influences flood hazards and exposure in other parts of Santa Monica Bay.
Given the observed feedbacks between actions taken in Marina del Rey, an unincorporated area in Los Angeles County, and the resulting flooding in the Venice Canals, part of the City of Los Angeles, coordination across jurisdictions is an important consideration in the planning and implementation of coastal adaptation strategies. Such planning efforts also require coordination with agencies at the local, state, and federal level that have varying levels of authority over management and adaptation in the coastal zone. These agencies include the Los Angeles County Department of Beaches and Harbors, which has authority over the Marina del Rey harbor, as well as the California Coastal Commission and California Coastal Conservancy, state agencies tasked with implementing the federal Coastal Zone Management Act in California. The modeling results presented here can inform which agencies and stakeholders are brought into planning discussions to address cross-jurisdictional flood hazards.
By integrating hydrodynamic, economic, and demographic factors, our developed framework can facilitate and inform multi-criteria analysis of adaptation alternatives across a range of stakeholder-identified values, such as those that emerged through our project workshops. In Santa Monica Bay, our results indicate trade-offs between priorities for economic damage reduction and population protection. While strategies that include the sluice gate provide the greatest economic benefits across the study region, beach-focused strategies are more effective at reducing population impacts as storm intensity increases. Variations in impacts between demographic groups are also notable, as beach-focused strategies benefit children more than the general population but benefit minority and low-income populations slightly less. Such information can thus provide stakeholders with a more comprehensive view of potential benefits and impacts of adaptation alternatives. This is especially critical in efforts to develop more equitable solutions to coastal hazards because it allows coastal managers and adaptation planners to identify potential disparate and/or disproportionate impacts to vulnerable populations in the planning phase so that these effects can be addressed and mitigated before project implementation.
From a cost-benefit perspective, our results suggest that hybrid strategies that include 4-m or 5-m dune construction coupled with a sluice gate are the most advantageous, as they provide almost complete flood risk reduction at less than a third of the cost of the seawall plus sluice gate strategy. The targeted dune scenario may also be an attractive option if funding is limited due to its high BCR and relatively low cost. While it does not completely eliminate flooding within the study area, the targeted dune scenario still provides a minimum 71% reduction in flood area in the Venice Canals. Although not quantified here, NNBF systems like dunes can also provide a range of secondary benefits, including habitat and recreational/aesthetic value (Sutton-Grier et al., 2015), and may be more palatable to local residents than an extensive sea wall (Palinkas et al., 2022).
We emphasize that this study is a first estimation of the potential impacts and trade-offs between gray and NNBF adaptation options for Marina del Rey and Venice aimed at supporting ongoing planning efforts. Additional work is needed to assess the long-term performance of the dune alternatives, particularly in light of past studies that have shown the project life of NNBF to be limited under anomalous water level and wave conditions (Ludka et al., 2016). In addition, loss of beach width due to sea level rise could constrain the functionality of dune systems and may require beach nourishment to provide a suitable level of protection (Aquarium of the Pacific, 2015; Aerts et al., 2018). Morphological modeling of dune and beach responses across coastal storm intensities could help to inform the comprehensive design of NNBF strategies (Elko et al., 2016). Other factors, such as the impacts of both gray and NNBF strategies on views and access to the beach (Oh et al., 2008) and the effects on drainage of inland runoff (Zoleta-Nantes, 2002), are also likely to influence decisions about shoreline management, and consideration of these factors as part of a thorough planning process may improve overall outcomes.
In addition to the initial construction costs of adaptation alternatives, ongoing operation and maintenance (O&M) costs are also an important consideration for communities. O&M costs can vary substantially between projects based on material/labor costs and storm conditions and will change over time with project age and increased exposure due to SLR (Aerts et al., 2013). Annual O&M costs for dune and beach nourishment were reported as approximately 8–19% of initial construction costs for a coastal risk reduction project in Long Beach, New York (USACE, 2006; Aerts et al., 2013). For gray infrastructure like seawalls and sluice gates, O&M costs are reported as closer to 1–4% of initial costs (Heberger et al., 2009; Aerts et al., 2013). However, given the higher construction costs of the seawall and sluice gate alternatives, annual O&M costs for gray infrastructure may still exceed annual costs for NNBF. Additional location-specific cost analysis is needed to constrain the range of potential O&M costs and to examine how costs may change over the project lifetime.
Although the results of this evaluation of adaptation scenarios for Santa Monica Bay are case-specific, the developed research approach is transferable to other areas facing similar challenges in planning for SLR and coastal storms, as long as adequate building and population data inventories are available. The application of this approach does require upfront investment in the development of a hydrodynamic model capable of capturing nearshore flood processes. However, once developed, the model can be used as part of an iterative process to identify, evaluate, and refine the design of adaptation strategies informed by stakeholder input. Subsequent evaluation of economic and demographic impacts leverages datasets with national coverage and could be further augmented with higher-resolution, local data where available.
In this study, we have developed a transferable framework that couples hydrodynamic modeling of flooding driven by sea level rise and storm scenarios with site-specific building stock and census block-level demographic data to evaluate the effectiveness of a range of engineered, nature-based, and hybrid coastal adaptation strategies at reducing flooding, economic damages, and impacts to the local population in Marina Del Rey and Venice, California. Our findings suggest that dual approaches that provide protection along the beachfront and the harbor, either using fully engineered or hybrid approaches, provide the greatest benefits. In contrast, strategies that prevent water from entering the harbor but provide incomplete or no protection along the beach can exacerbate flooding in beachfront communities, leading to unintended impacts on residents. Our results also indicate trade-offs between economic and social risk-reduction priorities, as strategies that reduce flood volume are most effective at minimizing economic damages, while strategies that reduce flood area are most effective at reducing the number of exposed people. Finally, we note that hybrid strategies can provide cost-savings over fully gray solutions while still maintaining similar flood risk reduction benefits. Overall, the framework presented here allows for the evaluation of adaptation options against a broader range of criteria consistent with stakeholder priorities and thus contributes to a more holistic approach to shoreline adaptation planning.
The raw data supporting the conclusions of this article will be made available by the authors, without undue reservation.
KS, MH, and KB designed the research. KS performed the simulations. KS, MH, and KB analyzed the data. All authors discussed and interpreted the results. KS and MH wrote the paper with input from all authors. All authors approved the submitted manuscript.
This research was supported by NOAA’s National Centers for Coastal Ocean Science Effects of Sea Level Rise Program (NOAA Project Number: NA19NOS4780177).
We would like to thank Andrea O’Neill for assistance with the CoSMoS model, Ashley Hoke for assistance with FEMA’s HAZUS and FAST programs, and Rae Taylor-Burns for providing a review of the manuscript. We used the STAMPEDE2 supercomputer of the Texas Advanced Computing Center (TACC) to run the hydrodynamic models. Any use of trade, firm, or product names is for descriptive purposes only and does not imply endorsement by the U.S. Government.
The authors declare that the research was conducted in the absence of any commercial or financial relationships that could be construed as a potential conflict of interest.
All claims expressed in this article are solely those of the authors and do not necessarily represent those of their affiliated organizations, or those of the publisher, the editors and the reviewers. Any product that may be evaluated in this article, or claim that may be made by its manufacturer, is not guaranteed or endorsed by the publisher.
The Supplementary Material for this article can be found online at: https://www.frontiersin.org/articles/10.3389/fmars.2022.1052373/full#supplementary-material
Adger W. N., Hughes T. P., Folke C., Carpenter S. R., Rockstrom J. (2005). Social-ecological resilience to coastal disasters. Science 309, 1036–1039. doi: 10.1126/science.1112122
Aerts J. C. (2018). A review of cost estimates for flood adaptation. Water 10, 1646. doi: 10.3390/w10111646
Aerts J. C., Barnard P. L., Botzen W., Grifman P., Hart J. F., De Moel H., et al. (2018). Pathways to resilience: Adapting to sea level rise in Los Angeles. Ann. New York Acad. Sci. 1427, 1–90. doi: 10.1111/nyas.13917
Aerts J. C., Botzen W. W., de Moel H., Bowman M. (2013). Cost estimates for flood resilience and protection strategies in new York city. Ann. New York Acad. Sci. 1294, 1–104. doi: 10.1111/nyas.12200
Alderman K., Turner L. R., Tong S. (2012). Floods and human health: A systematic review. Environ. Int. 47, 37–47. doi: 10.1016/j.envint.2012.06.003
Aquarium of the Pacific (2015). City of long beach climate resiliency assessment report. Aquarium of the Pacific, Long Beach, California
Barbier E. B., Hacker S. D., Kennedy C., Koch E. W., Stier A. C., Silliman B. R. (2011). The value of estuarine and coastal ecosystem services. Ecol. Monogr. 81, 169–193. doi: 10.1890/10-1510.1
Barnard P. L., Erikson L. H., Foxgrover A. C., Hart J. A. F., Limber P., O’Neill A. C., et al. (2019). Dynamic flood modeling essential to assess the coastal impacts of climate change. Sci. Rep. 9, 1–13. doi: 10.1038/s41598-019-40742-z
Barnard P. L., van Ormondt M., Erikson L. H., Eshleman J., Hapke C., Ruggiero P., et al. (2014). Development of the coastal storm modeling system (CoSMoS) for predicting the impact of storms on high-energy, active-margin coasts. Natural Hazards 74, 1095–1125. doi: 10.1007/s11069-014-1236-y
Beck M. W., Losada I. J., Menéndez P., Reguero B. G., Díaz-Simal P., Fernández F. (2018). The global flood protection savings provided by coral reefs. Nat. Commun. 9, 1–9. doi: 10.1038/s41467-018-04568-z
Bridges T. S., Burks-Copes K. A., Bates M. E., Collier Z. A., Fischenich J. C., Piercy C. D., et al. (2015). Use of natural and nature-based features (NNBF) for coastal resilience (Vicksburg, Mississippi: US Army Engineer Research and Development Center).
Bridges T., Henn R., Komlos S., Scerno D., Wamsley T., White K. (2013). Coastal risk reduction and resilience: Using the full array of measures. Washington, D.C.: US Army Corps of Engineers Directorate of Civil Works
Cazenave A., Cozannet G. L. (2014). Sea Level rise and its coastal impacts. Earth’s Future 2, 15–34. doi: 10.1002/2013EF000188
Chapman M., Underwood A. (2011). Evaluation of ecological engineering of “armoured” shorelines to improve their value as habitat. J. Exp. Mar. Biol. Ecol. 400, 302–313. doi: 10.1016/j.jembe.2011.02.025
Cummings C. A., Todhunter P. E., Rundquist B. C. (2012). Using the hazus-MH flood model to evaluate community relocation as a flood mitigation response to terminal lake flooding: The case of minnewaukan, north Dakota, USA. Appl. Geogr. 32, 889–895. doi: 10.1016/j.apgeog.2011.08.016
Dijkman J. (2007). A Dutch perspective on coastal Louisiana flood risk reduction and landscape stabilization (Delft, Netherlands: Netherlands Water Partnership Delft).
Elko N., Brodie K., Stockdon H., Nordstrom K., Houser C., McKenna K., et al. (2016). Dune management challenges on developed coasts (Vicksburg, Mississippi: US Army Engineer Research and Development Center).
Erikson L., Barnard P., O’Neill A., Wood N., Jones J., Finzi Hart J., et al. (2018). Projected 21st century coastal flooding in the southern California bight. part 2: tools for assessing climate change-driven coastal hazards and socio-economic impacts. J. Mar. Sci. Eng. 6, 76. doi: 10.3390/jmse6030076
FitzGerald D. M., Fenster M. S., Argow B. A., Buynevich I. V. (2008). Coastal impacts due to sea-level rise. Annu. Rev. Earth Planetary Sci. 36, 601–647. doi: 10.1146/annurev.earth.35.031306.140139
Foster-Martinez M. R., Lacy J. R., Ferner M., Variano E. (2018). Wave attenuation across a tidal marsh in San Francisco bay. Coast. Eng. 136, 26–40. doi: 10.1016/j.coastaleng.2018.02.001
Ghanbari M., Arabi M., Obeysekera J. (2020). Chronic and acute coastal flood risks to assets and communities in southeast Florida. J. Water Resour. Plann. Manage. 146, 04020049. doi: 10.1061/(ASCE)WR.1943-5452.0001245
Griffis F. (2007). Engineering failures exposed by hurricane Katrina. Technol. Soc. 29, 189–195. doi: 10.1016/j.techsoc.2007.01.015
Grifman P., Hart J., Ladwig J., Newton Mann A., Schulhof M. (2013). Sea Level rise vulnerability study for the city of Los Angeles (Los Angeles, California: University of Southern California Sea Grant).
Guerry A. D., Ruckelshaus M. H., Arkema K. K., Bernhardt J. R., Guannel G., Kim C.-K., et al. (2012). Modeling benefits from nature: Using ecosystem services to inform coastal and marine spatial planning. Int. J. Biodiversity Science Ecosystem Serv. Manage. 8, 107–121. doi: 10.1080/21513732.2011.647835
Hartmann T., Slavíková L., McCarthy S. (2019). Nature-based flood risk management on private land: Disciplinary perspectives on a multidisciplinary challenge (New York, New York: Springer Nature).
Hatheway D. J. (2008). Assessment of international coastal flood protection levels for disaster mitigation. In Solutions to Coast. Disasters 2008, 341–348. doi: 10.1061/40968(312)31
Heberger M., Cooley H., Herrera P., Gleick P. H., Moore E. (2009). The impacts of sea-level rise on the California coast (San Diego, California: California Climate Change Center).
Hinkel J., Lincke D., Vafeidis A. T., Perrette M., Nicholls R. J., Tol R. S., et al. (2014). Coastal flood damage and adaptation costs under 21st century sea-level rise. Proc. Natl. Acad. Sci. 111, 3292–3297. doi: 10.1073/pnas.1222469111
Holleman R. C., Stacey M. T. (2014). Coupling of sea level rise, tidal amplification, and inundation. J. Phys. Oceanography 44, 1439–1455. doi: 10.1175/JPO-D-13-0214.1
Hummel M. A., Griffin R., Arkema K., Guerry A. D. (2021). Economic evaluation of sea-level rise adaptation strongly influenced by hydrodynamic feedbacks. Proc. Natl. Acad. Sci. 118, e2025961118. doi: 10.1073/pnas.2025961118
Hummel M. A., Stacey M. T. (2021). Assessing the influence of shoreline adaptation on tidal hydrodynamics: The role of shoreline typologies. J. Geophysical Research: Oceans 126, e2020JC016705. doi: 10.1029/2020JC016705
King P. G., McGregor A. R., Whittet J. D. (2011). The economic costs of sea-level rise to California beach communities (Sacramento, California: California Department of Boating and Waterways).
Koenigstein S., Ruth M., Gößling-Reisemann S. (2016). Stakeholder-informed ecosystem modeling of ocean warming and acidification impacts in the barents Sea region. Front. Mar. Sci. 3, 93. doi: 10.3389/fmars.2016.00093
Ludka B. C., Gallien T., Crosby S. C., Guza R. T. (2016). Mid-El niño erosion at nourished and unnourished southern California beaches. Geophysical Res. Lett. 43, 4510–4516. doi: 10.1002/2016GL068612
Martinich J., Neumann J., Ludwig L., Jantarasami L. (2013). Risks of sea level rise to disadvantaged communities in the united states. Mitigation Adaptation Strategies Global Change 18, 169–185. doi: 10.1007/s11027-011-9356-0
Martin D. M., Specht J. A., Canick M. R., Leo K. L., Freeman K. (2022). Using decision analysis to integrate habitat and community values for coastal resilience planning. Estuaries Coasts 45, 331–344. doi: 10.1007/s12237-021-00970-x
McGuire C. J. (2021). The human dimensions of coastal adaptation strategies. Sustainability 13, 546. doi: 10.3390/su13020546
Moffatt & Nichol (2018). Venice Sea Level rise vulnerability assessment (Los Angeles, California: City of Los Angeles).
Mostafiz R. B., Friedland C. J., Rahman M. A., Rohli R. V., Tate E., Bushra N., et al. (2021). Comparison of neighborhood-scale, residential property flood-loss assessment methodologies. Front. Environ. Sci. 9. doi: 10.3389/fenvs.2021.734294
Nastev M., Todorov N. (2013). Hazus: A standardized methodology for flood risk assessment in Canada. Can. Water Resour. J. 38, 223–231. doi: 10.1080/07011784.2013.801599
Noble Consultants-G.E.C. Inc (2016). “Seasonal sand berm protection program,” in Los Angeles County Department of Beaches and Harbors (Los Angeles, California: NobleConsultants).
Oh C.-O., Dixon A. W., Mjelde J. W., Draper J. (2008). Valuing visitors’ economic benefits of public beach access points. Ocean Coast. Manage. 51, 847–853. doi: 10.1016/j.ocecoaman.2008.09.003
O’Neill A. C., Erikson L. H., Barnard P. L., Limber P. W., Vitousek S., Warrick J. A., et al. (2018). Projected 21st century coastal flooding in the southern California bight. part 1: development of the third generation CoSMoS model. J. Mar. Sci. Eng. 6, 59. doi: 10.3390/jmse6020059
Palinkas C. M., Orton P., Hummel M. A., Nardin W., Sutton-Grier A. E., Harris L., et al. (2022). Innovations in coastline management with natural and nature-based features (NNBF): Lessons learned from three case studies. Front. Built Environ. 62. doi: 10.3389/fbuil.2022.814180
Powell E. J., Tyrrell M. C., Milliken A., Tirpak J. M., Staudinger M. D. (2019). A review of coastal management approaches to support the integration of ecological and human community planning for climate change. J. Coast. Conserv. 23, 1–18. doi: 10.1007/s11852-018-0632-y
Reguero B. G., Beck M. W., Bresch D. N., Calil J., Meliane I. (2018). Comparing the cost effectiveness of nature-based and coastal adaptation: A case study from the gulf coast of the united states. PLoS One 13, e0192132. doi: 10.1371/journal.pone.0192132
Rolfe M. I., Pit S. W., McKenzie J. W., Longman J., Matthews V., Bailie R., et al. (2020). Social vulnerability in a high-risk flood-affected rural region of NSW, Australia. Natural Hazards 101, 631–650. doi: 10.1007/s11069-020-03887-z
Rosenbloom J. (2018). Fifty shades of gray infrastructure: Land use and the failure to create resilient cities. Washington Law Rev. 93, 317. Available at: https://digitalcommons.law.uw.edu/wlr/vol93/iss1/7
Rufat S., Tate E., Burton C. G., Maroof A. S. (2015). Social vulnerability to floods: Review of case studies and implications for measurement. Int. J. Disaster Risk Reduction 14, 470–486. doi: 10.1016/j.ijdrr.2015.09.013
Schneider P. J., Schauer B. A. (2006). HAZUS: Its development and its future. Natural Hazards Rev. 7, 40–44. doi: 10.1061/(ASCE)1527-6988(2006)7:2(40)
Sovacool B. K. (2011). Hard and soft paths for climate change adaptation. Climate Policy 11, 1177–1183. doi: 10.1080/14693062.2011.579315
Storlazzi C. D., Reguero B. G., Cole A. D., Lowe E., Shope J. B., Gibbs A. E., et al. (2019). Rigorously valuing the role of US coral reefs in coastal hazard risk reduction (Reston, Virginia: US Department of the Interior, US Geological Survey).
Sutton-Grier A. E., Gittman R. K., Arkema K. K., Bennett R. O., Benoit J., Blitch S., et al. (2018). Investing in natural and nature-based infrastructure: Building better along our coasts. Sustainability 10, 523. doi: 10.3390/su10020523
Sutton-Grier A. E., Wowk K., Bamford H. (2015). Future of our coasts: The potential for natural and hybrid infrastructure to enhance the resilience of our coastal communities, economies and ecosystems. Environ. Sci. Policy 51, 137–148. doi: 10.1016/j.envsci.2015.04.006
Sweet W. V., Hamlington B. D., Kopp R. E., Weaver C. P., Barnard P. L., Bekaert D., et al. (2022). Global and regional Sea level rise scenarios for the united states (Silver Spring, Maryland: National Oceanic and Atmospheric Administration).
Tebaldi C., Strauss B. H., Zervas C. E. (2012). Modelling sea level rise impacts on storm surges along US coasts. Environ. Res. Lett. 7, 1–11. doi: 10.1088/1748-9326/7/1/014032
Temmerman S., Kirwan M. L. (2015). Building land with a rising sea. Science 349, 588–589. doi: 10.1126/science.aac8312
USACE (2006). Atlantic Coast of long island, Jones lnlet to East Rockaway inlet. volume 2: Draft technical appendices. New York, New York: U.S. Army Corps of Engineers New York District
Van Slobbe E., de Vriend H. J., Aarninkhof S., Lulofs K., de Vries M., Dircke P. (2013). Building with nature: In search of resilient storm surge protection strategies. Natural Hazards 66, 1461–1480. doi: 10.1007/s11069-013-0612-3
Wang R. Q., Stacey M. T., Herdman L. M. M., Barnard P. L., Erikson L. (2018). The influence of sea level rise on the regional interdependence of coastal infrastructure. Earth’s Future 6, 677–688. doi: 10.1002/2017EF000742
Zahran S., Brody S. D., Peacock W. G., Vedlitz A., Grover H. (2008). Social vulnerability and the natural and built environment: A model of flood casualties in Texas. Disasters 32, 537–560. doi: 10.1111/j.1467-7717.2008.01054.x
Keywords: coastal flooding, sea level rise, adaptation, multi-criteria analysis, hydrodynamic modeling, California
Citation: Schroder K, Hummel MA, Befus KM and Barnard PL (2022) An integrated approach for physical, economic, and demographic evaluation of coastal flood hazard adaptation in Santa Monica Bay, California. Front. Mar. Sci. 9:1052373. doi: 10.3389/fmars.2022.1052373
Received: 23 September 2022; Accepted: 31 October 2022;
Published: 24 November 2022.
Edited by:
Siddharth Narayan, East Carolina University, United StatesReviewed by:
Alec Torres-Freyermuth, Universidad Nacional Autónoma de México, MexicoCopyright © 2022 Schroder, Hummel, Befus and Barnard. This is an open-access article distributed under the terms of the Creative Commons Attribution License (CC BY). The use, distribution or reproduction in other forums is permitted, provided the original author(s) and the copyright owner(s) are credited and that the original publication in this journal is cited, in accordance with accepted academic practice. No use, distribution or reproduction is permitted which does not comply with these terms.
*Correspondence: Michelle A. Hummel, bWljaGVsbGUuaHVtbWVsQHV0YS5lZHU=
Disclaimer: All claims expressed in this article are solely those of the authors and do not necessarily represent those of their affiliated organizations, or those of the publisher, the editors and the reviewers. Any product that may be evaluated in this article or claim that may be made by its manufacturer is not guaranteed or endorsed by the publisher.
Research integrity at Frontiers
Learn more about the work of our research integrity team to safeguard the quality of each article we publish.