- 1Institute of Coastal Environmental Pollution Control, College of Environmental Science and Engineering, Key Laboratory of Marine Environment and Ecology, Ministry of Education, Frontiers Science Center for Deep Ocean Multispheres and Earth System, Ocean University of China, Qingdao, China
- 2Marine Ecology and Environmental Science Laboratory, Pilot National Laboratory for Marine Science and Technology, Qingdao, China
- 3Sanya Oceanographic Institution, Ocean University of China, Sanya, China
- 4Faculty of Agricultural, Life and Environmental Science, University of Alberta, Edmonton, AB, Canada
Introduction: Biobased polymer blends have been recommended as an eco-friendly solution to abate plastic pollution in the environment. However, the formation of microplastics (MPs) by photodegradation of biobased polymer blends in the marine environment is still not well understood. In this study, we investigated the formation of MPs and the changes in the physicochemical properties of three types of biobased polymer blends after photodegradation in seawater.
Methods: The investigated materials included non-biodegradable polyethylene/ thermoplastic starch blends (PE/TPS) and polypropylene/thermoplastic starch blends (PP/TPS), as well as biodegradable polylactic acid/poly (butylene adipate-co-terephthalate)/thermoplastic starch blends (PLA/PBAT/TPS). The control groups were the corresponding neat polymers, including polyethylene (PE), polypropylene (PP), and polylactic acid (PLA).
Results: The size distribution of the pristine and aged MPs indicated that the polymer blends were more likely to produce small-sized particles after photodegradation due to their poorer mechanical properties and lower resistance to UV irradiation than the neat polymers. Noticeable surface morphology alterations, including cracks, holes, and pits, were observed for polymer blends after photodegradation, while neat polymers were relatively resistant. After photodegradation, the attenuated total reflection Fourier transformed infrared spectroscopy (ATR-FTIR) spectrum of the polymer blends showed a significant decrease in the characteristic bands of thermoplastic starch (TPS), indicating depletion of their starch fractions. The C1s spectra of the polymer blends demonstrated that the aged MPs contained fewer -OH groups than the pristine MPs, further confirming the photodegradation of TPS. The molecular weight distribution curve of the polymer blends shifted significantly towards low molecular weight, suggesting the occurrence of chain scission during photodegradation. These results indicate that the polymer blends have a higher degree of photodegradation than neat polymers, and thereby generate more small-sized MPs than neat polymers. Photodegradation caused changes in the contact angle and surface charge of MPs derived from biobased polymer blends, which may affect the vector effects of MPs on any coexisting pollutants.
Discussion: In summary, polymer blends may pose a higher risk to the marine environment than neat polymers, and caution should be taken in promoting biobased polymer blends.
1 Introduction
Although the production and use of plastics have been increasing worldwide because of their versatility (Chen et al., 2021), plastics also pose a great risk to environmental health (Hohn et al., 2020; Ainali et al., 2022). It has been estimated that about 60% of plastics produced globally ended up in landfills or the natural environment due to their excessive use and inadequate disposal (Geyer et al., 2017). Even though countries around the world make tremendous efforts to mitigate plastic pollution, the amount plastic waste emitted into aquatic ecosystems by 2030 is predicted to be 20–53 Mt year-1 (Borrelle et al., 2020). Main concerns of plastic pollutants are microplastics (MPs, ≤ 5 mm) and nanoplastics (NPs), including primary MP/NPs resulting from plastic manufacturing (Hartmann et al., 2019) and secondary MP/NPs resulting from the degradation of plastic wastes via mechanical abrasion (Song et al., 2017), ultraviolet (UV) irradiation (Wang et al., 2020), and biological breakdown (Zhang et al., 2021a). MPs and NPs are prone to accumulating in the marine environment and pose potential risks to organisms ranging from phytoplankton, zooplankton, bivalves, and fish (Carbery et al., 2018; Huang et al., 2021), and even to humans (Elizalde-Velázquez et al., 2021; Nor et al., 2021). Therefore, exploring the environmental degradation of plastics and the formation of MPs from plastic debris in the marine environment is urgently needed.
To reduce the problem of plastic pollution, recycling and the restricted use of fossil-fuel-based plastics such as polyethylene (PE) and polypropylene (PP) have been strongly encouraged in many countries (Chen et al., 2021; Horton, 2022). More importantly, fossil-fuel-based plastics are being replaced by biodegradable plastics such as polylactic acid (PLA), poly (butylene adipate-co-terephthalate) (PBAT), and poly (butylene succinate) (PBS) (European Bioplastics, 2021; Ghosh and Jones, 2021). These neat polymers are biodegradable and can be degraded by microorganisms under specific conditions (e.g., composting) (Haider et al., 2019; Shen et al., 2020). Some biodegradable polymers lack flexibility, strength, and toughness, so they are often mixed with appropriate additives (metal particles, inorganic, and organic nanoparticles) (Sanusi et al., 2021; Tarani et al., 2021). To achieve commercially functional properties, some biodegradable polymers often need to be blended (e.g., polylactic acid/thermoplastic starch blends [PLA/TPS], polylactic acid/poly [butylene adipate-co-terephthalate] blends [PLA/PBAT], and polylactic acid/poly [butylene adipate-co-terephthalate]/thermoplastic starch blends [PLA/PBAT/TPS]) (Yoksan et al., 2021). These biobased polymer blends can biodegrade and be processed through organic recycling under specific conditions (e.g., composting), and TPS derived from PLA/TPS and PLA/PBAT/TPS can be degraded by microorganisms in marine environment (Narancic et al., 2018). A wide range of non-biodegradable biobased polymer blends (e.g., polyethylene/thermoplastic starch blends [PE/TPS], polypropylene/thermoplastic starch blends [PP/TPS], and polylactic acid/polyethylene blends [PLA/PE]) have been manufactured in large quantities due to their low cost. However, the fossil-fuel-based components in these blends are difficult for microorganisms to degrade (Afkhami et al., 2019). Some studies have found that neat biodegradable polymers in the natural environment generated large amounts of MPs via photodegradation, biodegradation, and hydrolysis (Liao and Chen, 2021; Wei et al., 2021a). Similar to neat biodegradable polymers, biobased polymer blends, if inadvertently released into the marine environment, may lead to microplastic (MP) pollution and associated ecological risks. Nevertheless, our knowledge of the degradation of biobased polymer blends in the marine environment is still limited.
Photodegradation is a key process in the degradation of MPs (Cai et al., 2018; Zhou et al., 2021) that strongly affects their fate and potential hazard level (Kim et al., 2022; Luo et al., 2022a). Several studies have found that UV irradiation triggers the photodegradation of MPs derived from fossil-fuel-based plastics, leading to surface oxidation (Lin et al., 2020; Cao et al., 2021), structural disintegration (Wu et al., 2021), size reduction, and the generation of NPs (Wang et al., 2020). In recent years, the photodegradation of biodegradable MPs and their ecological effects have received increasing attention (Fan et al., 2021; Ainali et al., 2022). For example, Zhang et al. (2021b) found that photodegradation reduced the size of PLA MPs, creating NPs and elevating their toxicity to developing zebrafish by triggering mitochondrial dysfunction and apoptosis. Nevertheless, to the best of our knowledge, little information is available regarding the photodegradation of biobased polymer blends in seawater, hampering the risk assessment of polymer blends in marine environments.
The photodegradation of neat polymers is influenced by intrinsic factors such as chemical structure, molecular weight, shape, and size (Halle et al., 2016). For instance, biodegradable PLA was less resistant to UV irradiation due to the presence of ester groups, resulting in a higher degree of chemical weathering than fossil-fuel-based polyvinyl chloride (PVC) (Fan et al., 2021). Previous research on biobased polymer blends has found that both PLA/PE and PLA/PBAT do not easily photodegrade into small fragments or MPs compared to their respective neat biodegradable polymers, probably due to their enhanced mechanical properties after blending (Liao and Chen, 2021). However, polymer blends containing TPS (e.g., PLA/TPS, PE/TPS, and PP/TPS) generally exhibited poorer mechanical properties than the neat polymers, because the starch granules in the blends have poor miscibility with the surrounding polymer matrix (Narancic et al., 2018). The starch granules preserved inside the polymer matrix can be easily released by weathering; thus, large amounts of MPs can potentially be released due to the fragmentation of the polymer matrix (Wei et al., 2021b). Therefore, under the same weathering conditions, polymer blends containing TPS may be more likely to generate MPs than neat polymers. Accordingly, it is reasonable to hypothesize that under the same conditions, the biobased polymer blends containing TPS would have a higher degree of photodegradation and generate more small-sized MPs compared to the neat biodegradable or fossil-fuel-based polymers.
To test this hypothesis, three commercially available polymer blends (i.e., PE/TPS, PP/TPS and PLA/PBAT/TPS) were selected and corresponding neat polymers (i.e., PE, PP and PLA) were included as control groups. To simulate the fragmentation of discarded plastics caused by physical weathering (e.g., wave action and sand abrasion), the pristine MPs were prepared by mechanical grinding. Then photodegradation of pristine MPs under UV irradiation in natural seawater was investigated. The specific objectives of this study were to 1) analyze the changes in the size distribution, surface morphology, surface functional groups, molecular weight, contact angle, and surface charge of MPs derived from different biobased polymer blends after photodegradation, as compared to the neat polymers; 2) explore the possible photodegradation mechanisms of MPs in the seawater; 3) evaluate the potential environmental risks of photodegraded MPs in the context of reducing marine MP pollution, and make recommendations on the use and management of biobased polymer blends. These findings could provide new insights into the source and potential environmental risks of MPs derived from the biobased polymer blends in the marine environment.
2 Materials and methods
2.1 Preparation of MPs
We selected three commercially available polymer blends (i.e., PE/TPS, PP/TPS, and PLA/PBAT/TPS) for study and their corresponding neat polymers (i.e., PE, PP, and PLA) as control groups (Figure S1). PE/TPS, PP/TPS, and PLA/PBAT/TPS were chosen because they have gained a considerable share in the bioplastics market due to their versatility and low cost (European Bioplastics, 2021). PE/TPS and PP/TPS are non-biodegradable and PLA/PBAT/TPS is biodegradable. Basic information on the six plastics is listed in Table S1.
To simulate the fragmentation of discarded plastics caused by physical weathering (e.g., wave action and sand abrasion), the pristine MPs were prepared by mechanical grinding (Wang et al., 2020). The plastics were cut into square fragments (5 mm × 5 mm) using a clean stainless-steel scissor. To avoid external contamination of the material surface, the square fragments were rinsed five times with ultrapure water (Milli-Q, resistivity > 18.2 MΩ), and then left to dry at room temperature. Next, to obtain small-sized fragments, the square fragments were frozen with liquid nitrogen for ten minutes to make them brittle before being transferred to a ball grinder machine (JXFSTPRP-II, Jingxin, China) for grinding (1 min, 50 Hz). The small-sized fragments were then frozen for five minutes and ground for one minute. These steps were repeated 11 times to collect adequate MPs. Subsequently, the ground fragments were collected in clean glass Petri dishes and placed in an oven to dry at 40°C for 24 h. The dried fragments were then ground in a ceramic mortar to avoid adhesion and sieved with a 100 µm stainless steel mesh to obtain the pristine MPs smaller than 100 µm. The pristine MPs were stored in a dryer at room temperature and kept away from light until further experiments.
2.2 UV irradiation experiment
To simulate the photodegradation of MPs in the seawater, 100 mg of the dried pristine MPs were transferred into a 150-mL quartz tube that was previously filled with 100 mL of filtered natural seawater (1.2 µm GF/C filters, pH 8.1, and salinity 31‰). Next, 170 µL of HgCl2 stock solution (10 g L-1) was added to each quartz tube to avoid biodegradation (Paluselli et al., 2019). Triplicates were set up for each treatment group. The quartz tubes were exposed to continuous UV irradiation in a cylindrical quartz-wall photo-reactor (GHX-V, Bilon, China) for 2 months. The central part of the photo-reactor was a 1000 W mercury lamp screened with a UV cutoff filter (λ > 400 nm), so that the wavelength range covered was below 400 nm. The irradiation intensity of light was 68.6 ± 22.0 W m-2. A cooling water circulation system was used to maintain the temperature at 25°C to prevent elevated sample temperatures due to prolonged operation of the mercury lamp. During the irradiation, the quartz tubes were gently shaken twice a day to thoroughly mix the MPs and seawater. To prevent dramatic salinity fluctuations caused by the evaporation of seawater, the water volume in the quartz tube was checked, and Milli-Q water was added when needed.
After 2 months of UV irradiation, the MP suspension in the quartz tube was filtered through a 0.22-µm polytetrafluoroethylene (PTFE) membrane using a glass vacuum filter (JOANLAB, Jinteng, China) to collect the aged MPs. The aged MPs were retained on the filter membrane, placed in a cryopreservation tube, and then dried with a freeze dryer (FD5, SIM, USA).
2.3 Characterization of the pristine and aged MPs
2.3.1 Size distribution
The size distribution of the pristine and aged MPs was examined using confocal laser scanning microscopy (CLSM, A1, Nikon, Japan). Two milligrams of the dried MPs were transferred into a 2-mL centrifuge tube that was previously filled with 1 mL of ethanol solution (50%). Then MPs were ultrasonically dispersed for 15 min using an ultrasonic cleaner (KQ-3200B, Kunshan Shumei, China). Samples of the highly dispersed ethanol suspension (10 μL) of MPs were dropped onto a clean glass microscope slide and air-dried for subsequent observation by the CLSM. To obtain the size distribution, all particles in the CLSM images were measured using the Image J software (http://imagej.net/) (Liu et al., 2018). Three replicates were set up for each MP.
2.3.2 Surface morphology
For scanning electron microscopy (SEM, S-3400N, Hitachi, Japan) observation, MP samples were adhered onto a silver conductive tape sputter-coated with a layer of gold and then observed at different magnifications. Considering that the surface morphologies of MPs were heterogeneous, 10 surface sites from each sample were randomly selected for SEM imaging to ensure data reliability.
2.3.3 Surface functional groups and element composition
The surface functional groups of the pristine and aged MPs were analyzed by an attenuated total reflection Fourier transformed infrared spectroscopy (ATR-FTIR, UATR Two, PerkinElmer, USA). The spectral range was set from 4000 to 400 cm-1, and the instrument resolution was 4 cm-1, with 16 scans per spectrum. To guarantee data reproducibility, at least three samples for each data point were measured via ATR-FTIR. Surface elemental compositions and carbon (C) functional groups of the MPs were analyzed by X-ray photoelectron spectroscopy (XPS, ESCALAB250Xi, Thermo Scientific, USA) with a monochromatic Al Kα X-ray source (1486.6 eV). The peak deconvolution for C1s was analyzed using XPS peak 41 software. The surface functional groups for each type of MP were quantified and compared. Each type of MP was measured in triplicate.
2.3.4 Molecular weight distribution
The molecular weight of MPs was determined by gel permeation chromatography (GPC). GPC was carried out on an Agilent PL-GPC220 equipped with Agilent PLgel Olexis 300 × 7.5 mm columns (for PE, PP, PE/TPS, and PP/TPS) and a Waters1525 equipped with Agilent PLgel 5 um MIXED-C columns (for PLA and PLA/PBAT/TPS). The molecular weight of PE, PP, PE/TPS, and PP/TPS were analyzed at 150°C using 1, 2, 4-trichlorobenzene as the eluent and solvent with a flow rate of 1 mL min-1 (Gao and Sun, 2021). PLA was performed at 35°C using trichloromethane as the eluent and solvent with a flow rate of 1 mL·min-1. For the PLA/PBAT/TPS, tetrahydrofuran was used as the eluent and solvent with a flow rate of 1 mL min-1 at 35°C (Wei et al., 2021a). A sample concentration of 2 mg mL-1 was used for the pristine and aged MPs. The sample solutions were filtered through a 0.45-µm PTFE membrane before analysis. The Agilent PL-GPC220 and Waters1525 systems were pre-calibrated using polystyrene standards of known molecular mass.
2.3.5 Contact angle
The wettability of the MPs was characterized by contact angle (CA) measurements (DSA20, KRUSS, Germany) using the sessile drop method. A drop (3 μL) of Milli-Q water was deposited on the solid surface, and then CA was measured immediately at 22°C for three specimens of each MP (Isimjan et al., 2012).
2.3.6 Zeta potential
The zeta potentials were determined using a Zetasizer (Nano-ZS90, Malvern Instruments, UK). The MPs were diluted to 4 mg mL-1 in natural seawater (0.22 µm GF/C filters, pH 8.1, and salinity 31‰), and then sonicated (25°C, 40 Hz) for 20 min to disperse evenly before analysis. Each MP was measured three times.
2.4 Statistical analysis
Data are presented as mean ± standard deviation (SD). One-way analysis of variance (ANOVA) with S-N-K's multiple-comparison test (P < 0.05) was used for the significance test of different MPs using Statistical Product and Service Solutions Software (SPSS 16.0). The independent sample t test (P < 0.05) was used to analyze the significant difference between the pristine and aged MPs using SPSS 16.0.
3 Results
3.1 Changes in the size distribution of MPs
With respect to the size distribution of the pristine MPs, a considerable portion of polymer blend particles (PE/TPS, PP/TPS, and PLA/PBAT/TPS) were less than 10 μm (22.8%, 31.7%, and 25.2%, respectively) (Figure 1 and Table S2).The proportion of particle sizes smaller than 10 μm in PE, PP, and PLA were only 9.30%, 8.18%, and 6.32%, respectively. The average particle size of PE/TPS (38.3 ± 25.5 μm) was significantly smaller than that of PE (51.6 ± 25.7 μm). The average particle size of PP/TPS (29.8 ± 25.8 μm) was significantly smaller than that of PP (41.1 ± 22.2 μm). There is no significant difference between the average particle size of PLA (35.9 ± 22.6 μm) and PLA/PBAT/TPS (30.6 ± 19.6 μm). Compared to the neat polymers, the polymer blends such as PE/TPS, PP/TPS, and PLA/PBAT/TPS were mechanically ground to a higher degree of fragmentation, resulting in relatively smaller particle sizes.
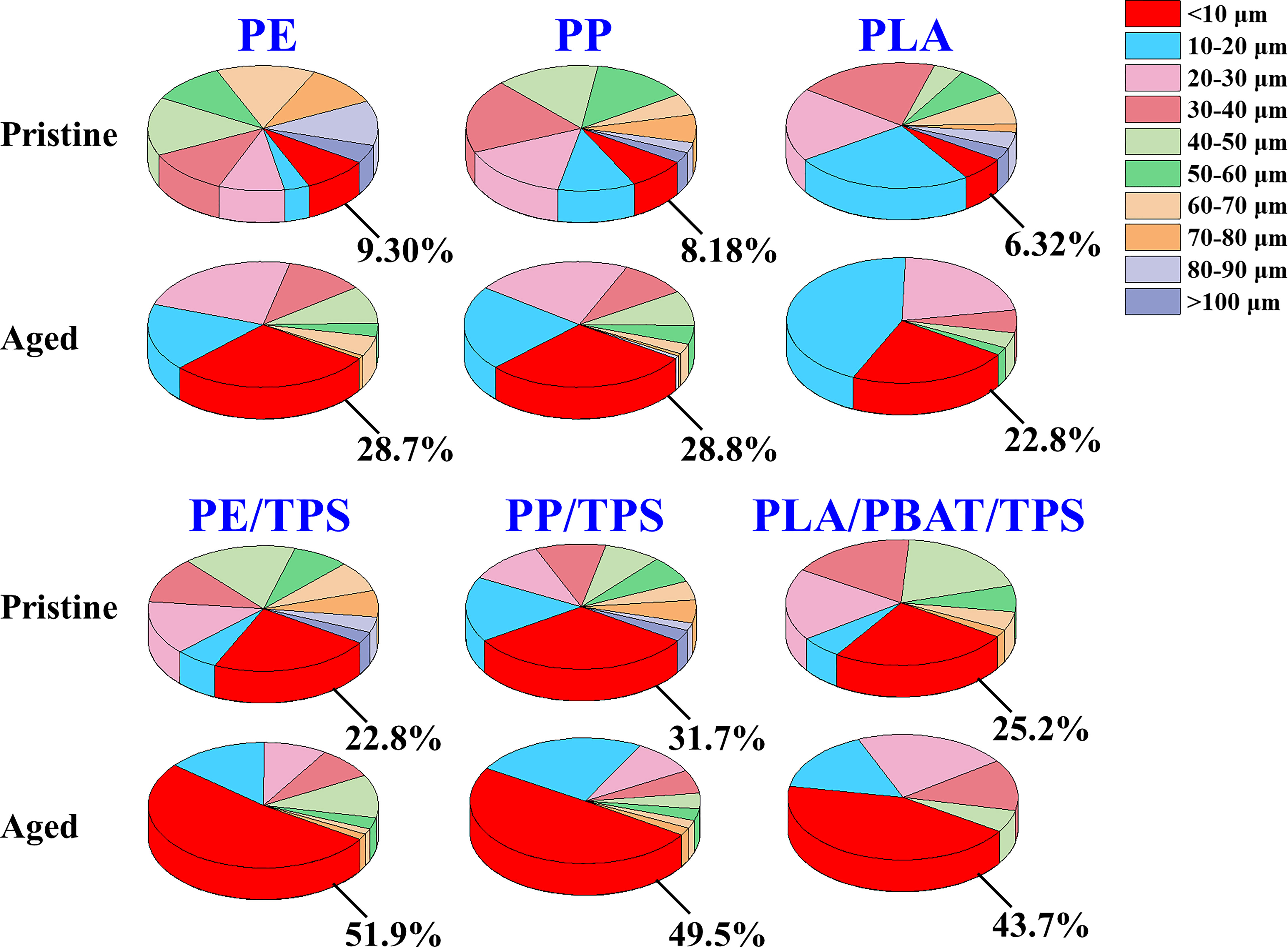
Figure 1 Size distribution of the pristine and aged MPs treated by 2 months of UV irradiation in natural seawater. To obtain the size distribution, all particles (n > 100) in the CLSM images were measured using Image J software (http://imagej.net/) and three replicates were used for each type of MP. PE, polyethylene; PP, polypropylene; PLA, polylactic acid; PE/TPS, polyethylene/thermoplastic starch blends; PP/TPS, polypropylene/thermoplastic starch blends; and PLA/PBAT/TPS, polylactic acid/poly (butylene adipate-co-terephthalate)/thermoplastic starch blends.
After 2 months of photodegradation, the proportion of particles smaller than 10 μm increased for all the aged MPs. The average particle size of the aged MPs was smaller than that of the pristine MPs (Figure 1 and Table S2). These results demonstrated that photodegradation exacerbated the breakdown of the pristine MPs particles, resulting in the formation of smaller particles. The proportion of particles less than 10 μm in the aged PE/TPS, PP/TPS and PLA/PBAT/TPS were 51.9%, 49.5%, and 43.7%, respectively, while only 28.7%, 28.8%, and 22.8% particles less than 10 μm were observed in the aged PE, PP, and PLA (Table S2). Additionally, the average particle size of the aged PLA (18.1 ± 10.6 μm), PE/TPS (18.9 ± 17.6 μm), PP/TPS (17.3 ± 16.3 μm), and PLA/PBAT/TPS (17.1 ± 12.6 μm) was smaller than for aged PE (24.5 ± 17.8 μm) and PP (23.8 ± 16.7 μm). These results suggested that polymer blends are more likely to generate small-sized particles after photodegradation than neat polymers.
3.2 Changes in the surface morphology of MPs
The surface morphological characteristics of the MPs were observed using SEM. For PE, PP, and PLA, the surface of the pristine MPs was relatively smooth (Figures 2A–C), whereas roughening of surfaces and formation of fragments (indicated by red arrows) were observed on the pristine PE/TPS, PP/TPS, and PLA/PBAT/TPS (Figures 2D–F). Spherical or ellipsoidal particles (indicated by green arrows) were observed on the surface of the pristine PE/TPS, PP/TPS, and PLA/PBAT/TPS, particularly on the pristine PP/TPS. A larger ellipsoidal particle (indicated by a white arrow) was observed (Figure 2E). The polymer blends used in this study had an island-sea structure (Wei et al., 2021b), in which starch granules are dispersed in the polymer matrix (Figures S2D–F). Moreover, the starch granules inside the polymer matrix released as a result of mechanical abrasion, fragmenting the polymer matrix and releasing a large number of MPs. Therefore, under the same mechanical stresses, the polymer blends tend to generate smaller-sized particles than the neat polymers.
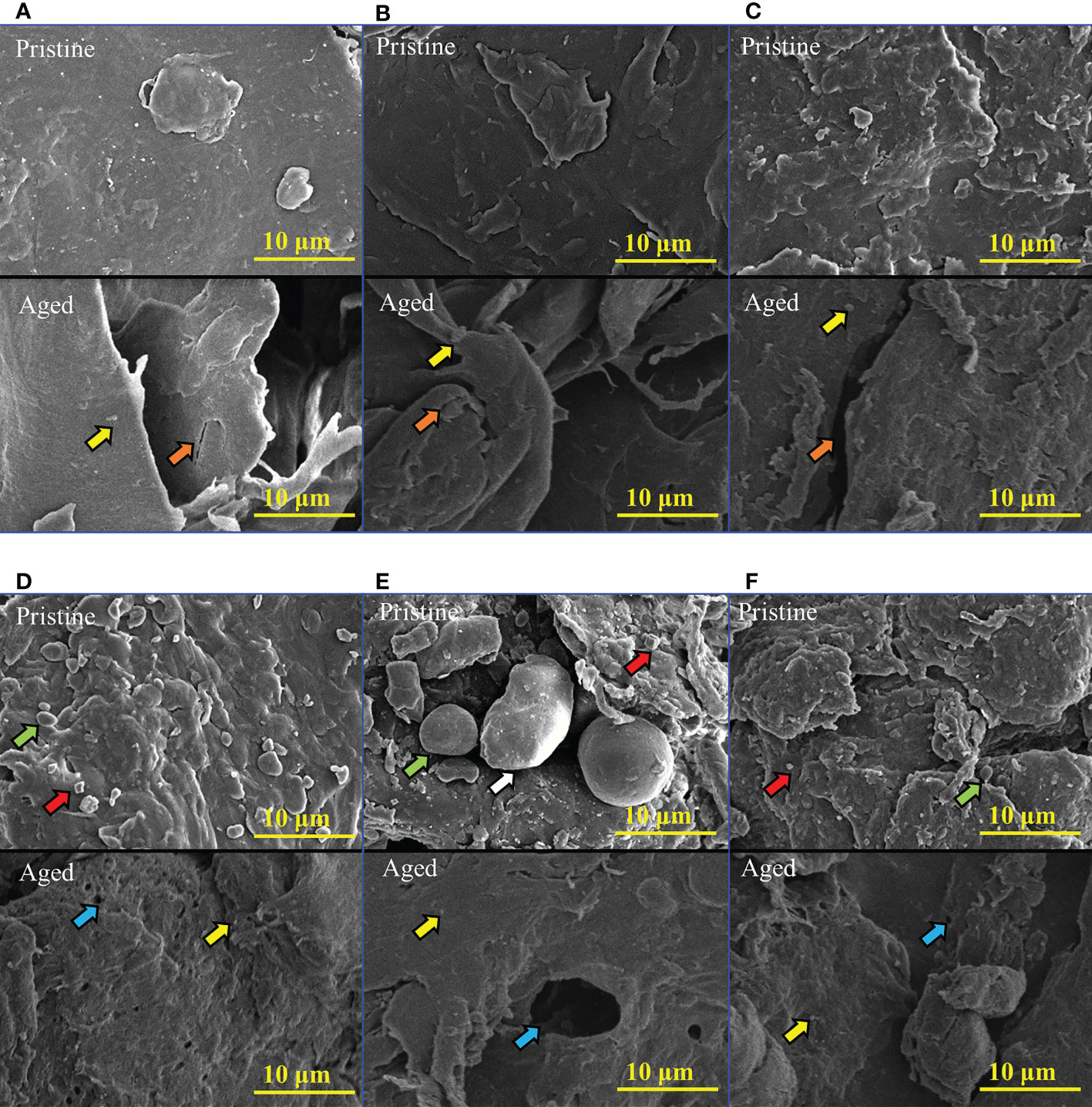
Figure 2 SEM images of the pristine and aged MPs treated by 2 months of UV irradiation in natural seawater. (A) PE, (B) PP, (C) PLA, (D) PE/TPS, (E) PP/TPS, and (F) PLA/PBAT/TPS. PE, polyethylene; PP, polypropylene; PLA, polylactic acid; PE/TPS, polyethylene/thermoplastic starch blends; PP/TPS, polypropylene/thermoplastic starch blends; and PLA/PBAT/TPS, polylactic acid/poly (butylene adipate-co-terephthalate)/thermoplastic starch blends. The red arrows indicate the formation of MP fragments on the surface of the pristine MPs. The green arrows indicate the spherical or ellipsoidal particles on the surface of the pristine MPs due to the release of starch granules. The white arrow indicates the larger starch granule. The yellow arrows indicate the smaller fragments on the surface of the aged MPs. The orange arrows indicate the formation of cracks. The blue arrows indicated the holes and pits that appeared on the surface of the aged MPs due to the migration and degradation of the starch granules.
After 2 months of photodegradation, smaller fragments (indicated by yellow arrows) were observed on the surface of all the aged MPs compared to the pristine MPs (Figures 2A–F). In addition, the PE, PP, and PLA MPs became rough and generated cracks (indicated by orange arrows) after photodegradation. The width and depth of the cracks on the surface of the aged PLA were wider and deeper than on aged PE and PP MPs (Figures 2A–C). The polymer blends also developed holes and pits (indicated by blue arrows) (Figures 2D–F), suggesting obvious degradation. These findings indicated that the polymer blends and neat polymers had different degrees of resistance to UV irradiation.
3.3 Changes in the surface properties of MPs
According to the spectra obtained with ATR-FTIR, there were no significant changes in the spectra of PE and PLA after 2 months of UV irradiation (Figures 3A, C). A carbonyl peak at 1715 cm-1 appeared in the spectrum of PP after photodegradation (Figure 3B). The ATR-FTIR spectra of PE/TPS, PP/TPS, and PLA/PBAT/TPS (Figures 3D–F) showed broad bands between 3000 and 3600 cm-1 associated with the TPS structure. These broad bands are due to the hydroxyl groups linked by hydrogen bonds (Raee et al., 2020). The adsorption bands of PE/TPS at 1078 and 1152 cm-1 are attributed to the -C-O bond stretching of -C-O-H in the TPS. For the PP/TPS, peaks at 1053 and 1151 cm-1 are both assigned to the -C-O bond stretching of -C-O-H in the TPS. We observed that the intensity of the TPS-related absorption peaks decreased significantly after UV irradiation. Specifically, the polymer blends showed spectrum variations after photodegradation, followed by a decrease in the characteristic bands of TPS, indicating the depletion of the starch fraction. With respect to PLA/PBAT/TPS, the spectrum of the aged MPs showed a broader carbonyl peak at 1712 cm-1 with an extra shoulder peak at 1500–1600 cm-1 as compared to the pristine MPs (Figure 3F). These peaks were formed by photodegradation of the PBAT in the blend.
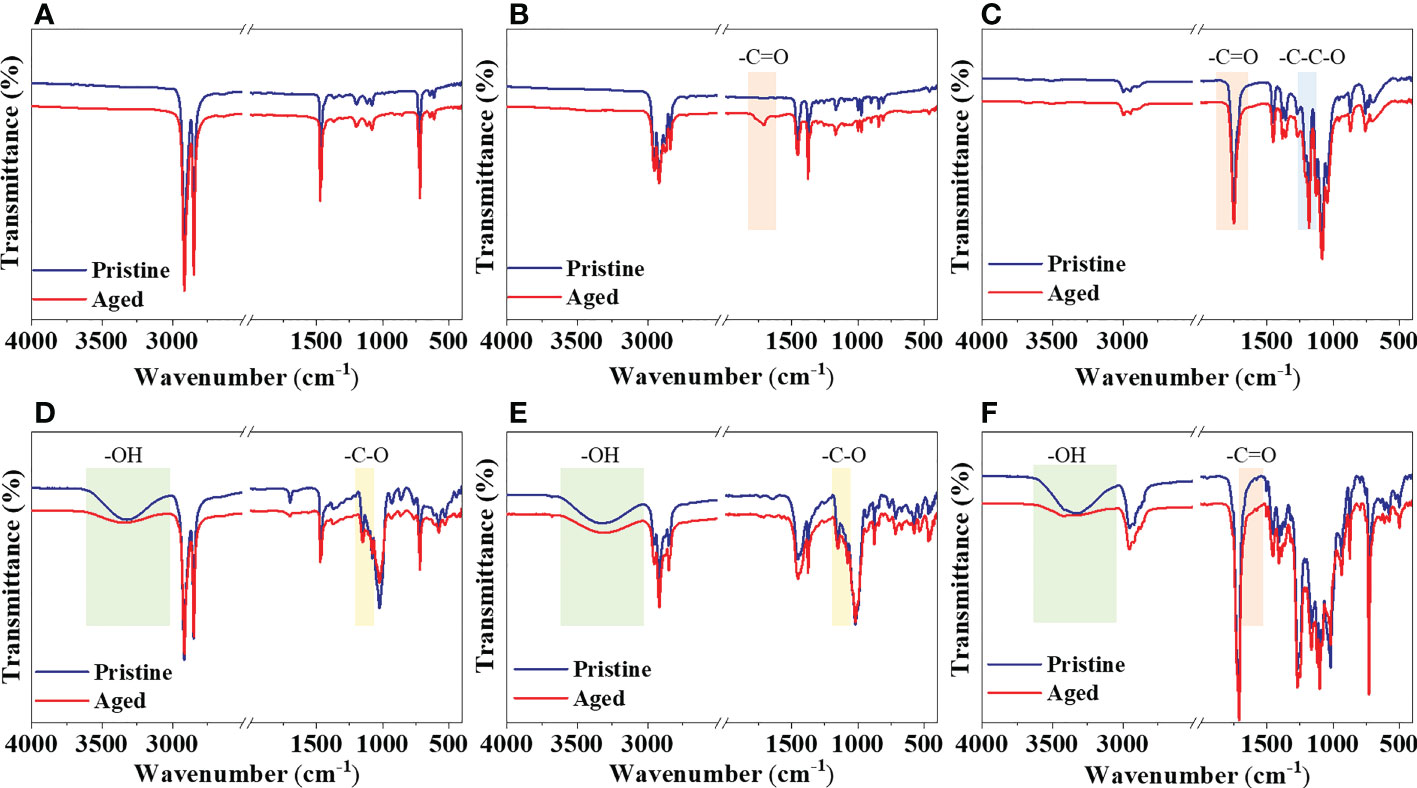
Figure 3 ATR-FTIR spectra of the pristine and aged MPs treated by 2 months of UV irradiation in natural seawater. (A) PE, (B) PP, (C) PLA, (D) PE/TPS, (E) PP/TPS, and (F) PLA/PBAT/TPS. PE, polyethylene; PP, polypropylene; PLA, polylactic acid; PE/TPS, polyethylene/thermoplastic starch blends; PP/TPS, polypropylene/thermoplastic starch blends; and PLA/PBAT/TPS, polylactic acid/poly (butylene adipate-co-terephthalate)/thermoplastic starch blends.
To further evaluate the changes in the surface functional groups of MPs, their surface was analyzed using XPS. There were no significant changes in the C1s spectra of PE after 2 months of UV irradiation (Figure 4A). As shown in Figure 4B, the surface O-containing functional group (C=O, 2.67 ± 0.35%) was formed on the surface of aged PP after UV irradiation (Table S3). Unlike PP, the aged PE/TPS and PP/TPS contained fewer -OH groups than the pristine MPs (Figures 4D, E), consistent with the results of ATR-FTIR spectral analysis, where the intensity of TPS-related absorption peaks decreased significantly after photodegradation (Figures 3D, E). Although there was no significant alteration in the ATR-FTIR spectrum obtained for PLA after photodegradation (Figure 3C), the C1s spectra demonstrated a reduction in C-O bonds (Figure 4C), which may be due to the cleavage of ester bonds during UV exposure (Zaaba and Jaafar, 2020). According to previous research, bands at 1750 cm-1 (vibration of the -C=O group) and 1180 cm-1 (stretching vibration of -C-C-O) often reflect the degradation of PLA (Oliveira et al., 2016; Nazareth et al., 2019). Yet both of these peaks were typical absorption peaks detected in the pristine PLA. Changes in the peak of the C-O bond, which is likely related to the photodegradation of TPS and cleavage of ester bonds during UV exposure, were found in PLA as well as PLA/PBAT/TPS (Figures 4C, F). The aged PLA/PBAT/TPS contained more O-C=O (12.1 ± 0.61%) than the pristine PLA/PBAT/TPS (10.5 ± 0.05%) (Table S3), which is consistent with the ATR-FTIR spectrum results (Figure 3F).
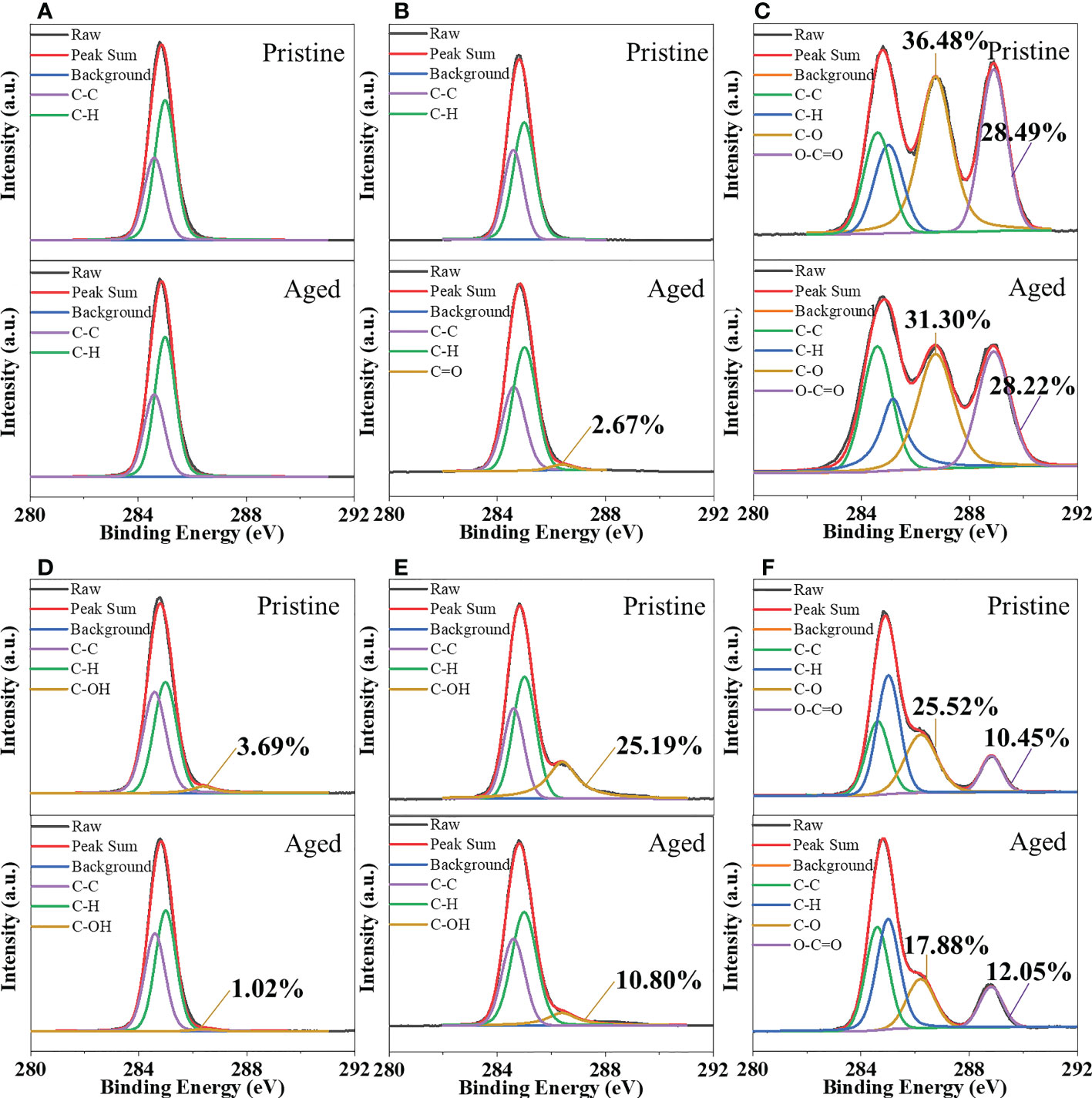
Figure 4 C1s XPS spectra of the pristine and aged MPs treated by 2 months of UV irradiation in natural seawater. (A) PE, (B) PP, (C) PLA, (D) PE/TPS, (E) PP/TPS, and (F) PLA/PBAT/TPS. PE, polyethylene; PP, polypropylene; PLA, polylactic acid; PE/TPS, polyethylene/thermoplastic starch blends; PP/TPS, polypropylene/thermoplastic starch blends; and PLA/PBAT/TPS, polylactic acid/poly (butylene adipate-co-terephthalate)/thermoplastic starch blends.
After 2 months of photodegradation, the contact angles of the PE and PP reduced significantly from 95.5 and 107.7° to 87.1 and 84.9°, respectively (Figure 5A). Similarly, the contact angle of the PE/TPS decreased significantly from 92.9° to 86.1° after photodegradation, indicating a decrease in the hydrophobicity of MPs. In contrast, the contact angle of the PP/TPS increased significantly from 84.8° to 94.1°, indicating a decrease in the hydrophilicity of MPs. The difference in the change of contact angle between PE/TPS and PP/TPS may be due to the different starch content of these two polymer blends. With respect to PLA and PLA/PBAT/TPS, there was no significant alteration in the contact angle after photodegradation, and the hydrophilicity was maintained due to the hydroxyl group content. The surface zeta potential of the pristine and aged MPs in natural seawater is shown in Figure 5B. We observed that the surface zeta potentials of all types of MPs were negatively charged. Except for PE, the surface zeta potential values for aged MPs exhibited a significant decrease after 2 months of photodegradation. These results indicate that photodegradation increases the negative surface charge of pristine MPs.
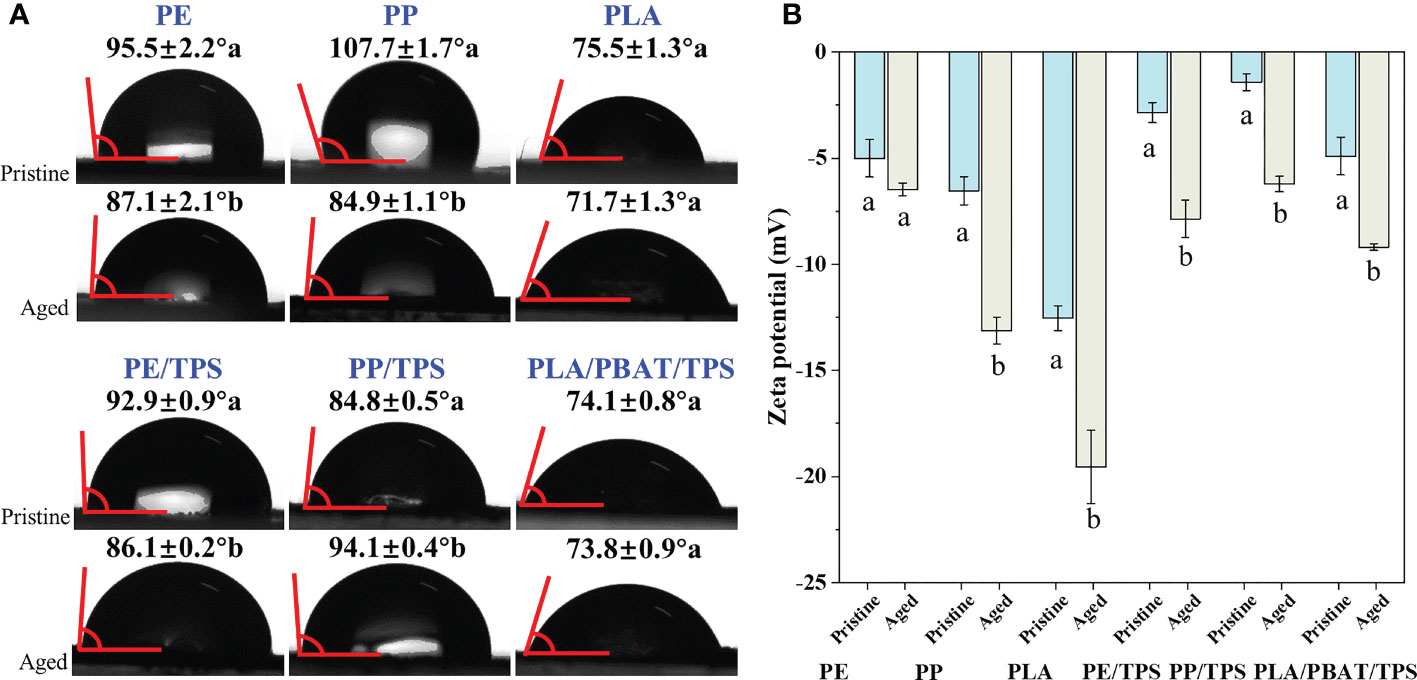
Figure 5 Contact angles (A) and zeta potentials (B) of the pristine and aged MPs treated by 2 months of UV irradiation in natural seawater. PE, polyethylene; PP, polypropylene; PLA, polylactic acid; PE/TPS, polyethylene/thermoplastic starch blends; PP/TPS, polypropylene/thermoplastic starch blends; and PLA/PBAT/TPS, polylactic acid/poly (butylene adipate-co-terephthalate)/thermoplastic starch blends. Different letters following the data or under each bar indicate significant differences between the pristine and aged MPs (Independent Samples t test; n = 3, P < 0.05). Error bars represent the standard deviation. Zeta potentials were measured at 4 mg mL-1 MPs in natural seawater (pH 8.1, salinity 31‰).
3.4 Changes in the molecular weight distribution of MPs
After being exposed to UV irradiation for 2 months, little change in the molecular weight distribution (MWD) curve of the aged MPs compared with the pristine PE, PP, and PLA was observed (Figures 6A–C). Whereas the MWD curves of PE/TPS, PP/TPS, and PLA/PBAT/TPS shifted towards low molecular weights with a visibly increased width after photodegradation (Figures 6D–F). This data suggested the occurrence of chain scission of the polymer blends. Compared with the neat polymers, the molecular weight of the polymer blends decreased considerably after UV irradiation, demonstrating that more severe photodegradation had occurred.
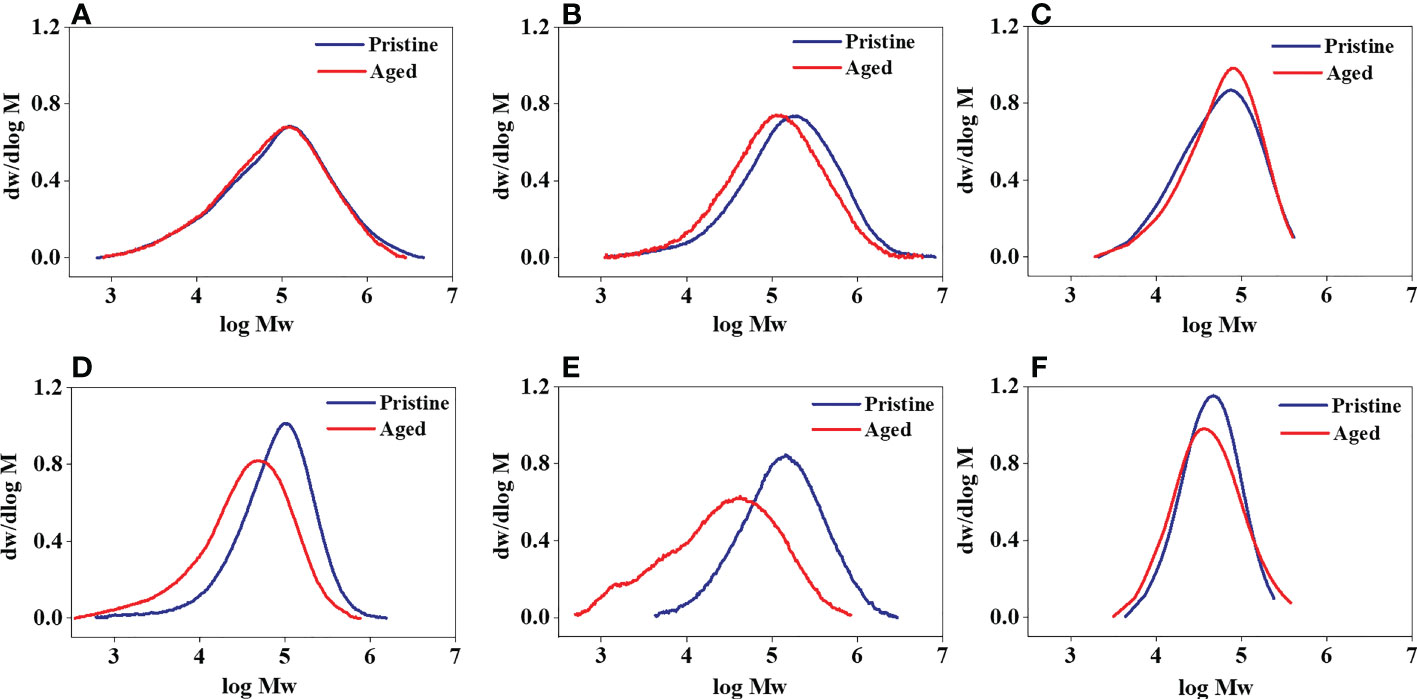
Figure 6 Molecular weight distribution curves of the pristine and aged MPs treated by 2 months of UV irradiation in natural seawater. (A) PE, (B) PP, (C) PLA, (D) PE/TPS, (E) PP/TPS, and (F) PLA/PBAT/TPS. PE, polyethylene; PP, polypropylene; PLA, polylactic acid; PE/TPS, polyethylene/thermoplastic starch blends; PP/TPS, polypropylene/thermoplastic starch blends; and PLA/PBAT/TPS, polylactic acid/poly (butylene adipate-co-terephthalate)/thermoplastic starch blends. The MWD curves shifted towards the low molecular weight side after photodegradation, indicating degradation at high molecular weight; the MWD curves shifted towards the high molecular weight side, indicating degradation at low molecular weight.
4 Discussion
4.1 Fragmentation of MPs derived from biobased polymer blends by photodegradation
MPs can be produced by small and large plastic litter after they have been weathered by wind, waves, and sand. The degree of fragmentation is largely affected by the mechanical properties of the polymers (Song et al., 2017). Our results proved that after mechanical grinding, the polymer blends experienced a higher degree of fragmentation than the neat polymers, generating more small-sized particles (Figure 1). This can be explained by the inferior mechanical properties of polymer blends. TPS had poor miscibility with the other polymers (i.e., PE, PP, PLA, and PBAT), which was confirmed by the island-sea structure of PE/TPS, PP/TPS, and PLA/PBAT/TPS (Figures S2D–F). Thus, polymer blends exhibited poorer mechanical properties than neat polymers. In addition, the mechanical properties of the polymer blends largely depended on the ratio of the polymer components (Ajitha and Thomas, 2020). However, the exact ratios of the polymer blends used in this study were not specified by the manufacturers. Therefore, there is a need for adequate monitoring and regulation of polymer blends markets, where information on the polymer composition is unclear. This information is essential to understanding the fragmentation of polymer blends when they are released into the ocean.
MPs composed of different polymers have different degrees of resistance to UV irradiation (Fan et al., 2021; Liao and Chen, 2021). Our results found that polymer blends were less resistant to UV irradiation than neat polymers in that they showed a higher degree of photodegradation. Furthermore, the island-sea structure of the polymer blends may influence the process of both photooxidation and subsequent fragmentation. We found that mechanical grinding damaged the island-sea structure of the polymer blends, assisting photooxidation by facilitating the depth of UV penetration and diffusion of radicals while increasing the availability of oxygen (Pilař et al., 2015). Due to poor adhesion between TPS and the other polymers (i.e., PE, PP, PLA, and PBAT), the starch granules migrated out of the polymer matrix during UV exposure. Apart from this, photodegradation of starch granules embedded in the surface of the polymer matrix also occurred, which can lead to the detachment of starch particles and plastic debris (Quispe et al., 2019). Consequently, the migration and degradation of the starch granules resulted in a porous structure of the polymer matrix, favoring further photooxidation and fragmentation (Wei et al., 2021b). As was shown by SEM, cracks, as well as many holes and pits, were observed on the surface of the aged polymer blends (Figure 2D-F). Photodegradation makes polymer blends less durable, thereby accelerating the release of small-sized MPs in their matrix. Therefore, the size of particle fragments varies under UV irradiation, with polymer blends exhibiting a higher degree of fragmentation than neat polymers.
As an inexpensive biodegradable polymer, TPS can be used as an alternative to PLA (Yoksan et al., 2021). Polymer blends containing TPS, such as PE/TPS and PLA/PBAT/TPS, have a higher commercial value than PLA/PE and PLA/PBAT. Hence polymer blends containing TPS have been predominately used in disposable tableware or plastic bags and are gaining a significant share in the bioplastics market (European Bioplastics, 2021). These disposable items have been unintentionally manufactured in large quantities due to the difficulty of classifying various types of polymer blends. However, polymer blends containing TPS that are extensively used and not properly disposed of end up in the ocean, thereby generating more and smaller MPs under the wave action, sand abrasion and UV irradiation than neat polymers. This poses a higher risk to the marine environment.
4.2 Potential photodegradation mechanism of MPs in the marine environment
UV irradiation can produce free radicals as primary photochemical products, leading to the dissociation of C-C bonds and C-H bonds from the polymer backbone, inducing chain scission and cross-linking reactions (Gewert et al., 2015). The photodegradation mechanism for the fossil-fuel-based MPs in our study is photooxidative degradation caused by free radical chain reactions (Figure 7A). For example, the carbonyl groups of aged PP (Figures 3B, 4B) can be attributed to the reaction of MPs with oxygen in seawater during UV irradiation (Wu et al., 2021). Unlike PP MPs, the photodegradation of PLA can be described by the Norrish type I reaction or Norrish type II reaction and the radical oxidation reaction (Figure 7B). The photodegradation of PLA occurs mainly through the cleavage of ester bonds. The C-O bond can be reduced following the cleavage of the ester bonds and consecutive reactions driven by free radicals during UV irradiation (Tsuji et al., 2006; Bocchini et al., 2010). Therefore, the difference in photodegradation mechanism between PLA and fossil-fuel-based MPs (i.e., PE and PP) mainly depends on the chemical structure of the polymers.
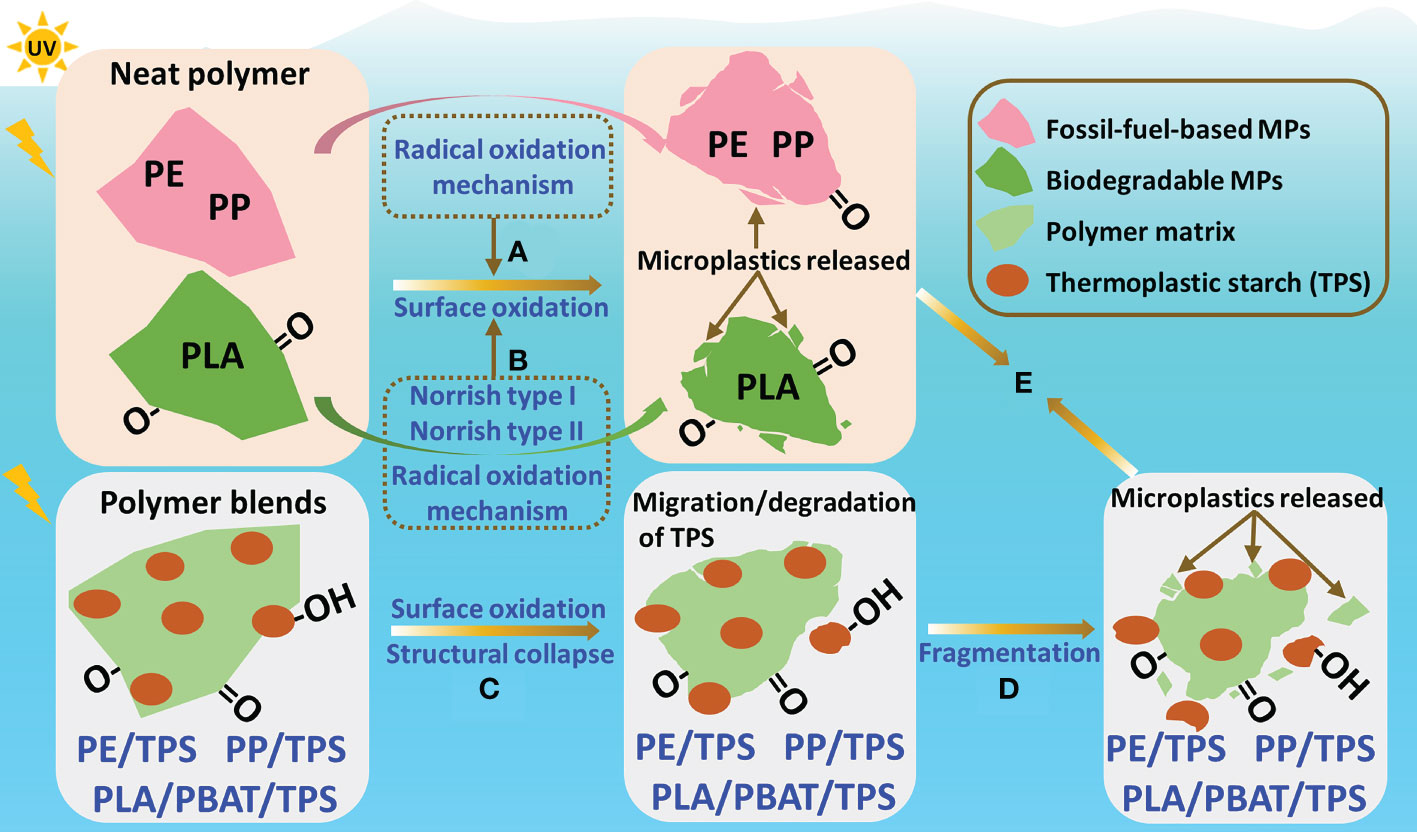
Figure 7 Schematic illustration of the possible photodegradation mechanisms of MPs. (A) Surface oxidation of PE and PP caused by free radical chain reactions; (B) surface oxidation of PLA caused by Norrish type I reaction, Norrish type II reaction, and radical oxidation reaction; (C) the island-sea structure of polymer blends collapses following surface oxidation; (D) fragmentation of polymer matrix; and (E) release of microplastics. Neat polymers: PE, PP, and PLA; polymer blends: PE/TPS, PP/TPS, and PLA/PBAT/TPS. PE, polyethylene; PP, polypropylene; PLA, polylactic acid; PE/TPS, polyethylene/thermoplastic starch blends; PP/TPS, polypropylene/thermoplastic starch blends; and PLA/PBAT/TPS, polylactic acid/poly (butylene adipate-co-terephthalate)/thermoplastic starch blends.
For polymer blends (i.e., PE/TPS, PP/TPS, and PLA/PBAT/TPS) containing starch and plasticizers, the addition of plasticizers can enhance adhesion between the starch and polymers, thereby improving miscibility (Raee et al., 2020). However, plasticizers have relatively weak interactions with the starch chain, so the island-sea structure of the polymer blends collapses following surface oxidation, leading to the migration and degradation of TPS (Figure 7C). As was shown by ATR-FTIR (Figures 3D–F), the characteristic bands of TPS decreased significantly after photodegradation. In addition, the migration and degradation of TPS made the polymer matrix less durable than neat polymers and more prone to fracture into small fragments (Figure 7D). The liberated starch particles eventually accumulate in the environment with the MPs formed from the fragmentation of the polymer matrix (Figure 7E). Of note, some of these small particles were fossil-fuel-based MPs, such as MPs generated by PE/TPS and PP/TPS. Thus, the fragmentation of polymer blends after photodegradation may generate persistent MPs, causing problems similar to those of neat fossil-fuel-based plastics.
4.3 Marine environmental risks and utilization recommendations for polymer blends
For biobased plastics to serve as a substitute for fossil-fuel-based plastics, their photodegradation performance needs to be well understood, especially with respect to biobased polymer blends, which are likely to take a significant share of the bioplastics market (European Bioplastics, 2021). Our results indicated that biobased polymer blends (i.e., PE/TPS, PP/TPS, and PLA/PBAT/TPS) are more likely to generate small-sized particles than neat polymers (i.e., PE, PP, and PLA) after photodegradation (Figure 1). Photodegradation may accelerate the release of MPs hidden in polymer blends, which may pose a higher ecological risk to the marine environment than neat polymers. Furthermore, MPs derived from biobased polymer blends, such as fossil-fuel-based MPs generated by PE/TPS and PP/TPS, can persist for decades in the marine environment. Although MPs derived from PLA/PBAT/TPS do biodegrade, they are relatively less biodegradable in the marine environment because their biodegradation is triggered in composting conditions with high temperatures or abundant microorganisms (Narancic et al., 2018). Moreover, photodegradation causes changes in the physicochemical properties of MPs derived from biobased polymer blends and affects their fate in the ocean (Wallmann et al., 2021; Luo et al., 2022b). For example, photodegradation can decrease the hydrophilicity of PP/TPS and increase its negative surface charge. The holes and pits formed on the surface of aged PP/TPS may provide more available reaction sites for the adsorption of pollutants (Wei et al., 2021b). Therefore, the potential vector effects of aged PP/TPS on coexisting pollutants might also be a significant concern. Taken together, special concerns over the MP risk were needed during the photodegradation of biobased polymer blends in the marine environment.
Knowledge of the photodegradation of biobased polymer blends can guide the development and application of biobased plastics to ensure a positive impact on plastic waste management and a minimal impact on the environment when inadvertently released (Narancic et al., 2018). As such, we suggest that fossil-fuel-based polymers should not be added into biodegradable polymers, especially when the polymer blends are intended for disposable products, such as PE/TPS and PP/TPS for single-use plastic bags or disposable tableware. Despite the biodegradability of TPS, MPs originating from the fossil-fuel-based polymer matrix remain persistent in the environment, which needs to be taken into consideration when developing biobased plastics. For polymer blends similar to PLA/PBAT/TPS, it is necessary to require that the manufacturers provide information on the polymer composition. This information is crucial to understanding the formation of MPs when polymer blends undergo weathering processes such as photodegradation, oxidation, mechanical abrasion, and hydrolysis. In summary, caution should be taken in the development, production, use, and management of biobased polymer blends.
Photodegradation is a critical pathway for the transformation of contaminants trapped in the MPs (Paluselli et al., 2019). Previous research has found that UV irradiation can trigger the photodegradation of commercially available polystyrene (PS) in seawater, leading to leaching of monomers and chemical additives such as phthalates (e.g., dibutyl phthalate [DBP] and di-isobutyl phthalate [DiBP]) (Wang et al., 2020). Another research demonstrated that organotin compounds (i.e., monomethyltin [MMT] and monobutyltin [MBT]) released from polyvinyl chloride (PVC) MPs were rapidly photodegraded under UV/visible light irradiation (Chen et al., 2019a). As plastic items photodegrade into small fragments or MPs, heavy metals (e.g., lead, chromium, and cadmium) may also be released into the marine environment (Turner et al., 2020). These studies showed that the photodegradation of MPs in the marine environment led to the leaching of diversified and toxic additives and degradation products. Up to now, toxic effects of additives derived from fossil-fuel-based polymers on aquatic organisms at different trophic levels have been widely verified (Chen et al., 2019b; Huang et al., 2021). Nevertheless, the photolytic transformation of endogenous contaminants (e.g., plasticizers, organotin catalysts, and heavy metals) of the biobased polymer blends and their potential toxicity to aquatic organisms required to be further studied.
5 Conclusions and environmental implications
This study investigated the formation of MPs and changes in the physicochemical properties of biobased polymer blends and their corresponding neat polymers after photodegradation in seawater. It was found that polymer blends were less resistant to UV irradiation and showed a higher degree of fragmentation after photodegradation than neat polymers; this resulted in more small-sized particles that may pose a higher ecological risk to the marine environment. From the evidence of changes in the surface morphology, contact angle, and surface charge of MPs derived from biobased polymer blends, we deduced that the photodegradation of polymer blends may have a greater negative effect on the marine environment than neat polymers. Thus, special attention should be paid to the risk associated with the MPs generated by the photodegradation of biobased polymer blends in the marine environment. Moreover, caution should be taken in the development, production, use, and management of biobased polymer blends to ensure that they have a minimal impact on the marine environment when inadvertently released.
Data availability statement
The original contributions presented in the study are included in the article/Supplementary Material. Further inquiries can be directed to the corresponding authors.
Author contributions
SZ: Investigation, data curation, formal analysis, methodology, writing-original draft. LL: Investigation, methodology, writing-review & editing. CL: Conceptualization, supervision, formal analysis, writing-review & editing. HZ: Writing-review & editing, funding acquisition. YL: Investigation, methodology. LP: Investigation, formal analysis. QL: Investigation, formal analysis. HYZ: Investigation, formal analysis. CS: Investigation, writing-review & editing. LC: Methodology, visualization. FL: Resources, formal analysis, funding acquisition, writing-review & editing. All authors contributed to the article and approved the submitted version.
Funding
This research was supported by the Hainan Provincial Joint Project of Sanya Yazhou Bay Science and Technology City (220LH061), Hainan Provincial Key Research and Development Projects (ZDYF2022SHFZ018), and National Natural Science Foundation of China (42077115).
Conflict of interest
The authors declare that the research was conducted in the absence of any commercial or financial relationships that could be construed as a potential conflict of interest.
Publisher’s note
All claims expressed in this article are solely those of the authors and do not necessarily represent those of their affiliated organizations, or those of the publisher, the editors and the reviewers. Any product that may be evaluated in this article, or claim that may be made by its manufacturer, is not guaranteed or endorsed by the publisher.
Supplementary material
The Supplementary Material for this article can be found online at: https://www.frontiersin.org/articles/10.3389/fmars.2022.1046179/full#supplementary-material
References
Afkhami A., Rezaei M., Malmiri H. J. (2019). Effect of chitosan incorporation on crystallinity, mechanical and rheological properties, and photodegradability of PE/TPS blends. J. Thermoplast. Compos. 34, 780–800. doi: 10.1177/0892705719854490
Ainali N. M., Kalaronis D., Evgenidou E., Kyzas G. Z., Bobori D. C., Kaloyianni M., et al. (2022). Do poly (lactic acid) microplastics instigate a threat? a perception for their dynamic towards environmental pollution and toxicity. Sci. Total Environ. 832, 155014. doi: 10.1016/j.scitotenv.2022.155014
Ajitha A. R., Thomas S. (2020). “Introduction: Polymer blends, thermodynamics, miscibility, phase separation, and compatibilization,” in Compatibilization of polymer blends. Eds. Ajitha A. R., Thomas S. (Elsevier: Amsterdam), 1–29.
Bocchini S., Fukushima K., Blasio A. D., Fina A., Frache A., Geobaldo F. (2010). Polylactic acid and polylactic acid-based nanocomposite photooxidation. Biomacromolecules. 11, 2919–2926. doi: 10.1021/bm1006773
Borrelle S. B., Ringma J., Law K. L., Monnahan C. C., Lebreton L., McGivern A., et al. (2020). Predicted growth in plastic waste exceeds efforts to mitigate plastic pollution. Science. 369, 1515–1518. doi: 10.1126/science.aba3656
Cai L. Q., Wang J. D., Peng J. P., Wu Z. Q., Tan X. L. (2018). Observation of the degradation of three types of plastic pellets exposed to UV irradiation in three different environments. Sci. Total Environ. 628–629, 740–747. doi: 10.1016/j.scitotenv.2018.02.079
Cao W. M., Wu N. N., Qu R. J., Sun C., Huo Z. L., Ajarem J. S., et al. (2021). Oxidation of benzophenone-3 in aqueous solution by potassium permanganate: kinetics, degradation products, reaction pathways, and toxicity assessment. Environ. Sci. pollut. Res. 28, 31301–31311. doi: 10.1007/s11356-021-12913-x
Carbery M., O’Connor W., Thavamani P. (2018). Trophic transfer of microplastics and mixed contaminants in the marine food web and implications for human health. Environ. Int. 115, 400–409. doi: 10.1016/j.envint.2018.03.007
Chen Y., Awasthi A. K., Wei F., Tan Q. Y., Li J. H. (2021). Single-use plastics: Production, usage, disposal, and adverse impacts. Sci. Total Environ. 752, 141772. doi: 10.1016/j.scitotenv.2020.141772
Chen C. Z., Chen L., Yao Y., Artigas F., Huang Q. H., Zhang W. (2019a). Organotin release from polyvinyl chloride microplastics and concurrent photodegradation in water: Impacts from salinity, dissolved organic matter, and light exposure. Environ. Sci. Technol. 53, 10741–10752. doi: 10.1021/acs.est.9b03428
Chen C. Z., Chen L., Huang Q. H., Chen Z. Y., Zhang W. (2019b). Organotin contamination in commercial and wild oysters from China: Increasing occurrence of triphenyltin. Sci. Total Environ. 650, 2527–2534. doi: 10.1016/j.scitotenv.2018.09.310
Elizalde-Velázquez G. A., Gómez-Oliván L. M. (2021). Microplastics in aquatic environments: A review on occurrence, distribution, toxic effects, and implications for human health. Sci. Total Environ. 780, 146551. doi: 10.1016/j.scitotenv.2021.146551
European Bioplastics (2021) Bioplastics market data. Available at: https://www.european-bioplastics.org/market (Accessed August 31, 2022).
Fan X. L., Zou Y. F., Geng N., Liu J. Q., Hou J., Li D. D., et al. (2021). Investigation on the adsorption and desorption behaviors of antibiotics by degradable MPs with or without UV ageing process. J. Hazard. Mater. 401, 123363. doi: 10.1016/j.jhazmat.2020.123363
Gao R. R., Sun C. M. (2021). A marine bacterial community capable of degrading poly(ethylene terephthalate) and polyethylene. J. Hazard. Mater. 416, 125928. doi: 10.1016/j.jhazmat.2021.125928
Gewert B., Plassmann M. M., MacLeod M. (2015). Pathways for degradation of plastic polymers floating in the marine environment. Environ. Sci.: Processes Impacts. 17, 1513–1521. doi: 10.1039/c5em00207a
Geyer R., Jambeck J. R., Law K. L. (2017). Production, use, and fate of all plastics ever made. Sci. Adv. 3, e1700782. doi: 10.1126/sciadv.1700782
Ghosh K., Jones B. H. (2021). Roadmap to biodegradable plastics-current state and research needs. ACS Sustain. Chem. Eng. 9, 6170–6187. doi: 10.1021/acssuschemeng.1c00801
Haider T. P., Völker C., Kramm J., Landfester K., Wurm F. R. (2019). Plastics of the future? the impact of biodegradable polymers on the environment and on society. Angew. Chem. Int. Ed. 58, 50–62. doi: 10.1002/anie.201805766
Halle A. T., Ladirat L., Gendre X., Goudouneche D., Pusineri C., Routaboul C., et al. (2016). Understanding the fragmentation pattern of marine plastic debris. Environ. Sci. Technol. 50, 5668–5675. doi: 10.1021/acs.est.6b00594
Hartmann N. B., Hüffer T., Thompson R. C., Hasselloüv M., Verschoor A., Daugaard A. E., et al. (2019). Are we speaking the same language? recommendations for a definition and categorization framework for plastic debris. Environ. Sci. Technol. 53, 1039–1047. doi: 10.1021/acs.est.8b05297
Hohn S., Acevedo-Trejos E., Abrams J. F., Moura J., Spranz R., Merico A. (2020). The long-term legacy of plastic mass production. Sci. Total Environ. 746, 141115. doi: 10.1016/j.scitotenv.2020.141115
Horton A. A. (2022). Plastic pollution: When do we know enough? J. Hazard. Mater. 422, 126885. doi: 10.1016/j.jhazmat.2021.126885
Huang W., Song B., Liang J., Niu Q. Y., Zeng G. M., Shen M. C., et al. (2021). Microplastics and associated contaminants in the aquatic environment: A review on their ecotoxicological effects, trophic transfer, and potential impacts to human health. J. Hazard. Mater. 405, 124187. doi: 10.1016/j.jhazmat.2020.124187
Isimjan T. T., Wang T. Y., Rohani S. (2012). A novel method to prepare superhydrophobic, UV resistance and anti-corrosion steel surface. Chem. Eng. J. 210, 182–187. doi: 10.1016/j.cej.2012.08.090
Kim T., Park K., Hong J. (2022). Understanding the hazards induced by microplastics in different environmental conditions. J. Hazard. Mater. 424, 127630. doi: 10.1016/j.jhazmat.2021.127630
Liao J., Chen Q. Q. (2021). Biodegradable plastics in the air and soil environment: Low degradation rate and high microplastics formation. J. Hazard. Mater. 418, 126329. doi: 10.1016/j.jhazmat.2021.126329
Lin J. L., Yan D. Y., Fu J. W., Chen Y. H., Ou H. (2020). Ultraviolet-c and vacuum ultraviolet inducing surface degradation of microplastics. Water Res. 186, 116360. doi: 10.1016/j.watres.2020.116360
Liu G. C., Zheng H., Jiang Z. X., Zhao J., Wang Z. Y., Pan B., et al. (2018). Formation and physicochemical characteristics of nano biochar: Insight into chemical and colloidal stability. Environ. Sci. Technol. 52, 10369–10369. doi: 10.1021/acs.est.8b01481
Luo H. W., Liu C. Y., He D. Q., Xu J., Sun J. Q., Li J., et al. (2022b). Environmental behaviors of microplastics in aquatic systems: A systematic review on degradation, adsorption, toxicity and biofilm under aging conditions. J. Hazard. Mater. 423, 126915. doi: 10.1016/j.jhazmat.2021.126915
Luo Y. D., Sun C. Z., Li C. G., Liu Y. F., Zhao S. S., Li Y. Y., et al. (2022a). Spatial patterns of microplastics in surface seawater, sediment, and sand along qingdao coastal environment. Front. Mar. Sci. 9. doi: 10.3389/fmars.2022.916859
Narancic T., Verstichel S., Chaganti S. R., Morales-Gamez L., Kenny S. T., Wilde B. D., et al. (2018). Biodegradable plastic blends create new possibilities for end-of-Life management of plastics but they are not a panacea for plastic pollution. Environ. Sci. Technol. 52, 10441–10452. doi: 10.1021/acs.est.8b02963
Nazareth M., Marques M. R. C., Leite M. C. A., Castro Í.B. (2019). Commercial plastics claiming biodegradable status: Is this also accurate for marine environments? J. Hazard. Mater. 366, 714–722. doi: 10.1016/j.jhazmat.2018.12.052
Nor N. H. M., Kooi M., Diepens N. J., Koelmans A. A. (2021). Lifetime accumulation of microplastic in children and adults. Environ. Sci. Technol. 55, 5084–5096. doi: 10.1021/acs.est.0c07384
Oliveira M., Santos E., Araújo A., Fechine G. J. M., Machado A. V. G., Botelho G. (2016). The role of shear and stabilizer on PLA degradation. Polym. Test. 51, 109–116. doi: 10.1016/j.polymertesting.2016.03.005
Paluselli A., Fauvelle V., Galgani F., Sempéré R. (2019). Phthalate release from plastic fragments and degradation in seawater. Environ. Sci. Technol. 53, 166–175. doi: 10.1021/acs.est.8b05083
Pilař J., Michálková D., Šlouf M., Vacková T. (2015). Long-term accelerated weathering of HAS stabilized PE and PP plaques: Compliance of ESRI, IR, and microhardness data characterizing heterogeneity of photooxidation. Polym. Degrad. Stab. 120, 114–121. doi: 10.1016/j.polymdegradstab.2015.06.011
Quispe M. M., López1 O. V., Villar M. A. (2019). Oxidative degradation of thermoplastic starch induced by UV radiation. J. Renew. Mater. 7, 383–391. doi: 10.32604/jrm.2019.04276
Raee E., Avid A., Kaffashi B. (2020). Effect of compatibilizer concentration on dynamic rheological behavior and morphology of thermoplastic starch/polypropylene blends. J. Appl. Polym. Sci. 137, 48742. doi: 10.1002/app.48742
Sanusi O. M., Benelfellah A., Papadopoulos L., Terzopoulou Z., Bikiaris D. N., Aït Hocine N. (2021). Properties of poly(lactic acid)/montmorillonite/carbon nanotubes nanocomposites: determination of percolation threshold. J. Mater. Sci. 56, 16887–16901. doi: 10.1007/s10853-021-06378-z
Shen M. C., Song B., Zeng G. M., Zhang Y. X., Huang W., Wen X. F., et al. (2020). Are biodegradable plastics a promising solution to solve the global plastic pollution? Environ. pollut. 263, 114469. doi: 10.1016/j.envpol.2020.114469
Song Y. K., Hong S. H., Jang M., Han M. G., Jung S. W., Shim W. J. (2017). Combined effects of UV exposure duration and mechanical abrasion on microplastic fragmentation by polymer type. Environ. Sci. Technol. 51, 4368–4376. doi: 10.1021/acs.est.6b06155
Tarani E., Črešnar K. P., Zemljič L. F., Chrissafis K., Papageorgiou G. Z., Lambropoulou D., et al. (2021). Cold crystallization kinetics and thermal degradation of pla composites with metal oxide nanofillers. Appl. Sci. 11, 3004. doi: 10.3390/app11073004
Tsuji H., Echizen Y., Nishimura Y. (2006). Photodegradation of biodegradable polyesters: A comprehensive study on poly(L-lactide) and poly(ϵ-caprolactone). Polym. Degrad. Stab. 91, 1128–1137. doi: 10.1016/j.polymdegradstab.2005.07.007
Turner A., Holmes L., Thompson R. C., Fisher A. S. (2020). Metals and marine microplastics: Adsorption from the environment versus addition during manufacture, exemplified with lead. Water Res. 173, 115577. doi: 10.1016/j.watres.2020.115577
Wallmann L., Krampe J., Lahnsteiner J., Radu E., Rensburg P. V., Slipko K., et al. (2021). Fate and persistence of antibiotic-resistant bacteria and genes through a multi-barrier treatment facility for direct potable reuse. Water Reuse. 11, 373–390. doi: 10.2166/wrd.2021.097
Wang X., Zheng H., Zhao J., Luo X. X., Wang Z. Y., Xing B. S. (2020). Photodegradation elevated the toxicity of polystyrene microplastics to grouper (Epinephelus moara) through disrupting hepatic lipid homeostasis. Environ. Sci. Technol. 54, 6202–6212. doi: 10.1021/acs.est.9b07016
Wei X. F., Bohlén M., Lindblad C., Hedenqvist M., Hakonen A. (2021a). Microplastics generated from a biodegradable plastic in freshwater and seawater. Water Res. 198, 117123. doi: 10.1016/j.watres.2021.117123
Wei X. F., Nilsson F., Yin H., Hedenqvist M. S. (2021b). Microplastics originating from polymer blends: An emerging threat? Environ. Sci. Technol. 55, 4190–4193. doi: 10.1021/acs.est.1c00588
Wu X. W., Liu P., Shi H. H., Wang H., Huang H. Y., Shi Y. Q., et al. (2021). Photo aging and fragmentation of polypropylene food packaging materials in artificial seawater. Water Res. 188, 116456. doi: 10.1016/j.watres.2020.116456
Yoksan R., Dang K. M., Boontanimitr A., Chirachanchai S. (2021). Relationship between microstructure and performances of simultaneous biaxially stretched films based on thermoplastic starch and biodegradable polyesters. Int. J. Biol. Macromol. 190, 141–150. doi: 10.1016/j.ijbiomac.2021.08.206
Zaaba N. F., Jaafar M. (2020). A review on degradation mechanisms of polylactic acid: Hydrolytic, photodegradative, microbial, and enzymatic degradation. Polym. Eng. Sci. 60, 2061–2075. doi: 10.1002/pen.25511
Zhang K., Hamidian A. H., Tubić A., Zhang Y., Fang J. K. H., Wu C. X., et al. (2021a). Understanding plastic degradation and microplastic formation in the environment: A review. Environ. pollut. 274, 116554. doi: 10.1016/j.envpol.2021.116554
Zhang X. L., Xia M. L., Su X. J., Yuan P., Li X. K., Zhou C. Y., et al. (2021b). Photolytic degradation elevated the toxicity of polylactic acid microplastics to developing zebrafish by triggering mitochondrial dysfunction and apoptosis. J. Hazard. Mater. 413, 125321. doi: 10.1016/j.jhazmat.2021.125321
Keywords: microplastic, photodegradation, polymer blends, neat polymers, thermoplastic starch
Citation: Zhao S, Liu L, Li C, Zheng H, Luo Y, Pang L, Lin Q, Zhang H, Sun C, Chen L and Li F (2022) Photodegradation of biobased polymer blends in seawater: A major source of microplastics in the marine environment. Front. Mar. Sci. 9:1046179. doi: 10.3389/fmars.2022.1046179
Received: 16 September 2022; Accepted: 09 November 2022;
Published: 29 November 2022.
Edited by:
Xiaoshan Zhu, Hainan University, ChinaReviewed by:
Daniel Rittschof, Duke University, United StatesZhuanxi Luo, Huaqiao University China
Lanfang Han, Guangdong University of Technology, China
Copyright © 2022 Zhao, Liu, Li, Zheng, Luo, Pang, Lin, Zhang, Sun, Chen and Li. This is an open-access article distributed under the terms of the Creative Commons Attribution License (CC BY). The use, distribution or reproduction in other forums is permitted, provided the original author(s) and the copyright owner(s) are credited and that the original publication in this journal is cited, in accordance with accepted academic practice. No use, distribution or reproduction is permitted which does not comply with these terms.
*Correspondence: Fengmin Li, bGlmZW5nbWluQG91Yy5lZHUuY24=; Chenguang Li, bGljaGVuZ3VhbmdAb3VjLmVkdS5jbg==