- 1South China Sea Bio-Resource Exploitation and Utilization Collaborative Innovation Center, Guangdong Provincial Key Laboratory of Marine Resources and Coastal Engineering, School of Marine Sciences, Sun Yat-Sen University, Guangzhou, China
- 2State Key Laboratory of Biocontrol, School of Life Sciences, Sun Yat-Sen University, Guangzhou, China
- 3Neilingding-Futian National Nature Reserve of Guangdong Province, Shenzhen, China
- 4School of Science and Technology, Hong Kong Metropolitan University, Hong Kong, Hong Kong SAR, China
- 5State Key Laboratory of Marine Pollution and Department of Chemistry, City University of Hong Kong, Hong Kong, Hong Kong SAR, China
Sediment biofilms, mainly composed of diatoms, bacteria, and related extracellular polymeric substances (EPS), play important roles in mangrove ecosystems. In order to better understand the microbial biodiversity in marine environment, this study aims to clarify the effects of wetland, season, and tide on benthic biofilms and related sediment properties based on in situ investigation in two mangrove wetlands (Futian and Qi’ao) in the Pearl River Estuary, South China. Owing to the tide current, the northeastern Futian is more influenced by seawater than the southwestern Qi’ao. As a consequence of the salinity-driven accumulation of nutrients in sediments, Futian is more eutrophic than Qi’ao. Compared to Qi’ao, the higher nutrimental condition in Futian conserves higher abundance of eutrophic indicators of Nitzschia and Cyclotella, which results in higher values of all pigments and bound polysaccharide with varied monosaccharide composition. Compared to the seaward site, the landward site has higher abundance of benthic (pennate) diatoms but lower abundance of planktonic (centric) diatoms due to the varied settling velocity by tidal disturbance. This varied diatom composition leads to higher levels of bound polysaccharide and more monosaccharides in the landward site than in the seaward site. Compared to late spring (March), early summer (May) appears to have similar diatom abundance but more EPS fractions (except bound protein) and monosaccharides due to the higher level of light intensity and temperature. The observed lower bacterial abundance in May than in March might be due to the higher predation pressure. The baseline data on biofilm compositions in mangrove ecosystems from this study can improve the understanding of microbial biodiversity in response to the tidal, temporal, and spatial changes.
Introduction
Biofilms are defined as aggregates of microorganisms in which cells are frequently embedded in a selfproduced matrix of extracellular polymeric substances (EPS) that are adherent to each other and/or a surface (Vert et al., 2012). As a “microbial skin”, biofilms drive crucial ecosystem processes and contribute to the biogeochemical cycling of carbon and nitrogen on Earth (Decho, 2000; Battin et al., 2016). Biofilms in intertidal sediments are complex systems with high cell densities ranging from 108 to 1011 cells/g wet weight and are typically composed of microalgae (mainly diatom) and prokaryotes (mainly bacteria) (Flemming et al., 2016; Stal et al., 2019). In biofilms, EPS act as crucial intermediaries that drive symbiotic interactions in bacterial–microalgal consortia, such as tolerance towards external stresses, cell–cell communication and collective behavior, and synergetic use of nutrients (Gerbersdorf et al., 2020; Perera et al., 2022). Epipelic diatoms (Bacillariophyceae) are the main producers of sediment EPS, and their composition depends on the environmental conditions and stresses (Smith and Underwood, 1998; Underwood et al., 2004; Bellinger et al., 2005).
Mangroves are intertidal forests along tropical and subtropical coastlines, providing a wide range of ecosystem services, such as fisheries support, coastal protection, and carbon sequestration (Lovelock et al., 2015). Although mangroves occupy only 0.5% of the global coastal area (~138,000 km2), they contribute 10%–15% (24 Tg C year−1) to coastal sediment carbon storage and export 10%–11% of the particulate terrestrial carbon to the ocean (Alongi, 2014). The Pearl River Delta (PRD) is currently considered as one of the highly polluted regions in South China. At the same time, it also conserves several subtropical mangrove forests. Historically, many urban and industrial centers were established adjacent to estuaries fringed by mangroves. Mangrove swamps had been used as convenient dumping sites for waste generated from human activities (Tam, 2006). The nutrients and contaminants from the upstream Pearl River and/or from flushing tidal water were likely to retain in these mangrove sediments (Wang et al., 2013; Liu and Wong, 2013; Zhang et al., 2014). As an intermediate habitat between terrestrial land and oceans, the mangrove wetland ecosystem has unique ecological functions, including purification of land-derived nutrients/pollutants and buffering wave actions to prevent coastal line erosion (Wang and Gu, 2021).
The sediment biofilms in mangrove ecosystems are likely to have a considerable capacity to withstand the inundation by tides and adjust to temporal and spatial changes. The gradient variations of bacterial diversity and metabolic function were observed from high tidal flat to mid/low tidal flat in in an artificial mangrove wetland (Yin and Yan, 2020) and mangrove-inhabited mudflat (Zhang et al., 2018). Microeukaryotic community structure was related to the spatial heterogeneity of mangrove sediment geochemistry due to tidal zonation and the rhizosphere effect (Zhu et al., 2018). Significant seasonal variations of the microphytobenthos community structure were observed in mangrove ecosystems along the southwest coast of India (Benny et al., 2021). Salinity and nutrients were the most significant variables related to diatom composition in surface sediments of Can Gio Mangrove Forest, Southern Vietnam (Costa-Boddeker et al., 2017). Besides microbial composition, the inhabited biofilms in mangrove sediments, especially EPS, are rarely characterized under the effects of environmental and spatiotemporal factors. To better understand the microbial biodiversity in marine environment, based on the in situ investigation in two mangrove wetlands (Futian and Qi’ao) in South China, this study clarified the effects of tide, season, and wetland on sediment biofilms and related sediment properties.
Materials and methods
Study areas and sample collection
As shown in Figure 1, Futian mangrove nature reserve (22°31’ N, 114°0’ E), situated at the northeastern coast of the estuary of the Pearl River Delta, South China, was selected as the representative sampling site. In the upstream Pearl River, the runoff during the wet season (from April to September) accounted for 75% of the annual flow and was nearly three times the runoff during the dry season (from October to March in the next year) (Zhen et al., 2016). Thus, two cruises in 2018 during moderate tide were carried out in March (temperature at 17–24°C and daylight hours of 12 h 2 min) and May (temperature at 25–31°C and daylight hours of 13 h 12 min), representing late spring and early summer, respectively.
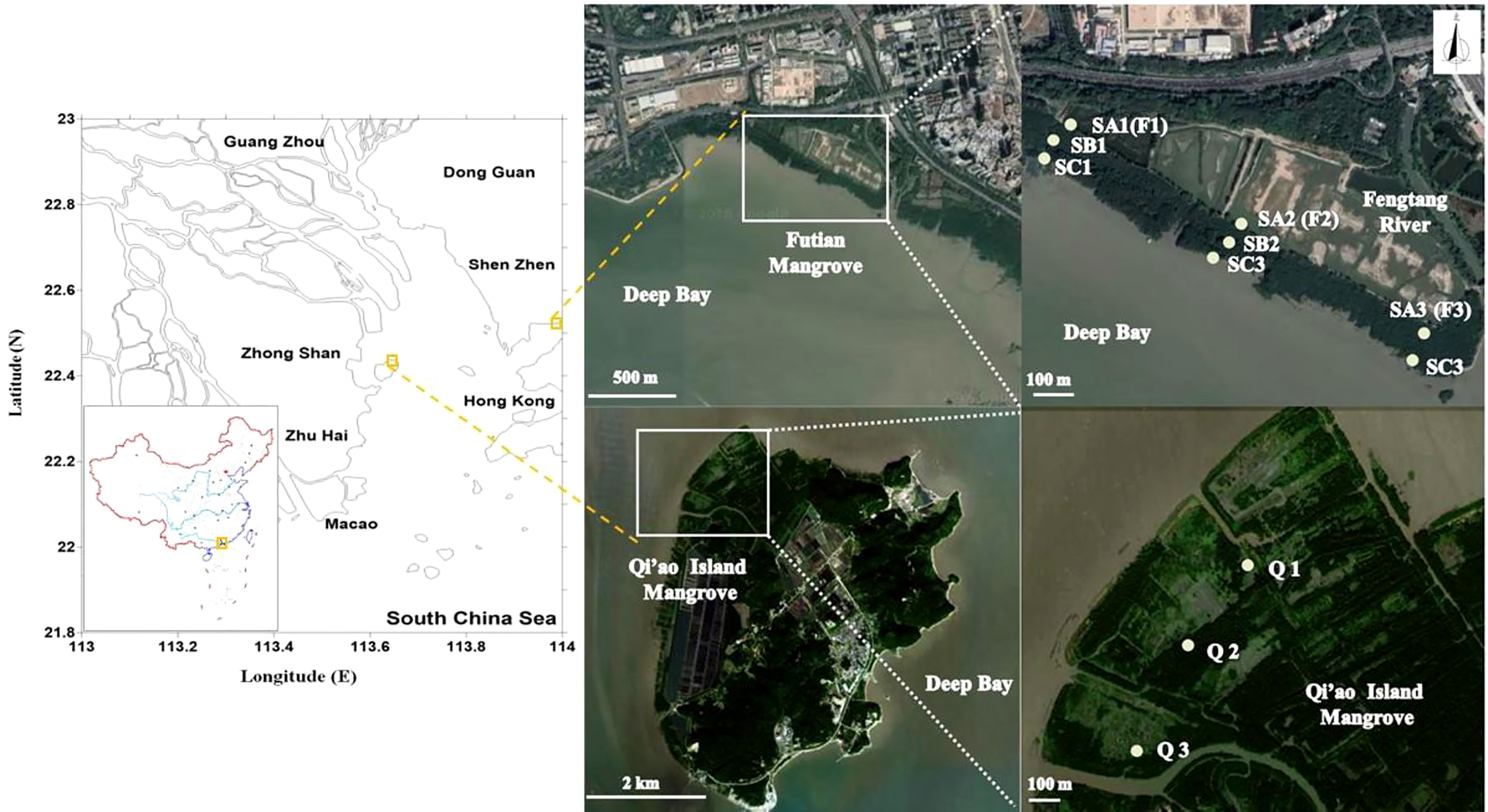
Figure 1 The sketch map showing sampling sites in Futian and Qi’ao mangrove wetlands. Sediments collected from sites 1, 2, and 3 in Futian were respectively marked as F1, F2, and F3 in spring samples and as SA1, SA2, and SA3 in summer samples, which were at the inner rim of the mature mangrove forest. In summer, the tidal gradient was additionally designed in each site with B in the middle (SB) and C in the outer rim (SC) of the mangrove forest. In Qi’ao, only summer samples were collected in three sites at the inner rim of the mature mangrove forest and labeled as Q1, Q2, and Q3.
In Futian, mangrove sediment samples were collected from three sites in the midtidal region, sites 1, 2, and 3, with a distance interval of approximately 1 km. All three sites were located at the inner rim of the mature mangrove forest near a wooden boardwalk. In addition, site 1 was adjacent to a ditch that was used as a drain outlet a decade ago (personal communication). The samples collected in March were marked as F1, F2, and F3 for sites 1, 2, and 3, respectively. Furthermore, the May samples were labeled as SA1, SA2, and SA3. In May, in addition to inner rim samples (SA), samples at a rectilinear direction to the seaward in each site were also collected to investigate the effect of tidal levels. They were labeled with prefixes SB and SC for samples collected in the middle and in the outer rim of the mangrove forest, respectively. Due to the difficulty in sampling in dense forest area, SB sample of site 3 (SB3) was not available. Comparatively, Qi’ao Island (22°26’ N, 113°37’ E), situated along the southwestern coasts of this estuary, was selected as another sampling site. In the Qi’ao mangrove wetland, samples were also collected only on May from three sites in the midtidal region, sites 1, 2 and 3, at the inner rim of the mature mangrove forest near a wooden board, and labeled as Q1, Q2, and Q3, respectively.
The mangrove sediments were collected under the canopy formed by the mature mangrove trees at low tides. Triplicate samples of approximately 0.5 kg fresh weight of 0–1 cm surface sediment in each site were randomly collected within a 1 × 1 m2 quadrat using stainless steel shovels. Salinity was determined in situ by a salinometer (Thermo Scientific™ Elite, Thermo Fisher Scientific, USA). Following the manual removal of stone, root, litter, and predators, the collected sediments were brought back to the laboratory at 4°C and stored in brown sampling bags. Each sediment sample was well mixed and then submitted to the same process for the determination of sediment properties, bacterial and diatom abundances, and EPS concentrations.
Determination of sediment properties including pigments
A portion of the sediments were freeze-dried and then submitted for the determination of sediment properties including pigments in all samples. Total organic matter (TOM) was measured by the loss on ignition of the freeze-dried sediment at 550°C in a muffle furnace. Elemental analysis of C and N by a Vario EL cube (Elementar, Germany) was performed at the Instrumental Analysis & Research Center in Sun Yat-Sen University (authorized by the Certification and Accreditation Administration of the PRC with MA 17002100896), while P was analyzed by inductively coupled plasma–atomic emission spectrometry (ICP-AES, iCAP6500Duo, ThermoFisher, USA). Pigments were extracted from the freeze-dried sediment using 90% acetone, incubated overnight in the dark, and centrifuged at 3,500 g, and the absorbance of pigment in the acetone extract was determined using a spectrophotometer (2600A, Unico, USA). Absorbance values were converted to the concentration of each pigment in the sediment (μg/g) following the ASTM international standard D3731-87 (2004) (ASTM International, 2004).
Abundance of bacteria and diatoms
The bacterial abundance was determined in fresh sediments in all samples by the direct counting method after 4′,6-diamidino-2-phenylindole (DAPI) staining (Porter and Feig, 1980; Yang et al., 2020). The bacterial number was counted using an epifluorescence microscope (Leica DM5000B, Wetzlar, Germany) with blue excitation light (450–490 nm) at a magnification of 10 × 100 under oil. Accurate amounts of 100 mg of freeze-dried sediments were digested by 37% hydrogen peroxide to remove organic matter, then by 10% hydrochloric acid to remove carbonate particles (Renberg, 1990). The digested sediments were diluted to 10 ml with ultrapure water. Permanent slides were prepared by pipetting the constant volume sample of 200 μl onto a coverslip and subsequently mounting onto a glass slide using the Naphrax® (refractive index = 1.7). For each sample, at least three slides were prepared and a total of 450–500 valves were counted. Diatoms in the slides were identified and calculated completely using an optical microscope equipped with a digital camera (DM 5000B, Leica, German). All diatoms were identified at 400× magnification to the taxonomic level of genus based on the morphologic characteristics of the frustules according to the Algaebase (https://www.algaebase.org/). The genus of diatoms was also confirmed under a scanning electron microscope (SEM, GeminiSEM 500, ZEISS, German), followed by gold coating.
Extraction and determination of EPS fractions
Colloidal and bound EPS in all samples were extracted from fresh sediment immediately after collection based on the Dowex-resin method (Takahashi et al., 2009; Yang et al., 2020). Twenty milliliters of artificial seawater (ASW) was added to 20 ml of fresh sediment, continuously agitated for 1 h at 4°C in the dark, and centrifuged at 3,500 rpm at 4°C for 15 min, and the supernatant was considered as colloidal fraction. The sediment pellet was then added with 20 ml of ASW and 1 g of activated Dowex 50WX8 (hydrogen form, 200–400 mesh, Sigma-Aldrich, MO, USA), mixed gently for 1 h in the dark, and centrifuged to obtain the supernatant representing the bound fraction. The colloidal and bound EPS were precipitated with the addition of −20°C ethanol and gently mixed overnight. The precipitate was obtained by centrifuging and then freeze-dried for further analysis. The polysaccharide concentration was quantified by a phenol sulfuric acid assay using glucose as the standard (DuBois et al., 1956). The protein concentration was determined using a modified bicinchoninic acid (BCA) protein assay kit (Sangon Biotech, China) with bovine serum albumin (BSA) as the standard (Smith et al., 1987). The limits of detection for polysaccharides and proteins were 2.63 and 2.46 mg/g (w/w, dry weight basis of EPS), respectively.
Neutral monosaccharide analysis
The monosaccharide composition in colloidal and bound EPS in all samples was determined according to the method from Pierre et al., 2010. Ten milligrams of lyophilized EPS was re-dissolved in 1 ml of 2 M HCl, added with 20 μl of 1 M myo-inositol (purity > 98%, Sigma) and heated at 90°C for 4 h in a water bath. The preparation was then dried by gentle nitrogen flow and derivatized at 60°C for 60 min with the addition of 50 μl of derivatization reagent (BSTFA:TMCS = 99:1) and 50 μl of pyridine. The derivatized products were identified and determined by an Agilent 7890A gas chromatograph coupled with a 5975C mass spectrometer (GC-MS) using a DB-5-MS capillary column (30 m × 0.25 mm × 0.25 mm). Quantification was based on the peak area and retention time of a mixed monosaccharide standard (purity > 99.9%, Supelco) with myo-inositol as the internal standard. The LODs of D(−)ribose (RIB), D(+)xylose (XYL), D(−)arabinose (ARA), D(+)galactose (GAL), D(+)mannose (MAN), and D(+)glucose (GLU) were 5.7, 3.5, 1.7, 4.6, 7.8, and 10.9 μg/g (w/w, dry weight basis of EPS), respectively. The monosaccharides in colloidal and bound EPS were abbreviated in the following text as C_ and B_, respectively.
Statistical analyses
The statistical analyses and graphics were performed based on Origin 8.5, Spyder (Python 3.8), and RStudio (R 3.6.1). In each sediment and each parameter, mean ± standard deviation (s.d.) of three replicates were calculated. The differences in each parameter among three tidal gradients in Futian (SA, SB, and SC) were tested by a parametric one-way analysis of variance (ANOVA), and post-hoc test was performed to determine exactly where the differences were if the ANOVA was significant at p < 0.05. The assumption of the parametric test was checked and no data transformation was needed. The differences between two mangrove wetlands (SA and Q), as well as between the two sampling times in Futian (SA and F), were determined by Student’s t-test. Sediment properties, microbial abundance, and EPS components were compared using nonmetric multidimensional scaling (NMDS) analysis by means of Bray–Curtis similarities. The analysis of similarities (ANOSIM) with 999 random permutations was also performed to assess the distance of samples based on Bray–Curtis similarities.
Results
Sediment properties including pigments
Insignificant differences of salinity were observed among SA, SB, and SC, and between SA and F, indicated that sediment salinity in Futian exhibited limited tidal and seasonal difference (Figure 2A). However, Futian was more influenced by seawater than Qi’ao, as shown by salinity, which was higher at 3 ± 0.7‰ in SA than at 1‰ in Q. Pearson correlation analysis showed positive correlation between salinity with TC, TN, and TP (Figure S1). Concentrations of TOM and nutrimental elements (TC, TN, and TP) were also significantly higher in Futian (SA) than in Qi’ao (Q) (Figures 2B–E), indicating more eutrophic environment in the Futian mangrove wetland. In Futian, these nutrimental elements appeared to have an insignificant difference along with tidal and seasonal conditions, while TOM was significantly higher in May (SA) than in March (F). Among all samples, SA1 appeared to have the highest concentrations of TOM, TC, and TN but not TP (Figure S2). The reason might be SA1 is located near a ditch and historically received discharges from human activities. It was worth noting that the concentrations of TOM in SA1 (255.3 ± 43.0 μg/g) were exceptionally higher than those in the other samples (81.3 to 163.3 μg/g).
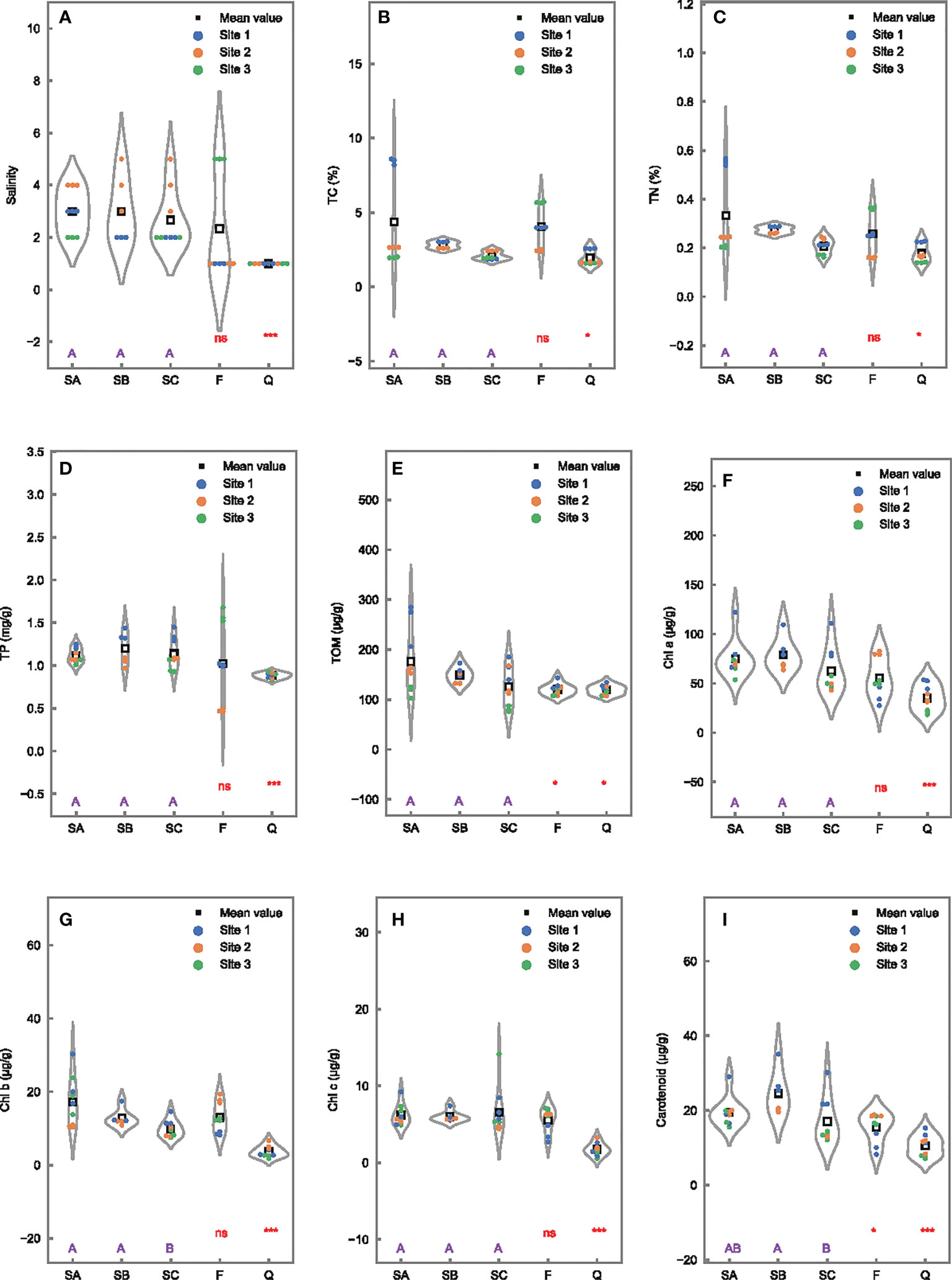
Figure 2 Differences of properties in 0- to 1-cm surface sediments among tidal levels in Futian (SA, SB, and SC), between sampling times (SA vs. F), and between Futian and Qi’ao wetlands (SA vs. Q). Different letter shows significant differences among tidal levels at p < 0.05 according to one-way ANOVA, while * and *** indicate significant differences between times, as well as between mangrove wetlands at p < 0.05, and 0.001, respectively, ns indicates not significant difference, according to Student’s t-test: (A) salinity; (B) TC; (C) TN; (D) TP; (E) TOM; (F) Chl a; (G) Chl b; (H) Chl c; and (I) carotenoid.
The summed concentrations of the four pigments (including Chl a, b, c, and carotenoid) varied from 31.6 to 140.2 μg/g in all sediment samples (Figure S3). Among these pigments, Chl a was the most dominant with a composition percentage ranging from 58.3% to 73.5%. Comparatively, the composition percentages of carotenoid, Chl b, and Chl c were only 15.1%–24.0%, 4.7%–17.4%, and 2.4%–10.0%, respectively. The concentrations of all pigments appeared to have significant differences in Futian (SA) compared to Qi’ao (Q), with higher concentrations in SA (Figures 2F–I). In Futian, significant tidal effects were found in Chl b and carotenoid with higher concentrations in the landward (SA or SB) than in the seaward (SC) sites. In addition, significant seasonal effects were observed in carotenoid with higher value in May (SA) than in March (F).
Abundance of diatoms and bacteria
The differences in the total abundance of diatoms among different tidal levels (SA, SB, and SC) and between seasons (SA vs. F) were insignificant (Figure 3A). However, an obvious variation was observed with a higher abundance of the total diatoms in Futian (SA, 13.2 ± 2.5 × 106 cells/g) than in Qi’ao (Q, 6.5 ± 1.2 × 106 cells/g). The bacterial number did not indicate any significant differences among different tidal levels and between Futian and Qi’ao wetlands in samples collected in May, with values ranging from 1.5 to 14.0 × 108 cells/g (Figure 3B). However, the bacterial number exhibited a significant difference between two sampling times, with up to one order of magnitude higher at (27.9–57.4) × 108 cells/g in March samples (F).
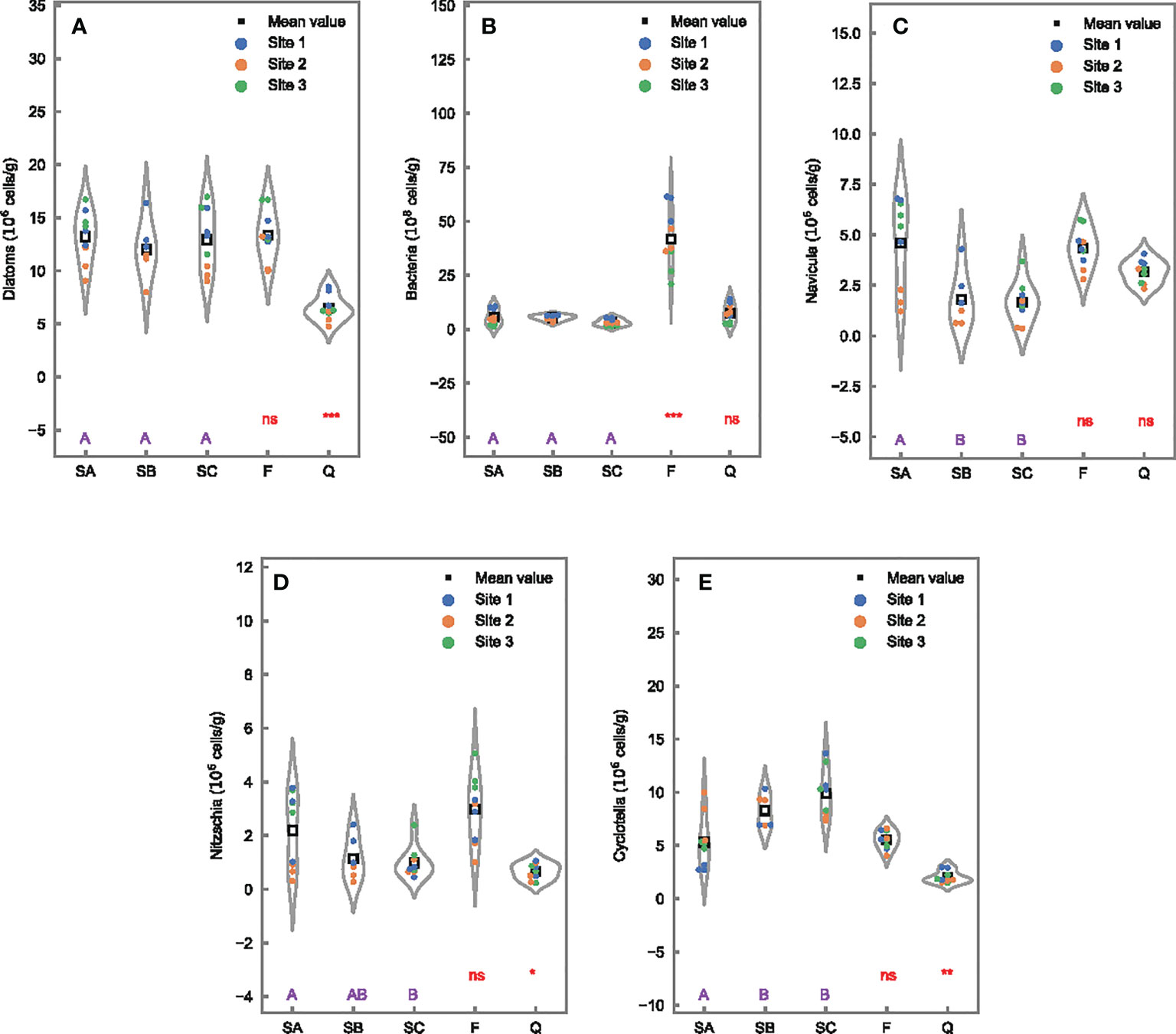
Figure 3 Microbial composition in 0- to 1-cm surface sediments and differences of microbial abundance among tidal levels in Futian (SA, SB, and SC), between sampling times (SA vs. F), and between Futian and Qi’ao wetlands (SA vs. Q). Different letter shows significant differences among tidal levels at p < 0.05 according to one-way ANOVA, while *, **, and *** indicate significant differences between times, as well as between mangrove wetlands, at p < 0.05, 0.01, and 0.001, respectively, ns indicates not significant difference, according to Student’s t-test: (A) diatoms; (B) bacteria; (C) Navicula; (D) Cyclotella; and (E) Nitzschia.
Furthermore, significant tidal differences were found in the dominant diatom genera, with more Navicula and Nitzschia in landward (SA) than in seaward (SB or SC) sites (Figures 3C, D), while an opposite trend was observed for Cyclotella with a lower concentration in SA (Figure 3E). An obvious wetland difference was observed between Futian (SA) and Qi’ao (Q) with higher abundance of Nitzschia and Cyclotella in SA than in Q, and comparable abundance of Navicula. The abundance of the three dominant taxa was relatively stable between the two sampling times (SA vs. F).
Bound protein and saccharides
The concentration of protein in bound EPS ranged from 22.6 to 38.4 mg/g and maintained insignificant difference among tidal, seasonal, and wetland samples (Figure 4A. However, polysaccharide in bound EPS appeared to have significant differences among three tidal levels (SA vs. SC), two sampling times (SA vs. F), and two wetlands (SA vs. Q), with higher concentrations in SA (17.5 ± 8.4 mg/g) than in SC (8.5 ± 3.4 mg/g), F (5.3 ± 1.3 mg/g), and Q (6.5 ± 2.1 mg/g) (Figure 4B). The most monosaccharides in bound EPS, except XYL, were significantly affected by tidal conditions with lower concentrations in seaward (SC) than in landward (SA or SB) sites in Futian (Figures 4C–H). In addition, the concentrations of GAL, ARA, and XYL in bound EPS were higher in SA than in F, while GAL, RIB, and XYL were lower in SA than in Q.
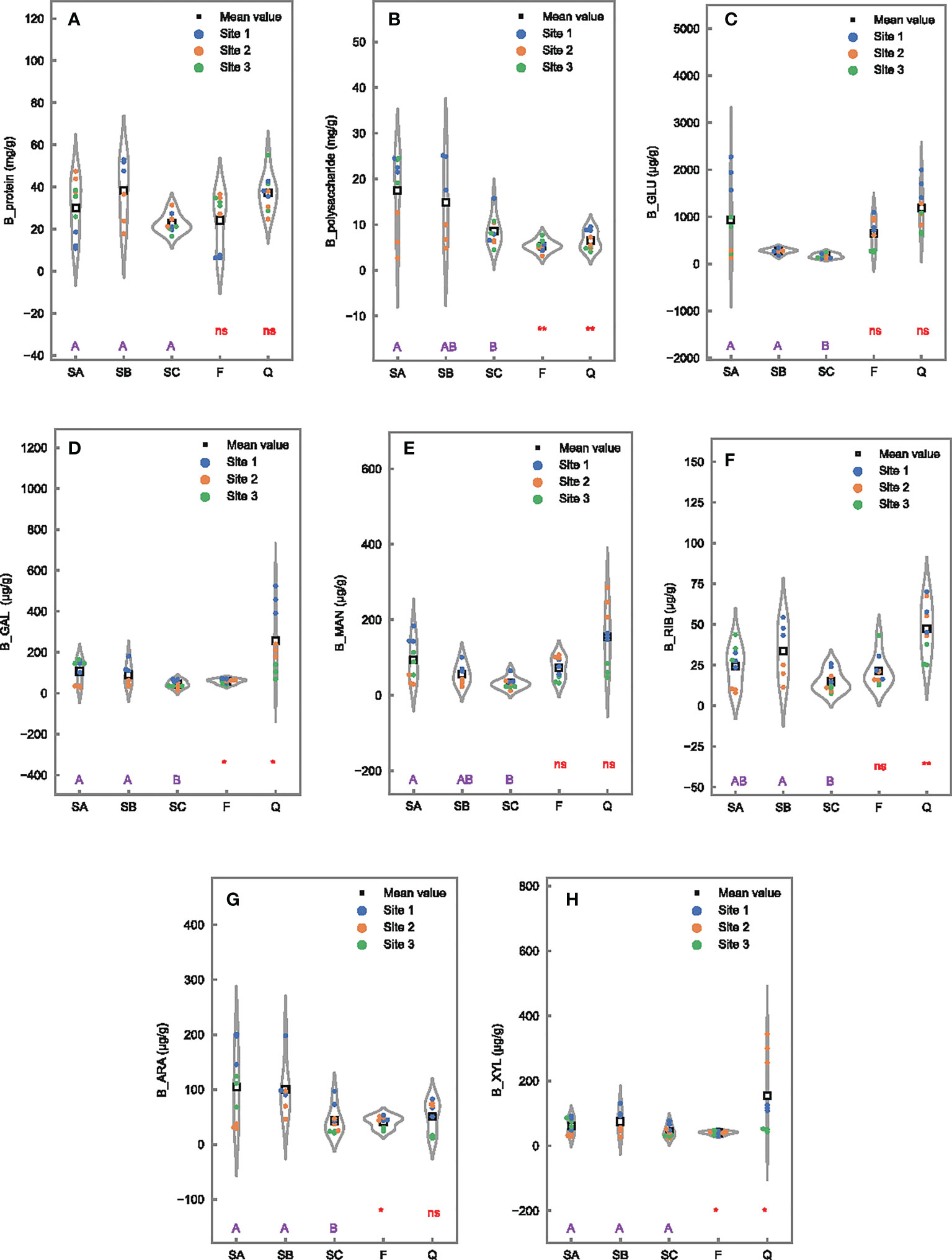
Figure 4 Differences of EPS constituents in bound fraction in 0- to 1-cm surface sediments among tidal levels in Futian (SA, SB, and SC), between sampling times (SA vs. F), and between Futian and Qi’ao wetlands (SA vs. Q). Different letter shows significant differences among tidal levels at p < 0.05 according to one-way ANOVA, while * and ** indicate significant differences between times, as well as between mangrove wetlands, at p < 0.05 and 0.01, respectively, ns indicates not significant difference, according to Student’s t-test: (A) B_protein; (B) B_polysaccharide; (C) B_GLU; (D) B_GAL; (E) B_MAN; (F) B_RIB; (G) B_ARA; and (H) B_XYL.
Colloidal protein and saccharides
The concentration of protein in colloidal EPS was higher at 17.5 ± 7.2 mg/g in the SB sample in Futian than the comparable level of SA (7.6 ± 1.9 mg/g) and SC (8.1 ± 2.3 mg/g) (Figure 5A). Protein in colloidal EPS exhibited an insignificant difference between two mangrove wetlands, while significantly seasonal differences were higher in May (SA) than in March (F) samples. The differences of polysaccharide in colloidal EPS among different tidal locations and between the two mangrove wetlands were insignificant, with concentrations at 9.7–14.1 mg/g (Figure 5B). However, colloidal polysaccharide was also significantly affected by seasonal conditions with higher concentrations in May (SA) than in March (F) samples. Almost all monosaccharides in colloidal EPS were significantly affected by tidal, seasonal, and wetland conditions, with lower concentrations in seaward (SC) than in landward sites (SA and SB), higher concentrations in May (SA) than in March (F), and higher values in Futian (SA) than in Qi’ao (Q) (Figures 5C–H).
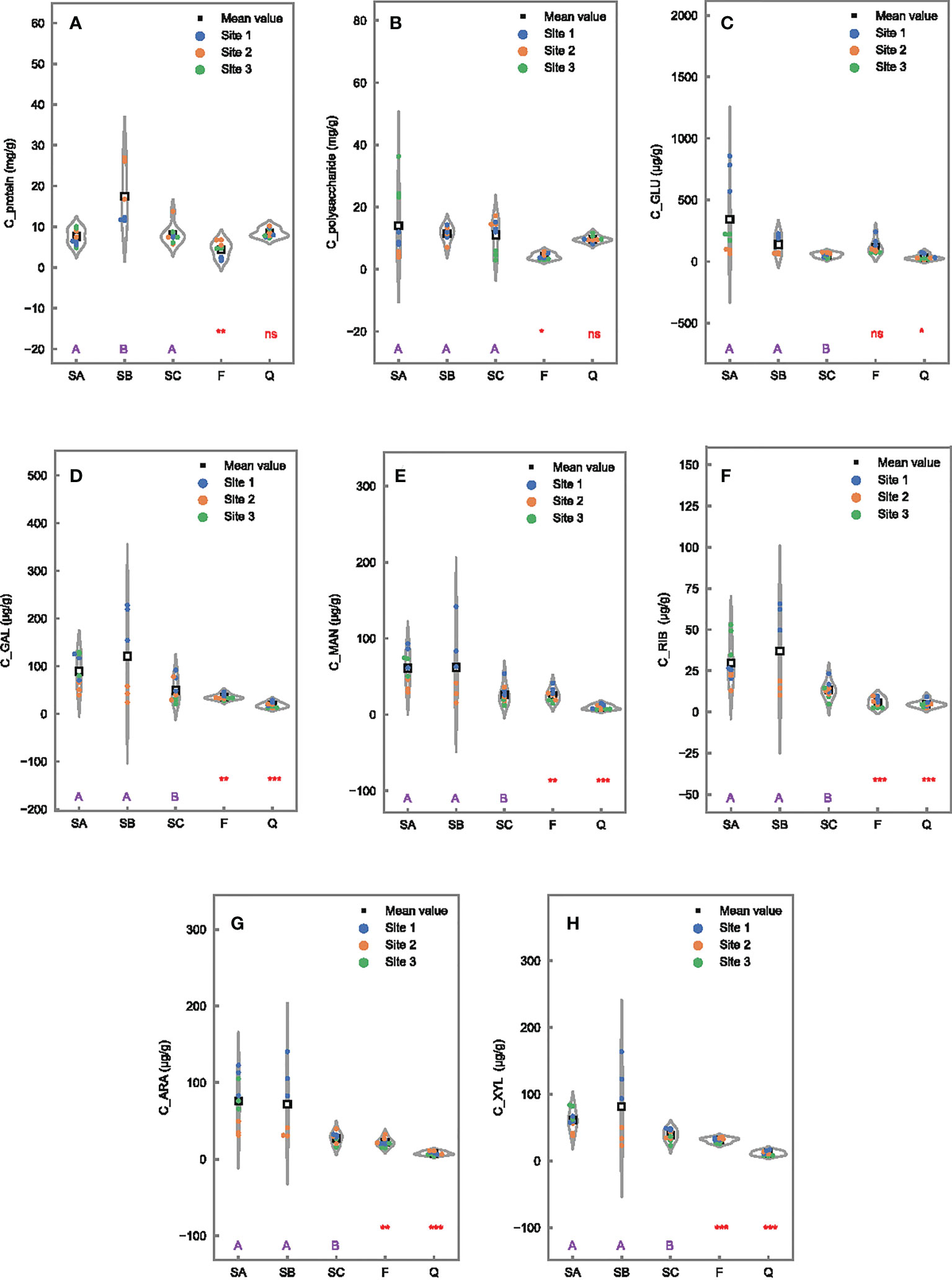
Figure 5 Differences of EPS constituents in colloidal fraction in 0- to 1-cm surface sediments among tidal levels in Futian (SA, SB, and SC), between sampling times (SA vs. F), and between Futian and Qi’ao wetlands (SA vs. Q). Different letter shows significant differences among tidal levels at p < 0.05 according to one-way ANOVA, while *, **, and *** indicate significant differences between times, as well as between mangrove wetlands at p < 0.05, 0.01, and 0.001, respectively, ns indicates not significant difference, according to Student’s t-test: (A) C_protein; (B) C_polysaccharide; (C) C_GLU; (D) C_GAL; (E) C_MAN; (F) C_RIB; (G) C_ARA; and (H) C_XYL.
Assemblage analysis
The NMDS plots were acceptable with a stress of 0.1490 for sediment properties, 0.1193 for microbial abundance, and 0.0970 for EPS components (Figures 6A–C). A large overlap among samples of SA, SB, and SC in the three sub-figures indicated that the dissimilarities among different tidal levels of sediment properties, microbial abundance, and EPS components were not significant. However, SA was well separated from F in Figures 6B, C, indicating significant seasonal effects in microbial abundance and EPS components. The separation between SA and Q was clear in Figures 6A, C, indicating significant effects from wetland conditions on the sediment properties and the EPS components.
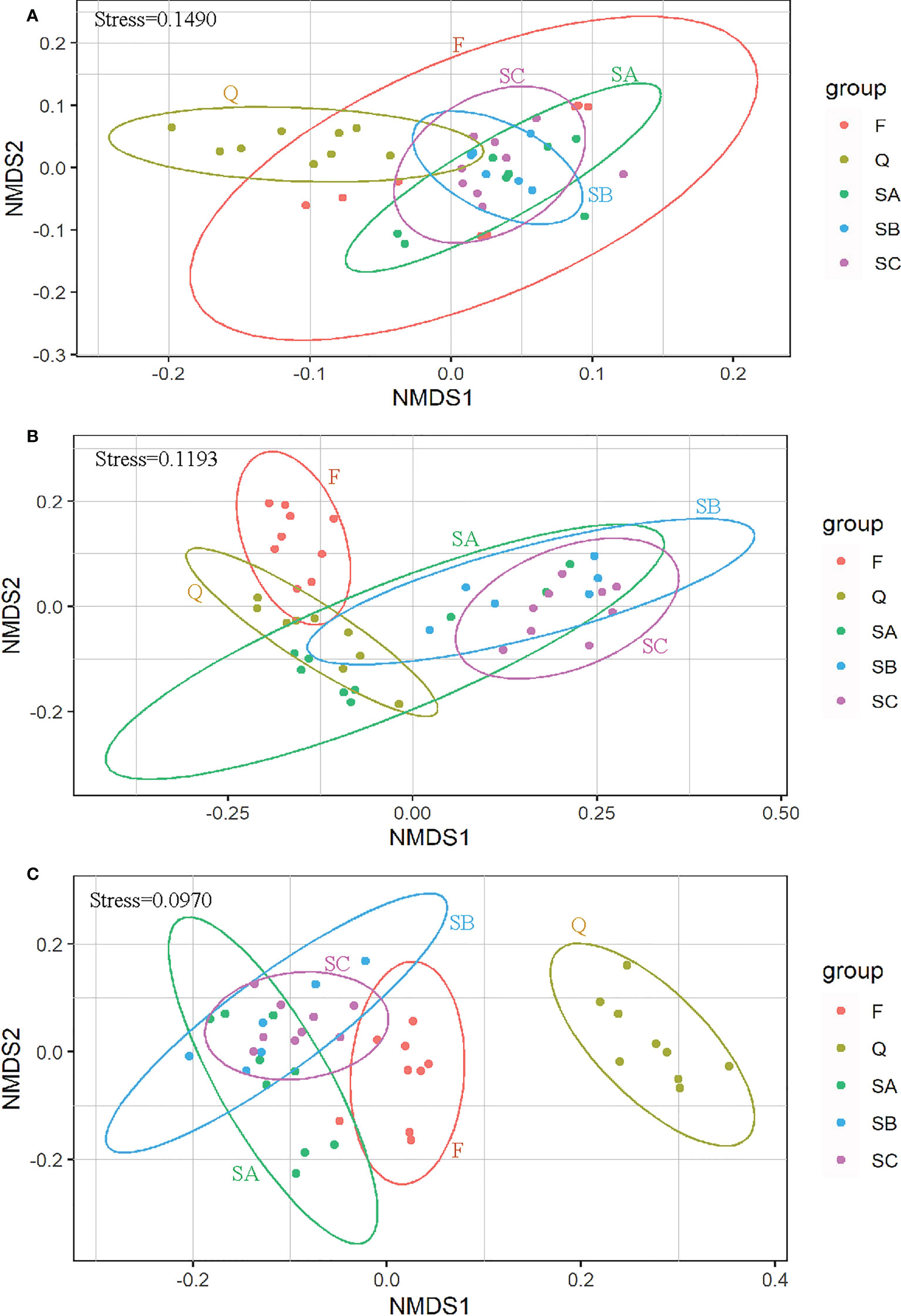
Figure 6 Non-metric multidimensional scaling (NMDS) plot depicting Bray–Curtis dissimilarity among these groups in (A) sediment properties including pigments, (B) microbial abundance, and (C) EPS components.
ANOSIM results indicated significant differences in sediment properties (R = 0.308, p = 0.001), microbial abundance (R = 0.742, p = 0.001), and EPS components (R = 0.505, p = 0.001) among all samples (Table 1). Furthermore, significant tidal effect was found in microbial abundance between SA and SC, in EPS components between SB and SC as well as SA and SC. Significant seasonal and wetland effects were also observed in sediment properties, microbial abundance, and EPS components.
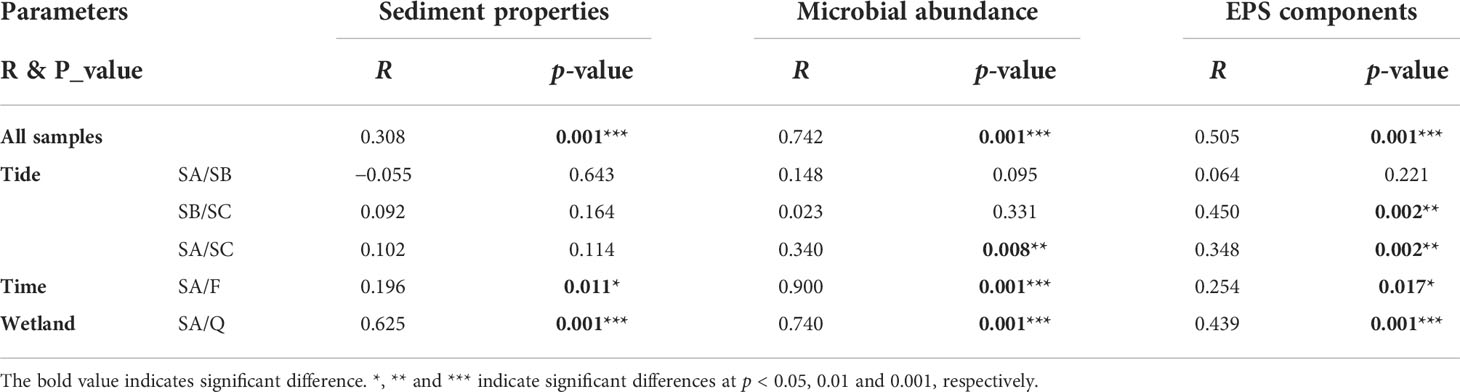
Table 1 ANOSIM results based on Bray–Curtis distance showing the influences of tide, season, and wetland on sediment properties, microbial abundance, and EPS components.
Discussion
Higher nutrients in Futian than in Qi’ao conserve more diatom abundance and B_polysaccharide concentration
With higher values of salinity and nutrimental elements, Futian (SA) was more influenced by seawater and more eutrophic than Qi’ao (Q). The tide current analysis in this estuary indicated that freshwater outflow occurred via the western channel, while the salt water intrusion occurred mainly via the eastern channel (Mao et al., 2004). This water circulation led to the more significant effects from overlying seawater to the northeastern part (Futian wetland) than the southwestern part (Qi’ao) of the estuary. Due to the “salting-out effect”, the aqueous solubility of chemicals would reduce and more chemicals would attract to the non-aqueous phase of suspended particles and sediment (Yang et al., 2016). The positive correlation between salinity and nutrimental elements indicated this salinity-driven accumulation of nutrients in mangrove sediment by seawater, which led to the observation that Futian was more eutrophic than Qi’ao. The concentrations of nutrients and organic matter in sediments reflected the net results of interactions among many biogeochemical factors, including pollutant inputs, plant uptake, releases from litter decomposition and microbial transformation, tidal flushing, and leaching (Tam, 2006). In addition, the higher concentrations of nutrients in SA1 might be attributed to the historic input of untreated wastewater from surrounding factories and illegally constructed houses at the landward side of Futian mangrove wetland in previous years (based on personal communication from a senior staff member in this reserve).
Due to the limited mobility, the growth of diatoms was primarily driven by region-specific parameters such as nutrients (TC, TN, and TP). Compared to Qi’ao, the eutrophic Futian appeared to have higher abundance of the total diatoms and thus higher values of all pigments, accompanied by higher abundance of Nitzschia and Cyclotella and comparable abundance of Navicula. Several diatom species belonging to Nitzschia and Cyclotella were indicators for eutrophic conditions. Therefore, the abundance of these groups was higher at sampling sites with higher nutrient concentrations. Compared to the centric diatom, pennate diatoms with raphes were the main EPS producers with prominent roles in the process of sediment biostabilization (de Brouwer et al., 2005). B_polysaccharide and almost all colloidal monosaccharides appeared to have significant differences between two mangrove wetlands with higher amounts in Futian than in Qi’ao. The EPS biosynthesis of these monosaccharide components in colloidal fraction might be favored by the higher nutrient concentrations and diatom abundance in Futian. The other possible reason was attributed to the difference in monosaccharide composition between the two wetlands, as indicated by the significant difference in all C_monosaccharides and the insignificant variation in C_polysaccharide. However, such wetland difference in B_monosaccharides was contrary to the difference in B_polysacharide. This indicated significant variations in the monosaccharide composition between these two wetlands.
Higher benthic diatoms in landward than in seaward sites leads to higher B_polysaccharide concentration
In Futian, the differences in the total abundance of diatoms among different tidal levels were insignificant. However, significant differences were observed in dominant diatom genera with more benthic (pennate) diatoms of Navicula and Nitzschia in landward (SA) than in seaward (SB or SC) sites, while an opposite trend was found in planktonic (centric) diatom of Cyclotella. Navicula and Nitzschia were highly motile taxa and tended to be benthic in sediment while Cyclotella was mostly planktonic (Kuczynska et al., 2015). Compared to planktonic taxa, more abundance of benthic (pennate) diatoms accumulated in landward than in seaward sites. This was probably due to the higher capacity of benthic (pennate) diatoms attaching onto different types of substrates (e.g., sediments and plants). This might also be caused by the effects of environmental factors (e.g., hydrodynamics, turbidity, and grain size) on the settling of planktonic taxon from water column to sediment. Microalgae characteristically found in benthic habitats with greater specific gravities might settle faster than those usually present as planktonic (Stevenson, 1996). This might lead to more benthic species accumulated in the high-tide line while more planktonic species in the low-tide line after the ebb. Compared to the centric diatom, pennate diatoms with raphes were the main EPS producers with prominent roles in the process of sediment biostabilization (de Brouwer et al., 2005). The higher abundance of pennate diatoms in landward than in seaward sites led to the observed higher levels of B_polysaccharide and most monosaccharides in the landward site. The tidal effect on diatom composition mentioned above might be attributed to the tidal effect on monosaccharides in bound fraction, as monosaccharide composition considerably differed in each pennate and centric diatom (Gugi et al., 2015). Hanlon et al. (2006) reported the potential loss of the carbohydrate components by washaway during tidal cover over the tidal emersion–immersion period. This might be another reason for the lower concentration of carbohydrates in seaward than in landward sites in this study.
Higher bacterial abundance but lower EPS concentrations in March than in May
In Futian, comparable diatom abundances were observed in early summer (May, SA) and late spring (March, F). However, significantly different (up to one order of magnitude) bacterial abundances were observed in these two seasons. Sivri et al. (2012) also observed that the bacterial density in the Gulf of Gemlik (Marmara Sea) reduced from spring to summer. Orvain et al. (2014) found that the lower abundance of bacteria in summer coincided with a high abundance of the gastropod Peringia ulvae having intense grazing at the Brouage mudflat (France). In this study, the lower bacterial abundance in the mangrove sediment in May was possibly due to the higher predation pressure. Most EPS fractions and related monosaccharides, except bound protein, were significantly affected by seasonal conditions in Futian with higher concentrations in summer than in spring. A similar seasonal effect was also reported in the freshwater biofilms cultured in summer with the highest polysaccharide and proteins, while the spring biofilms contained the lowest value (Wang et al., 2019). More C_protein was found during summer in surface sediments of a diatom-dominated intertidal mudflat (Marennes–Oléron, France) (Pierre et al., 2014). In order to dissipate excess energy and connect to dissimilatory metabolic pathways, colloidal EPS was probably produced as a result of unbalanced growth (Stal, 2010). As the abundance and composition of diatoms maintained at relatively stable values between two sampling times, it might be possible that the more optimal condition with higher light intensity and temperature in May favored the production of polysaccharide in benthic microalgae. The lower abundance of bacteria in May than March samples might be another key factor contributing to the time difference of B_polysaccharide, as bacteria were found to be the main consumer of EPS (Miyatake et al., 2014).
Conclusion
This research investigated the biofilm compositions and sediment properties at different tidal levels, two sampling times, and two mangrove wetlands in South China. Futian was more influenced by seawater and eutrophic than Qi’ao, leading to higher values of diatom abundance, related pigments, B_polysaccharide, and monosaccharides in Futian. B_protein was the most dominant fraction in EPS and was maintained at relatively steady levels in mangrove sediments, while the other EPS fractions (including C_protein, C_polysaccharide, and B_polysaccharide) accompanied by their monosaccharide components varied considerably with tidal levels, sampling times, and wetlands. More pennate diatoms accumulated in landward than in seaward sites, but the seaward site had more centric diatoms. This difference of diatom composition and potential tidal loss led to the lower concentrations of B_polysaccharide and more monosaccharide components in the seaward site. Comparable abundance of diatoms but less bacteria was observed in May than in March, which contributed to the higher concentrations of fresh OMs and most EPS fractions and their monosaccharide components in May. This is the first study reporting the baseline data on sediment biofilms and related environmental parameters in mangrove sediments in response to the variation of wetland, tide, and season.
Data availability statement
The raw data supporting the conclusions of this article will be made available by the authors, without undue reservation.
Author contributions
LY: Writing and funding acquisition. QiaY: Investigation. PL: Visualization. LL: Validation. QioY: Investigation. TL: Conceptualization. NT: Review and editing. All authors contributed to the article and approved the submitted version.
Funding
This work was supported by the National Natural Science Foundation of China (Nos. 22176224 and 21876211), the Guangdong Provincial Natural Science Foundation (No. 2021A1515011397), the Key-Area Research and Development Program of Guangdong Province (No. 2020B1111350003), and the Research Grants Council of the Hong Kong SAR [No. UGC/IDS(R)16/19].
Conflict of interest
The authors declare that the research was conducted in the absence of any commercial or financial relationships that could be construed as a potential conflict of interest.
Publisher’s note
All claims expressed in this article are solely those of the authors and do not necessarily represent those of their affiliated organizations, or those of the publisher, the editors and the reviewers. Any product that may be evaluated in this article, or claim that may be made by its manufacturer, is not guaranteed or endorsed by the publisher.
Supplementary material
The Supplementary Material for this article can be found online at: https://www.frontiersin.org/articles/10.3389/fmars.2022.1043826/full#supplementary-material
References
Alongi D. M. (2014). Carbon cycling and storage in mangrove forests. Annu. Rev. Mar. Sci. 6, 195–219.
ASTM International (2004). Standard practices for measurement of chlorophyll content of algae in surface waters. designation: D Vol. 2004) (United States: The ASTM Committee on Standards) 3731–3787.
Battin T. J., Besemer K., Bengtsson M. M., Romani A. M., Packmann A. I. (2016). The ecology and biogeochemistry of stream biofilms. Nat. Rev. Microbiol. 14 (4), 251–263.
Bellinger B. J., Abdullahi A. S., Gretz M. R., Underwood G. J. C. (2005). Biofilm polymers: relationship between carbohydrate biopolymers from estuarine mudflats and unialgal cultures of benthic diatoms. Aquat. Microb. Ecol. 38 (2), 169–180.
Benny N., Thomas L. C., Padmakumar K. B. (2021). Community structure of microphytobenthos associated with mangrove ecosystems along the southwest coast of India. Estuar. Coast. 44 (5), 1380–1391.
Costa-Boddeker S., Thuyen L. X., Schwarz A., Huy H. D., Schwalb A. (2017). Diatom assemblages in surface sediments along nutrient and salinity gradients of thi vai estuary and can gio mangrove forest, southern Vietnam. Estuar. Coast. 40 (2), 479–492.
de Brouwer J. F. C., Wolfstein K., Ruddy G. K., Jones T. E. R., Stal L. J. (2005). Biogenic stabilization of intertidal sediments: The importance of extracellular polymeric substances produced by benthic diatoms. Microb. Ecol. 49 (4), 501–512.
Decho A. W. (2000). Microbial biofilms in intertidal systems: An overview. Cont. Shelf Res. 20 (10-11), 1257–1273.
DuBois M., Gilles K. A., Hamilton J. K., Rebers P. A., Smith F. (1956). Colorimetric method for determination of sugars and related substances. Anal. Chem. 28 (3), 350–356.
Flemming H. C., Wingender J., Szewzyk U., Steinberg P., Rice S. A., Kjelleberg S. (2016). Biofilms: An emergent form of bacterial life. Nat. Rev. Microbiol. 14 (9), 563–575.
Gerbersdorf S. U., Koca K., de Beer D., Chennu A., Noss C., Risse-Buhl U., et al. (2020). Exploring flow-biofilm-sediment interactions: Assessment of current status and future challenges. Water Res. 185, 116182.
Gugi B., Le Costaouec T., Burel C., Lerouge P., Helbert W., Bardor M. (2015). Diatom-specific oligosaccharide and polysaccharide structures help to unravel biosynthetic capabilities in diatoms. Mar. Drugs 13 (9), 5993–6018.
Hanlon A. R. M., Bellinger B., Haynes K., Xiao G., Hofmann T. A., Gretz M. R., et al. (2006). Dynamics of extracellular polymeric substance (EPS) production and loss in an estuarine, diatom-dominated, microalgal biofilm over a tidal emersion-immersion period. Limnol. Oceanogr. 51 (1), 79–93.
Kuczynska P., Jemiola-Rzeminska M., Strzalka K. (2015). Photosynthetic pigments in diatoms. Mar. Drugs 13 (9), 5847–5881.
Liu J. L., Wong M. H. (2013). Pharmaceuticals and personal care products (PPCPs): A review on environmental contamination in China. Environ. Int. 59, 208–224.
Lovelock C. E., Cahoon D. R., Friess D. A., Guntenspergen G. R., Krauss K. W., Reef R., et al. (2015). The vulnerability of indo-pacific mangrove forests to sea-level rise. Nature 526 (7574), 559–565.
Mao Q. W., Shi P., Yin K. D., Gan J. P., Qi Y. Q. (2004). Tides and tidal currents in the pearl river estuary. Cont. Shelf Res. 24 (16), 1797–1808.
Miyatake T., Moerdijk-Poortvliet T. C. W., Stal L. J., Boschker H. T. S. (2014). Tracing carbon flow from microphytobenthos to major bacterial groups in an intertidal marine sediment by using an in situ c-13 pulse-chase method. Limnol. Oceanogr 59 (4), 1275–1287.
Orvain F., De Crignis M., Guizien K., Lefebvre S., Mallet C., Takahashi E., et al. (2014). Tidal and seasonal effects on the short-term temporal patterns of bacteria, microphytobenthos and exopolymers in natural intertidal biofilms (Brouage, France). J. Sea Res. 92, 6–18.
Perera I. A., Abinandan S., Subashchandrabose S. R., Venkateswarlu K., Cole N., Naidu R., et al. (2022). Extracellular polymeric substances drive symbiotic interactions in bacterial-microalgal consortia. Microb. Ecol. 83 (3), 596–607.
Pierre G., Graber M., Orvain F., Dupuy C., Maugard T. (2010). Biochemical characterization of extracellular polymeric substances extracted from an intertidal mudflat using a cation exchange resin. Biochem. Syst. Ecol. 38 (5), 917–923.
Pierre G., Zhao J. M., Orvain F., Dupuy C., Klein G. L., Graber M., et al. (2014). Seasonal dynamics of extracellular polymeric substances (EPS) in surface sediments of a diatom-dominated intertidal mudflat (Marennes-oleron, France). J. Sea Res. 92, 26–35.
Porter K., Feig Y. (1980). The use of DAPI for identifying and counting aquatic microflora. Limnol. Oceanogr. 25), 1943–1948.
Renberg I. (1990). A procedure for preparing large sets of diatom slides from sediment cores. J. Paleolimnol. 4), 87–90.
Sivri N., Balci M., Durmus T., Seker D. Z., Balkis N. (2012). Analysis of enteric bacteria distribution in the gulf of gemlik (Marmara Sea) by means of gis. Fresen. Environ. 21 (11), 3224–3232.
Smith P. K., Krohn R. I., Hermanson G. T., Mallia A. K., Gartner F. H., Provenzano M. D., et al. (1987). Measurement of protein using bicinchoninic acid. Anal. Biochem. 150 (1), 76–85.
Smith D. J., Underwood G. J. C. (1998). Exopolymer production by intertidal epipelic diatoms. Limnol. Oceanogr 43 (7), 1578–1591.
Stal L. J. (2010). Microphytobenthos as a biogeomorphological force in intertidal sediment stabilization. Ecol. Eng. 36 (2), 236–245.
Stal L. J., Bolhuis H., Cretoiu M. S. (2019). Phototrophic marine benthic microbiomes: the ecophysiology of these biological entities. Environ. Microbiol. 21 (5), 1529–1551.
Stevenson R. J. (1996). “An introduction to algal ecology in freshwater benthic habitats,” in Algal ecology: freshwater benthic ecosystems. Eds. Stevenson R. J., Bothwell M. L., Lowe R. L. (United States: Oxford University Press) 3–30.
Takahashi E., Ledauphin J., Goux D., Orvain F. (2009). Optimising extraction of extracellular polymeric substances (EPS) from benthic diatoms: comparison of the efficiency of six EPS extraction methods. Mar. Freshw. Res. 60 (12), 1201–1210.
Tam N. F. Y. (2006). “Pollution studies on mangroves in Hong Kong and mainland China,” in Environment in Asia pacific harbours. Ed. Wolanski ,. E. (The Netherlands: Springer) 147–163.
Underwood G. J. C., Boulcott M., Raines C. A., Waldron K. (2004). Environmental effects on exopolymer production by marine benthic diatoms: Dynamics, changes in composition, and pathways of production. J. Phycol 40 (2), 293–304.
Vert M., Doi Y., Hellwich K. H., Hess M., Hodge P., Kubisa P., et al. (2012). Terminology for biorelated polymers and applications (IUPAC recommendations 2012). Pure Appl. Chem. 84 (2), 377–408.
Wang Y. S., Gu J. D. (2021). Ecological responses, adaptation and mechanisms of mangrove wetland ecosystem to global climate change and anthropogenic activities. Int. Biodeter. Biodegr 162, 105248.
Wang L. T., Hua X. Y., Zhang L. W., Song N., Dong D. M., Guo Z. Y. (2019). Influence of organic carbon fractions of freshwater biofilms on the sorption for phenanthrene and ofloxacin: The important role of aliphatic carbons. Sci. Total Environ. 685, 818–826.
Wang S. L., Xu X. R., Sun Y. X., Liu J. L., Li H. B. (2013). Heavy metal pollution in coastal areas of south China: A review. Mar. pollut. Bull. 76 (1-2), 7–15.
Yang L. H., Cheng Q., Tam N. F. Y., Lin L., Su W. Q., Luan T. G. (2016). Contributions of abiotic and biotic processes to the aerobic removal of phenolic endocrine-disrupting chemicals in a simulated estuarine aquatic environment. Environ. Sci. Technol. 50 (8), 4324–4334.
Yang L. H., Xiao S. R., Yang Q., Luan T. G., Tam N. F. Y. (2020). Recovery of subtropical coastal intertidal system prokaryotes from a destruction event and the role of extracellular polymeric substances in the presence of endocrine disrupting chemical. Environ. Int. 144, 106023.
Yin Y. C., Yan Z. Z. (2020). Variations of soil bacterial diversity and metabolic function with tidal flat elevation gradient in an artificial mangrove wetland. Sci. Total Environ. 718, 137385.
Zhang X. Y., Hu B. X., Ren H. J., Zhang J. (2018). Composition and functional diversity of microbial community across a mangrove-inhabited mudflat as revealed by 16S rDNA gene sequences. Sci. Total Environ. 633, 518–528.
Zhang Z. W., Xu X. R., Sun Y. X., Yu S., Chen Y. S., Peng J. X. (2014). Heavy metal and organic contaminants in mangrove ecosystems of China: A review. Environ. Sci. pollut. R 21 (20), 11938–11950.
Zhen G. C., Li Y., Tong Y. D., Yang L., Zhu Y., Zhang W. (2016). Temporal variation and regional transfer of heavy metals in the pearl (Zhujiang) river, China. Environ. Sci. pollut. R. 23 (9), 8410–8420.
Keywords: mangrove sediments, diatoms, bacteria, polysaccharide, protein, nutrients
Citation: Yang L, Yang Q, Lin L, Luan T, Yang Q, Li P and Tam NFY (2022) Effects of wetland, tide, and season on benthic biofilms and related sediment properties in mangrove ecosystems. Front. Mar. Sci. 9:1043826. doi: 10.3389/fmars.2022.1043826
Received: 14 September 2022; Accepted: 24 October 2022;
Published: 10 November 2022.
Edited by:
Hao Cheng, South China Sea Institute of Oceanology (CAS), ChinaReviewed by:
Fulin Sun, South China Sea Institute of Oceanology (CAS), ChinaKe Pan, Shenzhen University, China
Copyright © 2022 Yang, Yang, Lin, Luan, Yang, Li and Tam. This is an open-access article distributed under the terms of the Creative Commons Attribution License (CC BY). The use, distribution or reproduction in other forums is permitted, provided the original author(s) and the copyright owner(s) are credited and that the original publication in this journal is cited, in accordance with accepted academic practice. No use, distribution or reproduction is permitted which does not comply with these terms.
*Correspondence: Pu Li, bGlwdThAbWFpbC5zeXN1LmVkdS5jbg==; Nora F. Y. Tam, YmhudGFtQGNpdHl1LmVkdS5oaw==