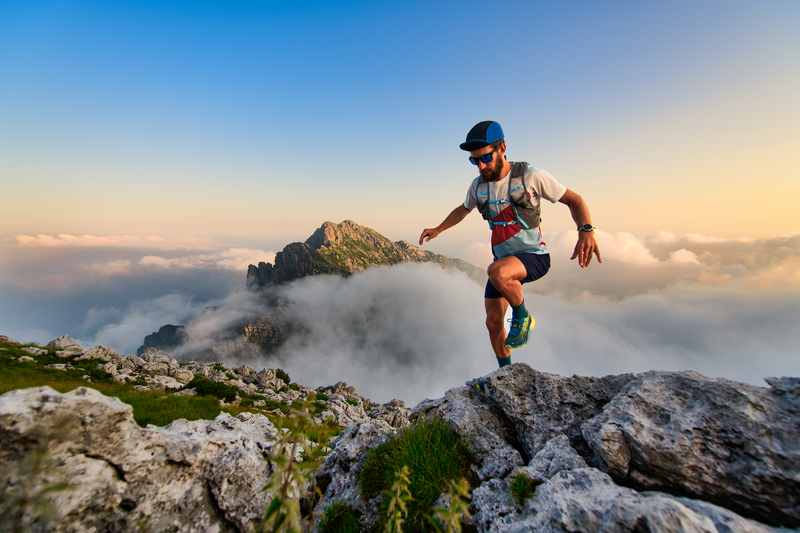
95% of researchers rate our articles as excellent or good
Learn more about the work of our research integrity team to safeguard the quality of each article we publish.
Find out more
ORIGINAL RESEARCH article
Front. Mar. Sci. , 16 November 2022
Sec. Marine Pollution
Volume 9 - 2022 | https://doi.org/10.3389/fmars.2022.1041003
This article is part of the Research Topic New Insights to Coastal Zone Environmental Pollution, Ecosystem Disturbance and Remediation View all 5 articles
Plastic particles have the ability to transfer harmful chemical pollutants due to their high adsorption capacity. Therefore, this study aimed to investigate the effects of combined exposure to polyethylene microplastics (PE-MPs) and 4-nonylphenol (4-NP) on juvenile common carp (Cyprinus carpio) using histopathological and histochemical biomarkers. Fish were separated into a control group and three treatment groups (10 mg/L PE-MPs; 10 mg/L PE-MPs + 200 µg/L 4-NP; 200 µg/L 4-NP) for a two-week continuous exposure experiment followed by two weeks of recovery. The three treatment groups showed histopathological changes compared to the control. These alterations included severe edema, lifting of the outer epithelium, interlamellar fusion and vacuolation, secondary lamellar shortening and complete fusion, increased mucous cell numbers in the gill tissue, enlargement of inner layer stratum periventricular, cell degeneration with pyknotic nuclei, increased blood capillaries, spongiosis in the brain tissue (optic tectum), central vein hemorrhage, shrunken and fatty degeneration of hepatocytes, rosette shapes around small congested blood sinusoids, vacuoles, necrosis, and severe glycogen reduction in the liver tissue. Some tissue changes improved during the two-week recovery period but did not return to normal. In conclusion, the mixture exposure of the PE-MPs and 4-NP on fish carp induced some histological alterations in most studied tissues and post-exposure made improvement in cellular and tissue structure.
Plastic pollution is a serious global problem, especially in aquatic environments contaminated by industrial chemicals that can cause serious damage during the developmental and adult stages of fish, amphibians, and other organisms (Radhaiah et al., 1987). Plastic wastes decompose into small fragments when exposed to ultraviolet light, hydrolysis, mechanical wearing, and biodegradation (Andrady, 2011). The capacity for heavy metal adsorption on microplastic (MP) surfaces increases as particle size decreases, confirming the importance of particle size in adsorption (Wang et al., 2019a; Wang et al., 2019b). Due to their small size and low density, MPs can be transported over long distances (Cózar et al., 2017; Barboza et al., 2018a) and have become globally distributed (Suaria et al., 2016; Auta et al., 2017). MPs are defined as plastic particle fragments ranging in size from 5 to 100 nm (Gigault et al., 2018) and can be classified according to their monomer backbone structure as polypropylene, polystyrene, polyamide, polyvinyl chloride, or polyethylene (PE) (Bouwmeester et al., 2015; Li et al., 2016). PE is the most common type of plastic, with the chemical composition (C2H4)n (da Costa et al., 2016; de Sá et al., 2018). Products made from PE include plastic bags, storage containers, packaging, and toys (Lusher et al., 2020). The toxic effects of exposure to PE-MPs can produce more severe alterations in some tissues than exposure to larger PE particles (Hamed et al., 2022).
As a result of their high adsorption capacity, plastic particles can adsorb or accumulate other environmental contaminants on their surface, exceeding the concentrations found in the surrounding water (Mato et al., 2001). Aquatic organisms may respond differently to combined exposures to MPs and other contaminants than to individual contaminants (Yi et al., 2019). The presence of MPs has been suggested to aggravate the toxicity of coexisting contaminants in aquatic organisms in this regard, according to some previous studies (Jinhui et al., 2019). The toxic effects of MPs on aquatic communities can be ascribed to the ingestion of MPs and their additives by fish, mussels, turtles, and zooplankton (Fossi et al., 2016; Botterell et al., 2019; Li et al., 2019). Ingestion of MPs can physically and chemically affect aquatic organisms, with additives and adsorbed organic chemicals having a significant impact on physiology (Barboza et al., 2018a; Barboza et al., 2018b). Plastic debris can cause negative effects via structural damage caused by the particles, chemicals added to plastic products during their manufacture and their use (such as abrasives), and environmental contaminants absorbed by the plastic (Teuten et al., 2009; Frias et al., 2010; Hahladakis et al., 2018).
Chemical contaminants and MPs have been shown to synergistically interact, causing significant changes to the liver, brain, muscle, and intestinal homeostasis (Rainieri et al., 2018). MPs are accumulated in the guts of aquatic organisms and transported to other organs via the circulatory system (Jin et al., 2018). Plastic additives like nonylphenol and brominates are known carcinogens and environmental endocrine disruptors. In freshwater and marine organisms, 4-nonylphenol (4-NP) bioaccumulates from wastewater discharges and other sources (Ekelund et al., 1990; Uguz et al., 2003; Ishibashi et al., 2006; Spehar et al., 2010). At elevated concentrations, 4-NP can cause cell lysis, kidney exhaustion, tissue damage, and reduced hemopoietic activity in aquatic life (Senthil Kumaran et al., 2011). Exposure to plastics and 4-NP separately can cause various alterations, starting from changes in single cells and ending with changes in the whole tissue and organism (Sayed and Soliman, 2018; Ahrendt et al., 2020; Hamed et al., 2022). In teleost fishes, the liver is essential for the metabolism of lipids and carbohydrates and is highly sensitive to changes in the environment. Heavy metals and other contaminants accumulate in the liver, causing tissue damage; thus, liver histopathology can be used as biomarker for exposure to contaminants (Hinton and Laurén, 1990). Additionally, fish regulate osmotic pressure and excrete waste products through their gills, which function as gas exchange organs. This delicate vital organ is in direct contact with aquatic environments and often suffers damage due to environmental contaminants (Parashar and Banerjee, 2002). As a result of water filtration, MPs are retained in the gills. Toxins are blocked from entering the body through the gills, which decreases their uptake by other organs but magnifies their effect (Shah et al., 2020). MP uptake through the gills is dependent on their size and morphology, as well as the efficiency of the filtering apparatus (Collard et al., 2017). Contaminant exposure can also be detected by biomarkers in the brain that are regarded as good indicators of pollution (Ferrando and Andreu-Moliner, 1991).
We selected the common carp, Cyprinus carpio, to assess the toxicity of PE-MPs because it is one of the most widely cultured freshwater fish in the world (Xu et al., 2012) and a common ecotoxicological test organism (Hamed et al., 2022). There is a lack of understanding of the combined impact of PE-MPs on aquatic organisms, which warrants investigation. Therefore, in the current study, we investigated the combined toxic effects of PE-MPs and 4-NP on juvenile C. carpio using histopathological and histochemical biomarkers.
We obtained 4-NP with a purity of 99.3% from Sigma-Aldrich (Schnelldrof, Germany) and PE-MPs from Toxemerge Pty Ltd., Australia.
One gram of PE-MPs was dissolved in 1 L of distilled water, and1g of 4-NP was dissolved in 1 L of distilled water to prepare the stock solutions. Both stock solutions were maintained at 4°C in the dark and shaken before every use.
Juvenile common carp (C. carpio) (weight: 5 ± 1 g; length: 8.5 ± 1 cm) were used for this experiment where fish stocks were regularly maintained in the laboratory. Fish acclimation and exposure experiments were conducted at the Fish Biology and Pollution Laboratory of the Zoology Department, Faculty of Science, Assiut University under physicochemical conditions: 28.5°C, pH 7.4, 6.9 mg/L DO, 12:12 h (light:dark), and 260.8 mM/cm conductivity.
Fish were divided into four groups, with each treatment group containing 36 fish; treatments were performed in triplicate. The control group and three treatment groups (10 mg/L PE-MPs; 10 mg/L PE-MPs + 200 µg/L 4-NP; 200 µg/L 4-NP) underwent a two-week continuous exposure followed by two weeks of post exposure. The doses were selected according to (Sayed and Soliman, 2018; Hamed et al., 2019). Fish were fed a fish food pellet diet (3% of their body weight per day), and the water was changed (50%) every day with redosing. The physicochemical properties of the water were monitored in the tanks (100 cm × 70 cm × 50 cm), where acclimatization and exposure experiments were done. The water exhibited in average 28.5°C, pH 7.4, 6.9 mg/L of dissolved oxygen, and conductivity of 260.8 mM/cm. After the two-week exposure and after the two-week post exposure period, six fish from each treatment were randomly collected and ice-benumbed to lessen the handling stress (Wilson et al., 2009).
The gills, brain, and liver tissues of juvenile carp were collected and fixed in 10% neutral buffered formalin. Samples were routinely processed using paraffin techniques and cut into 5-μm thick sections. The sections were stained using Harris’s hematoxylin and eosin (H & E) stain for general histology, periodic acid Schiff’s reagent (PAS) for polysaccharides, and crystal violet for Nissl bodies. Stained sections were examined using a VE-T2 microscope, and selected regions were photographed using a 14MP OMAX Camera (MN: A35140U3, China).
Gill sections from the control group showed primary gill filaments containing blood capillaries and secondary gill filaments with epithelial cells in their cores, which appeared in a ladder shape. Supportive pillar cells alternate with blood capillaries that rest on the basement membrane and are externally surrounded by pavement cells also resting on the basement membrane (Figure 1A). All treated groups showed severe deformation in gill morphology, including shortening of the secondary lamellae (SL), atrophy, decreases in pillar cells and blood capillaries, fusion, and vacuolation of the interlamellar epithelium(Figure 1B). The primary gill contains thick cartilage and shortening of the SL, epithelial cell hyperplasia and complete fusion reaching the whole length of the SL, and displacement of epithelial cells toward the periphery, leaving wide spaces and vacuolated blood capillaries (Figure 1C). The primary gill contains thick and wavy cartilage surrounded by dense connective fibers. The secondary gill lamellae are curly in appearance on one side and directed upward on the other side. Displacement of the interlamellar epithelium from the basement membrane, leaving a large irregular space, hyperplasia of cells inside the core of SL with clear hyperemia and edema, and lifting of the epithelium were observed (Figure 1D). Narrowing and deformation of the primary gill core and connective tissue degeneration, severe edema, thinning core of the SL, hyperemia, and lifting of the outer epithelium, deformation, atrophy, and displacement of interlamellar epithelial cells from the basement membrane leaving a large irregular space, were observed (Figure 1E). Wing shape and displacement of the SL core at one side, lifting of the outer epithelial cells leaving space, and hyperemia were observed (Figure 1F). The SL core disappeared, and the interlamellar epithelium becomes connected, except the central line of SL, marked thinning and elongation of the SL were observed, and brown pigments were noticed in the core of primary lamellae (PL) (Figure 1G).
Figure 1 H & E-stained gill sections of common carp showing the normal morphological structure of the control group (A) and selected deformations after treatment with10 mg/L PE-MPs (B, G), 10 mg/L PE-MPs + 200 µg/L 4-NP (C, F), and 200 µg/L 4-NP (D, E), as (B, C, E) for exposure period and (D, F, G) for post exposure period. Primary lamellae (PL), secondary lamellae (SL), epithelial cells (EC), blood capillaries (BC), pavement cells (PC), supportive pillar cells (SPC), vacuoles(V), space (S), connective fibers (CF), basement membrane (BM), lifting epithelium (LE), and edema (E).
After applying PAS reagent for the detection of polysaccharide sat the end of the two-week continuous exposure, the control groups showed marked intensity in the BM of the PL, the external boundary of the SL, and a few mucous-secreting cells at the surface of the interlamellar epithelium between or at tips of the SL (Figure 2A). The treatment groups showed marked increases in polysaccharides contents compared to the control group. In the treatment groups, polysaccharides were concentrated at the basal membrane of the PL and the periphery of the SL. Deeply stained mucous cells migrating upward toward the external part of the SL were also observed after exposure to PE-MPs (Figure 2B). Exposure to PE-MPs + 4 NP resulted in increased polysaccharide contents concentrated in the connective tissues of the PL. A faint reaction was observed in the gill tissues, and the main concentration occurred at the apical surface of the SL, with an increase in mucous cells migrating upward at separate or fused SL (Figure 2C). In the 4-NP-treated group, a faint positive reaction in gill polysaccharides contents was located at the rim of the covering epithelial, with an increase in mucous cells (Figure 2D).
Figure 2 Gill sections (PAS reaction showing polysaccharides) of common carp showing the control group, and after treatment with 10 mg/L PE-MPs, 10 mg/L PE-MPs + 200 µg/L 4-NP, and 200 µg/L 4-NP, as (A–D) for exposure and (E–H) for post exposure, respectively. Primary lamellae (PL), secondary lamellae (SL), connective tissue (CT), basement membrane (BM), and mucous cell (MC).
After the two-week post exposure period, the control group showed a normal distribution of polysaccharides in the BM of the PL and the surface contour of the SL, as well as mucous-secreting cells located at the surface of interlamellar epithelium between the SL (Figure 2E).The intensity of positive color of polysaccharide content was observed in tissues with an increment of mucous-secreting cells in different locations at the boundary of covering epithelium for the PE-MP-treated group (Figure 2F). A marked increase in polysaccharides was observed throughout the gill, especially at the contour of the SL. Deeply stained enlarged mucous cells were arranged at the contour of the covering epithelium between the SL at one side in the group exposed to PE-MPs + 4-NP (Figure 2G). An increase in mucous cells containing a deeply stained red mucous substance were arranged at the contour of the covering epithelium between the SL in the group exposed to 4-NP (Figure 2H).
The brain of common carp in the control group showed the optic tectum region containing six major layers: the first layer SPV in contact with ependyma containing large groups of densely populated and small-sized neurons with deeply stained nuclei connected by fibers and glial cells; the second layer stratum album centrale (SAC); the third layer stratum griseum centrale (SGC); the fourth layer stratum fibrosum et grisium superficiale (SFGS)containing several tiny irregular spaces in an acidophilic neuropile, spongiosis, glial cells, blood capillaries located beside the unstained area and few large neurons with vesicular nuclei were dispersed in these layers; the fifth layer stratum opticum (SO) containing neuropile with unstained space rich with blood capillaries; the outer sixth layer, the stratum marginale (SM), is divided into three layers, the internal layer(1) contains acidophilic neuropile with large regions of spongiosis with blood capillaries and glial cells, followed by the external layer (3), which consists of blood capillaries and connective tissues covered by epithelial cells. The internal and external layers are separated by unstained region (2) (Figures 3A–D). Treated groups showed enlargement of the SPV, including large groups of densely populated differentiated neurons with vesicular nuclei at the basal regions separated by unstained space containing fibers. Separation of the apical regions, which contain degenerated cells with pyknotic nuclei, hydropic degeneration, and hemorrhage with congested blood capillaries, was observed. The SAC, SGC, and SFGC layers contain several tiny irregular spaces in an acidophilic neuropile edema/spongiosis, small blood capillaries, many glial cells, and a few large, dispersed neurons with vesicular nuclei. The SO layer contains meshwork acidophilic neuropile with deeply stained degenerated neurons aggregated at the boundary and more vascularization than the control group. The SM layer (1) contains homogenous acid ophilic neuropile with a tiny unstained space of edema surrounded by blood capillaries and a small area of unstained neuropile (2), followed by (3) which contains degenerated connective tissues and squamous epithelial, edema, and high vascularization compared with the control group (Figures 3E–M).
Figure 3 H & E-stained sections of the optic tectum region of the brain of juvenile common carp showing general morphological structure of the control group (A–D). Selective deformations in the optic tectum after treatment with 10 mg/L PE-MPs (E–G, L), 10 mg/L PE-MPs +200 µg/L 4-NP (H, K), and 200 µg/L 4-NP (I, J, M), as (E–J) for exposure period, and (K–M) for post exposure period. Stratum periventricular (SPV), stratum album centrale (SAC), stratum griseum centrale (SGC), stratum fibrosum et grisium superficiale (SFGS), stratum opticum (SO), stratum marginale (SM), neurons (N), fibers (F), glial cells (g), neuropil (NP), large neurons (LN), blood capillaries (BC), hemorrhage (h), pyknotic nuclei (PN), hydropic degeneration (HD), spongiosis (S), edema (E), connective tissue (CT), epithelial cells (EC).
In the control group optic tectum, a high intensity of violet color was observed in the small neurons of the SPV filled with Nissl bodies. The second to fifth layers contain small basophilic Nissl bodies scattered within the large neurons; a purple stain was present in the epithelial cells and connective tissues of the last layer alongside glial cells (Figures 4A, a). The violet color in the neuronal cells of the internal layer appears less compacted and filled with ribonucleic acids. The second to fifth layers contain dispersed shapes of large neurons with deeply stained basophilic Nissl granules and perinuclei. The last layer shows deeper staining after exposure to PE-MPs than the control group (Figures 4B, b). Small neurons in the internal layer have a slight reduction in color intensity compared to control. Degenerated large neurons are dispersed among a layer of deeply stained basophilic ribonucleic acids in the second to fourth layers. The fifth layer contains deeply stained aggregated neurons, and the last layer shows less staining after exposure to PE-MPs compared with the control group (Figures 4C, c). Internal layer small neurons show a slight increase in color intensity, the second to fourth layers contain large degenerating neurons with perinuclei that are stained with basophilic ribonucleic acids, the fifth layer contains deeply stained aggregated neurons, and the last layer shows less staining after exposure to 4-NP compared with the control group (Figures 4D, d).
Figure 4 Crystal violet-stained sections of the optic tectum of common carp showing the control group (A, a), after a two-week exposure to 10 mg/L PE-MPs (B, b), 10 mg/L PE-MPs +200 µg/L 4-NP (C, c), and 200 µg/L4-NP (D, d). Glial cells (g), large neurons (LN), connective tissue (CT), epithelial cells (EC).
After the post exposure period, the control group shows lower intensity of violet color in all optic tectum layers compared with the control fish (Figures 5A, a). Within the internal layer, there is a strong violet color. The second to fourth layers contain large numbers of dispersed and different shapes of neurons with deeply stained basophilic Nissl granules with perinuclei. The fifth layer is negatively stained, and the last layer shows deeper staining concentrated in connective tissues and the outer epithelial contour in the group exposed to PE-MPs (Figures 5B, b). Within the internal layer, there is a strong violet color in the small neurons, and the second to fourth layers show numerous large neuronal structures containing basophilic ribonucleic acids and peri nuclei dispersed widely in the tissue. The fifth layer contains a few deeply stained neurons, and the last layer shows deeply stained connective tissues and outer epithelial contour in the groups exposed to PE-MPs + 4-NP and 4-NP (Figures 5C, c; D, d), respectively.
Figure 5 Crystal violet-stained sections of the optic tectum of common carp after the two-week post exposure period showing control group (A, a), 10 mg/L PE-MP group (B, b), 10 mg/L PE-MPs + 200 µg/L 4-NPgroup (C, c), and 200 µg/L 4-NP group (D, d). Large neurons (LN), connective tissue (CT), epithelial cells (EC).
Liver sections of control carp show normal histological structure with aggregated polygonal hepatocytes separated by blood sinusoids; each hepatocyte contains acidophilic cytoplasm situated around the central nuclei (Figure 6A). Treated groups show selected deformations of the hepatocyte shape after exposure to PE-MPs, PE-MPs +4-NP, and 4-NP, including hepatocytes collected as a rosette shape around small congested blood sinusoids, hepatocytes with faint heterogeneous acidophilic cytoplasm concentrated around nuclei with degenerated or unclear borders, necrotic areas of degenerated hepatocytes, small deeply stained nuclei with eccentric positions in the cells and many pyknotic nuclei, dilated and congested blood sinusoids, hemorrhage in the central veins, and shrunken and fatty degeneration of the hepatocytes characterized by large unstained vacuoles with sharp outlines (Figures 6B–G).
Figure 6 H&E-stained sections of liver of common carp showing the normal morphology of hepatocytes of the control group (A) and selective deformations in shape after treatment with 10 mg/L PE-MPs, 10 mg/L PE-MPs +200 µg/L 4-NP, and 200 µg/L 4-NP, as (B–D) for exposure period and (E–G) for post exposure period, respectively. Blood sinusoids (BS), pyknotic nuclei (P), fatty degeneration (fd), hemorrhage (h), necrotic area (N), rosette shape (R), vacuoles (v).
Liver sections of the control group treated with PAS show polysaccharides are abundant in hepatic cells, particularly around both perinuclear regions, and periphery cells appear with clear contours, fine glycogen granules in the cytoplasm, and most positive staining occurring in the blood sinusoids (Figure 7A). The PE-MP-treated group shows diversity in the distribution of positive staining materials, regions contain glycogen granules normally distributed in hepatocytes and other regions contain high intensity of staining, indicating increased deposition of heavy glycogen (Figure 7B). Fewer positively stained materials, faint reaction at the hepatocyte contour, and internal rim beside blood sinusoids with severe depletion of polysaccharides was observed after exposure to PE-MPs + 4-NP (Figure 7C). This group also shows an increase in the amount of polysaccharides (glycogen) in hepatocytes compared with the previous two treatments. Whole hepatocytes are engorged with glycogen deposition, and a few cells contain aggregates of these positive materials with perinuclei regions after exposure to 4-NP (Figure 7D). After the post exposure period, polysaccharides are abundant in hepatocytes, particularly around both basophilic materials with perinuclei, and at the contour of cells with clear margins, marked fine glycogen granules in the cytoplasm and positive materials are observed at the circumference of blood sinusoids in the control group (Figure 7E). High intensity of positive color signals the deposition of polysaccharides due to the fatty deposition. The deposition of material is located and concentrated around these vacuoles, and the main cells contain glycogen at one end of the hepatocytes for the group exposed to PE-MPs (Figure 7F). Marked increase in stained materials abundant located at the cell boundary and perinuclei and depletion of these materials beside the blood sinusoids was observed in the group exposed to PE-MPs with 4-NP (Figure 7G). A sharp increase of positive material occurred at one side of the hepatocytes or located in the perinuclei, and other cells contain homogenous distribution of glycogen granules around vacuoles for the group exposed to 4-NP (Figure 7H).
Figure 7 Sections of the liver (PAS reaction showing polysaccharides) of common carp showing the control group, and after treatment with 10 mg/L PE-MP, 10 mg/L PE-MPs + 200 µg/L 4-NPgroup, and 200 µg/L 4-NPgroup, as (A–D) for 2 weeks of exposure and (E–H) for 2 weeks of post exposure, respectively. Blood sinusoids (BS).
Several contaminants have been shown to alter some tissues in different types of fish (Sayed and Soliman, 2018; Sayed et al., 2021; Hamed et al., 2022). In this study, the liver, gills, and brain of juvenile carp showed some alterations after continuous exposure to 10 mg/L PE-MPs, 10 mg/L PE-MPs + 200 µg/L 4-NP, and 200 µg/L 4-NP for two weeks, followed by two weeks of recovery.
Liver tissue in the exposed fish showed deformation of hepatocytes, including hepatocytes collected in a rosette shape around the blood sinusoids, necrotic areas of degenerated hepatocytes, many pyknotic nuclei, dilated and congested blood sinusoids, hemorrhage in the central veins, shrunken and fatty degeneration of hepatocytes, and severe depletion of polysaccharide contents. Similar alterations were reported in common carp exposed to lead acetate (Mustafa et al., 2017) and PE-MPs (Hamed et al., 2022), in catfish exposed to MPs (Sayed et al., 2022) and 4-NP (Sayed and Soliman, 2018), in zebrafish as combined effects of MPs and chemical contaminants (Rainieri et al., 2018), as well as other types of fish exposed to different toxins (Figueiredo-Fernandes et al., 2007; da Costa et al., 2016; Espinosa et al., 2019; Soliman et al., 2019; da Costa Araújo et al., 2020; Naguib et al., 2020; Sayed et al., 2021). In addition to glycogen and lipid deposition, the observed hepatic vacuolations may reflect abnormal liver metabolic processes (Myers et al., 1987). Increased vacuolation of the hepatocytes suggests metabolic damage resulting from degenerative processes caused by contaminated water (Pacheco and Santos, 2002). Hepatocytes vacuolate to protect themselves against potentially harmful materials (Mollendroff, 1973). Microplastics and 4-NP may accumulate in the liver tissue causing hepatic necrosis and degenerative changes. Histopathology studies indicate that hepatocyte damage is related to energy reductions and metabolic disturbances, microtubule disaggregation, protein synthesis inhibition, and an increase in hepatic red blood cell production (Laurén et al., 1990; da Costa Araújo et al., 2020; Hamed et al., 2021). In the liver, PE plastics may block hepatic capillaries, causing sinusoidal dilatation (Brancatelli et al., 2018; Hamed et al., 2022). Some morphological changes might be associated with these hepatic lesions due to the major role of the liver in metabolism and the excretion of toxic agents (Rocha and Monteiro, 1999).
The gills are directly exposed to pollution because they are frequently in contact with water. In this study, the gill tissue showed deformation, including shortening of the SL, fusion and vacuolation of the interlamellar epithelium, displacement of epithelial cells, vacuolation of the blood capillaries, severe edema, hyperemia, and lifting of the epithelium. These alterations were similarly reported in carp exposed to lead acetate (Mustafa et al., 2017), catfish exposed to 4-NP (Sayed et al., 2012), Oreochromis niloticus exposed to MPs (Hamed et al., 2021), and other types of fish exposed to different contaminants (Cengiz and Unlu, 2006; Figueiredo-Fernandes et al., 2007; Farrell et al., 2010; Hadi and Alwan, 2012; Sayed et al., 2020). The gills of teleost fishes can be damaged by exposure to high concentrations of different contaminants (Dutta et al., 1996; Wendelaar Bonga, 1997). MP accumulation in the gills may damage the filaments, increase the probability of infections, and complicate the entry of MPs into the body (Movahedinia et al., 2012; Jabeen et al., 2018). Contaminants may cause changes in the gill morphology via mechanical damage or leached chemical additives (Hu et al., 2020). Osmoregulation disorders can be caused by epithelium separation from the basal membrane, which may act as a protective mechanism (Movahedinia et al., 2012). If the epithelium lifts or hyperplasia reactions occur, the fish could collapse and ultimately die from asphyxiation. Such phenotypic changes could result from toxicant consumption or from increased capillary permeability, which prevents pollutants from entering the gills (Mallatt, 1985). Ion balance is negatively affected by discontinuities in the epithelium of the gills (Peuranen et al., 1994). Increased diffusion distance for gas exchange could be caused by fluid infiltration between the epithelium and basement membranes. There is some evidence to suggest that vacuolation may be a protection mechanism against injury that affects fish health (Shahid et al., 2020).
Abdelnaeim Hussein and Cao (2018) state that the histological and anatomical structure of the brain may differ from one type of fish to another, but the number of parts is consistent. In this study, brain tissue showed deformation, including highly vascularized regions, edema/spongiosis, hemorrhage, enlargement of the SPV, and hydropic degeneration. The alterations observed are similar to those reported in Channa punctatus exposed to the pesticide chlorpyrifos (Mishra and Devi, 2014), Gambusia affinis exposed to lead chloride (Alkshab and Taha, 2021), carp exposed toquinalphos (Chamarthi et al., 2014), carp exposed to organophosphate insecticide (Lakshmaiah, 2017), African catfish exposed to glyphosate herbicide (Erhunmwunse et al., 2014), Catlacatla exposed to heavy metals (Bose et al., 2013), Carassius gibelio exposed to toxic cyanobacteria (Berillis et al., 2014), and other fish exposed to different contaminants (Das and Mukherjee, 2000; Ayoola, 2008; Ayoola and Ajani, 2008; Pugazhvendan et al., 2009; Lakshmaiah, 2017). The histological changes observed in this study in the brain of carp fish may be a defense mechanism. Accumulation of MPs and/or 4-NP in the brain of carp fish may destroy the brain structures. Edematous alterations were observed in the brain that are likely caused by abnormal blood vessel function and damage to the blood–brain barrier, which can cause edemas to appear (Scorticati et al., 2004).
This study indicated the exposure of the combined PE-MPs and 4-NP on some organs (liver, gills, and brain) of juvenile common carp (C. carpio) showed histopathological alterations. The degree of the damage were as 4-NP + PE-MPs > 4-NP> PE-MPs and the post-exposure improved some of the histopathological changes. Accordingly, these compounds need to be managed and controlled to protect aquatic environments and according to the location of fish species in the food web, developing pollutant recovery strategies is essential.
The original contributions presented in the study are included in the article/supplementary material. Further inquiries can be directed to the corresponding author.
Experiments were approved by the Research Ethical Committee of the Molecular Biology Research and Studies Institute (MB-21-11-R), Assiut University, Assiut, Egypt.
EA, MM, and AHS: conceptualization; EA and AHS: methodology; EA and AHS: visualization and investigation; EA and AHS: data curation and writing- original draft preparation. All authors: final draft writing- reviewing and editing. All authors contributed to the article and approved the submitted version.
The authors are grateful to Prof. Hanem Saad of the Assiut University for her fruitful discussion and useful comments, which stimulate to advance this study.
The authors declare that the research was conducted in the absence of any commercial or financial relationships that could be construed as a potential conflict of interest.
All claims expressed in this article are solely those of the authors and do not necessarily represent those of their affiliated organizations, or those of the publisher, the editors and the reviewers. Any product that may be evaluated in this article, or claim that may be made by its manufacturer, is not guaranteed or endorsed by the publisher.
Abdelnaeim Hussein M. N., Cao X. (2018). Brain anatomy and histology in teleosts. Benha. Veterinary. Med. J. 35, 446–463. doi: 10.21608/bvmj.2018.96440
Ahrendt C., Perez-Venegas D. J., Urbina M., Gonzalez C., Echeveste P., Aldana M., et al. (2020). Microplastic ingestion cause intestinal lesions in the intertidal fish girella laevifrons. Mar. Pollut. Bull. 151, 110795. doi: 10.1016/j.marpolbul.2019.110795
Alkshab A., Taha A. (2021). Histological effect of lead chloride on the brain of gambusia affinis. J. Physics.: Conf. Ser. 1818, 012024. doi: 10.1088/1742-6596/1818/1/012024
Andrady A. L. (2011). Microplastics in the marine environment. Mar. pollut. Bull. 62, 1596–1605. doi: 10.1016/j.marpolbul.2011.05.030
Auta H. S., Emenike C. U., Fauziah S. H. (2017). Distribution and importance of microplastics in the marine environment: A review of the sources, fate, effects, and potential solutions. Environ. Int. 102, 165–176. doi: 10.1016/j.envint.2017.02.013
Ayoola S. O. (2008). Toxicity of glyphosate herbicide on Nile tilapia (Oreochromis niloticus) juvenile. Afr. J. Agric. Res. 3, 825–834.
Ayoola S. O., Ajani E. K. (2008). Histopathological effect of cypermethrin on juvenile African catfish (Clarias gariepinus). World J. Biol. Res. 001, 1–14.
Barboza L. G. A., Vieira L. R., Branco V., Carvalho C., Guilhermino L. (2018a). Microplastics increase mercury bioconcentration in gills and bioaccumulation in the liver, and cause oxidative stress and damage in dicentrarchus labrax juveniles. Sci. Rep. 8, 15655. doi: 10.1038/s41598-018-34125-z
Barboza L. G. A., Vieira L. R., Guilhermino L. (2018b). Single and combined effects of microplastics and mercury on juveniles of the European seabass (Dicentrarchus labrax): Changes in behavioural responses and reduction of swimming velocity and resistance time. Environ. pollut. 236, 1014–1019. doi: 10.1016/j.envpol.2017.12.082
Berillis P., Kormas K., Kagalou I., Petridou E., Papadimitriou T. (2014). Brain and liver histopathological examination of carassius gibelio from a newly reconstructed lake with toxic cyanobacteria. Turkish. J. Fisher. Aquat. Sci. 213-212, 219. doi: 10.4194/1303-2712-v14_1_23
Bose M. T., Ilavazhahan M., Tamilselvi R., Viswanathan M. (2013). Effect of heavy metals on the histopathology of gills and brain of fresh water fish catla catla. Biomed. Pharmacol. J. 6, 99–105. Available at: http://biomedpharmajournal.org/?p=2632
Botterell Z. L. R., Beaumont N., Dorrington T., Steinke M., Thompson R. C., Lindeque P. K. (2019). Bioavailability and effects of microplastics on marine zooplankton: A review. Environ. Pollut. 245, 98–110. doi: 10.1016/j.envpol.2018.10.065
Bouwmeester H., Hollman P. C. H., Peters R. J. B. (2015). Potential health impact of environmentally released micro- and nanoplastics in the human food production chain: Experiences from nanotoxicology. Environ. Sci. Technol. 49, 8932–8947. doi: 10.1021/acs.est.5b01090
Brancatelli G., Furlan A., Calandra A., Dioguardi Burgio M. (2018). Hepatic sinusoidal dilatation. Abdominal. Radiol. 43, 295–322. doi: 10.1007/s00261-018-1465-8
Cengiz E. I., Unlu E. (2006). Sublethal effects of commercial deltamethrin on the structure of the gill, liver and gut tissues of mosquitofish, gambusia affinis: A microscopic study. Environ. Toxicol. Pharmacol. 21, 246–253. doi: 10.1016/j.etap.2005.08.005
Chamarthi R., Bangeppagari M., Mohiddin J., Mandala S., Ortiz Tirado J., Marigoudar S. R. (2014). Histopathological alterations in the gill, liver and brain of cyprinus carpio on exposure to quinalphos. Am. J. Life Sci. 2, 211–216. doi: 10.11648/j.ajls.20140204.14
Collard F., Gilbert B., Eppe G., Roos L., Compère P., Das K., et al. (2017). Morphology of the filtration apparatus of three planktivorous fishes and relation with ingested anthropogenic particles. Mar. pollut. Bull. 116, 182–191. doi: 10.1016/j.marpolbul.2016.12.067
Cózar A., Marti E., Duarte C., Lomas J., Sebille E., Ballatore T., et al. (2017). The Arctic ocean as a dead end for floating plastics in the north Atlantic branch of the thermohaline circulation. Sci. Adv. 3, e1600582. doi: 10.1126/sciadv.1600582
da Costa Araújo A. P., de Melo N. F. S., de Oliveira Junior A. G., Rodrigues F. P., Fernandes T., de Andrade Vieira J. E., et al. (2020). How much are microplastics harmful to the health of amphibians? a study with pristine polyethylene microplastics and physalaemus cuvieri. J. Hazard. Mater. 382, 121066. doi: 10.1016/j.jhazmat.2019.121066
da Costa J. P., Santos P. S. M., Duarte A. C., Rocha-Santos T. (2016). (Nano)plastics in the environment – sources, fates and effects. Sci. Total. Environ. 566-567, 15–26. doi: 10.1016/j.scitotenv.2016.05.041
Das B. K., Mukherjee S. C. J. V. A. (2000). A histopathological study of carp (Labeo rohita) exposed to hexachlorocyclohexane, Vol. 70. 169–180.
de Sá L. C., Oliveira M., Ribeiro F., Rocha T. L., Futter M. N. (2018). Studies of the effects of microplastics on aquatic organisms: What do we know and where should we focus our efforts in the future? Sci. Total. Environ. 645, 1029–1039. doi: 10.1016/j.scitotenv.2018.07.207
Dutta H. M., Munshi J. S. D., Roy P. K., Singh N. K., Adhikari S., Killius J. (1996). Ultrastructural changes in the respiratory lamellae of the catfish, heteropneustes fossilis after sublethal exposure to malathion. Environ. Pollut. 92, 329–341. doi: 10.1016/0269-7491(95)00101-8
Ekelund R., Bergman Å., Granmo Å., Berggren M. (1990). Bioaccumulation of 4-nonylphenol in marine animals— a re-evaluation. Environ. Pollut. 64, 107–120. doi: 10.1016/0269-7491(90)90108-O
Erhunmwunse N., Sa E., Ainerua M., Ewere E., Ee (2014). Histopathological changes in the brain tissue of Africa catfish exposure to glyphosate herbicide. J. Applied Sci. Environ. Management 18(2), 275–280. doi: 10.4314/jasem.v18i2.19
Espinosa C., Esteban M., Cuesta A. (2019). Dietary administration of PVC and PE microplastics produces histological damage, oxidative stress and immunoregulation in European sea bass (Dicentrarchus labrax l.). Fish. Shellfish. Immunol. 95, 574–583. doi: 10.1016/j.fsi.2019.10.072
Farrell A. P., Ackerman P. A., Iwama G. K. (2010). “Disorders of the cardiovascular and respiratory systems,” in Fish diseases and disorders, non-infectious disorders. Eds. Leatherland J. F., Woo P. T. K. (Wallingford, CN: CABI), 287–322.
Ferrando M. D., Andreu-Moliner E. (1991). Acute lethal toxicity of some pesticides to brachionus calyciflorus and brachionus plicatilis. Bull. Environ. Contam. Toxicol. 47, 479–484. doi: 10.1007/BF01702214
Figueiredo-Fernandes A., Ferreira-Cardoso J., Garcia Santos S., Monteiro S., Carrola J., Matos P., et al. (2007). Histopathological changes in liver and gill epithelium of Nile tilapia, oreochromis niloticus, exposed to waterborne copper. Pesquisa. Veterinaria. Bras. -. CBPA. 27. doi: 10.1590/S0100-736X2007000300004
Fossi M. C., Marsili L., Baini M., Giannetti M., Coppola D., Guerranti C., et al. (2016). Fin whales and microplastics: The Mediterranean Sea and the Sea of cortez scenarios. Environ. pollut. 209, 68–78. doi: 10.1016/j.envpol.2015.11.022
Frias J. P. G. L., Sobral P., Ferreira A. M. (2010). Organic pollutants in microplastics from two beaches of the Portuguese coast. Mar. pollut. Bull. 60, 1988–1992. doi: 10.1016/j.marpolbul.2010.07.030
Gigault J., Halle A. T., Baudrimont M., Pascal P.-Y., Gauffre F., Phi T.-L., et al. (2018). Current opinion: What is a nanoplastic? Environ. pollut. 235, 1030–1034. doi: 10.1016/j.envpol.2018.01.024
Hadi A., Alwan S. (2012). Histopathological changes in gills, liver and kidney of fresh water fish, tilapia zillii, exposed to aluminum. Int. J. Pharm. Life Sci. 3.
Hahladakis J. N., Velis C. A., Weber R., Iacovidou E., Purnell P. (2018). An overview of chemical additives present in plastics: Migration, release, fate and environmental impact during their use, disposal and recycling. J. Hazard. materials. 344, 179–199. doi: 10.1016/j.jhazmat.2017.10.014
Hamed M., Monteiro C. E., Sayed A.E.-D.H. (2022). Investigation of the impact caused by different sizes of polyethylene plastics (nano, micro, and macro) in common carp juveniles, cyprinus carpio l., using multi-biomarkers. Sci. Total. Environ. 803, 149921. doi: 10.1016/j.scitotenv.2021.149921
Hamed M., Soliman H. A., Badrey A. E., Osman A. G. (2021). Microplastics induced histopathological lesions in some tissues of tilapia (Oreochromis niloticus) early juveniles. Tissue Cell 71, 101512. doi: 10.1016/j.tice.2021.101512
Hamed M., Soliman H. A. M., Osman A. G. M., Sayed A.E.-D.H. (2019). Assessment the effect of exposure to microplastics in Nile tilapia (Oreochromis niloticus) early juvenile: I. blood biomarkers. Chemosphere 228, 345–350. doi: 10.1016/j.chemosphere.2019.04.153
Hinton D., Laurén D. (1990). Integrative histopathological approaches to detecting effects of environmental stressors on fishes. Am. Fisheries Soc. Symposium 8, 51–66. doi: 10.2141/jpsa.0120013
Hu L., Chernick M., Lewis A. M., Ferguson P. L., Hinton D. E. (2020). Chronic microfiber exposure in adult Japanese medaka (Oryzias latipes). PloS One 15, e0229962. doi: 10.1371/journal.pone.0229962
Ishibashi H., Hirano M., Matsumura N., Watanabe N., Takao Y., Arizono K. (2006). Reproductive effects and bioconcentration of 4-nonylphenol in medaka fish (Oryzias latipes). Chemosphere 65, 1019–1026. doi: 10.1016/j.chemosphere.2006.03.034
Jabeen K., Li B., Chen Q., Su L., Wu C., Hollert H., et al. (2018). Effects of virgin microplastics on goldfish (Carassius auratus). Chemosphere 213, 323–332. doi: 10.1016/j.chemosphere.2018.09.031
Jinhui S., Sudong X., Yan N., Xia P., Jiahao Q., Yongjian X. (2019). Effects of microplastics and attached heavy metals on growth, immunity, and heavy metal accumulation in the yellow seahorse, hippocampus kuda bleeker. Mar. pollut. Bull. 149, 110510. doi: 10.1016/j.marpolbul.2019.110510
Jin Y., Xia J., Pan Z., Yang J., Wang W., Fu Z. (2018). Polystyrene microplastics induce microbiota dysbiosis and inflammation in the gut of adult zebrafish. Environ. Pollut. 235, 322–329. doi: 10.1016/j.envpol.2017.12.088
Lakshmaiah G. (2017). Brain histopathology of the fish cyprinus carpio exposed to lethal concentrations of an organophosphate insecticide phorate. Brain 2, 668–672.
Laurén D. J., Teh S. J., Hinton D. E. (1990). Cytotoxicity phase of diethylnitrosamine-induced hepatic neoplasia in medaka. Cancer Res. 50, 5504–5514.
Li Q., Sun C., Wang Y., Cai H., Li L., Li J., et al. (2019). Fusion of microplastics into the mussel byssus. Environ. pollut. 252 Pt A, 420–426. doi: 10.1016/j.envpol.2019.05.093
Li W. C., Tse H. F., Fok L. (2016). Plastic waste in the marine environment: A review of sources, occurrence and effects. Sci. total. Environ. 566-567, 333–349. doi: 10.1016/j.scitotenv.2016.05.084
Lusher A., Welden N., Sobral P., Cole M. (2020). Sampling, isolating and identifying microplastics ingested by fish and invertebrates, analysis of nanoplastics and microplastics in food (USA, CRC Press), 119–148.
Mallatt J. (1985). Fish gill structural changes induced by toxicants and other irritants: a statistical review. Can. J. Fisher. Aquat. Sci. 42, 630–648. doi: 10.1139/f85-083
Mato Y., Isobe T., Takada H., Kanehiro H., Ohtake C., Kaminuma T. (2001). Plastic resin pellets as a transport medium for toxic chemicals in the marine environment. Environ. Sci. Technol. 35, 318–324. doi: 10.1139/f85-083
Mishra A., Devi Y. (2014). Histopathological alterations in the brain (optic tectum) of the fresh water teleost channa punctatus in response to acute and subchronic exposure to the pesticide chlorpyrifos. Acta histochemica. 116, 176–181. doi: 10.1016/j.acthis.2013.07.001
Movahedinia A., Abtahi B., Bahmani M. J. A. J. O. A., Advances V. (2012). Gill histopathological lesions of the sturgeons. Asian Journal of Animal and Veterinary Advances 7, 710–717. doi: 10.3923/ajava.2012.710.717
Mustafa S., Al-Faragi J., Salman N., Al-Rudainy A. (2017). Histopathological alterations in gills, liver and kidney of common carp, cyprinus carpio exposed to lead acetate. Adv. Anim. Vet. Sci. 5, 371–376. doi: 10.17582/journal.aavs/2017/5.9.371.376
Myers M. S., Rhodes L. D., McCain B. B. (1987). Pathologic anatomy and patterns of occurrence of hepatic neoplasms, putative preneoplastic lesions, and other idiopathic hepatic conditions in English sole (Parophrys vetulus) from puget sound, Washington. J. Natl. Cancer Inst. 78, 333–363. doi: 10.1093/jnci/78.2.333
Naguib M., Mahmoud U. M., Mekkawy I. A., Sayed A.E.-D.H. (2020). Hepatotoxic effects of silver nanoparticles on clarias gariepinus; biochemical, histopathological, and histochemical studies. Toxicol. Rep. 7, 133–141. doi: 10.1016/j.toxrep.2020.01.002
Pacheco M., Santos M. A. (2002). Biotransformation, genotoxic, and histopathological effects of environmental contaminants in European eel (Anguilla anguilla l.). Ecotoxicol. Environ. Saf. 53, 331–347. doi: 10.1016/j.toxrep.2020.01.002
Parashar, Banerjee T. (2002). Toxic impact of lethal concentration of lead nitrate solution on the gills of the air-breathing catfish heteropneustes fossilis (Bloch). Veterinarski. Arhiv. 73, 167–183.
Peuranen S., Vuorinen P. J., Vuorinen M., Hollender A. (1994). The effects of iron, humic acids and low pH on the gills and physiology of brown trout (Salmo trutta). Annales. Zool. Fennici. 31, 389–396. Available at: https://www.jstor.org/stable/23735677
Pugazhvendan S. R., Jothi N. N., Kumaran R. G., Kumaran S., Alagappan K. M. (2009). Effect of malathion toxicity in the freshwater fish ophiocephalus punctatus- a histological and histochemical study. World J. of Fish. Mar. Sci. 1, 218–224.
Radhaiah V., Girija M., Rao K. J. (1987). Changes in selected biochemical parameters in the kidney and blood of the fish, tilapia mossambica (Peters), exposed to heptachlor. Bull. Environ. Contam. Toxicol. 39. doi: 10.1007/BF01689591
Rainieri S., Conlledo N., Larsen B. K., Granby K., Barranco A. (2018). Combined effects of microplastics and chemical contaminants on the organ toxicity of zebrafish (Danio rerio). Environ. Res. 162, 135–143. doi: 10.1016/j.envres.2017.12.019
Rocha E., Monteiro R. A. E. (1999). “Histology and cytology of fish liver,” in Ichthyology: Recent research advances. Ed. Saksena D. N. (New Hampshire: Science Publishers), 54. doi: 10.5772/29544
Sayed A. E. H., Hamed M., Soliman H. A. M. (2021). Spirulina platensis alleviated the hemotoxicity, oxidative damage and histopathological alterations of hydroxychloroquine in catfish (Clarias gariepinus). Front. Physiol. 12, 683669. doi: 10.1007/s11356-022-23789-w
Sayed A.E.-D.H., Hana M. N., Hamed M., Abdel-Latif H. M. R., Lee J.-S., Soliman H. A. M. (2022). Protective efficacy of dietary natural antioxidants on microplastic particle-induced histopathological lesions in African catfish (Clarias gariepinus). Environ. Sci. Pollut. Res. doi: 10.1007/s11356-022-23789-W
Sayed A., Mekkawy I. A., Mahmoud U. M. (2012). Histopathological alterations in some body organs of adult clarias gariepinus (Burchell 1822) exposed to 4-nonylphenol. zoology 8, 163–184. doi: 10.3389/fphys.2021.683669
Sayed A.E.-D.H., Mekkawy I. A., Mahmoud U. M., Nagiub M. (2020). Histopathological and histochemical effects of silver nanoparticles on the gills and muscles of African catfish (Clarias garepinus). Sci. Afr. 7, e00230. doi; 10.1016/j.sciaf.2019.e00230
Sayed A.E.-D.H., Soliman H. A. M. (2018). Modulatory effects of green tea extract against the hepatotoxic effects of 4-nonylphenol in catfish (Clarias gariepinus). Ecotoxicol. Environ. Saf. 149, 159–165. doi; 10.1016/j.ecoenv.2017.11.007
Scorticati C., Prestifilippo J. P., Eizayaga F. X., Castro J. L., Romay S., Fernández M. A., et al. (2004). Hyperammonemia, brain edema and blood-brain barrier alterations in prehepatic portal hypertensive rats and paracetamol intoxication. World J. Gastroenterol. 10, 1321–1324. doi: 10.3748%2Fwjg.v10.i9.1321
Senthil Kumaran S., Kavitha C., Ramesh M., Grummt T. (2011). Toxicity studies of nonylphenol and octylphenol: Hormonal, hematological and biochemical effects in clarias gariepinus. J. Appl. Toxicol. 31, 752–761. doi: 10.1002/jat.1629
Shahid S., Sultana T., Sultana S., Hussain B., Irfan M., Al-Ghanim K., et al. (2020). Histopathological alterations in gills, liver, kidney and muscles of ictalurus punctatus collected from pollutes areas of river. Braz. J. Biol. 81, 814–821. doi: 10.1590/1519-6984.234266
Shah N., Khan A., Ali R., Marimuthu K., Uddin M. N., Rizwan M., et al. (2020). Monitoring bioaccumulation (in gills and muscle tissues), hematology, and genotoxic alteration in ctenopharyngodon idella exposed to selected heavy metals. BioMed. Res. Int. 2020, 6185231. doi: 10.1155/2020/6185231
Soliman H. A., Hamed M., Lee J.-S., Sayed A.E.-D.H. (2019). Protective effects of a novel pyrazolecarboxamide derivative against lead nitrate induced oxidative stress and DNA damage in clarias gariepinus. Environ. pollut. 247, 678–684. doi: 10.1016/j.envpol.2019.01.074
Spehar R. L., Brooke L. T., Markee T. P., Kahl M. D. (2010). Comparative toxicity and bioconcentration of nonylphenol in freshwater organisms. Environ. Toxicol. Chem. 29, 2104–2111. doi: 10.1002/etc.262
Suaria G., Avio C. G., Mineo A., Lattin G. L., Magaldi M. G., Belmonte G., et al. (2016). The Mediterranean plastic soup: synthetic polymers in Mediterranean surface waters. Sci. Rep. 6, 1–10. doi: 10.1038/srep37551
Teuten E. L., Saquing J. M., Knappe D. R. U., Barlaz M. A., Jonsson S., Björn A., et al. (2009). Transport and release of chemicals from plastics to the environment and to wildlife. Philos. Trans. R. Soc. B.: Biol. Sci. 364, 2027–2045. doi: 10.1098/rstb.2008.0284
Uguz C., Iscan M., Ergüven A., Isgor B., Togan I. (2003). The bioaccumulation of nonyphenol and its adverse effect on the liver of rainbow trout (Onchorynchus mykiss). Environ. Res. 92, 262–270. doi: 10.1016/S0013-9351(03)00033-1
Wang J., Liu X., Liu G., Zhang Z., Wu H., Cui B., et al. (2019b). Size effect of polystyrene microplastics on sorption of phenanthrene and nitrobenzene. Ecotoxicol. Environ. Saf. 173, 331–338. doi: 10.1016/j.ecoenv.2019.02.037
Wang F., Yang W., Cheng P., Zhang S., Zhang S., Jiao W., et al. (2019a). Adsorption characteristics of cadmium onto microplastics from aqueous solutions. Chemosphere 235, 1073–1080. doi: 10.1016/j.chemosphere.2019.06.196
Wendelaar Bonga S. E. (1997). The stress response in fish. Physiol. Rev. 77, 591–625. doi: 10.1152/physrev.1997.77.3.591
Wilson M. M., Peng J., Dabiri J. O., Eldredge J. D. (2009). Lagrangian Coherent structures in low reynolds number swimming. J. Physics.: Condensed. Matter. 21, 204105. doi: 10.1088/0953-8984/21/20/204105
Xu J., Ji P., Zhao Z., Zhang Y., Feng J., Wang J., et al. (2012). Genome-wide SNP discovery from transcriptome of four common carp strains. PloS One 7, e48140. doi: 10.1371/journal.pone.0048140
Keywords: polyethylene, 4-nonylphenol, common carp, liver, gills, brain (optic tectum)
Citation: Ammar E, Mohamed MS and Sayed AE-DH (2022) Polyethylene microplastics increases the tissue damage caused by 4-nonylphenol in the common carp (Cyprinus carpio) juvenile. Front. Mar. Sci. 9:1041003. doi: 10.3389/fmars.2022.1041003
Received: 10 September 2022; Accepted: 26 October 2022;
Published: 16 November 2022.
Edited by:
Kyu-Jung Chae, Korea Maritime and Ocean University, South KoreaReviewed by:
Mohamed Hamed, Al Azhar University, EgyptCopyright © 2022 Ammar, Mohamed and Sayed. This is an open-access article distributed under the terms of the Creative Commons Attribution License (CC BY). The use, distribution or reproduction in other forums is permitted, provided the original author(s) and the copyright owner(s) are credited and that the original publication in this journal is cited, in accordance with accepted academic practice. No use, distribution or reproduction is permitted which does not comply with these terms.
*Correspondence: Alaa El-Din H. Sayed, YWxhYXNheWVkQGF1bi5lZHUuZWc=
Disclaimer: All claims expressed in this article are solely those of the authors and do not necessarily represent those of their affiliated organizations, or those of the publisher, the editors and the reviewers. Any product that may be evaluated in this article or claim that may be made by its manufacturer is not guaranteed or endorsed by the publisher.
Research integrity at Frontiers
Learn more about the work of our research integrity team to safeguard the quality of each article we publish.