- 1Israel Oceanographic and Limnological Research (IOLR), National Institute of Oceanography, Haifa, Israel
- 2The Dr. Moses Strauss Department of Marine Geosciences, Charney School of Marine Sciences, University of Haifa, Haifa, Israel
- 3Department of Earth and Environmental Sciences, Ben Gurion University of the Negev, Beer-Sheva, Israel
- 4The Interuniversity Institute for Marine Sciences, Eilat, Israel
- 5The Hatter Department of Marine Technologies, Charney School of Marine Sciences, University of Haifa, Haifa, Israel
Deep-sea anoxic brine pools are unique and extreme, yet habitable environments. However, their extent and processes of formation are not fully understood. Using geophysical analysis and seafloor surveying, we discovered the eastmost brine pools known in the ultraoligotrophic Eastern Mediterranean Sea, at the Palmahim Disturbance offshore Israel (~1150 m water depth). These brine pools are located directly above a ~1km wide piece of the Messinian evaporites section, which was up thrusted to ~350 m below the seafloor. We sampled brines and short cores to characterize the chemical composition of several small (up to 5m diameter) anoxic, methanic and warm (21.6°C) brine pools and adjacent seafloor sediments porewater. The maximal salinities measured at the pools and adjacent porewater were 63.9 and 72 PSU, respectively. The brines are characterized by enriched Na and Cl concentrations by a factor of ~1.8 and depleted Mg, SO4, K and Ca contents by factors of circa 6, 3, 2 and ~1.3, respectively, compared to the ambient seawater. Relations of the major element concentrations reveal a mixing curve between seawater and enriched Na/Cl and depleted Mg/Cl, K/Cl and SO4/Cl end-members, and do not coincide with relics of fossil residual evaporated seawater. We propose their composition reflects: 1) dissolution of Messinian halite (NaCl) by seawater, supported by their low Br/Cl ratios; 2) additional small rise in Na/Cl ratios due to the impact of clay mineral dehydration or/and dissolution of trace (~1% of the Na) amounts of detrital trona (Na3H(CO3)2•2H20), coinciding with the enriched alkalinity concentrations; 3) diagenesis processes depleting Mg, K and SO4, mainly by the formation of authigenic K-rich Mg-smectite, clay mineral dehydration, dolomitization/Mg-calcite precipitation and redox processes. The δ18O and δD values of the Palmahim brine may reflect the impact of clay mineral dehydration. Comparison to all other East Mediterranean brine lakes shows that the Palmahim brine pool system represents similar provenance of brines as observed for the Eastern Mediterranean Napoli, Nadir and Tyro lakes, while potentially recording additional processes attributed to its proximity to the coastal area.
1 Introduction
Deep-sea brine pools accumulated in seafloor depressions have been discovered in different water bodies, notably in the Gulf of Mexico (Shokes et al., 1977), the Mediterranean (Jongsma et al., 1983; Scientific Staff of Cruise Bannock 1984-12 - Cita et al., 1985) and the Red Seas (Charnock, 1964; Swallow and Crease, 1965; and recently Purkis et al., 2022). The Red Sea occupies the utmost number of brine pools attributed to the dissolution of sub-surface evaporites, which were geochemically categorized into two main types, those situated along the deep axis influenced by seismic/rift spreading and consequent hydrothermal fluid and water/volcanic rock interactions and those tied to sediment alterations (Schmidt et al., 2015; Purkis et al., 2022).
The eastern Mediterranean basin contains several complexes of seafloor brine pools at different sub-basins, in which the Tyro and Bannock were the first brine pools discovered in 1983-4 (Jongsma et al., 1983; Cita et al., 1985; De Lange et al., 1990). The Discovery Basin found in 1993-94 had the highest salinity found in the marine environment (Wallmann et al., 1997b) till the recent discovery of Lake Hephaestus, the youngest athalassohaline deep-sea formation (La Cono et al., 2019). Due to their unique chemical composition, these mostly anoxic brine pools that occupy only a small area of the basin were considered hostile to life. Yet, they were found to host unique macrofauna and microbial biodiversity, being extreme environmental hot spots of productivity (Wallmann et al., 1997a; Aloisi et al., 2002; Van Der Wielen et al., 2005; Daffonchio et al., 2006; Edgcomb et al., 2007; Bernhard et al., 2014; Pachiadaki et al., 2014; Merlino et al., 2018; Steinle et al., 2018).
Brines accumulate at the seabed of collapsed basins in the East Mediterranean, having diverse thicknesses up to a few hundred meters (Cita, 2006). Moreover, high salinity values of interstitial waters (up to 350 g/L) were measured in post-Miocene sediments from different Mediterranean Deep Sea Drilling Project sites (McDuff and Gieskes, 1976; Vengosh and Starinsky, 1993; Vengosh et al., 1994). The creation and variable chemical composition of such deep anoxic brine-filled basins are related to the distribution of Messinian evaporites and suggest the dissolution of different layers or levels of the Messinian suite (Cita, 2006).
The origin of these brines is attributed to two main processes: 1) dissolution of the late Miocene (Messinian) evaporites by seawater; 2) upwards advection of fossil relict brines produced during the Messinian evaporites deposition (Vengosh et al., 1998). The second mechanism was suggested by them to represent relict seawater evaporated to different degrees of concentration and hence different chemical compositions. Several studies infer the brine origin based on the chemical composition of the brines, considering their modification by diagenetic and advection-diffusion processes. Thus, some brine lakes like the Discovery, Kryos and Hephaestus are MgCl2-dominated systems that were attributed to the dissolution of Mg evaporites, mainly bischofite (La Cono et al., 2019). Other brine lakes like, Urania, Bannock and Tyro were related to either the dissolution of different stages of evaporites precipitated in the Messinian or the consequent relics of fossil evaporated seawater entrapped in the sediments and advected upwards (De Lange et al., 1990; Vengosh et al., 1998; Cita, 2006).
The Levant Basin is underlain by Messinian evaporites, reaching a thickness of about 2 kilometers in the center of the basin and pinching out underneath the basin's margins (Gardosh and Druckman, 2006; Roveri et al., 2014; Gvirtzman et al., 2017; Meilijson et al., 2019; Manzi et al., 2021). The salt had undergone extensive and multiphasic deformation from near syn- to post-depositional (Gvirtzman et al., 2013; Feng et al., 2017). The deformation is controlled by both the marginal loading of the sediments as well as the underlying structure (Reiche et al., 2014; Gvirtzman et al., 2015; Ben Zeev and Gvirtzman, 2020). As the salt deforms, it modifies and shapes the seafloor morphology in the region. The Palmahim Disturbance is a significant (c. 50 x 15 km) submarine slide deforming the continental margin of southern Israel, attributed to gravitational slumping above the Messinian evaporites (Garfunkel et al., 1979). Local evocation of Messinian evaporites and extensive faulting at the base of the Palmahim disturbance form potential conduits that allow fluid seepage to the surface (Eruteya et al., 2018).
Following a geophysical study of the area, we conducted in April and November 2021, remotely operated vehicle (ROV) based visual surveys of the ~1150 m deep seabed in the toe domain of Palmahim Disturbance, 60 km offshore the Israeli Mediterranean coast. During this survey, we discovered a complex of several small brine pools, located geographically at the eastmost part of the basin, east of the other deep anoxic brine pools discovered in the Mediterranean Sea. Here, we report on our discovery and aim to characterize the physical and chemical settings of the brines, assessing the brine origin. Our study is based on unique in-situ measurements that were performed with a CTD mounted on an ROV arm, as well as measurements of the chemical composition in samples from the brine pool and porewater from a short sediment core at the edge of one pool.
2 Materials and methods
2.1 Geophysical data and bathymetry
Preliminary site evaluation and site selection were carried out based on an analysis of the Oz 3D seismic volume. These commercial 3D seismic data were acquired by Ion-GTX over an area of 400 km2 using ten 8 km long streamers with a cable separation of 100 m. The data were depth migrated to produce a 25 × 12.5 × 8 m resolution 3D volume. Our geophysical analysis was carried at the University of Haifa Applied Marine Exploration Lab. (AMEL) using the AspenTech Subsurface Science & Engineering software suite.
Initial bathymetric mapping of the study area was carried out using a Kongsberg EM302 and by a Knudsen chirp 3260 Sub-Bottom profiler with 3.5 kHz central frequency mounted in a gondola beneath Israel Oceanographic and Limnological (IOLR) R/V Bat Galim (Kanari et al., 2020). In January 5, 2021, a high-resolution seafloor survey was carried out using the University of Haifa ECA robotics A18D Autonomous Underwater Vehicle (AUV, SNAPIR) deployed from R/V Bat Galim. The SNAPIR AUV surveyed the seafloor with a Kraken MINSAS120 Interferometric Synthetic Aperture Sonar (SAS) at 3cm/pixel resolution, Edgetech 2205 Sub-bottom profiler and a NORBIT 400 kHz central frequency WBMS Multibeam Echo sounder. A preliminary analysis of these data, carried at AMEL, was used for the planning of the ROV survey.
2.2 ROV-based visual surveying and water and sediment sampling
Two ROV surveys, incorporating visual video and in situ measurements and sampling, were carried out in the Palmahim Disturbance toe site (Figure 1) using the University of Haifa SAAB Seaeye Leopard Yona ROV, deployed off R/V Bat-Galim. The Yona ROV is equipped with a Schilling Orion 7P Manipulator, SubC 1CAM lite Mk6 (4K) main camera as well as 3 additional SD cameras. An exploratory ROV survey of the site was carried out April 19-29, resulting in the discovery of the brine pools. The main sampling and in-situ measurement survey at the site were conducted during 15-17 November 2021.
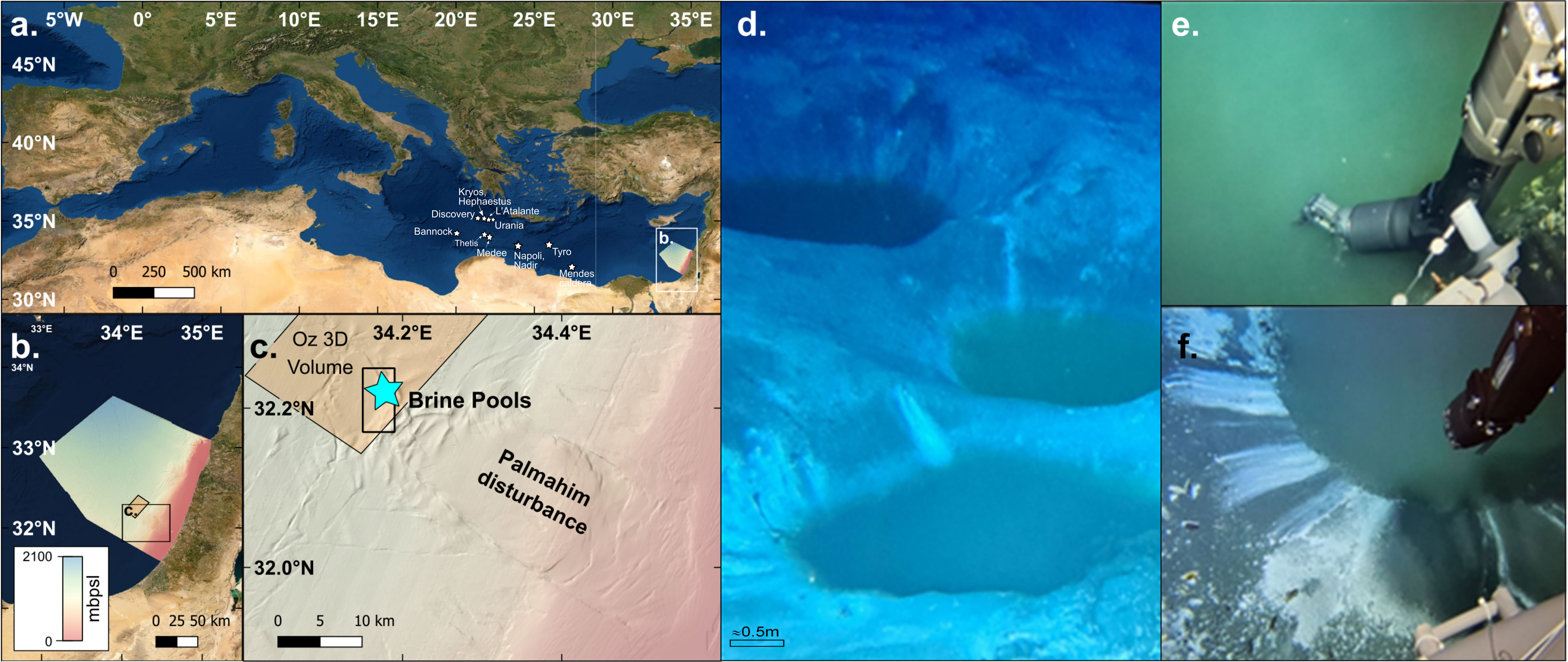
Figure 1 Bathymetry and location map of the discovered brine pools at the edge of Palmahim Disturbance, 1100m water depth (A-C). Extracts of video documentation of the brine pools as visualized by the remotely-operated vehicle (ROV) (D-F, scale in panel (D) is approximated) and in-situ CTD measurement of the brine by pumping water via a tigon tube attached to the arm of the ROV (E, F).
A Sea-Bird SBE16plusV2 CTD system was mounted on the ROV to collect in-situ measurements of pressure, temperature, salinity and dissolved oxygen. To enable a precise controlled in-situ measurements of the brine we attached a tigon tube to the arm of the ROV (Figure 1) and connected its other end to the CTD pump. Measurements of the brine pools were performed by introducing the arm alone into the pool (Figure 1). The manufacturer reported precision of the SBE16plusV2 CTD is ±0.004 for salinity (inferred from the ±0.0005 S/m conductivity precision) and ±0.005°C for temperature. The pressure, conductivity and temperature sensors were calibrated by the manufacturer (as described in Ozer et al., 2020). CTD data was processed using the Sea-Bird data processing software following the manufacturer's recommendations. In addition, water samples for dissolved oxygen were sampled and measured onboard immediately upon retrieval following the modified Winkler method (Carpenter–Winkler titration procedure Carpenter, 1965), using an automated Metrohm Titrando 905 titration system to calibrate the dissolved oxygen of the CTD.
A Niskin bottle was mounted horizontally on the front part of the ROV and was triggered using the ROV arm once inserted into the brine pool. Short push-cores (30 cm long Perspex tubes) were collected adjacent to the pool edge using the ROV. Porewaters for major and minor ions were sampled using Rhizons immediately upon retrieval of the ROV onboard from 5 depth horizons and the sediment overlaying water. Sediment samples (~2 ml) for methane concentrations were collected with edge cut syringe from the perspex corer which contains side holes (1 cm in diameter) and immediately transferred into a flushed argon glass bottle containing 5 ml sodium hydroxide (1.5 N) for headspace measurements of methane concentration (after Nusslein et al., 2003). The bottle was sealed with a crimper.
Ambient seawater samples were collected with the R/V Bat-Galim at parallel depths (~1150 m) using Niskin bottles mounted on a Seabird rosette at about 50 km northward to Palmahim Disturbance for the comparison between the chemical composition of the brine pools and porewater to the ambient seawater.
2.3 Chemical analysis
Onboard, water samples were immediately collected directly from the Niskin bottles. The brine salinity (expressed as conductivity), pH, and dissolved oxygen concentrations were measured onboard immediately upon sampling using a WTW Multi 3630 IDS sensor. The pH electrode was calibrated against the standard NBS buffers.
2.3.1 Major and minor ions
Chemical analysis of the brine, porewater and seawater samples was performed by standard spectrometric methods: Na, K, Mg, Ca, and Sr were analyzed by ICP-OES (Inductively Coupled Plasma Optical Emission Spectrometry) Optima 5300 Perkin Elmer. Samples dilution was 1:10, an internal standard of Scandium (Sc) was used (final concentration of 5 ppm) and the analytical precision was estimated within ±0.5%. Cl and Br were analyzed by Dionex ICS-2000 Ion Chromatography. The analytical precision was within ±3%. Ba, Li and Mn were measured by ICP-MS (Inductively coupled Plasma Mass Spectrometry) NexION 300D, Perkin Elmer. The analytical precision was within ±10%. Quality control was performed by the run of 3 Standard Reference Samples (SRSs) of the U.S. Geological Survey (USGS).
2.3.2 Total alkalinity
was measured by potentiometric titration with a Methrom, 848 Titrino plus system using the Gran method to calculate it from acid volumes and corresponding pH measurements between pH 3.3 and 3.8 (Sass and Ben-Yaakov, 1977). The titration acid was 0.05 M HCl, which was verified and adjusted using certified reference seawater supplied by the Certified Reference Materials Laboratory, Scripps Institution of Oceanography, CA (Dickson et al., 2003). Duplicate measurements were made for each sample and the precision error was ±1 µmole·kg-1.
2.3.3 Methane
Was measured from the headspace on a Focus Gas Chromatograph (Thermo) equipped with a flammable ionization detector (FID) at a precision of 2 mmol CH4 L-1 (Sela-Adler et al., 2017).
2.3.4 δ18O, δD measurements
For δ18O measurements clean vacuum vessels were flushed with a gas mixture of He (99.6%) and CO2 (0.4%) for 10 minutes to remove the original atmosphere. After flushing, 0.7 cm of the sampled water was injected to the vessels and left to equilibrate with the CO2 gas for at least 48 hours at 25°C. Values of δ18O were measured using a Finnigan Gas Bench II extraction system in continuous flow connection with a ThermoFinnigan Delta V mass-spectrometer, following the CO2 – H2O equilibration technique (Epstein and Mayeda, 1953). All oxygen isotopic measurements were made in duplicates and are reported relative to Standard Mean Ocean Water (SMOW). Four well-quantified internal laboratory standards were used for calibration. δD measurements were performed using a Thermo Finnigan High-Temperature Conversion Elemental Analyzer (Flush2000-EA) attached to a Delta V mass-spectrometer at a reaction temperature of 1450°C (Nelson, 2000). δD values are reported relative to SMOW. Analytical reproducibility of duplicates was better than 0.1‰ for δ18O and 1.0‰ for δD.
3 Results and discussion
3.1 Geophysical setting at the Palmahim Disturbance brine pools
The study area is located in the northwest part of the Palmahim Disturbance compressional toe domain, as defined by Garfunkel et al. (1979). Our analysis shows that the Messinian to present sedimentary section in this area has undergone complex deformation (Figure 2). This includes folding and combined southeastward up thrusting and left lateral strike-slip displacements along multiple salt-rooted faults. These faults accommodate hundreds of meters of offsets, changing their modes and deformation amplitude along their strike and producing ridges and throughs in the present bathymetry. In particular, an up to ~ 1 km wide and ~3 km long portion of the Messinian evaporites section was up-thrusted by ~1 km, with its top reaching ~350 m below the present seafloor. Directly above the shallowest tip of the up-thrust Messinian sliver, we observe tens of meters wide bathymetric depressions, underlain by high amplitude reflectivity within tens of meters below the seafloor. Similar reflections have been related to active methane seepage farther to the south at the toe domain of Palmahim Disturbance (Rubin-Blum et al., 2014; Eruteya et al., 2018; Tayber et al., 2019). These observations prompted the focused seafloor surveying of this site, leading to the discovery of the Palmahim Disturbance brine pools. We, therefore, suggest that focused seepage of fluids and methane, routed through the subsurface Messinian evaporites sliver, lead to the formation and on-going seepage of the discovered brine pools. The deformation and faulting of the salt have been suggested as a mechanism that may facilitate the migration of fluids to the seafloor in the Levant basin (Eruteya et al., 2018; Tayber et al., 2019; Oppo et al., 2021).
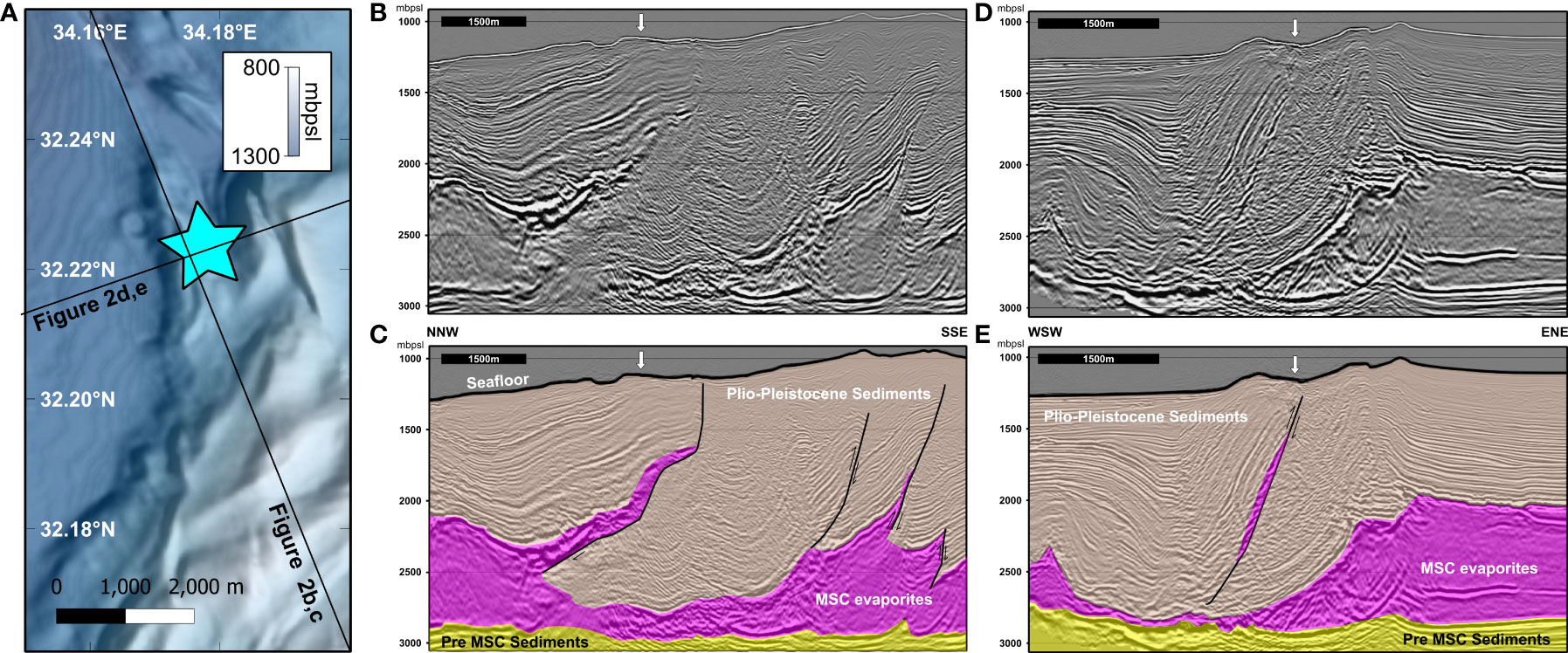
Figure 2 Seismic imaging of the subsurface near the brine pools. Two intersecting depth migrated profiles across the location or the brine pools (star in A, arrow in B-E), one along an NNW-SSE trend (B, C) and one along a WSW-ENE (D, E) show the Messinian salt being segmented by faults and up thrusted towards the surface, reaching to shallowest levels beneath the discovered brine pools.
Seafloor surveying of the site discovered a complex of several small (up to 5 m diameter) brine pools (Figure 1) at 1150 m water depth, in the toe domain of Palmahim Disturbance, 60 km off the Israeli Mediterranean southern coastline. At least 5 brine pools were positively identified. The pools are clustered within an area of ~1,500 m2 and visually seem to be inter-connected (in part) by a system of stream channels. All pools have circular shapes probably owning to their mode of development and formation.
3.2 Geochemistry of the Palmahim Disturbance small brine pools
We mapped the distribution patterns of salinity, temperature and dissolved oxygen in selected brine pools and their vicinity, based on the in-situ CTD measurements, which were segmental, as the CTD pump was not enabled constantly to avoid damage by sediment particles (Figure 3). The maximal recorded salinity and temperature were 63 PSU and 21.6°C, whereas all measurements adjacent and above the pool complex or brine-seawater interface, were higher than the ambient background levels (Figure 3). Our observations suggest that regardless of the sharp interface between the brine and bottom seawater, the latter exhibits minor enrichment of salinity and temperature probably attributed to certain diffusion or advection caused by the bubbling out or upwards of methane bubbles, as visualized by the ROV survey. We also consider disturbance by biota, mainly by the shark Galeus melastomus, which occurred in large numbers in the brine pools area, and was often observed swimming next to the brine pools. In-situ dissolved oxygen measurements indicate anoxic conditions (<0.1% saturation) within the brine pools. This coincides with the onboard measurements showing values of 0.04-0.7 mg L-1 (decreasing), likely representing both the limited mixing with the bottom seawater and the substantial respiration at the interface. We measured high concentrations of dissolved Mn (up to 10.1 µM) in the brine pools and porewater (Figure 4; Tables 1, 2), which are typical below oxic-anoxic interfaces, including anoxic brines (De Lange et al., 1990).
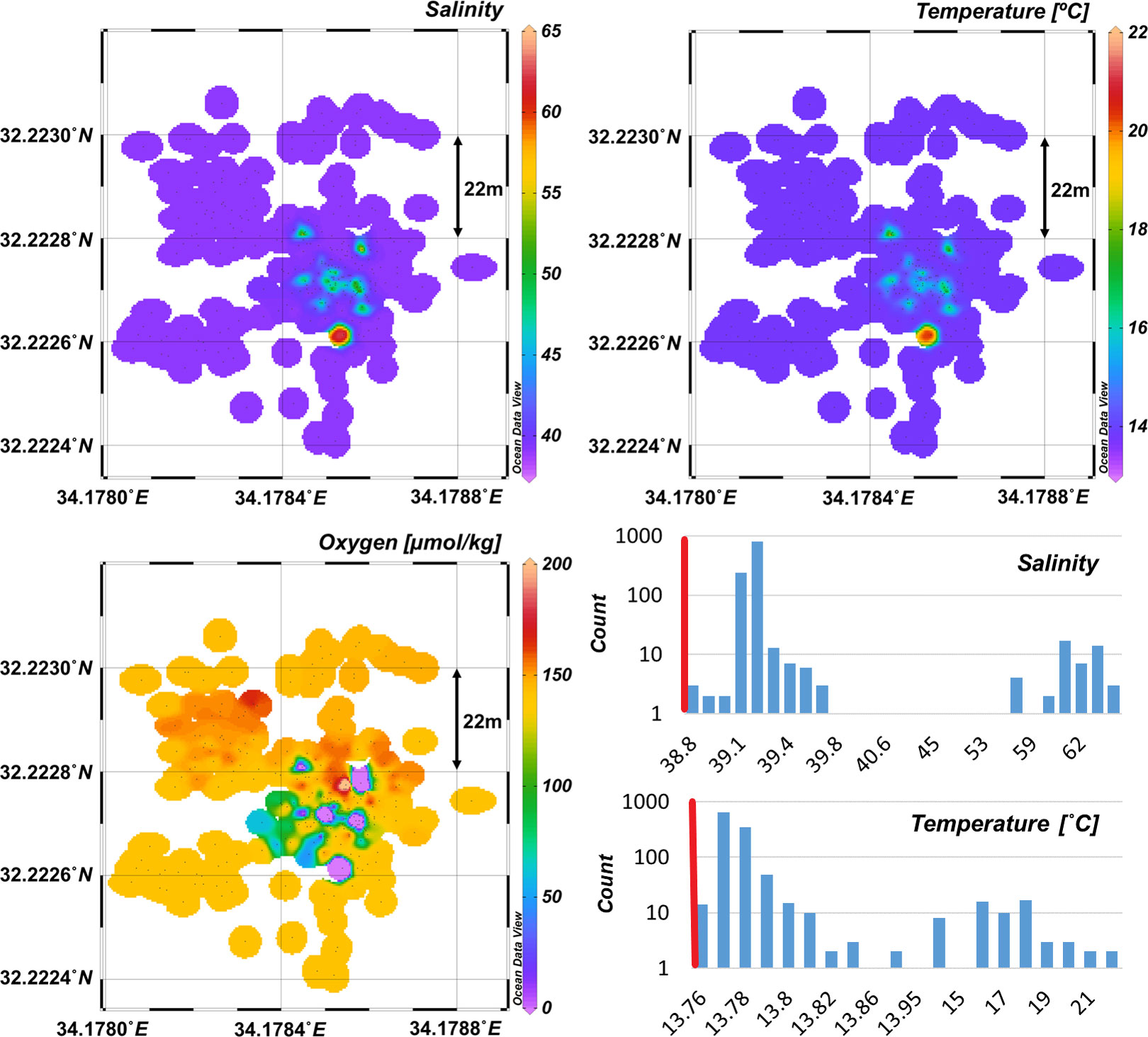
Figure 3 Distribution map of salinity, temperature and dissolved oxygen concentrations measured in-situ (CTD mounted on the ROV). The column plots represent all the recorded in-situ data and the vertical dotted red line the ambient background levels for salinity and temperature (38.767 psu and 13.75°C; recorded at the same depth close to the study area during 2013 (March); 2016 (Sept.); 2017 (Sept)).
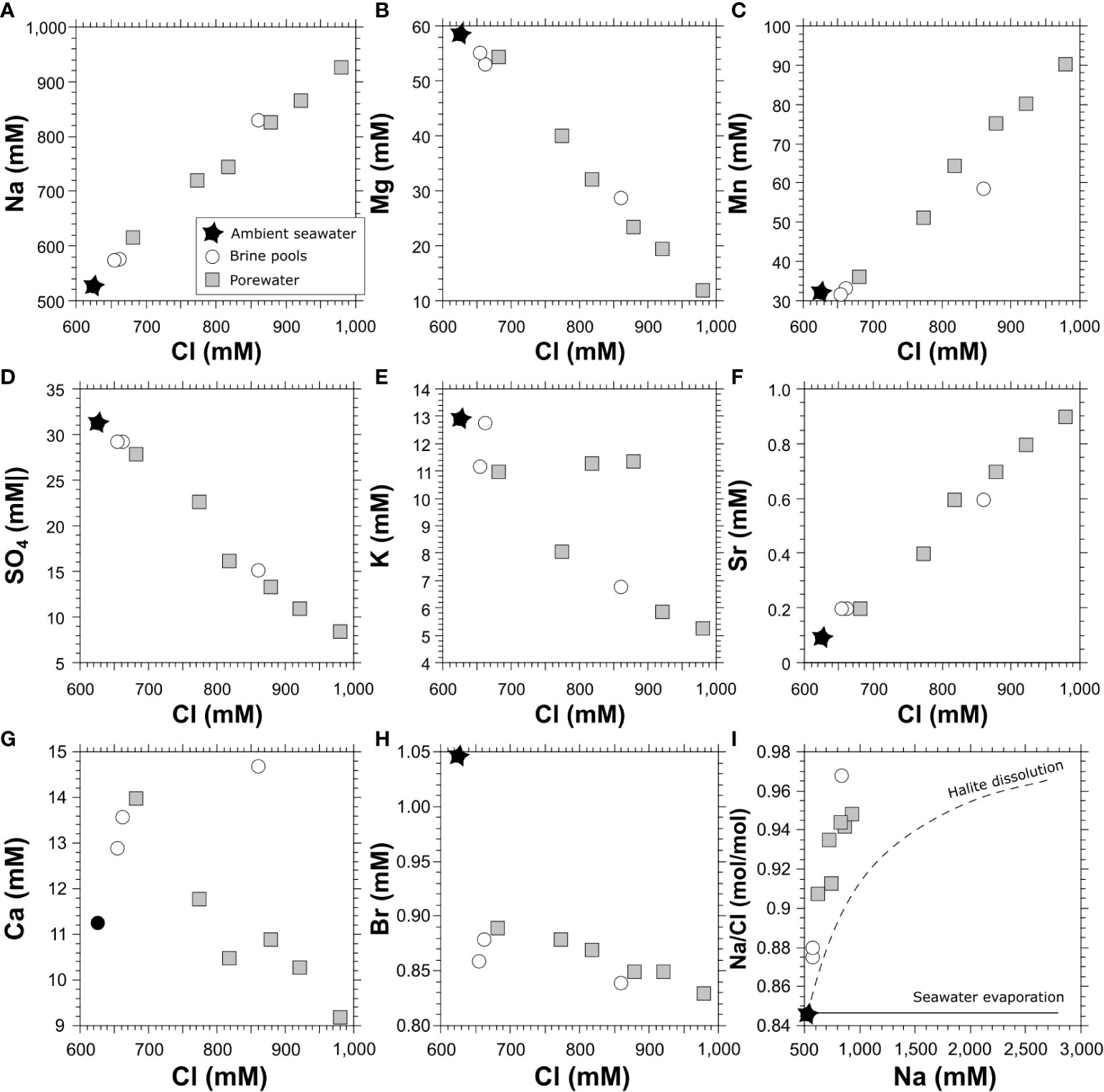
Figure 4 Ion concentrations variations vs. chloride concentrations of brine water and porewater from Palmahim site, and of ambient seawater. The lower plot includes the evaporation of seawater curve and the mixing curve between seawater and dissolution of halite.
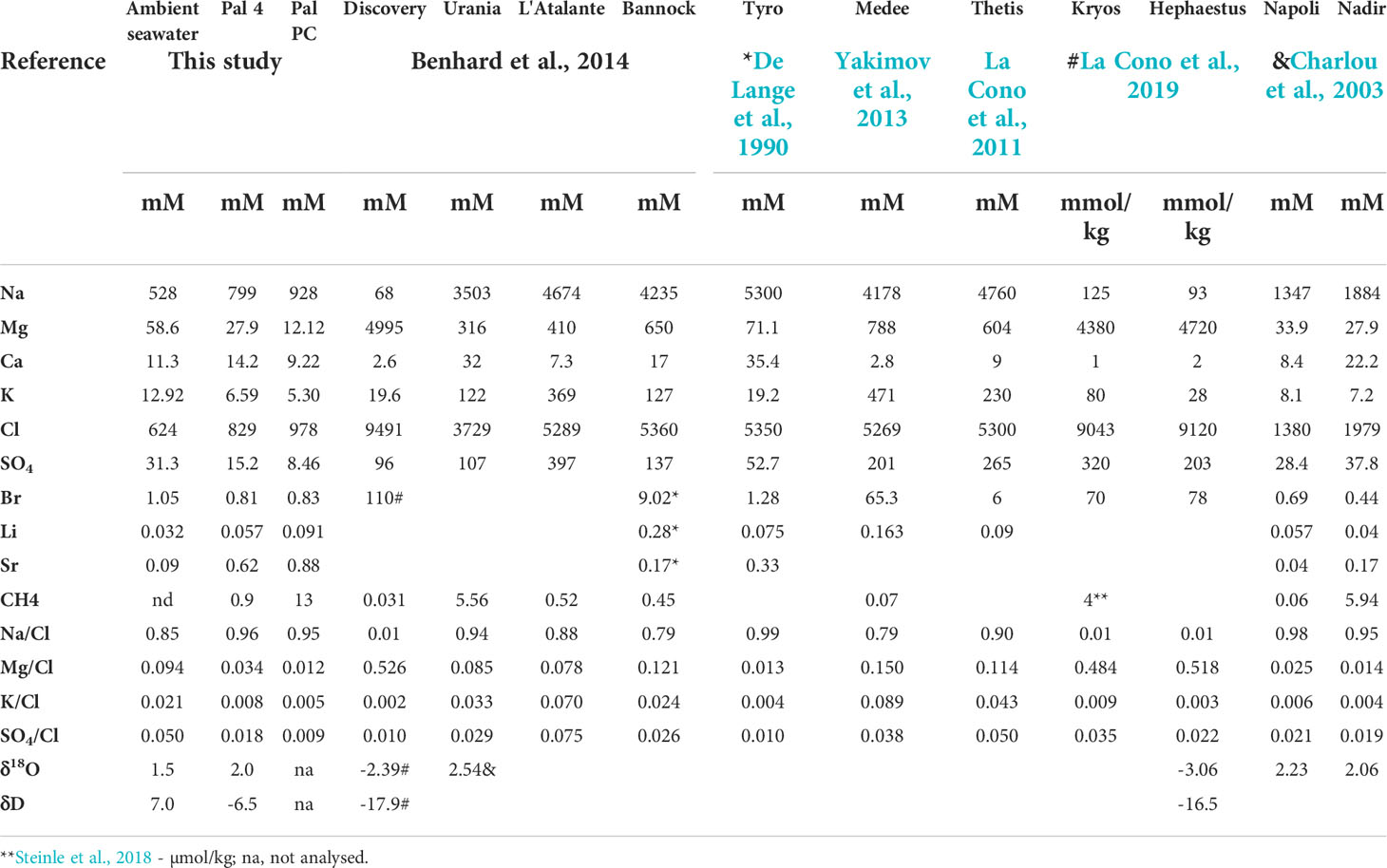
Table 2 Chemical composition of the Palmahim brine pool (Pal 4) and porewater (Pal PC), ambient seawater and brine pools in the Eastern Mediterranean seafloor.
We collected 3 samples from two distinct brine pools with a horizontal Niskin bottle mounted and triggered by the ROV. While it was technically impossible to set the Niskin vertically, the replacement of water via the horizontal Niskin was limited in the smaller and shallower brine pool (samples BP3 and BP5, Table 1) and thus the collected water represents the brine and some "contamination" of bottom seawater. Therefore, the chemical composition of those samples represents a mixture between the brine end-member and the ambient seawater. We additionally analyzed the porewater chemical composition of a short (~12 cm) push core collected by the ROV at the edge of one of the pools (Figure 1). The chemical composition of the most saline brine and porewater samples at Palmahim Disturbance, ambient seawater and other brine lakes in the Eastern Mediterranean Sea were compared to assess their origin (Table 2). In the Palmahim Disturbance brine, Na, Cl, Li and Sr are enriched, while Mg, K, SO4, Br are depleted compared to the ambient seawater (Figure 4). Compared to other Mediterranean brine pools, our results exhibited the lowest Mg concentrations and similar Na/Cl and Mg/Cl ratios as Napoli, Nadir and Tyro brine pools (Table 2).
The chemical composition of all the samples collected here, 3 brine pools and 6 porewater samples, are presented in Table 1, and were used to assess their provenance (end-members) by comparison to the expected seawater evaporation composition, dissolution of Messinian evaporites and potential diagenetic processes.
Cross-plotting relationships of the major element concentrations reveal a mixing curve between seawater and enriched Na or Cl and depleted Mg, K, SO4 end-member (Figure 4). The linear increasing trend line for Na, Li and Sr vs. Cl, and the linear decreasing trend line for Mg and SO4 vs. Cl (Figure 4) suggests an enriched NaCl and depleted Mg and SO4 end member impacting the brine pool and top porewater composition. The potential enrichment of Na and Cl via the dissolution of subsurface evaporitic halite attributed to the Late Miocene (Messinian) crisis by seawater cannot explain the observed relatively high Na/Cl ratios to the full extent (Figure 4). These ratios are much higher than the expected ratios attributed to relics of seawater evaporation path or modified/residual brines after the precipitation of evaporites, starting with halite at a degree of evaporation >10 or Na concentration of ~5500 mM (McCaffrey et al., 1987; Shalev et al., 2018).
A recent study suggests that porewater from the Napoli mud volcano may be affected by clay mineral dehydration hence a decreasing chlorinity and thus increasing Na/Cl ratios (Behrendt et al., 2022). Such a process may explain an enriched Na/Cl end-member which consists of halite dissolution by seawater and additional diagenetic removal of Cl. The relatively low Br/Cl ratios also support the dissolution of early-stage halite evaporites containing low crystalized Br (Shalev et al., 2018).
Considering the linear decreasing relationships between Mg and SO4 vs. Na or Cl (Figure 4) and assuming a Na end-member at complete removal of Mg and SO4, a value of 1034-1056 mM Na is calculated. This range represents a contribution of approximately 35% additional Na due to halite dissolution and removal of ~8% of Cl by dehydration. An alternative process that may explain Na/Cl ratios higher than expected by seawater dissolution of halite is the addition of Na via dissolution of trace amounts (~1% Na) of detrital trona (Na3H(CO3)2•2H20) and/or thenardite (Na2SO4), which were observed in sediment cores off the Nile delta (Stanley and Sheng, 1979). The most dominant alkalinity-producing reactions are anaerobic oxidation of methane and carbonate dissolution, followed by sulfate reduction and denitrification processes (Aloisi et al., 2002; Brenner et al., 2016). While the anaerobic oxidation of methane results in a very alkaline environment which promotes carbonate precipitation, sulfide oxidation results in a highly corrosive environment due to the production of sulfuric acid. In addition, at the brine-seawater interface the organic matter mineralization and release of CO2 may also have a small corrosive impact (Sisma-Ventura et al., 2021). The increased alkalinity in the anoxic brine pools, may thus reflect the latter reactions. However, given that the change in alkalinity is not conservative, and that it does not correlate to a change in Ca concentration, another mechanism may also contribute. This might be the dissolution of trona which would contribute bicarbonate. Indeed, the enriched alkalinity concentrations in the 3 pool samples (Table 1; up to 15,363 µmol kg-1) show much higher values than ~ 2610 µM of the ambient seawater (Sisma-Ventura et al., 2016), similar to the corresponding dissolution of trona, assuming an addition of approximately 1% Na.
The lower Mg, K and SO4 contents support interaction with sediments during early-to-late diagenesis (Boschetti et al., 2011) and redox processes (Van Der Weijden, 1992). A depletion of SO4 is attributed to sulfate reduction and the anaerobic oxidation of methane. The Palmahim Disturbance porewater show clear methane enrichment ranging from 4 to 13.8 mmol CH4 L-1. Methane emission may be related to compressed sediments or gas hydrate destabilization, which may occur at mud volcanoes, seeps and vents related to fault systems (Charlou et al., 2003). We note that considering the anoxic conditions in the brine pools and porewater, it is likely that a certain portion was originally presented as H2S and thus the SO4 versus Cl would have been different.
The depletion of K and Mg from porewater may be attributed to the formation of authigenic K-rich Mg-smectite in marine evaporative environments (Hover, 1999) and dolomitization. In addition, subsurface processes of burial diagenesis of the transformation of smectite to illite clay mineral fixes K from porewater even under relatively low temperatures (Hover et al., 2002; Ijiri et al., 2018)
The δ18O and δD values of the most saline brine pool at Palmahim Disturbance are 2.0‰ and -6.5‰, respectively. Slightly enriched in 18O but heavily depleted in 2H (D) compared to mean values of 1.5‰ and 7.0 ‰ of the EMS deep water (Sisma-Ventura et al., 2016), respectively (Figure 5). Messinian halite fluid inclusions may carry depleted or enriched, positively correlated δ18O and δD values (Rigaudier et al., 2011; Evans et al., 2015), yet counting for only ~0.2% wt, their dissolution results with minimal isotopic impact during mixing with the seawater end-member. The δ18O and δD values of the most saline brine pool may therefore reflect the impact of clay mineral dehydration (Dählmann and de Lange, 2003) as for the isotopic composition of pore and fluid water from sediment cores at the Napoli and Milano mud volcanoes dome sites, yet slightly depleted in the δ18O. They indicate a deep end-member typified by enriched δ18O and depleted δD values related mainly to the smectite-illite transformation process and estimated that this reaction efficiently removes Cl. The latter may explain both, the isotopic composition and the excessively high Na/Cl ratios in the Palmahim Disturbance brines. In addition, the δ18O values in Palmahim Disturbance are somewhat lower than the expected levels of evaporated seawater to the salinity of 63 PSU, reinforcing that its brine isotopic composition is not solely sourced from evaporated seawater. Yet, the slightly enriched δ18O but much depleted in δD compared to the EMS deep water indicates that clay mineral dehydration is likely affecting the brine pool isotopic composition.
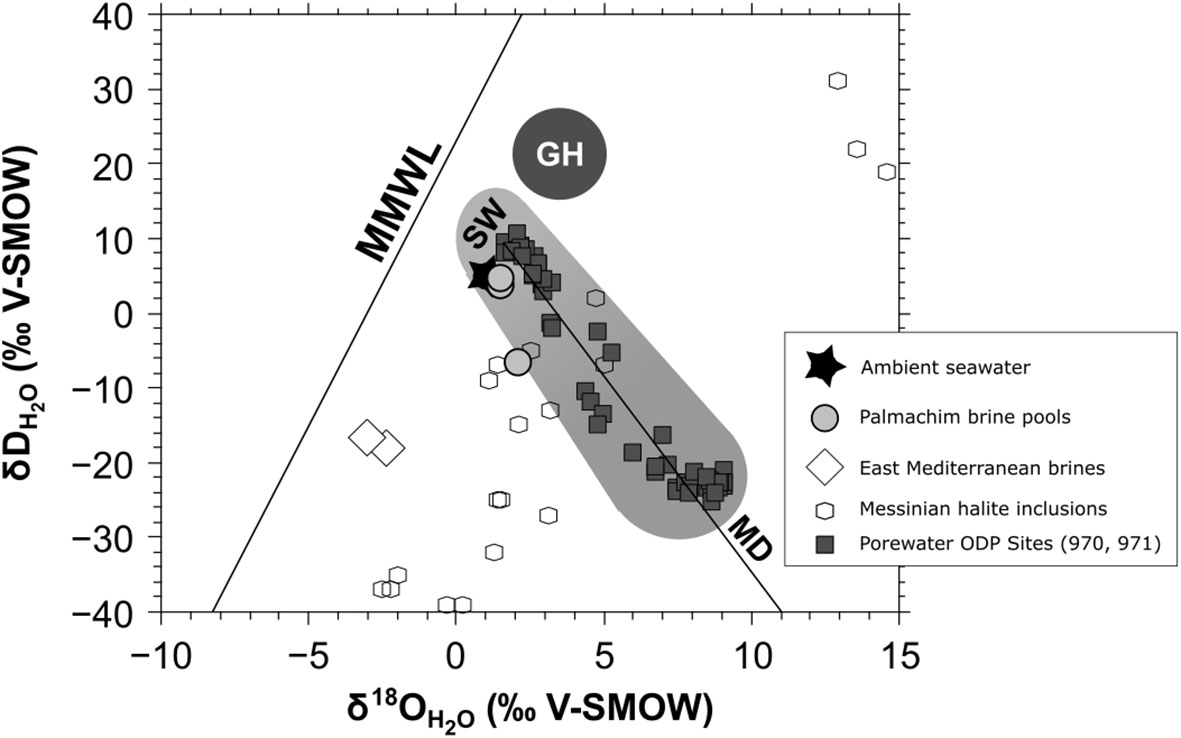
Figure 5 δ18O and δD values of Palmahim Disturbance brine samples (circles), ambient seawater (star) and the Discovery and Hephaestus brine lakes (diamonds). From Dählmann and de Lange (2003): the distribution range of pore fluids from 2 ODP sites (970 and 971) at Milano and Napoli mud volcanoes (gray squares and mixing line); GH - gas hydrate (δ18O +3‰, δD +20‰); MD - clay mineral dehydration (trend leading towards δ18O +20‰, δD -70‰). MMWL - Mediterranean meteoric water line (δD =8 δ18O +22‰) with meteoric water that corresponds to the SW value. Messinian halite inclusions fall on the line (δD =3.82 δ18O -26.41‰) (Rigaudier et al., 2011).
3.3 Comparison to other Eastern Mediterranean brine pools
The major ion ratios, mainly cross plotting of ion/chloride ratios (Figure 6) assist in revealing the characteristics and origin of the Palmahim Disturbance brine/porewater as compared to other East Mediterranean brines (Table 2 and references therein). Two main mechanisms were suggested as a source of the East Mediterranean brine pools: 1) dissolution of Messinian, mainly late-stage evaporites, precipitated along different degrees of seawater evaporation. The following main minerals were experimentally identified at different degrees of evaporation: gypsum (CaSO4.2H2O), halite (NaCl), epsomite (MgSO4•7H2O), kainite (KMgClSO4•3H2O), carnallite (MgKCl3•6H2O), kieserite (MgSO4•H2O) and bischofite (MgCl2•6H2O) (McCaffrey et al., 1980; Shalev et al., 2018); 2) relics of fossil residual evaporated seawater representing different degrees of evaporation/salinity. During the evaporation path of seawater, the composition or ions ratios of the relict brine change and they become relatively enriched in Li, Br and B (Vengosh et al., 1998; Vengosh et al., 2000; Shalev et al., 2018) attributed to salts precipitation (McCaffrey et al., 1980; Shalev et al., 2018). The latter two processes may interact and are followed by diagenetic processes mainly water-rock interactions, which further changes their composition, mainly regarding Mg, Ca, K and SO4.
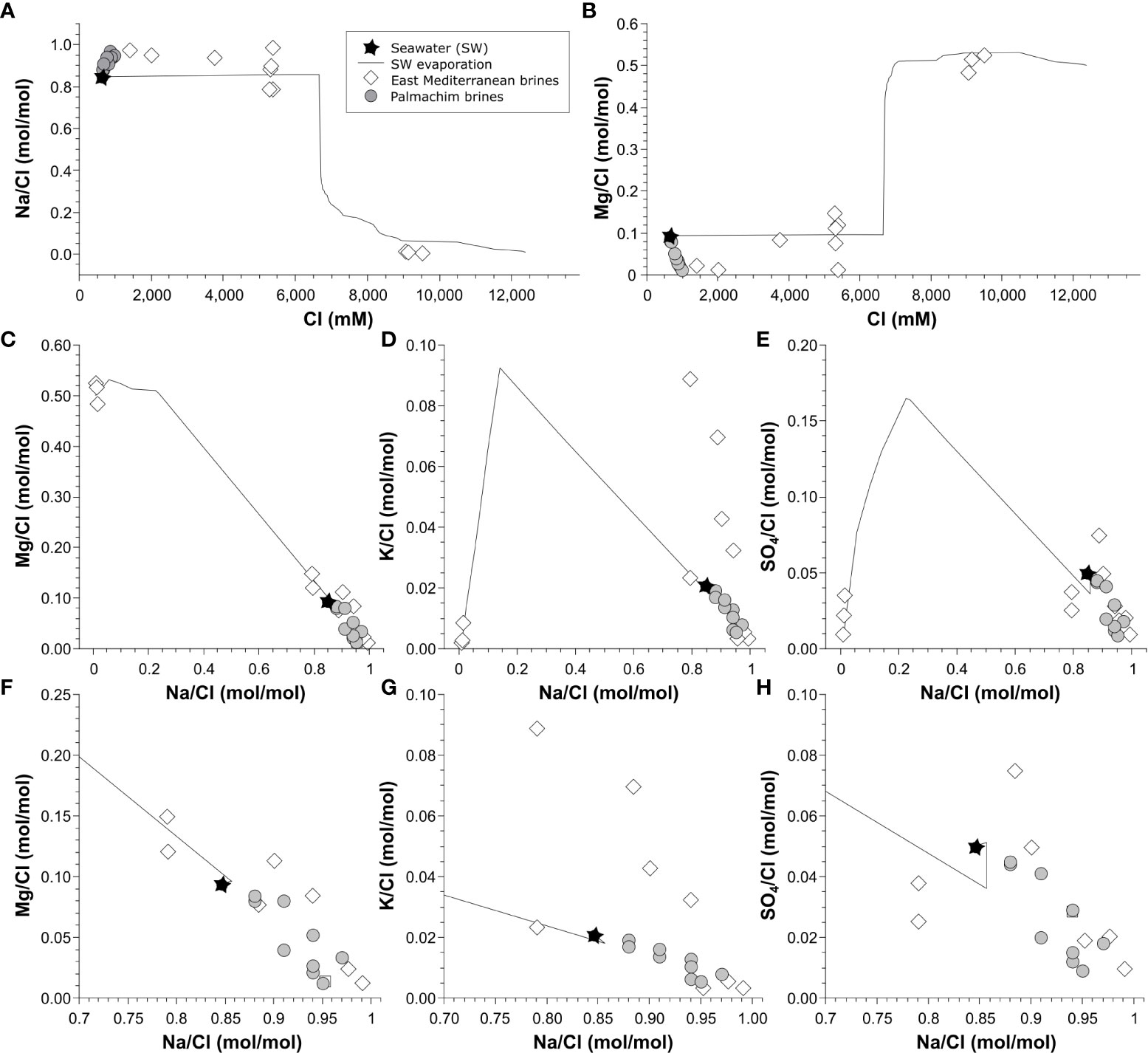
Figure 6 Relationships of the ratio between ions and chloride of the Palmahim Disturbance (circles) and other East Mediterranean brine pools (diamonds; based on Table 2). The seawater evaporation path is presented by the black line (based on Shalev et al., 2018).
The Na/Cl and Mg/Cl ratios vs. Cl (Figure 6) show brine pools attributed to halite dissolution (e.g. Lakes Napoli, Tyro), dissolution of Mg salts under late-stage evaporation, mainly bischofite, for example, lakes Hephaestus, Discovery and Kryos (La Cono et al., 2019) and of relics evaporated brines, such as Urania and Bannock (Vengosh et al., 1998; Charlou et al., 2003). The Palmahim Disturbance brine represents the lowest Mg/Cl ratios and a Na/Cl ratio beyond the maximum that can be attributed to the dissolution of halite based on its Na or Cl enrichments from the ambient seawater concentration. Nonetheless, the Palmahim Disturbance brine show Na/Cl, Mg/Cl and K/Cl ratios similar to the Napoli and Nadir lakes, as well as to Tyro lake, except Mg/Cl (Figure 6).
The Palmahim Disturbance brine/porewater represents separate characteristics from other East Mediterranean brines in terms of its relatively high and low Na/Cl and Mg/Cl ratios, respectively. While most other brine lakes are located at the deep basin (bathyal depths), the Palmichim Disturbance site is at the toe of the continental slope (relatively close to the coastline), potentially exposed to coastal shelf sabkhas in the past (Lugli et al., 2013). These coastal features would also be connected to the base of the slope through an extensive canyon system that excavated the Levant margin prior and during the salt emplacement (Buchbinder and Zilberman, 1997; Reolid et al., 2022).
4 Summary
We report here for the first time the chemical characteristics of the eastmost brine pools discovered in the eastern Mediterranean basin. Based on their chemical composition it is suggested that this small brine pools system represent similar provenance of brines as observed in the Napoli lake while recording additional process attributed to water-rock interactions, redox processes and potential impacts of its proximity to the past coast and evaporative sabkhas. Its physical-chemical characteristics and methane-degassing environment create a unique biological oasis in contrast to its barren, ultra-oligotrophic surroundings.
Data availability statement
The original contributions presented in the study are included in the article/supplementary material. Further inquiries can be directed to the corresponding authors.
Author contributions
Conceptualization: MY, R-BM and HB; Data acquiring: HB, S-VG, OT, KM, AG, GA, JY, BO, MY, R-BM; Formal analysis: HB, S-VG, BO, LM, MY; Project administration: R-BM, MY; Writing original draft: HB, S-VG, BO, R-BM, MY with the help of authors. All authors approved the submitted version.
Funding
This study was supported by the Israel Ministry of Energy; by the Israeli Science Foundation (ISF) grant 913/19, the Ministry of Science and Technology grant (001126), and partly supported by the National Monitoring Program of Israel's Mediterranean waters, the Mediterranean Sea Research Center of Israel (MERCI), the University of Haifa – GEOMAR Helmholtz Abroad funded EMSFORE project and the University of Haifa Charney School of Marine Sciences internal funds.
Acknowledgments
The authors thank the captain and crew of the R/V Bat Galim operated by the Israel Oceanographic and Limnological institute (IOLR), as well as the University of Haifa Underwater Vehicle Lab team, and Oded Ezra, Astral Subsea, operating the Yona ROV. The authors thank all individuals who helped during the expeditions, including onboard technical and scientific personnel. We thank Prof. Orit Sivan and Efrat Eliani-Rusak for performing the methane analyses and the research assistants at the physical oceanography and marine chemistry departments at IOLR. We thank the Oil Commissioner's office of the State of Israel Ministry of Energy for permitting the use of the Oz seismic data; and AspenTech for sponsoring their SSE software suite to the University of Haifa.
Conflict of interest
The authors declare that the research was conducted in the absence of any commercial or financial relationships that could be construed as a potential conflict of interest.
Publisher’s note
All claims expressed in this article are solely those of the authors and do not necessarily represent those of their affiliated organizations, or those of the publisher, the editors and the reviewers. Any product that may be evaluated in this article, or claim that may be made by its manufacturer, is not guaranteed or endorsed by the publisher.
References
Aloisi G., Bouloubassi I., Heijs S., Pancost R. D., Pierre C., Sinninghe Damsteé J. S., et al. (2002). CH4-consuming microorganisms and the formation of carbonate crusts at cold seeps. Earth Planet. Sci. Lett. 203, 195–203. doi: 10.1016/S0012-821X(02)00878-6
Behrendt N., Menapace W., Bohrmann G., Kopf A. J. (2022). “The Mediterranean ridge 25 years after ODP leg 160 drilling: New discoveries on mud volcanism and fluid-rock interactions in the olimpi mud volcano field,” in EGU general assembly 2022(Vienna, Austria), EGU22–E2339. doi: 10.5194/egusphere-egu22-2339
Ben Zeev Y., Gvirtzman Z. (2020). When two salt tectonics systems meet: Gliding downslope the Levant margin and salt out-squeezing from under the Nile delta. Tectonics 39, e2019TC005715. doi: 10.1029/2019TC005715
Bernhard J. M., Kormas K., Pachiadaki M. G., Rocke E., Beaudoin D. J., Morrison C., et al. (2014). Benthic protists and fungi of Mediterranean deep hypsersaline anoxic basin redoxcline sediments. Front. Microbiol. 5. doi: 10.3389/fmicb.2014.00605
Boschetti T., Toscani L., Shouakar-Stash O., Iacumin P., Venturelli G., Mucchino C., et al. (2011). Salt waters of the northern apennine foredeep basin (Italy): Origin and evolution. Aquat. Geochem. 17, 71–108. doi: 10.1007/s10498-010-9107-y
Brenner H., Braeckman U., Le Guitton M., Meysman F. J. R. (2016). The impact of sedimentary alkalinity release on the water column CO2 system in the north Sea. Biogeosciences 13, 841–863. doi: 10.5194/bg-13-841-2016
Buchbinder B., Zilberman E. (1997). Sequence stratigraphy of Miocene-pliocene carbonate-siliciclastic shelf deposits in the eastern Mediterranean margin (Israel): effects of eustasy and tectonics. Sediment. Geol. 112, 7–32. doi: 10.1016/S0037-0738(97)00034-1
Carpenter J. H. (1965). The accuracy of the winkler method for dissolved oxygen analysis. Limnol. Oceanogr. 10, 135–140. doi: 10.4319/lo.1965.10.1.0135
Charlou J., Donval J., Zitter T., Roy N., Jean-Baptiste P., Foucher J., et al. (2003). Evidence of methane venting and geochemistry of brines on mud volcanoes of the eastern Mediterranean Sea. Deep Sea Res. Part I Oceanogr. Res. Pap. 50, 941–958. doi: 10.1016/S0967-0637(03)00093-1
Cita M. B. (2006). Exhumation of messinian evaporites in the deep-sea and creation of deep anoxic brine-filled collapsed basins. Sediment. Geol 188–189, 357–378. doi: 10.1016/j.sedgeo.2006.03.013
Cita M. B., Kastens K. A., McCoy F. W., Aghib F., Cambi A., Camerlenghi A., et al. (1985). Gypsum precipitation from cold brines in an anoxic basin in the eastern Mediterranean. Nature 314, 152–154. doi: 10.1038/314152a0
Daffonchio D., Borin S., Brusa T., Brusetti L., van der Wielen P. W. J. J., Bolhuis H., et al. (2006). Stratified prokaryote network in the oxic-anoxic transition of a deep-sea halocline. Nature 440, 203–207. doi: 10.1038/nature04418
Dählmann A., de Lange G. (2003). Fluid–sediment interactions at Eastern Mediterranean mud volcanoes: a stable isotope study from ODP leg 160. Earth Planet. Sci. Lett. 212, 377–391. doi: 10.1016/S0012-821X(03)00227-9
De Lange G., Middelburg J., van der Weijden C., Catalano G., Luther G., Hydes D., et al. (1990). Composition of anoxic hypersaline brines in the tyro and bannock basins, eastern Mediterranean. Mar. Chem. 31, 63–88. doi: 10.1016/0304-4203(90)90031-7
Dickson A. G., Afghan J. D., Anderson G. C. (2003). Reference materials for oceanic CO2 analysis: a method for the certification of total alkalinity. Mar. Chem. 80, 185–197. doi: 10.1016/S0304-4203(02)00133-0
Edgcomb V. P., Bernhard J. M., Jeon S. (2007). Deep-Sea microbial eukaryotes in anoxic, microoxic, and sulfidic environments. In: Seckbach J.. (eds) Algae and Cyanobacteria in Extreme Environments. Cellular Origin, Life in Extreme Habitats and Astrobiology, Springer, Dordrecht 11. doi: 10.1007/978-1-4020-6112-7_39
Epstein S., Mayeda T. (1953). Variation of O18 content of waters from natural sources. Geochim. Cosmochim. Acta 4, 213–224. doi: 10.1016/0016-7037(53)90051-9
Eruteya O. E., Reshef M., Ben-Avraham Z., Waldmann N. (2018). Gas escape along the palmachim disturbance in the Levant basin, offshore Israel. Mar. Pet. Geol. 92, 868–879. doi: 10.1016/j.marpetgeo.2018.01.007
Evans N. P., Turchyn A. V., Gázquez F., Bontognali T. R. R., Chapman H. J., Hodell D. A. (2015). Coupled measurements of δ 18 O and δ d of hydration water and salinity of fluid inclusions in gypsum from the messinian yesares member, sorbas basin (SE Spain). Earth Planet. Sci. Lett. 430, 499–510. doi: 10.1016/j.epsl.2015.07.071
Feng Y. E., Steinberg J., Reshef M. (2017). Intra-salt deformation: Implications for the evolution of the messinian evaporites in the Levant basin, eastern Mediterranean. Mar. Pet. Geol. 88, 251–267. doi: 10.1016/j.marpetgeo.2017.08.027
Gardosh M. A., Druckman Y. (2006). Seismic stratigraphy, structure and tectonic evolution of the levantine basin, offshore Israel Vol. 260 (London, Special Publications: Geological Society), 201–227.
Garfunkel Z., Arad A., Almagor G. (1979). The palmahim disturbance and its regional setting. Geol. Surv. Isr. Bull., 56.
Gvirtzman Z., Manzi V., Calvo R., Gavrieli I., Gennari R., Lugli S., et al. (2017). Intra-messinian truncation surface in the Levant basin explained by subaqueous dissolution. Geology 45, 915–918. doi: 10.1130/G39113.1
Gvirtzman Z., Reshef M., Buch-Leviatan O., Ben-Avraham Z. (2013). Intense salt deformation in the Levant basin in the middle of the messinian salinity crisis. Earth Planetary Sci. Lett. 379, pp.108–pp.119. doi: 10.1016/j.epsl.2013.07.018
Gvirtzman Z., Reshef M., Buch-Leviatan O., Groves-Gidney G., Karcz Z., Makovsky Y., et al. (2015). Bathymetry of the Levant basin: interaction of salt-tectonics and surficial mass movements. Mar. Geol. 360, 25–39. doi: 10.1016/j.margeo.2014.12.001
Hover V. C. (1999). Mg-smectite authigenesis in a marine evaporative environment, Salina ometepec, Baja California. Clays Clay Miner. 47, 252–268. doi: 10.1346/CCMN.1999.0470302
Hover V. C., Walter L. M., Peacor D. R. (2002). K Uptake by modern estuarine sediments during early marine diagenesis, Mississippi delta plain, Louisiana, U.S.A. J. Sediment. Res. 72, 775–792. doi: 10.1306/032502720775
Ijiri A., Iijima K., Tsunogai U., Ashi J., Inagaki F. (2018). Clay mineral suites in submarine mud volcanoes in the kumano forearc basin, nankai trough: constraints on the origin of mud volcano sediments. Geosciences 8, 220. doi: 10.3390/geosciences8060220
Jongsma D., Fortuin A. R., Huson W., Troelstra S. R., Klaver G. T., Peters J. M., et al. (1983). Discovery of an anoxic basin within the strabo trench, eastern Mediterranean. Nature 305, 795–797. doi: 10.1038/305795a0
Kanari M., Tibor G., Hall J. K., Ketter T., Lang G., Schattner U. (2020). Sediment transport mechanisms revealed by quantitative analyses of seafloor morphology: New evidence from multibeam bathymetry of the Israel exclusive economic zone. Mar. Pet. Geol. 114, 104224. doi: 10.1016/j.marpetgeo.2020.104224
La Cono V., Smedile F., Bortoluzzi G., Arcadi E., Maimone G., Messina E., et al. (2011). Unveiling microbial life in new deep-sea hypersaline lake thetis part I: Prokaryotes and environmental settings. Environ. Microbiol. 13, 2250–2268. doi: 10.1111/j.1462-2920.2011.02478.x
La Cono V., Bortoluzzi G., Messina E., La Spada G., Smedile F., Giuliano L., et al. (2019). The discovery of lake Hephaestus, the youngest athalassohaline deep-sea formation on earth. Sci. Rep. 9, 1–11. doi: 10.1038/s41598-018-38444-z
Lugli S., Gennari R., Gvirtzman Z., Manzi V., Roveri M., Schreiber B. C. (2013). Evidence of clastic evaporites in the canyons of the Levant basin (Israel): Implications for the messinian salinity crisis. J. Sediment. Res. 83, 942–954. doi: 10.2110/jsr.2013.72
Manzi V., Gennari R., Lugli S., Persico D., Roveri M., Gavrieli I., et al. (2021). Synchronous onset of the messinian salinity crisis and diachronous evaporite deposition: New evidences from the deep Eastern Mediterranean basin. Palaeogeogr. Palaeoclimatol. Palaeoecol. 584, 110685. doi: 10.1016/j.palaeo.2021.110685
McCaffrey M. A., Lazar B., Holland H. D. (1987). The evaporation path of seawater and the coprecipitation of br- and k+ with halite. SEPM J. Sediment. Res. 57, 928–938. doi: 10.1306/212F8CAB-2B24-11D7-8648000102C1865D
McCaffrey R., Myers A., Davey E., Morrison G., Bender M., Luedtke N., et al. (1980). The relation between pore water chemistry and benthic fluxes of nutrients and manganese in Narragansett bay, Rhode island. Limnol 25, 31–44. doi: 10.2307/2835636
McDuff R. E., Gieskes J. M. (1976). Calcium and magnesium profiles in DSDP interstitial waters: Diffusion or reaction? Earth Planet. Sci. Lett. 33, 1–10. doi: 10.1016/0012-821X(76)90151-5
Meilijson A., Hilgen F., Sepúlveda J., Steinberg J., Fairbank V., Flecker R., et al. (2019). Chronology with a pinch of salt: Integrated stratigraphy of messinian evaporites in the deep Eastern Mediterranean reveals long-lasting halite deposition during Atlantic connectivity. Earth Sci. Rev. 194, 374–398. doi: 10.1016/j.earscirev.2019.05.011
Merlino G., Barozzi A., Michoud G., Ngugi D. K., Daffonchio D. (2018). Microbial ecology of deep-sea hypersaline anoxic basins. FEMS Microbiol. Ecol. 94, 1–15. doi: 10.1093/femsec/fiy085
Nelson S. T. (2000). A simple, practical methodology for routine VSMOW/SLAP normalization of water samples analyzed by continuous flow methods. Rapid Commun. Mass Spectrom. 14, 1044–1046. doi: 10.1002/1097-0231(20000630)14:12<1044::AID-RCM987>3.0.CO;2-3
Nusslein B., Eckert W., Conrad R. (2003). Stable isotope biogeochemistry of methane formation in profundal sediments of lake kinneret (Israel). Limnol. Oceanogr. 48, 1439–1446. doi: 10.4319/lo.2003.48.4.1439
Oppo D., Evans S., Iacopini D., Kabir S. M. M., Maselli V., Jackson C. A.-L. (2021). Leaky salt: Pipe trails record the history of cross-evaporite fluid escape in the northern Levant basin, Eastern Mediterranean. Basin Res. 33, 1798–1819. doi: 10.1111/bre.12536
Pachiadaki M. G., Yakimov M. M., LaCono V., Leadbetter E., Edgcomb V. (2014). Unveiling microbial activities along the halocline of thetis, a deep-sea hypersaline anoxic basin. ISME J. 8, 2478–2489. doi: 10.1038/ismej.2014.100
Purkis S. J., Shernisky H., Swart P. K., Sharifi A., Oehlert A., Marchese F., et al. (2022). Discovery of the deep-sea NEOM brine pools in the gulf of aqaba, red Sea. Commun. Earth Environ. 3, 146. doi: 10.1038/s43247-022-00482-x
Reiche S., Hübscher C., Beitz M. (2014). Fault-controlled evaporite deformation in the Levant basin, Eastern Mediterranean. Mar. Geol. 354, 53–68. doi: 10.1016/j.margeo.2014.05.002
Reolid J., Bialik O. M., Puga-Bernabéu Á., Zilberman E., Cardenal J., Makovsky Y. (2022). Evolution of a Miocene canyon and its carbonate fill in the pre-evaporitic eastern Mediterranean. Facies 68, 6. doi: 10.1007/s10347-022-00644-5
Rigaudier T., Lécuyer C., Gardien V., Suc J.-P., Martineau F. (2011). The record of temperature, wind velocity and air humidity in the δD and δ18O of water inclusions in synthetic and messinian halites. Geochim. Cosmochim. Acta 75, 4637–4652. doi: 10.1016/j.gca.2011.05.034
Roveri M., Flecker R., Krijgsman W., Lofi J., Lugli S., Manzi V., et al. (2014). The messinian salinity crisis: past and future of a great challenge for marine sciences. Mar. Geology 352, 25–58. doi: 10.1016/j.margeo.2014.02.002
Rubin-Blum M., Antler G., Turchyn A. V., Tsadok R., Goodman-Tchernov B. N., Shemesh E., et al. (2014). Hydrocarbon-related microbial processes in the deep sediments of the Eastern Mediterranean levantine basin. FEMS Microbiol. Ecol 87, 780–796. doi: 10.1111/1574-6941.12264
Sass E., Ben-Yaakov S. (1977). The carbonate system in hypersaline solutions: dead sea brines. Mar. Chem. 5, 183–199. doi: 10.1016/0304-4203(77)90006-8
Schmidt M., Al-Farawati R., Botz R. (2015). “Geochemical classification of brine-filled red Sea deeps,” in The red Sea. Eds. Rasul N., Stewart I. (Berlin, Heidelberg: Springer), 219–233.
Sela-Adler M., Ronen Z., Herut B., Antler G., Vigderovich H., Eckert W., et al. (2017). Co-Existence of methanogenesis and sulfate reduction with common substrates in sulfate-rich estuarine sediments. Front. Microbiol. 8. doi: 10.3389/fmicb.2017.00766
Shalev N., Lazar B., Köbberich M., Halicz L., Gavrieli I. (2018). The chemical evolution of brine and mg-k-salts along the course of extreme evaporation of seawater – an experimental study. Geochim. Cosmochim. Acta 241, 164–179. doi: 10.1016/j.gca.2018.09.003
Shokes R. F., Trabant P. K., Presley B. J., Reid D. F. (1977). Anoxic, hypersaline basin in the northern gulf of Mexico. Science (80) 196, 1443–1446. doi: 10.1126/science.196.4297.1443
Sisma-Ventura G., Kress N., Silverman J., Gertner Y., Ozer T., Biton E., et al. (2021). Post-eastern Mediterranean transient oxygen decline in the deep waters of the southeast Mediterranean Sea supports weakening of ventilation rates. Front. Mar. Sci. 7. doi: 10.3389/fmars.2020.598686
Sisma-Ventura G., Yam R., Kress N., Shemesh A. (2016). Water column distribution of stable isotopes and carbonate properties in the south-eastern levantine basin (Eastern mediterranean): Vertical and temporal change. J. Mar. Syst. 158, 13–25. doi: 10.1016/j.jmarsys.2016.01.012
Stanley D. J., Sheng H. (1979). Trona in Nile cone late quaternary sediments: Probable redepositional origin. Mar. Geol 31, M21–M28. doi: 10.1016/0025-3227(79)90049-5
Steinle L., Knittel K., Felber N., Casalino C., De Lange G., Tessarolo C., et al. (2018). Life on the edge: Active microbial communities in the kryos MgCl2-brine basin at very low water activity. ISME J. 12, 1414–1426. doi: 10.1038/s41396-018-0107-z
Swallow J. C., Crease J. (1965). Hot salty water at the bottom of the red Sea. Nature 205, 165–166. doi: 10.1038/205165a0
Ozer T., Gertman I., Gildor H., Goldman R., Herut B. (2020). Evidence for recent thermohaline variability and processes in the deep water of the southeastern levantine basin, Mediterranean Sea. Deep. Res. Part II Top. Stud. Oceanogr. 171, 104651. doi: 10.1016/j.dsr2.2019.104651
Tayber Z., Meilijson A., Ben-Avraham Z., Makovsky Y. (2019). Methane hydrate stability and potential resource in the Levant basin, southeastern Mediterranean Sea. Geosciences 9, 306. doi: 10.3390/geosciences9070306
Van Der Wielen P. W. J. J., Bolhuis H., Borin S., Daffonchio D., Corselli C., Giuliano L., et al. (2005). The enigma of prokaryotic life in deep hypersaline anoxic basins. Sci. (80-. ). 307, 121–123. doi: 10.1126/science.1103569
Vengosh A., De Lange G. J., Starinsky A. (1998). Boron isotope and geochemical evidence for the origin of urania and bannock brines at the eastern Mediterranean: Effect of water-rock interactions. Geochim. Cosmochim. Acta 62, 3221–3228. doi: 10.1016/S0016-7037(98)00236-1
Vengosh A., Gieskes J., Mahn C. (2000). New evidence for the origin of hypersaline pore fluids in the Mediterranean basin. Chem. Geol. 163, 287–298. doi: 10.1016/S0009-2541(99)00131-X
Vengosh A., Starinsky A. (1993). Relics of evaporated sea water in deep basins of the Eastern Mediterranean. Mar. Geol. 115, 15–19. doi: 10.1016/0025-3227(93)90071-3
Vengosh A., Starinsky A., Anati D. A. (1994). The origin of Mediterranean interstitial waters-relics of ancient Miocene brines: A re-evaluation. Earth Planet. Sci. Lett. 121, 613–627. doi: 10.1016/0012-821X(94)90095-7
Wallmann K., Linke P., Suess E., Bohrmann G., Sahling H., Schlüter M., et al. (1997a). Quantifying fluid flow, solute mixing, and biogeochemical turnover at cold vents of the eastern Aleutian subduction zone. Geochim. Cosmochim. Acta 61, 5209–5219. doi: 10.1016/S0016-7037(97)00306-2
Wallmann K., Suess E., Westbrook G. H., Winckler G., Cita M. B. (1997b). Salty brines on the Mediterranean sea floor. Nature 387, 31–32. doi: 10.1038/387031a0
Keywords: Mediterranean Sea, brine pools, chemical composition, deep-sea, seawater, evaporites, Levant Basin
Citation: Herut B, Rubin-Blum M, Sisma-Ventura G, Jacobson Y, Bialik OM, Ozer T, Lawal MA, Giladi A, Kanari M, Antler G and Makovsky Y (2022) Discovery and chemical composition of the eastmost deep-sea anoxic brine pools in the Eastern Mediterranean Sea. Front. Mar. Sci. 9:1040681. doi: 10.3389/fmars.2022.1040681
Received: 09 September 2022; Accepted: 01 November 2022;
Published: 17 November 2022.
Edited by:
Paolo Favali, ERIC foundation, ItalyReviewed by:
Jacek Raddatz, Goethe University Frankfurt, GermanyAndré Antunes, Macau University of Science and Technology, China
Copyright © 2022 Herut, Rubin-Blum, Sisma-Ventura, Jacobson, Bialik, Ozer, Lawal, Giladi, Kanari, Antler and Makovsky. This is an open-access article distributed under the terms of the Creative Commons Attribution License (CC BY). The use, distribution or reproduction in other forums is permitted, provided the original author(s) and the copyright owner(s) are credited and that the original publication in this journal is cited, in accordance with accepted academic practice. No use, distribution or reproduction is permitted which does not comply with these terms.
*Correspondence: Barak Herut, YmFyYWtAb2NlYW4ub3JnLmls; Maxim Rubin-Blum, bXJ1YmluQG9jZWFuLm9yZy5pbA==; Yizhaq Makovsky, eWl6aGFxQHVuaXYuaGFpZmEuYWMuaWw=