- 1Halmos College of Arts and Sciences, Nova Southeastern University, Dania Beach, FL, United States
- 2Earth and Life Institute, UCLouvain, Louvain-la-Neuve, Belgium
- 3Institute of Mechanics, Materials and Civil Engineering, UCLouvain, Louvain-la-Neuve, Belgium
Since the 1980s, populations of Acropora cervicornis and A. palmata have experienced severe declines due to disease and anthropogenic stressors; resulting in their listing as threatened, and their need for restoration. In this study, larval survival and competency data were collected and used to calibrate a very high-resolution hydrodynamic model (up to 100m) to determine the dispersal patterns of Acropora species along the Florida’s Coral Reef. The resulting connectivity matrices was incorporated into a metapopulation model to compare strategies for restoring Acropora populations. This study found that Florida’s Coral Reef was historically a well-connected system, and that spatially selective restoration may be able to stimulate natural recovery. Acropora larvae are predominantly transported northward along the Florida’s Coral Reef, however southward transport also occurs, driven by tides and baroclinic eddies. Local retention and self-recruitment processes were strong for a broadcast spawner with a long pelagic larval duration. Model simulations demonstrate that it is beneficial to spread restoration effort across more reefs, rather than focusing on a few reefs. Differences in population patchiness between the Acropora cervicornis and A. palmata drive the need for different approaches to their management plans. This model can be used as a tool to address the species-specific management to restore genotypically diverse Acropora populations on the Florida’s Coral Reef, and its methods could be expanded to other vulnerable populations.
Introduction
Despite their ecological importance and economic value, coral reefs are experiencing a severe decline worldwide (Wilkinson, 2000; Bruno and Selig, 2007; Hoegh-Guldberg, 2011; Jackson et al., 2014; Hughes et al., 2017; Hughes et al., 2018). Ocean warming, pollution, and overfishing are the main contributors to the demise of adult corals. These negative impacts extend to their reproduction and early life stages, from fertility to fertilization, larval development, settlement, and post-settlement survival and growth (Hunte and Wittenberg, 1992; Randall and Szmant, 2009; Albright et al., 2010; Hoey et al., 2016; Fourney and Figueiredo, 2017). This curtailing of recruitment limits the ability for populations to replenish themselves and amplifies the cycle of decline (Williams and Miller, 2012).
Coral sexual reproduction increases genotypic diversity and promotes reef connectivity. New genotypes can facilitate adaptation through environmental changes, and thus can potentially boost the resilience of the system (Baums et al., 2006; Drury and Lirman, 2017). Additionally, the larvae produced via sexual reproduction are planktonic and can disperse across long distances, connecting populations, and potentially recolonizing disturbed areas and/or establishing new populations. Larvae remain in the water column until they become competent, i.e., able to search the benthos for a suitable substrate for settlement and undergo metamorphosis into a polyp (Richmond and Hunter, 1990). The suitability of the substrate is determined by positive and negative environmental cues, such as the presence or absence of crustose coralline algae, bacterial biofilms, macroalgae, light, and reef sounds (Munday and Babcock, 1998; Kuffner et al., 2006; Gleason et al., 2009; Ritson-Williams et al., 2009; Vermeij et al., 2010). The length of time larvae can survive and maintain competency are important determinants of local retention (proportion of larvae released by a reef that settled back on that reef), self-recruitment (proportion of settlers on a reef that originated from larvae produced on that reef), and a species’ range, as competency directly influences how far from the natal reef settlement can occur (Richmond and Hunter, 1990; Figueiredo et al., 2013). Hence, sexual reproductive output, larval biology, and larval ecology are influential on the broad scale connectivity of reefs, their recovery, and genetic diversity (Treml et al., 2012).
The limited ability of corals to recover naturally from severe declines in some regions, such as the Caribbean, the Persian Gulf, or the Red Sea, has prompted the need for active coral restoration (Rinkevich, 2008). Typically, this is conducted by growing coral fragments at in situ nurseries, and after grow-out, outplanting them on a reef (Epstein et al., 2001). While this asexual way of propagating corals has been shown to significantly increase local coral cover (Young et al., 2012; Miller et al., 2016), it is ineffective at a large scale. Outplanting is time-consuming and requires ample labor, equating to high costs for minimal returns at reef-system scales (Young et al., 2012). Furthermore, site selection for restoration is, in the best cases, based on ease of access, the availability of hard substrate, low predator and competitor abundance, water quality, light levels, and the presence of adult and juvenile colonies (Edwards, 2010; Johnson et al., 2011); a site’s potential larval contribution to downstream reefs is not considered (Edwards, 2010). The lack of consideration of larval dispersal and connectivity patterns diminishes the potential wide scale influence that restoration efforts could provide beyond the restored site. Understanding species’ connectivity patterns is vital so that reefs which act as strong larval sources can be identified, not only to protect them, but also to restore, so they may contribute to the recovery of surrounding unrestored reefs.
To estimate larval dispersal and population connectivity, early studies often relied on additive diffusion modeling and passive particle models based on mean currents but were unable to account for realistic ocean conditions (Cowen and Sponaugle, 2009). Now, high resolution hydrodynamic models can account for small scale circulation, allowing for more realistic simulations of dispersal (Wood et al., 2014; Thomas et al., 2015; Mayorga-Adame et al., 2017). Further, when these high-resolution oceanographic models are combined with biological data, such as larval survival, time to competency, loss of competency, and swimming abilities (Cowen et al., 2006; Metaxas and Saunders, 2009; Mayorga-Adame et al., 2017; Lequeux et al., 2018), they allow for seascape wide views and can be used to simulate the processes of dispersal and provide estimates on demographic connectivity and the factors controlling it (Cowen and Sponaugle, 2009; Wood et al., 2014; Drury et al., 2018; Limer et al., 2020). The length of time many larvae can survive and maintain competency is still unknown, particularly for Caribbean corals, because it is difficult to keep healthy larval cultures in laboratory for a long period of time, the duration of the study is too short to accurately estimate long-term survival, or larvae are provided with continuous settlement cues (Graham et al., 2008; Connolly and Baird, 2010).
To date, few biophysical models of dispersal of corals have been used on Florida’s Coral Reef, which could be essential for assisting severely diminished populations (Drury et al., 2018; Frys et al., 2020). Acropora cervicornis and A. palmata have experienced severe populations declines since the 1980s due to disease and anthropogenic stressors (Gladfelter, 1982; Aronson and Precht, 2001), eliciting their listings as threatened in 2006 under the United States Endangered Species Act and the need for restoration (Hogarth, 2006). For these important reef builders (Jackson, 1994), current restoration efforts on Florida’s Coral Reef primarily focus on increasing coral cover at outplant sites. In this study, larval survival and competency data were collected and used to calibrate a very high-resolution hydrodynamic model (up to 100m) to determine potential connectivity and dispersal patterns Acropora species along Florida’s Coral Reef. The resulting connectivity matrix was incorporated into the development of a metapopulation model to compare strategies for re-establishing spatially connected populations. Ultimately, we hope our model will be used as a tool to address the species-specific management needs by incorporating more ecological considerations into restoration plans, and thereby coupling natural recovery processes with active human effort to restore genotypically diverse Acropora populations on Florida’s Coral Reef.
Methodology
Long term survival
Acropora cervicornis and A. palmata are hermaphroditic broadcast spawners, typically releasing their egg and sperm bundles a few days after the full moon of August (Richmond and Hunter, 1990). Both species have similar survival and time to competency (Miller et al., 2020; Ritson-Williams et al., 2020), thus due to the inability of collecting larval survival and competency data from A. palmata, the data from A. cervicornis was used for both species. Gametes of A. cervicornis released on the night of August 11th, 2017, at the Coral Restoration Foundation Nursery in Tavernier, Florida was used to assess long-term survival and competency. The resulting embryos used for the experiment were derived from sixteen different genotypes from all regions of Florida’s Coral Reef (Figure 1). Gametes were combined with a 99% fertilization success rate. Eight hours post fertilization, 50 embryos were randomly assigned to sixteen 100mL jars with fresh sterile seawater (FSW) and no settlement cues. The jars were placed in water baths at 29°C, the average summer seawater temperature in South Florida. Each day, a 100% water change was performed on the jar, and the total number of surviving larvae was counted.
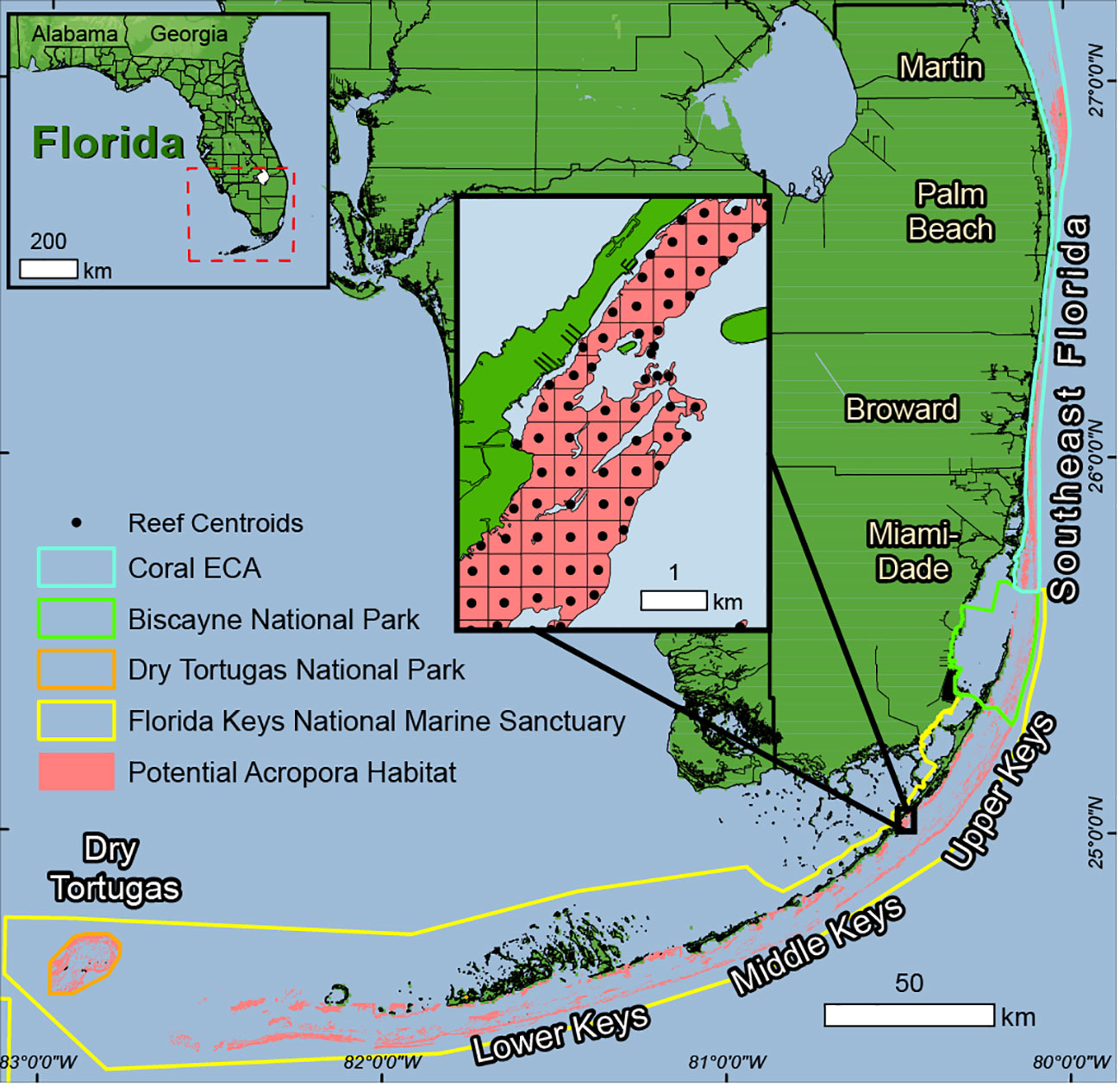
Figure 1 Map of Acropora habitat on Florida’s Coral Reef used from Wirt et al., 2015 in pink with the 500m grid and centroids to subdivide the habitat into relevant management units. Each grid square with its centroid is a reef, of which there 10277 in the dataset. The locations of the five regions of the reef system are labelled, with the Dry Tortugas the most west, followed by the Lower Keys, Middle Keys, Upper Keys, and Southeast Florida the most North.
Long term competency
The planktonic, lecithotrophic planula of Acropora typically become competent 6-8 days after spawning (Miller et al., 2020). To measure the long-term competency dynamics, larvae were reared in five 2L plastic bowls at a concentration of<1 larva mL-1 seawater in the absence of settlement cues. The bowls were kept in water baths at 29°C. Every day, larvae were washed with FSW and an 100% water change was performed. On days 4-10 post fertilization, and then once a week for two weeks (days 17 and 24), 80 larvae were randomly removed from the cultures and distributed between four 100mL glass jars with FSW and a pre-conditioned tile. The tiles were conditioned in situ at the NSU Layer Cakes Nursery in ~7m depth, offshore Fort Lauderdale, FL, for approximately two months prior to the experiment to allow for the development natural settlement cues such as crustose coralline algae and microbial biofilm. After 24 h of exposure to the settlement cue, the number of larvae that had settled and metamorphosed was counted to estimate the proportion of competent larvae each day.
Survival and competency modeling
To determine the probability that a larva is alive and competent at a given time, as is needed to inform the biophysical dispersal model, the empirically collected data on long term survival and competency were modeled using the methods described by Connolly and Baird (2010) and extended by Figueiredo et al. (2013) and Figueiredo et al. (2014). Briefly, survival and competency were determined separately, with several models tested and compared for best fit based on Akaike information crierion (AIC). The parameters of a given model were estimated using maximum log likelihood (see the Appendix in the Supplementary Material for a complete description of models and parameterization). While initially calculated separately, the final two models of best fit for mortality and competency can be multiplied to find the probability of a larva being alive and competent, such that if t ≥ tc,
Biophysical dispersal model
The model used in this study was the Second-generation Louvain-la-Neuve Ice-ocean Model (SLIM) for the years 2016 and 2017 (Dobbelaere et al., 2020; Frys et al., 2020; Dobbelaere et al., 2022). SLIM solves the ocean circulation governing equations on an unstructured mesh with the discontinuous finite element method. The mesh resolution can be varied in space to accurately reproduce the coastal and reef topography. In coastal areas where small scale features dominate, a very high resolution of up to 100m was used, but a coarse mesh (down to 15km resolution) was used in offshore areas with more uniform flow and large-scale processes (Frys et al., 2020). The high resolution reflects small scale circulation and retention processes, such as recirculation eddies that form around a reef, thereby greatly increasing the accuracy of the dispersal models, as coarse resolution around reefs can vastly overestimate connectivity and underestimate local retention (Cowen et al., 2000). The 95% Acropora Habitat Map (Wirt et al., 2015) was subdivided into a 500m grid to create reefs sites for use by management bodies. This resulted in 10,277 Acropora reef polygons (maximum 500m × 500m) to assess potential connectivity of the system of Florida’s Coral Reef (Figure 1).
The methods used for the larval dispersal simulation were expanded from Frys et al. (2020) to include more details of larval biology and ecology. The simulation began by a continuous seeding of reef polygons over peak spawning nights of each year, August 22-24, 2016, and August 10-12, 2017 from 10pm until midnight. The model ran for two months. If, as determined by the biological parameters, live competent “larvae” passed over reef habitat they were assumed to settle on a reef at a rate of 20% per hour. For each year the model was run, the resulting connectivity matrix records, for each reef, the total mass of particles it sent that settled on a reef. These values were normalized as a proportion by dividing each value by the mass of particles released by a reef to produce the two connectivity matrices used for analyses (CM 2016, CM 2017), as well as a mean of the two years (CM Mean).
Dispersal metrics
The following dispersal metrics for each reef were calculated for each connectivity matrix (CM 2016, CM 2017, CM Mean):
The source metric (Ki) is a measure of the capacity of a reef to replenish other reefs, i.e., spill-over:
Where is the number of reefs to which reef i sends larvae to, and is the total proportion of larvae it sends to those reefs. Reefs with a higher source metric provide greater value to the entire reef system.
The sink index (Si) is a measure of a reef’s ability to be replenished by others, thus it is estimated based on the input connections:
Where is the number of reefs that reef i receives larvae from, and is the total proportion of larvae it receives.
Local retention is a measure of the capacity of a reef to self-replenish, thus it is the proportion of larvae released from a reef that settle back on that reef:
Where Cii are the proportion of larvae from reef i that settled on reef i.
Self-recruitment is the proportion of settlers on a reef which originated from larvae released on that reef, i.e., a measure of isolation (as described in Burgess et al., 2014):
Where Cii are the proportion of larvae from reef i that settled on reef i and is the total proportion of larvae seeded on reef i that settles on reef j.
The mean and standard deviation for each metric were calculated for each reef in the three connectivity matrices, as well as the mean and median proportion of larvae that settled each year and by each of the five regions of Florida’s Coral Reef (Figure 1). These regions are well established in the literature based on the geologic (Lidz and Shinn, 1991), hydrographic (Lee et al., 1994; Lee et al., 1995), and ecological divisions (Walker, 2012; Walker and Gilliam, 2013), and are used for strata for large scale surveys of the system. To determine the distance larvae travelled before settling, the geographical distance between reefs was calculated using the function distGeo in R. The dispersal metrics for each reef were compared between the two years using a paired t-test. ANOVA tests were used to compare the dispersal metrics between the five regions of Florida’s Coral Reef using each connectivity matrix (CM 2016, CM 2017, CM Mean).
Using the connectivity metrics from CM Mean the following analyses were performed. Spatial autocorrelation was tested using a Moran’s I analysis with inverse distance weighting to determine any spatial biases in the site locations. Kernel density layers were created to illustrate the magnitude-per-unit area of the metrics. Hots Spot Analysis spatial statistics tool in ArcGIS was used to analyze spatial clustering of the connectivity metrics. This tool uses a Getis-Ord Gi* statistic to identify spatial clusters of high and low values by calculating a z-score and p-value of live coral cover for each plot (Getis and Ord, 1992). The z-scores and p-values determined whether spatial clustering of high and low live metric values was different than those expected in a random distribution. High z-scores (> 2) and low p-values (< 0.05) indicated a spatial clustering of high values for the tested metric. Z-scores near zero indicated no apparent spatial clustering. Survey sites were then coded by their Gi Z score for each year to indicate spatiotemporal patterns of clustering in the map.
Metapopulation model
A metapopulation model was created where coral cover of a reef over discrete time (t, years) was described as:
Where ci, t is the coral cover (area) on patch i at time t and ci, t-1 is the coral cover on patch i the year prior. R is the growth rate of a patch (accounting for budding/asexual growth and mortality), A is the area of a patch, sis the probability of a recruit to survive and mature, a is the mean area of a polyp, j is the number of reefs in the study, fis the average fecundity of a polyp (eggs produced), and Ci,j is the connectivity between patch j and i (larvae transported from patch j to patch i).
Thus, for any given year, the coral cover is dependent on the coral cover from the previous year, the growth of the patch, and recruitment success of the year prior. The first term of the equation refers to the growth of a reef that occurred during year t. Growth is density-dependent, i.e., at lower densities there will be a higher growth (lower mortality and quicker budding). This is due to low intraspecific competition for space, whereas at higher densities there is a lower growth (higher mortality, slower budding) due to increased competition for space. The second term represents new recruitment to the reef. The number of successful recruits is dependent upon the number of polyps on a reef that survived from the year prior (as seen by one minus the first term), polyp fecundity, and the ability of the larvae to reach and survive on reef i, as stipulated from the connectivity matrices. Finally, for settlers to be recruited into the population and preempt space, their post-settlement survival is considered, as well as how much area is available on the reef, based on the free space from the current cover and the size of the polyp recruit. Of all these potential recruits, the following factors determine if recruits will increase a reef’s coral cover in addition to its annual growth: quantity of live, competent larvae that pass over the reef, the availability of free space on the reef, and recruit survival during the first year.
Model parameters were gathered from previously published studies and the connectivity matrices estimated in this study. The initial coral cover A. cervicornis and A. palmata were set to 0.018 and 0.008, respectively, as this was the mean live cover density of the species at sites across the Florida Keys from 1999-2017 (Miller et al., 2013). These values are under half the mean cover reported by Vargas-Ángel et al., 2003 in 1998 (5%), and thus seem to accurately reflect the recent losses experienced on this reef system. Area of a reef (A) was the size of the polygon, which was a maximum of 2.5×105 m2. Surface area of a polyp (a) was estimated by dividing the surface area of five fragments of adult A. cervicornis from both Broward County and the Florida Keys National Marine Sanctuary by its number of polyps; the mean value of these measurements, 8.158×10-8m2, was used as estimate for a. Acroporids are known to have very similar life history traits (Szmant, 1986; Ritson-Williams et al., 2010; Miller et al., 2020), thus we used the life history parameters of A. cervicornis for both species. Since Acropora are simultaneous hermaphrodites, fertilization should not be sperm-limited, therefore a polyp’s fecundity was estimated at 21 eggs per polyp, the mean value measured in previous fecundity studies (Soong, 1991). The post-settlement survival success of a recruit (s) was assumed to be 3% based on long term survival of coral recruits in the field (Chamberland et al., 2015). Growth rate (R, encompassing both budding and mortality) was estimated as the mean of the increases in percent live cover of two long term monitored A. cervicornis patches, 0.014 m2y-1 (Goergen et al., 2019). Fecundity (f), post-settlement survival (s) and growth rate (R) were assumed to be constant, as there was no reasonable way to estimate how these would vary spatially and temporally.
All modeling was done in R version 4.0.2.
Restoration simulations
To test methods of restoration optimization, simulations can be performed using the metapopulation model to compare the effect that different restoration and outplanting scenarios would have on the total cover of a species on Florida’s Coral Reef. Since the connectivity matrices generated by the biophysical dispersal model (CM 2016, CM 2017, CM Mean) represented the potential connectivity between reefs of Acropora habitat, these matrices represented all the areas which could potentially harbor the species. We then created a second version of these matrices to use in the metapopulation model to represent connectivity of the current demography of each species. Such that, only reefs in which the species is present can send larvae to other reefs, but all reefs which are considered habitat for the species can receive larvae.
To do this, the reef sites used in the biophysical dispersal model with current coral presence needed to be identified. Presence data on A. cervicornis and A. palmata from the years 2012-2017 were pooled from various monitoring surveys: Florida Reef Resilience Program (e.g. Stein and Ruzicka, 2021), Southeast Florida Coral Reef Evaluation and Monitoring (Gilliam et al., 2021), and NOAA (Miller et al., 2013). This time frame included the years of the hydrodynamic models, and several years prior to account for differences in monitoring frequencies between surveys. ARCGIS Pro’s Intersect function was used map the sites identified with species presence onto the matching habitat polygons of the biophysical dispersal model. All sites that did not match presence data were changed to seed zero reefs in a new connectivity matrix for each species (CM Acer Mean; CM Apal Mean). Since habitat reefs could be seeded, and to account for asexual growth on reefs that reaches reproductive maturity, polyps generated by the model at any site can contribute larvae (“spawn”) in the model after they are four years old (Chamberland et al., 2016). Thus, after four years of the simulation, the reefs that were barren at t-3 and had received larvae, would then use the connectivity data from CM Mean to then be able to “spawn” and see other reefs.
Below, we provide a few examples of how a metapopulation model could be used to aid restoration management decisions. For instance, to compare between sites more useful to restore (i.e., contribute to a greater increase of the overall coral cover on the system) or how to spatially vary outplant efforts. Note that while these comparisons between different restoration/outplanting scenarios assume no disturbance (reductions of coral cover or “wipes out” reefs in a certain area or across Florida’s Coral Reef), it is possible to force reductions in cover in the model to simulate this.
Site to site comparison
The metapopulation model was used to compare where outplanting would be most effective. For instance, if funding was limited, to compare between multiple environmentally favorable sites and select the site that would provide the best contribution to higher cover of the entire reef system. As an example, we ran 5 different simulations of the site-to-site scenario for each species, one for each region of Florida’s Coral Reef.
In the simulation, we randomly selected three reefs with no existing cover to be “outplanted” using the sample function in R. To simulate outplanting, the starting coral cover of the reef was changed from 0 to 100 m2. To account for outplanting effects, the area was reduced by 23% in year two of the simulation to simulate outplant mortality (Goergen and Gilliam, 2018), and during year one of the simulation, the outplants did not “spawn” to account for outplant stress. The simulation ran for 15 years and recorded the cover (area in m2) for each reef, each year of the simulation. The same reefs were used in the simulations between species, to observe if there were any species-specific differences. A one-way Repeated Measures ANOVA was used to compare percent cover at t=15 between the scenarios (outplanting at each reef, no restoration = 4 levels), to see if outplanting a reef could statistically improve the cover of Florida’s Coral Reef, and which would do it the best.
Spatial scaling comparison
The metapopulation model was also used to test optimal spatial scaling of restoration; specifically, if outplanting less across more reefs (20m2, n=20, total= 400 m2) or outplanting more at fewer reefs (80m2, n=5, total= 400 m2), is more effective. We ran simulations for each region of Florida’s Coral Reef (Figure 1) with both species, to see if the optimal outplanting strategy varied between regions for each species.
In a simulation, twenty reefs without coral cover were randomly selected from within a region using the function sample in R for the “many” scenarios, and of these, five reefs were randomly selected for the “few” scenario. As before, the same reefs were used between species, to observe if there were any species-specific differences. The same assumptions of outplanting effects on survival and spawning were used as in described in the site-to-site simulations, and the simulations ran for t=15 years, with cover (area in m2) being recorded at each time point. A paired t-test was used to compare percent cover at t=15 years between the scenarios (many vs few) to determine which provided significantly greater coral cover on Florida’s Coral Reef.
Results
Survival and competency
The survival data was best fit with a Weibull model, such that the mortality rate was initially high, but decreased over time (Appendix, Equation 2). Larvae began acquiring competency at day 5 (tc=5.12 days) and acquired competency at a constant rate (a = 0.063 day-1). Loss of competency was modelled equally well by the exponential and Weibull models. Therefore, we used the Weibull model (Appendix, Equation 8) because it had was the best choice to model loss of competency for Acropora millepora that used a longer data collection period (Figueiredo et al., 2022). Thus, the per capita rate of loss of competency was not constant over time (η=60.36698), with the rate of loss being initially high, and decreasing over time. As a result, the proportion of larvae that are alive and competent is null the first 5 days (tc=5.12 days), and then it increases, peaking around day 20, and then rapidly decreasing over time until around day 40, when it then slowly decreases (Figure 2).
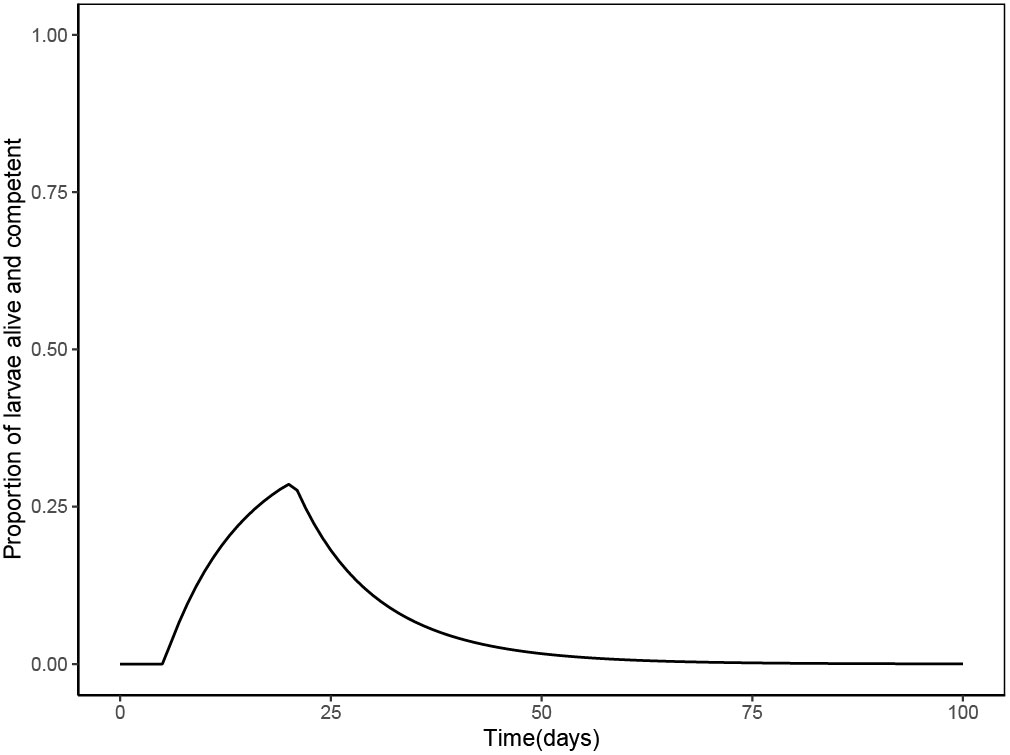
Figure 2 The proportion of larvae that are alive and competent over time (since spawning) based on the Weibull model for mortality rate and Weibull model for loss of competency. Larvae begin acquiring competency at 5 days (tc=5.12), the number of live, competent larvae peaks at about day 20, and then rapidly decreases over time, until about day 40, where it then slowly decreases.
Dispersal metrics
Annual variability
In both years connectivity was high, with less than 0.4% of reefs experiencing no settlement. On average, 15.4% of the larvae released by reefs settled in 2016 and 18.3% in 2017, with each reef seeding on average 13.8% of the reefs in 2016 and 13.7% in 2017. The standard deviation of the connections was 0 for 80.4%, with only 0.3% of connections having a standard deviation greater than 0.001, indicative of minimal variance between reefs each year.
There was not a significant difference in the source metrics between years, but the distance larvae travelled before settling was significantly less in 2016 than in 2017 (Paired t-test, p<2.2×10-16) (Appendix Figure S1). Thus, localized interactions were stronger in 2016 when reefs had significantly higher sink, local retention, and self-recruitment metrics than in 2017 (Paired t-test; p= 2.756×10-4, p=1.648×10-9, p= 1.192×10-13, respectively).
Spatial analysis
Spatial autocorrelation was not significant (p=0.726) indicating that the location of the reef points did not bias the cluster analysis. The Getis-Ord spatial clustering indicates locations of high and low values based on the potential connectivity from CM Mean produced by the biophysical dispersal model that are statistically different from an expected random distribution. Localized hot spots for each metric were distributed throughout much of the reef system (Figure 3).
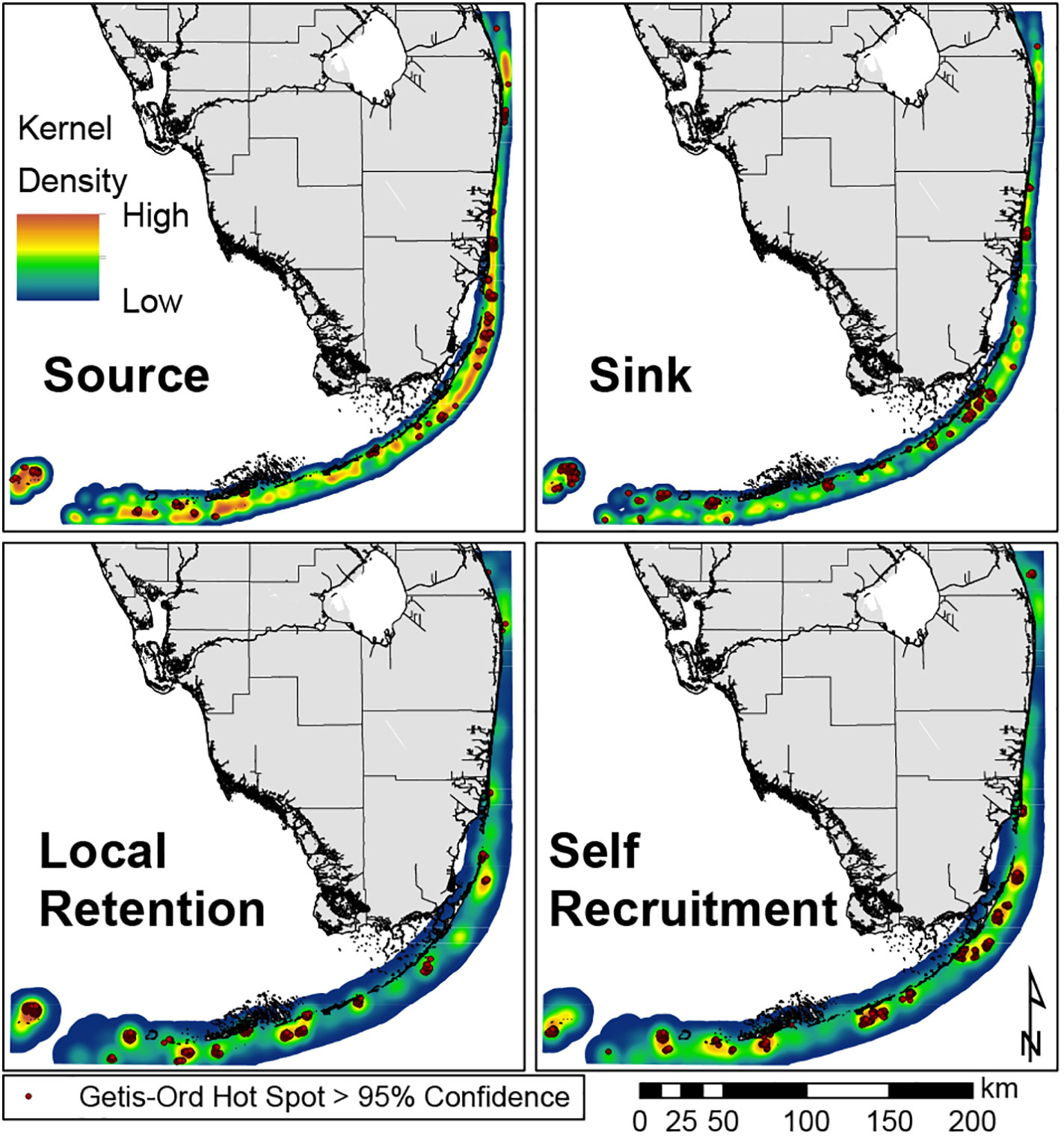
Figure 3 Getis-Ord Hot Spot analysis with >95% confidence overlying a Kernel Density interpolation for each connectivity metric (Source, Sink, Local Retention, and Self Recruitment). Warmer colors denote higher metric values, while cooler colors denote lower metric values. Hot Spots for each metric (dots) are well distributed throughout the reef system, indicating a well-connected system. Source clusters indicate better reefs for outplanting, as they will be good for replenishing downstream sites, whereas sink clusters indicate better reefs for measures to improve settlement probability. Sink clusters reefs could indicate better reefs to obtain nursery genotypes from, as these sites should have higher genetic diversity from their connections from other sites. High self-recruitment clusters indicate better reefs for greater conservation efforts due to stronger isolation. These may be good sources for genotypes with certain local adaptations. Local retention clusters indicate reefs better at replenishing themselves, so these may have reduced natural recovery after a disturbance.
Regional variability
In 2016, there were significant differences between regions of Florida’s Coral Reef in their capacities to function as sinks, retain larvae, and the distance larvae dispersed (One-way ANOVA, p=6.515×10-4; Kruskal-Wallis, p=0.034; one way ANOVA, p=8.577×10-10, respectively). Thus, only the ability of reefs to act as sources did not vary significantly between regions. The Dry Tortugas and Middle Keys were significantly better sinks than in the Southeast; all the other regions were not significantly different from each other. The Local Retention in the Middle Keys was signficantly greater than in the Upper Keys; all the other regions were not significantly different from each other. The distance larvae settled from their natal reef was greatest for larvae from the Dry Tortugas, and not significantly different among the other regions.
In 2017, there was greater similarity in the connectivity metrics between regions, with the exception of distance larvae travelled before settling (Kruskal-Wallis, p<2.2×10-16). Larvae from Dry Tortugas travel longer distances, while larvae from the Upper and Middle Keys dispersed the shortest distances.
When comparing metrics between regions and years, larvae travelled further in 2017 than in 2016, particularly for larvae from the Dry Tortugas (Appendix Figure S2). On average, in 2017, reefs in the Dry Tortugas received 8.77% less larvae from the other regions, while the Lower Keys only received 0.41% less, and the Middle Keys 2.81% less, while and the Upper Keys and Southeast received 5.46% and 5.41% more respectively (Figure 4).
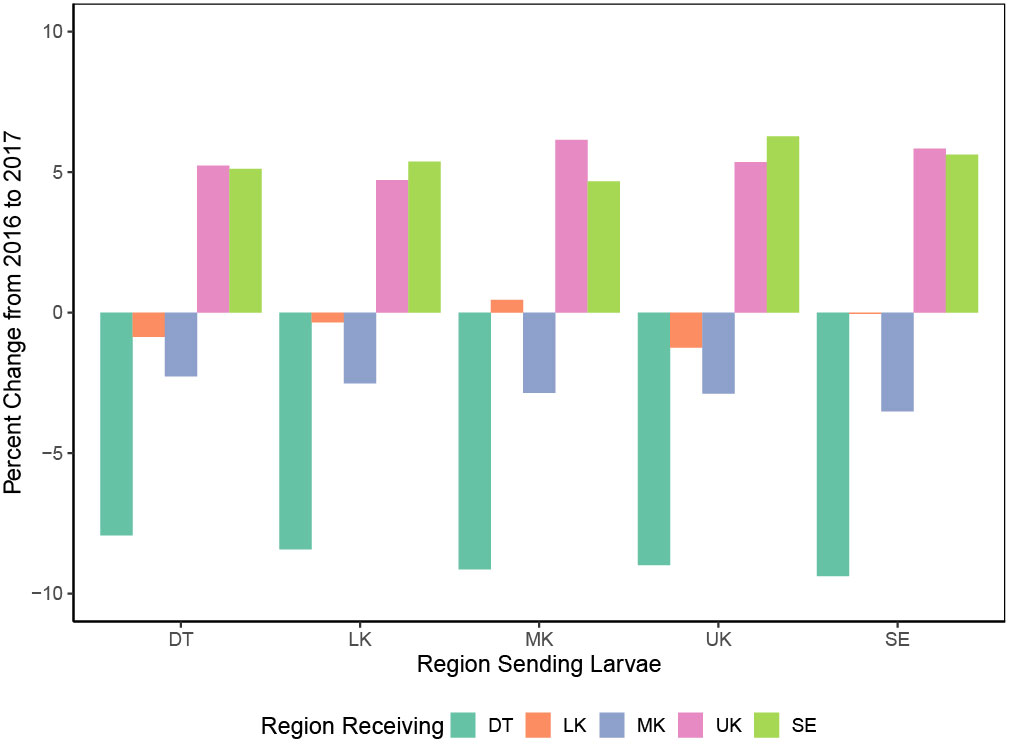
Figure 4 The percent change in the proportion of larvae exchanged between each region from 2016 to 2017. Each region of Florida’s Coral Reef is denoted by a different color: Dry Tortugas (DT) teal; Lower Keys (LK) in orange; Middle Keys (MK) blue; Upper Keys (UK) pink; Southeast (SE) lime green. DT, LK, and MK all received a smaller proportion of larvae in 2017, while UK and SE received a greater proportion of larvae in 2017 than in 2016.
When using CM Mean, there was significant difference between the region’s reefs in their local retention metrics and the distance they sent larvae to (p=0.032, p<2.2×10-16). The Middle Keys had significantly higher local retention then the Upper Keys; local retention was not significantly different among the other regions. Larvae travelled significantly further from the Dry Tortugas, then the Lower Keys and Southeast, while the Middle Keys and Upper Keys sent larvae the shortest distance.
Restoration simulations
These simulations were used as examples of the applications metapopulation models in the support of management decisions, and to test if there were differences between regions.
Site to Site comparison
In each region, there was no significant difference between outplanting on one reef vs. another reef (p>0.05). For both species, in the Lower Keys, Middle Keys, and Upper Keys simulations, the reef with the highest source index provided the best outcome (i.e., highest mean percent cover of reefs in the system). For the Dry Tortugas, the reef with the middle value source index provided the best outcome. In the Southeast, the reef with the lowest source index provided the best outcome for A. palmata, but the reef with the highest did for A. cervicornis (Appendix Table S1).
Spatial scaling comparison
For both species, regardless of region, spreading the restoration effort across many reefs was equally as good (not significantly different p > 0.05) or significantly better (p<0.05) than concentrating effort on a small number of reefs (Appendix Table S2). Specifically, for A. palmata, it was better to spread the outplant effort in 6 of the 25 simulations, while for A. cervicornis, it was better in 16 out 25 simulations.
Discussion
This study found that there is high potential for system-wide larval exchange between all regions of the Florida’s Coral Reef, indicating that historically, it was a well-connected system, and that spatially selective restoration may stimulate natural recovery. Acropora larvae are predominantly transported northward along Florida’s Coral Reef, however southward transport also occurs, driven by tides and baroclinic eddies. The potential for local retention and self-recruitment processes is higher than expected for a broadcast spawner with a long pelagic larval duration (Figueiredo et al., 2013). Model simulations suggest that it is beneficial to spread restoration effort across more reefs, rather than focusing more heavily on a few reefs. Finally, differences in population patchiness between the two Acropora species drive the need for different approaches to their management plans.
The larval dispersal model indicated the potential for high connectivity across the entirety of Florida’s Coral Reef, with minimal differentiation between regions of the system. Larvae are predominantly transported northward, some for very long distances, but some are also dispersed southward. The long-distanced connections across all the regions are likely a result of the life history traits of Acropora larvae which have a lengthy pre-competency period and a strong ability to maintain competency, furthering their ability to disperse (Miller et al., 2020; Figueiredo et al., 2022). The connectivity patterns revealed by the biophysical dispersal model generally reflected greater exchange than anticipated, particularly southward, opposite to the direction of the major Florida currents, although there have been recent findings of periodic southward currents (Soloviev et al., 2017). Southwards larval transport is primarily driven by eddies (Limouzy-Paris et al., 1997; Sponaugle et al., 2005; D’Alessandro et al., 2007), and typically shorter-distanced; however, the model notably predicts that larvae from the Southeast region can be transported as far as the Dry Tortugas. Previously, it was hypothesized that the high connectivity between the regions was driven solely by southern reefs seeding northern ones, however our findings suggest the opposite is also true and southward transport also contributes to the homogenization of the population. The five different regions send larvae fairly equally between regions, with each having a similar ability to act as sources, and each region containing equal distributions of very strong sources (Figure 3). The high degree of regional exchange found corroborates the findings of the genetic relatedness and continuity of Florida Acropora populations (Baums et al., 2010; Hemond and Vollmer, 2010; Drury et al., 2017; Drury et al., 2018; Drury et al., 2019). Overall, connectivity is vital for the replenishment of disturbed reefs, where even a seemingly negligible supply of larvae can lead to rapid recovery in the absence of chronic anthropogenic stressors (Gilmour et al., 2013).
Despite the general trend of system continuity, a few areas show potential for differentiation, due to having higher levels of self-recruitment (isolation), receiving less larvae, and/or lower connections to other regions in some years. Our model suggests that the Southeast region (i.e., Kristen Jacobs Coral Reef Ecosystem Conservation Area and Biscayne National Park) receives less larvae than the other regions of Florida’s Coral Reef, while the Middle Keys is more heavily reliant on self-recruitment, both being processes that could lead to genetically distinct populations. Genetic studies seem to reflect this trend, as Broward, the Lower Keys, and the Middle Keys populations of Acropora cervicornis have been found to be genetically distinct from the rest of Florida’s Coral reefs (Drury et al., 2017). This genetic differentiation may continue to grow as low to nonexistent recruitment continues (van Woesik et al., 2014). We also found inconsistency in flow between the Dry Tortugas and the rest of Florida’s Coral Reef. For instance, there was a greater proportion of larvae sent to the Upper Keys and Southeast in 2017, likely due to larvae from the Dry Tortugas being lost into the Gulf of Mexico in 2016, rather than transported by the Gulfstream along Florida’s Coral Reef in 2017. This could lead to relatedness between the Dry Tortugas and other regions of the Gulf of Mexico and Eastern Caribbean. For instance, in Montastraea cavernosa, the Dry Tortugas population is highly similar genetically to the mesophotic populations of Belize (Studvian and Voss, 2018) but seems to still maintain similarity to other Florida reefs (Serrano et al., 2014). However, recent genetic studies seem to show that, despite inconsistent, the existent pulses of connectivity, may be sufficient to build relatedness the Dry Tortugas and the other Florida’s Acropora populations (Drury et al., 2018). It is also possible that the inter-regions homogenization in Florida is driven, at least partially, by seeding from nearby regions, such as Cuba, Flower Gardens or Bahamas and the wider Caribbean (Schill et al., 2015) to all of Florida’s Coral Reef regions, which our study could not include, but could be considered in the future if the hydrodynamic data was available.
The biophysical model of larval dispersal for Acropora captured reefs with high levels of local retention and self-recruitment across all regions of Florida’s Coral Reef. The majority of reefs (96%) retained at least some of their larvae, but we found some reefs that retained or self-recruited a considerable amount of larvae (top 1% retained 5.5-100% or self-recruited 8.5- 99.9%). These high levels are unusual for species with a long larval development such as a Acropora (Figueiredo et al., 2013), which are known to disperse great distances due to long periods of pre-competency and retention of competency (Moneghetti et al., 2019; Figueiredo et al., 2022). These areas of the reef track likely have small-scale circulation dynamics that can trap larvae around their natal reef, such as recirculation eddies driven by the flow interactions with the topography. Such processes were able to be captured due to the high-resolution of the hydrodynamic model, which also prevented overestimation of long-distance dispersal. High levels of local retention indicate that reefs have the capacity to replenish themselves and reduces the risk of loss of larvae from the system or recruitment failure due to phenotype-environment mismatches (Marshall et al., 2010; Weese et al., 2010). It also suggests that reefs will be responsive to local management actions, i.e., protection of an adult coral population on a reef should be reflected in higher recruitment on that reef (high stock-recruitment relationship). Similarly, due to their dispersal ability, one would expect less isolation in Acropora, however, Suzuki et al. (2011) also found indications of high self-recruitment in Pacific Acropora, indicating that once competency is acquired, if the larvae have not been dispersed by currents, they will settle on their natal reef. Self-recruitment is a measure of isolation, where most of the recruits on a reef originated from larvae released on that reef, and very few from other reefs were received. Isolated reefs, such as many reefs in the Middle Keys, are more vulnerable to disturbances, as they are less likely to be rescued/replenished with larvae from other reefs. Reduced input from other reefs increases the likelihood of local adaptation (Strathmann et al., 2002) but may reduce their capacity to cope with environmental changes (Baums et al., 2006).
Determining the location of sources and sinks in the system is essential for the successful management of species, as it allows managers to prioritize the protection and/or restoration of reefs. Reefs with a high source index, such as Newfound Harbor, should be considered for restoration and protection as their larvae reach a greater number of reefs, making them extremely valuable for the recovery following disturbances and persistence of the reef network. Reefs with higher sink indexes such as Carysfort North are important to consider for protection due their ability to harbor greater genetic diversity but are not particularly useful to actively restore. Additionally, as depositories of genetic diversity, sinks have network-persistence, which may qualify them as good reefs to collect donor fragments for nurseries or outplanting. Finally, reefs with relatively high source and sink indexes, as is the case with Eastern Dry Rocks, may constitute important stepping stones for multigenerational connectivity (Frys et al., 2020), as they receive larvae from a large number of reefs (becoming highly diverse), and the larvae they produce are exported to large number of reefs, making them important to protect and to consider for restoration. Reefs in the Upper Keys, such as Carysfort, generally have a lower sink and local retention value, making them more vulnerable to disturbances, although some of these also have higher sink indexes, so if connectivity is restored, they could be replenished from upstream sources. Reefs in the Southeast generally have a somewhat lower sink index, and average levels of local retention, self-recruitment, and source index relative to the other regions, suggesting that they are less connected and more vulnerable to disturbance, but could act as an important exporter of larvae, especially since it has some of the highest cover of A. cervicornis in the system (Walker et al., 2012; Walker, 2017). The Dry Tortugas has a high sink index, but not significantly higher self-recruitment or local retention, it is likely that there is strong intra-regional connectivity with neighboring reefs seeding each other rather than themselves.
The metapopulation model developed revealed that, if present environmental conditions are maintained or improved, restoration can be effective when accounting for connectivity patterns. Metapopulation models incorporating reef connectivity through larval dispersal, but also demographic parameters such as asexual growth, settlement rates, post-settlement mortality, and fecundity, are an essential tool to manage reefs. These models allow us to compare reefs to determine which would be more advantageous to restore, i.e., that would provide a greater contribution for the recovery of all reefs, and to determine how to spatially and/or temporally organize the outplanting effort. Our site-to-site simulations showed that even outplanting a single reef, given successful spawning and settlement, could improve the overall cover of the whole reef system. This result suggests that, for both species, strategic outplanting and larval seeding could help improve recent trends of low-to-nonexistent recruitment of Acropora on Florida reefs (van Woesik et al., 2014). Our site-to-site simulations also suggest that to increase cover of Florida’s Coral Reef it is more valuable to restore sites that replenish a greater number of impoverished reefs, not necessarily the site which seeds the greatest number of reefs (highest source index). The ability to replenish a greater number of impoverished reefs (i.e., those with a strong rescue effect (Brown and Kodric-Brown, 1977), will partly be related to the reef’s source index, but is also dependent on the population size of the reefs it seeds. Since most of the habitat sites on Florida’s Coral Reef, currently have little to no Acropora, it means that the best outplanting choice generally was also the reef with greatest rescue effect.
Spreading the restoration effort across more sites, instead of concentrating the same effort on just one or a few reefs had a similar or significantly greater contribution the coral cover in the Florida Coral Reef. While in practice conducting restoration in multiple reefs could prove more laborious and therefore expensive, it could also reduce the risk of a disturbance destroying all restored reefs. To date, these results have not been validated in the field, as research in spatial scaling thus far has only investigated outplanting density and spatial planning within a site, not amongst sites (Griffin et al., 2015; Goergen et al, 2019). Additionally, few restoration studies incorporate ecological processes, such as recruitment and spawning following outplanting (Ladd et al., 2018). Considering how depauperate the populations have become; it is vital to address questions regarding methodologies for reestablishing spatially connected populations in a manner that promotes natural recovery and genotypic diversity (Lirman and Schopmeyer, 2016). Ecological modeling can provide good suggestions to design experimental trials to answer these questions.
Despite their very similar life history traits, there are distinct differences in the current population distribution of Acropora cervicornis and Acropora palmata in Florida that require differing management plans. The preferred habitat and larval dynamics of Acropora species are very similar and thus they have the same potential connectivity. However, the current greater population patchiness and mean cover on Florida’s Coral Reef of A. palmata means it requires a greater restoration effort (i.e., a greater number of colonies of diverse genotypes need to be outplanted), and more consideration should be used in spatial and temporal planning outplanting, which can be strategized with a metapopulation model. Currently, restoration projects in Florida focus majorly on A. cervicornis (Miller et al., 2016), despite the declines in both species. The model was able to detect preferential outplanting strategies for A. cervicornis, however the highly depauperate condition of A. palmata populations along Florida’s Coral Reef made any restoration simulation largely improve its cover on the reef system, regardless of scenario. Additionally, in the site-to-site simulation the outplanted reef that resulted in the highest increase of the mean reef cover was not the same for both species in the Southeast, indicating a stronger degree of rescue effect not captured by the source index. The differences in the success of outplanting strategies implies that the two species need separate management plans with different considerations for effective restoration. Further understanding of the differences driving the recovery of these species will improve the likelihood of their persistence.
While we found Florida’s Coral Reef to have good potential connectivity, a promising sign for their ability to recover, we cannot forget that historically, there have been very few observations of Acropora recruits (Williams et al., 2008; Harper et al., 2021– note: van Woesik et al., 2014 paper incorrectly identifies Acropora recruits; picture is an octocoral recruit). This apparent contradiction could be explained by two different constraints. First, the model assumes reefs will be able to produce embryos, however, distance between colonies of different genotypes limit that possibility of successful fertilization and embryo formation on the reef system. Existent genetic information indicates that thickets of Acropora in Florida are often monoclonal (Neigel and Avise, 1983; Baums et al., 2006), with patches and colonies often far from each other, reducing the chances eggs and sperm of different colonies to ever meet. Restoring reefs helps to resolve this, by increasing coral abundance with outplants from diverse genetic pools (Baums et al., 2019) and contributes to the reduction of distances between genotypes. Second the contradiction could come from the fact that, coral recruitment is currently limited by the suitability of substrate for coral settlement and recruits’ survival and growth. It is possible that while some fertilization is occurring, and embryos are developing into competent larvae, the latter never find a suitable place to settle. Or, if they do, the present conditions, e.g., high macroalgal cover and sedimentation, prevent the newly settled corals to survive and grow to a size detectable by surveyors. Increasing coral cover will improve settlement cues, and substrate condition could be improved by reductions of dredging and coastal construction that cause sedimentation and reduction of nutrient run-off, overfishing of herbivores which contribute to high macroalgae cover. Such actions are essential to boost the success of natural processes of coral recovery, sexual reproduction, and recruitment.
The connectivity matrix generated in this study encapsulates all Acropora habitat, including sites from which corals are currently absent. Thus, while some areas have been identified as potentially being strong sources of larvae and thus technically ideal candidates for restoration, in reality these sites may no longer be practical or environmentally suitable for corals. Hence, this connectivity matrix can be used to identify restoration sites which are potentially better sources, but then their suitability needs to be validated in the field, i.e., determine if the species is present at the site, and its health (absence of disease), absence predators, and other local threats. These identified sites could be used for outplanting colonies, as we demonstrated here, but with slight modifications other strategies, such as seeding with competent larvae could be used as well.
The resulting connectivity matrix should be validated using genetic studies, and any discrepancies be used to improve the existent models. Nevertheless, it is important to recall that most coral genetic studies (e.g., microsatellite analysis that only identify shared haplotypes) have only identified historical exchange of larvae between sites and may not accurately reflect the current demographic connectivity, which is more useful for management decisions. Some newer genetic techniques such as assignment tests can be used to identify demographic connectivity (Casado-Amezùa et al., 2012), but due to the temporal and spatial scale of the sampling they would require to capture broad connectivity, they are difficult to apply to coral population in large reef systems such as the Florida’s Coral Reef. Future studies could provide improved parameters on differences in survival and growth between the species to improve the metapopulation model and ascertain the best strategy for each species.
Finally, for restoration to be successful in the long-term, carbon emissions need to be curbed (Duarte et al., 2020). As oceans warm, local scale refugia will be lost (Dixon et al., 2022) causing reefs to experience mass bleaching annually within the next twenty years (Hoegh-Guldberg et al., 2017). This could result in a range of impacts to the ecosystem, not least of which includes high mortality of corals (Eakin et al., 2019), and latent impacts like reduced the energy for surviving corals to allocate to growth (McClanahan et al., 2009; Cantin et al., 2010; Muller et al., 2018) and reproduction (Ward et al., 2002; Jones and Berkelmans, 2011). Ocean warming also reduces larval survival and competency, limiting dispersal and distanced connections between reefs, which would further decrease their ability to recover following disturbances (Figueiredo et al., 2014; Figueiredo et al., 2022). However, by incorporating considerations of sexual reproduction, the chances these species will persist as climate changes, by allowing for increased genetic diversity and opportunities for adaptation and transgenerational acclimation.
In this study we developed a high-resolution biophysical model of larval dispersal to estimate the connectivity of Acropora on Florida’s Coral Reef, and a metapopulation model to improve restoration that incorporated concepts of demographic connectivity. Utilizing high resolution bio-physical dispersal models, empirically calibrated with larval survival and competency dynamics, ensures local processes are captured and the degree of distanced connections and the degree of openness of system are not overestimated (Cowen et al., 2000). Such considerations are particularly important in Acropora which have longer pre-competency periods than most corals and have the capacity to maintain competency for extensive periods of time (Figueiredo et al., 2022). These models, fulfill a vital need for coral reef management and restoration. Incorporating considerations of sexual reproduction and connectivity into the decision-making process facilitates the maintenance of the genetic diversity of Floridian Acropora populations and the successful re-establishment of the population, provided chronic anthropogenic stressors are lessened.
Data availability statement
The datasets presented in this study can be found in online repositories. The names of the repository/repositories and accession number(s) can be found below: https://doi.org/10.5061/dryad.0zpc8671f.
Author contributions
JF, EH, and SK contributed to the conception and design of the study. AS-A and EH ran the dispersal model and generated the connectivity matrices. SK conducted larval experiments, generated the metapopulation models and performed statistical analyses. BW created the reef layer grid, performed spatial analyses, and generated figures. SK wrote the first draft of the manuscript. JF wrote sections of the manuscript. All authors contributed to the article and approved the submitted version.
Funding
This manuscript is the result of research funded by National Oceanic and Atmospheric Association (NOAA) Coral Reef Conservation Program (CRCP) award number NA17NOS4820081.
Acknowledgments
This project was supported by National Oceanic and Atmospheric Association (NOAA) Coral Reef Conservation Program (CRCP) award number NA17NOS4820081. Computational resources were provided by the Consortium des Eíquipements de Calcul Intensif (CEíCI), funded by the F.R.S.-FNRS under Grant No. 2.5020.11. We also thank the Florida Aquarium and the Coral Restoration Foundation for allowing us to partner with them for Acropora cervicornis spawning.
Conflict of interest
The authors declare that the research was conducted in the absence of any commercial or financial relationships that could be construed as a potential conflict of interest.
Publisher’s note
All claims expressed in this article are solely those of the authors and do not necessarily represent those of their affiliated organizations, or those of the publisher, the editors and the reviewers. Any product that may be evaluated in this article, or claim that may be made by its manufacturer, is not guaranteed or endorsed by the publisher.
Supplementary material
The Supplementary Material for this article can be found online at: https://www.frontiersin.org/articles/10.3389/fmars.2022.1038463/full#supplementary-material
References
Albright R., Mason B., Miller M., Langdon C. (2010). Ocean acidification compromises recruitment success of the threatened Caribbean coral acropora palmata. Proc. Natl. Acad. Sci. 107 (47), 20400–20404. doi: 10.1073/pnas.1007273107
Aronson R. B., Precht W. F. (2001). “White-band disease and the changing face of Caribbean coral reefs,” in The ecology and etiology of newly emerging marine diseases. developments in hydrobiology, vol. 159 . Ed. Porter J. W. (Springer: Dordrecht). doi: 10.1007/978-94-017-3284-0_2
Babcock R. C., Munday C. N. (1998). Role of light intensity and spectral quality in coral settlement: Implications for depth-dependent settlement? J. Exp. Mar. Biol. Ecol. 223 (7), 235–255. doi: 10.1016/S0022-0981(97)00167-6
Baums I. B., Baker A. C., Davies S. W., Grottoli A. G., Kenkel C. D., Kitchen S. A., et al. (2019). Considerations for maximizing the adaptive potential of restored coral populations in the western Atlantic. Ecol. Appl. 29 (8), e01978. doi: 10.1002/eap.1978
Baums I. B., Johnson M. E., Devlin-Durante M. K., Miller M. W. (2010). Host population genetic structure and zooxanthellae diversity of two reef-building coral species along the Florida reef tract and wider Caribbean. Coral reefs 29 (4), 835–842. doi: 10.1007/s00338-010-0645-y
Baums I. B., Miller M. W., Hellberg M. E. (2006). Geographic variation in clonal structure in a reef building Caribbean coral, acropora palmata. Ecol. Monogr. 76 (4), 503–519. doi: 10.1890/0012-9615(2006)076[0503:GVICSI]2.0.CO;2
Brown J. H., Kodric-Brown A. (1977). Turnover rates in insular biogeography: effect of immigration on extinction. Ecology 58, 445–449. doi: 10.2307/1935620
Bruno J. F., Selig E. R. (2007). Regional decline of coral cover in the indo-pacific: Timing, extent, and subregional comparisons. PloS One 2 (8), e711. doi: 10.1371/journal.pone.0000711
Burgess S. C., Nickols K. J., Griesemer C. D., Barnett L. A. K., Dedrick A. G., Satterthwaite E. V., et al. (2014). Beyond connectivity: How empirical methods can quantify population persistence to improve marine protected-area design. Ecol. Appl. 24 (2), 257–270. doi: 10.1890/13-0710.1
Cantin N. E., Cohen A. L., Karnauskas K. B., Tarrant A. M., McCorkle D. C. (2010). Ocean warming slows coral growth in the central red Sea. Science 329 (5989), 322–325. doi: 10.1126/science.1190182
Casado-Amezùa P., Goffredo S., Templado J., Machordom A. (2012). Genetic assessment of population structure and connectivity in the threatened Mediterranean coral astroides calycularis (Scleractinia, dendrophylliidae) at different spatial scales. Mol. Ecol. 21 (15), 3671–3685. doi: 10.1111/j.1365-294X.2012.05655.x
Chamberland V. F., Petersen D., Latijnhouwers K. R. W., Snowden S., Mueller B., Vermeij M. J. A. (2016). Four-year-old Caribbean acropora colonies reared from field-collected gametes are sexually mature. Bull. Mar. Sci. 92 (2), 263–264. doi: 10.5343/bms.2015.1074
Chamberland V. F., Vermeij M. J. A., Brittsan M., Carl M., Schick M., Snowden S., et al. (2015). Restoration of critically endangered elkhorn coral (Acropora palmata) populations using larvae reared from wild-caught gametes. Global Ecol. Conserv. 4, 526–537. doi: 10.1016/j.gecco.2015.10.005
Connolly S. R., Baird A. H. (2010). Estimating dispersal potential for marine larvae: Dynamic models applied to scleractinian corals. Ecol. Soc. America 91 (12), 3572–3583. doi: 10.1890/10-0143.1
Cowen R. K., Lwiza K. M., Sponaugle S., Paris C. B., Olson D. B. (2000). Connectivity of marine populations: Open or closed? Science 287 (5454), 857–859. doi: 10.1126/science.287.5454.85
Cowen R. K., Paris C. B., Srinivasan A. (2006). Scaling of connectivity in marine populations. Science 311 (5760), 522–527. doi: 10.1126/science.1122039
Cowen R. K., Sponaugle S. (2009). Larval dispersal and marine population connectivity. Annu. Rev. Mar. Sci. 1 (1), 443–466. doi: 10.1146/annurev.marine.010908.163757
D’Alessandro E., Sponaugle S., Lee T. (2007). Patterns and processes of larval fish supply to the coral reefs of the upper Florida keys. Mar. Ecol. Prog. Ser. 331, 85–100. doi: 10.3354/meps331085
Dixon A. M., Forster P. M., Heron S. F., Stoner A. M. K., Beger M. (2022). Future loss of local-scale thermal refugia in coral reef ecosystems. PloS Climate 1 (2), e0000004. doi: 10.1371/journal.pclm.0000004
Dobbelaere T., Holstein D. M., Muller E., Gramer L. G., McEachron L., Williams S. D., et al. (2022). Connecting the dots: Transmission of stony coral tissue loss disease from the marquesas to the dry tortugas. Front. Mar. Sci. 80. doi: 10.3389/fmars.2022.778938
Dobbelaere T., Muller E. M., Gramer L. J., Holstein D. M., Hanert E. (2020). Coupled epidemio-hydrodynamic modeling to understand the spread of a deadly coral disease in Florida. Front. Mar. Sci. 7, 591881. doi: 10.3389/fmars.2020.591881
Drury C., Greer J. B., Baums I., Gintert B., Lirman D. (2019). Clonal diversity impacts coral cover in acropora cervicornis thickets: Potential relationships between density, growth, and polymorphisms. Ecol. Evol. 9 (8), 4518–4531. doi: 10.1002/ece3.5035
Drury C., Lirman D. (2017). Making biodiversity work for coral reef restoration. Biodiversity 18 (1), 23–25. doi: 10.1080/14888386.2017.1318094
Drury C., Paris C. B., Kourafalou V. H., Lirman D. (2018). Dispersal capacity and genetic relatedness in acropora cervicornis on the Florida reef tract. Coral Reefs 37 (2), 585–596. doi: 10.1007/s00338-018-1683-0
Drury C., Schopmeyer S., Goergen E., Bartels E., Nedimyer K., Johnson M., et al. (2017). Genomic patterns in acropora cervicornis show extensive population structure and variable genetic diversity. Ecol. Evol. 7 (16), 6188–6200. doi: 10.1002/ece3.3184
Duarte C. M., Agusti S., Barbier E., Britten G. L., Castilla J. C., Gattuso J.-P., et al. (2020). Rebuilding marine life. Nature 580 (7801), 39–51. doi: 10.1038/s41586-020-2146-7
Eakin C. M., Sweatman H., Brainard R. E. (2019). The 2014–2017 global-scale coral bleaching event: Insights and impacts. Coral Reefs 38 (4), 539–545. doi: 10.1007/s00338-019-01844-2
Edwards A. J. (2010). “Coral reef targeted research & capacity building for management program,” in Reef rehabilitation manual (St. Lucia, Australia: The Coral Reef Targeted Research & Capacity Building for Management Program), 166.
Epstein N., Bak R. P., Rinkevich B. (2001). Strategies for gardening denuded coral reef areas: The applicability of using different types of coral material for reef restoration. Restor. Ecol. 9 (4), 432–442. doi: 10.1046/j.1526-100X.2001.94012.x
Figueiredo J., Baird A. H., Connolly S. R. (2013). Synthesizing larval confidence dynamics and reef scale retention reveals a high potential for self-recruitment in corals. Ecology 94 (3), 650–659. doi: 10.1890/12-0767.1
Figueiredo J., Baird A. H., Harii S., Connolly S. R. (2014). Increased local retention of reef coral larvae as a result of ocean warming. Nat. Climate Change 4 (6), 498–502. doi: 10.1038/nclimate2210
Figueiredo J., Thomas C. J., Deleersnijder E., Lambrechts J., Baird A. H., Connolly S. R., et al. (2022). Global warming decreases connectivity among coral populations. Nat. Climate Change 12 (1), 83–87. doi: 10.1038/s41558-021-01248-7
Fourney F., Figueiredo J. (2017). Additive negative effects of anthropogenic sedimentation and warming on the survival of coral recruits. Sci. Rep. 7 (1), 1–8. doi: 10.1038/s41598-017-12607-w
Frys C., Saint-Amand A., Le Henaff M., Figueiredo J., Kuba A., Walker B., et al. (2020). Fine-scale coral connectivity pathways in the Florida reef tract: Implications for conservation and restoration. Front. Mar. Sci. 7. doi: 10.3389/fmars.2020.00312
Getis A., Ord J. K. (1992). The analysis of spatial association by use of distance statistics. Geogr. Anal. 24, 189–206. doi: 10.1111/j.1538-4632.1992.tb00261.x
Gilliam D. S., Hayes N. K., Ruzicka R., Colella M. (2021). Southeast Florida coral reef evaluation and monitoring project 2020 year 18 final report (Miami Beach, FL: Florida DEP & FWC), 82.
Gilmour J., Smith L., Heyward A., Baird A., Pratchett M. (2013). Recovery of an isolated coral reef system following severe disturbance. Science 340 (69), 69–71. doi: 10.1126/science.1232310
Gladfelter E. H. (1982). White-band disease in acropora palmata: Implications for the structure and growth of shallow reefs. Bull. Mar. Sci. 32 (2), 639–643(5).
Gleason D. F., Danilowiez B. S., Nolan C. J. (2009). Reef waters stimulate substratum exploration in planulae from brooding Caribbean corals. Coral Reefs 28, 549–554. doi: 10.1007/s00338-009-0480-1
Goergen E. A., Moulding A. L., Walker B. K., Gilliam D. S. (2019). Identifying causes of temporal changes in acropora cervicornis populations and the potential for recovery. Front. Mar. Sci. 36. doi: 10.3389/fmars.2019.00036
Goergen E. A., Gilliam D. S. (2018). Outplanting technique, host genotype, and site affect the initial success of outplanted Acropora cervicornis. PeerJ 6, e4433. doi: 10.7717/peerj.4433
Graham E. M., Baird A. H., Connolly S. R. (2008). Survival dynamics of scleractinian coral larvae and implications for dispersal. Coral reefs 27 (3), 529–539. doi: 10.1007/s00338-008-0361-z
Griffin J. N., Schrack E. C., Lewis K. A., Baums I. B., Soomdat N., Silliman B. R. (2015). Density-dependent effects on initial growth of a branching coral under restoration. Restor. Ecol. 23 (3), 197–200. doi: 10.1111/rec.12173
Harper L. M., Huebner L. K., O’Cain E. D., Ruzicka R., Gleason D. F., Fogarty N. D. (2021). Methodological recommendations for assessing scleractinian and octocoral recruitment to settlement tiles. PeerJ 9, e12549. doi: 10.7717/peerj.12549
Hemond E. M., Vollmer S. V. (2010). Genetic diversity and connectivity in the threatened staghorn coral (Acropora cervicornis) in Florida. PloS One 5 (1), e865. doi: 10.1371/journal.pone.0008652
Hoegh-Guldberg O. (2011). Coral reef ecosystems and anthropogenic climate change. Regional Environ. Change 11 (1), 215–227. doi: 10.1007/s10113-010-0189-2
Hoegh-Guldberg O., Poloczanska E. S., Skirving W., Dove S. (2017). Coral reef ecosystems under climate change and ocean acidification. Front. Mar. Sci. 4, 158. doi: 10.3389/fmars.2017.00158
Hoey A. S., Howells E., Johansen J. L., Hobbs J. P. A., Messmer V., McCowan D. M., et al. (2016). Recent advances in understanding the effects of climate change on coral reefs. Diversity 8 (2), 12. doi: 10.3390/d8020012
Hogarth W. T. (2006). Endangered and threatened species: Final listing determinations for elkhorn coral and staghorn coral. Federal Register 71 (89), 26852–26861.
Hughes T. P., Anderson K. D., Connolly S. R., Heron S. F., Kerry J. T., Lough J. M., et al. (2018). Spatial and temporal patterns of mass bleaching of corals in the anthropocene. Science 359 (6371), 80–83. doi: 10.1126/science.aan8048
Hughes T. P., Kerry J. T., Álvarez-Noriega M., Álvarez-Romero J. G., Anderson K. D., Baird A. H., et al. (2017). Global warming and recurrent mass bleaching of corals. Nature 543 (7645), 373–377. doi: 10.1038/nature21707
Hunte W., Wittenberg M. (1992). Effects of eutrophication and sedimentation on juvenile corals. Mar. Biol. 112 (1), 131–138. doi: 10.1007/BF00357259
Jackson J. B. C. (1994). Community unity. Science 264(5164) 1412–1413. doi: 10.1126/science.264.5164.1412
Jackson J. B. C., Donovan M. K., Cramer K. L., Lam V. V. (2014). Status and trends of Caribbean coral reefs (Gland, Switzerland: Global Coral Reef Monitoring Network, IUCN).
Johnson M. E., Lustic C., Bartels E. (2011). Caribbean Acropora restoration guide: best practices for propagation and population enhancement (Arlington, VA: The Nature Conservancy).
Jones A. M., Berkelmans R. (2011). Tradeoffs to thermal acclimation: Energetics and reproduction of a reef coral with heat tolerant symbiodinium type-d. J. Mar. Biol. 2011, 12. doi: 10.1155/2011/185890
Kuffner I. B., Walters L. J., Becerro M. A., Paul V. J., Ritson-Williams R., Beach K. S. (2006). Inhibition of coral recruitment by macroalgae and cyanobacteria. Mar. Ecol. Prog. Ser. 323, 107–117. doi: 10.3354/meps323107
Ladd M. C., Miller M. W., Hunt J. H., Sharp W. C., Burkepile D. E. (2018). Harnessing ecological processes to facilitate coral restoration. Front. Ecol. Environ. 16 (4), 239–247. doi: 10.1002/fee.1792
Lee T. N., Clarke M. E., Williams E., Szmant A. F., Berger T. (1994). Evolution of the tortugas gyre and its influence on recruitment in the Florida keys. Bull. Mar. Sci. 54 (3), 621–646.
Lee T. N., Leaman K., Williams E., Berger T., Atkinson L. (1995). Florida Current meanders and gyre formation in the southern straits of Florida. J. Geophys. Res: Oceans 100 (C5), 8607–8620. doi: 10.1029/94jc02795
Lequeux B. D., Ahumada-Sempoal M. A., López-Pérez A., Reyes-Hernández C. (2018). Coral connectivity between equatorial eastern pacific marine protected areas: A biophysical modeling approach. PloS One 13 (8), e0202995. doi: 10.1371/journal.pone.0202995
Lidz B. H., Shinn E. A. (1991). Paleoshorelines, reefs, and a rising sea: South Florida, USA. J Coastal Res, 203–229.
Limer B. D., Bloomberg J., Holstein D. M. (2020). The influence of eddies on coral larval retention in the flower garden banks. Front. Mar. Sci. 7. doi: 10.3389/fmars.2020.00372
Limouzy-Paris C. B., Graber H. C., Jones D. L., Roüpke A. W., Richards W. J. (1997). Translocation of larval coral reef fishes via sub-mesoscale spin-off eddies from the Florida current. Bull. Mar. Sci. 60, 966–983.
Lirman D., Schopmeyer S. (2016). Ecological solutions to reef degradation: Optimizing coral reef restoration in the Caribbean and Western Atlantic. PeerJ 4, e2597. doi: 10.7717/peerj.2597
Marshall D. J., Monro K., Bode M., Keough M. J., Swearer S. (2010). Phenotype–environment mismatches reduce connectivity in the sea. Ecol. Lett. 13 (1), 128–140. doi: 10.1111/j.1461-0248.2009.01408.x
Mayorga-Adame C. G., Batchelder H. P., Spitz Y. H. (2017). Modeling larval connectivity of coral reef organisms in the Kenya-Tanzania region. Front. Mar. Sci. 4, 92. doi: 10.3389/fmars.2017.00092
McClanahan T. R., Weil E., Maina J. (2009). Strong relationship between coral bleaching and growth anomalies in massive porites. Global Change Biol. 15 (7), 1804–1816. doi: 10.1111/j.1365-2486.2008.01799.x
Metaxas A., Saunders M. (2009). Quantifying the “bio-” components in biophysical models of larval transport in marine benthic invertebrates: Advances and pitfalls. Biol. Bull. 216 (3), 257–272. doi: 10.1086/BBLv216n3p257
Miller M. W., Bright A. J., Pausch R. E., Williams D. E. (2020). Larval longevity and competency patterns of Caribbean reef-building corals. PeerJ 8, e9705. doi: 10.7717/peerj.9705
Miller S. L., Chiappone M., Rutten L. M. (2013). Distribution, abundance, and condition of acropora corals, other benthic coral reef organisms, and marine debris in Biscayne national park and the Florida keys national marine sanctuary: 2012 quick look and data summary report Vol. 237 (Dania Beach, FL: National Coral Reef Institute, Nova Southeastern University Oceanographic Center).
Miller M. W., Kerr K., Williams D. E. (2016). Reef scale trends in Florida acropora spp. abundance and the effects of population enhancement. Peer J. 4, e2523. doi: 10.7717/peerj.2523
Moneghetti J., Figueiredo J., Baird A. H., Connolly S. R. (2019). High-frequency sampling and piecewise models reshape dispersal kernels of a common reef coral. Ecology 100 (8), e02730. doi: 10.1002/ecy.2730
Muller E. M., Bartels E., Baums I. B. (2018). Bleaching causes loss of disease resistance within the threatened coral species acropora cervicornis. Elife 7, e35066. doi: 10.7554/eLife.35066.028
Neigel J. E., Avise J. C. (1983). Clonal diversity and population structure in a reef-building coral, acropora cervicornis: Self-recognition analysis and demographic interpretation. Evolution 37 (3), 437–453. doi: 10.2307/2408259
Randall C. J., Szmant A. M. (2009). Elevated temperature affects development, survivorship, and settlement of the elkhorn coral, Acropora palmata (Lamarck 1816). The Biological Bulletin 217 (3), 269–282.
Richmond R. H., Hunter C. L. (1990). Reproduction and recruitment of corals: comparisons among the Caribbean, the tropical pacific, and the red Sea. Mar. Ecol. Prog. Series. Oldendorf 60 (1), 185–203. doi: 10.3354/meps060185
Rinkevich B. (2008). Management of coral reefs: We have gone wrong when neglecting active reef restoration. Mar. pollut. Bull. 56 (11), 1821–1824. doi: 10.1016/j.marpolbul.2008.08.014
Ritson-Williams R., Paul V. J., Arnold S. N., Steneck R. S. (2009). Larval settlement preferences and post-settlement survival of the threatened Caribbean corals acropora palmata and a. cervicornis. Coral Reefs 29 (1), 71–81. doi: 10.1007/s00338-009-0555-z
Ritson-Williams R., Paul V. J., Arnold S. N., Steneck R. S. (2010). Larval settlement preferences and post-settlement survival of the threatened Caribbean corals acropora palmata and a. cervicornis. Coral Reefs 29 (1), 71–81. doi: 10.1007/s00338-009-0555-z
Ritson-Williams R., Arnold S. N., Paul V. J. (2020). The impact of macroalgae and cyanobacteria on larval survival and settlement of the scleractinian corals Acropora palmata, A. cervicornis and Pseudodiploria strigosa. Mar. Biol. 167, 31. doi: 10.1007/s00227-019-3639-5
Schill S. R., Raber G. T., Roberts J. J., Treml E. A., Brenner J., Halpin P. N. (2015). No reef is an island: Integrating coral reef connectivity data into the design of regional-scale marine protected area networks. PloS One 10 (12), e0144199. doi: 10.1371/journal.pone.0144199
Serrano X., Baums I. B., O'Reilly K., Smith T. B., Jones R. J., Shearer T. L., et al. (2014). Geographic differences in vertical connectivity in the Caribbean coral Montastraea cavernosa despite high levels of horizontal connectivity at shallow depths. Mol. Ecol. 23, 4226–40. doi: 10.1111/mec.12861
Soloviev A. V., Hirons A., Maingot C., Dean C. W., Dodge R. E., Yankovsky A. E., et al. (2017). Southward flow on the western flank of the Florida current. Deep Sea Res. Part I: Oceanog. Res. Papers 125, 94–105. doi: 10.1016/j.dsr.2017.05.002
Soong K. (1991). Sexual reproductive patterns of shallow water reef corals in Panama. Bull. Mar. Sci. 49 (3), 832–846.
Sponaugle S., Lee T., Kourafalou V., Pinkard D. (2005). Florida Current frontal eddies and the settlement of coral reef fishes. Limnol. Oceanog. 50, 1033–1048. doi: 10.4319/lo.2005.50.4.1033
Stein J., Ruzicka R. (2021). Florida Reef resilience program disturbance response monitoring 2020 quick look report (Fish and Wildlife Research Institute). Available at: https://ocean.floridamarine.org/FRRP/Home/About.
Strathmann R. R., Hughes T. P., Kuris A. M., Lindeman K. C., Morgan S. G., Pandolfi J. M., et al. (2002). Evolution of local recruitment and its consequences for marine populations. Bull. Mar. Sci. 70 (1), 377–396.
Studvian M. S., Voss J. D. (2018). Population connectivity among shallow and mesophotic montastrea cavernosa corals in the gulf of Mexico identifies potential for refugia. Coral Reefs 37, 1183–1196. doi: 10.1007/s00338-018-1733-7
Suzuki G., Arakaki S., Hayashibara T. (2011). Rapid in situ settlement following spawning by acropora corals at ishigaki, southern Japan. Mar. Ecol. Prog. Ser. 421, 131–138. doi: 10.3354/meps08896
Szmant A. M. (1986). Reproductive ecology of Caribbean reef corals. Coral reefs 5 (1), 43–53. doi: 10.1007/BF00302170
Thomas C. J., Bridge T. C., Figueiredo J., Deleersnijder E., Hanert E. (2015). Connectivity between submerged and near-sea-surface coral reefs: can submerged reef populations act as refuges? Diversity Distribut. 21 (10), 1254–1266. doi: 10.1111/ddi.12360
Treml E. A., Roberts J. J., Chao Y., Halpin P. N., Possingham H. P., Riginos C. (2012). Reproductive output and duration of the pelagic larval stage determine seascape-wide connectivity of marine populations. Integr. Comp. Biol. 52 (4), 525–537. doi: 10.1093/icb/ics101
van Woesik R., Scott W. J., Aronson R. B. (2014). Lost opportunities: Coral recruitment does not translate to reef recovery in the Florida keys. Mar. pollut. Bull. 88 (2), 110–117. doi: 10.1016/j.marpolbul.2014.09.017
Vargas-Ángel B., Thomas J. D., Hoke S. M. (2003). High-latitude acropora cervicornis thickets off fort Lauderdale, Florida, USA. Coral Reefs 22 (4), 465–473. doi: 10.1007/s00338-003-0336-z
Vermeij M. J., Marhaver K. L., Huijbers C. M., Nagelkerken I., Simpson S. D. (2010). Coral larvae move toward reef sounds. PloS One 5 (5), e10660. doi: 10.1371/journal.pone.0010660
Walker B. K. (2012). Spatial analyses of benthic habitats to define coral reef ecosystem regions and potential biogeographic boundaries along a latitudinal gradient. PloS One 7 (1), e30466. doi: 10.1371/journal.pone.0030466
Walker B. (2017). Characterize the condition of previously known and newly identified large dense acropora cervicornis patches in southeast Florida (Miami, FL: Florida DEP), 1–35.
Walker B. K., Gilliam D. S. (2013). Determining the extent and characterizing coral reef habitats of the northern latitudes of the Florida reef tract (Martin county). PloS One 8 (11), e80439. doi: 10.1371/journal.pone.0080439
Walker B. K., Larson E. A., Moulding A. L., Gilliam D. S. (2012). Small-scale mapping of indeterminate arborescent acroporid coral (Acropora cervicornis) patches. Coral Reefs 31 (3), 885–894. doi: 10.1007/s00338-012-0910-3
Ward S., Harrison P., Hoegh-Guldberg O. (2002). “Coral bleaching reduces reproduction of scleractinian corals and increases susceptibility to future stress,” in Proceedings of the ninth international coral reef symposium, vol. 2. (Bali: The International Coral Reef Society), 1123–1128.
Weese D. J., Schwartz A. K., Bentzen P., Hendry A. P., Kinnison M. T. (2011). Eco-evolutionary effects on population recovery following catastrophic disturbance. Evolution. Appl. 4, 354–366. doi: 10.1111/j.1752-4571.2010.00169.x
Wilkinson C. (2000). Status of coral reefs of the world: 2000 (Australian Institute of Marine Science). Available at: https://icriforum.org/documents/status-of-coral-reefs-of-the-world-2000/.
Williams D. E., Miller M. W. (2012). Attributing mortality among drivers of population decline in acropora palmata in the Florida keys (USA). Coral Reefs 31 (2), 369–382. doi: 10.1007/s00338-011-0847-y
Williams D. E., Miller M. W., Kramer K. L. (2008). Recruitment failure in Florida keys acropora palmata, a threatened Caribbean coral. Coral Reefs 27 (3), 697–705. doi: 10.1007/s00338-008-0386-3
Wirt K. E., Hallock P., Palandro D., Lunz K. S. (2015). Potential habitat of acropora spp. on reefs of Florida, Puerto Rico, and the US virgin islands. Global Ecol. Conserv. 3, 242–255. doi: 10.1016/j.gecco.2014.12.001
Wood S., Paris C. B., Ridgwell A., Hendy E. J. (2014). Modelling dispersal and connectivity of broadcast spawning corals at the global scale. Global Ecol. Biogeog. 23 (1), 1–11. doi: 10.1111/geb.12101
Keywords: Acropora cervicornis, Acropora palmata, biophysical dispersal modeling, connectivity, metapopulation modeling, larval dispersal, Florida
Citation: King S, Saint-Amand A, Walker BK, Hanert E and Figueiredo J (2023) Larval dispersal patterns and connectivity of Acropora on Florida’s Coral Reef and its implications for restoration. Front. Mar. Sci. 9:1038463. doi: 10.3389/fmars.2022.1038463
Received: 07 September 2022; Accepted: 15 December 2022;
Published: 17 January 2023.
Edited by:
Hajime Kayanne, The University of Tokyo, JapanReviewed by:
Go Suzuki, Seikai National Fisheries Research Institute, JapanJean-Baptiste Ledoux, University of Porto, Portugal
Copyright © 2023 King, Saint-Amand, Walker, Hanert and Figueiredo. This is an open-access article distributed under the terms of the Creative Commons Attribution License (CC BY). The use, distribution or reproduction in other forums is permitted, provided the original author(s) and the copyright owner(s) are credited and that the original publication in this journal is cited, in accordance with accepted academic practice. No use, distribution or reproduction is permitted which does not comply with these terms.
*Correspondence: Samantha King, c2Fta2luZ0Bhcml6b25hLmVkdQ==
†Present Address: Samantha King, Department of Ecology and Evolutionary Biology, University of Arizona, Tucson, AZ, United States