- 1Key Laboratory of Marine Ecosystem Dynamics, Second Institute of Oceanography, Ministry of Natural Resources, Hangzhou, China
- 2Fujian Key Laboratory on Conservation and Sustainable Utilization of Marine Biodiversity, Fuzhou Institute of Oceanography, Minjiang University, Fuzhou, China
Seawater temperatures have increased with global climate change. Coolant water discharged from coastal nuclear power-generating and coal-powered plants, coupled with already increasing seawater temperatures, can adversely affect local fish communities. A sudden drop in temperature caused by the winter shutdown of power plants can also affect fish health and behavior. To assess the effects of temperature change on fish populations, we subjected early life stages of the once commercially important large yellow croaker (Larimichthys crocea) to various water temperature experiments. Fertilized eggs showed the highest hatching rate at 23.4°C and the lowest rate of deformity in hatched larvae at 23.0°C. We determined the incipient lethal temperature for each life stages using derivation models. Ranges between the upper and lower incipient lethal temperatures increased during development from yolk-sac larvae to juveniles, especially in response to cold shock, indicating that later developmental stages in this species are more tolerant of temperature fluctuations. However, thermal tolerance is not solely determined by life stage. Our results suggest that rapid changes in seawater temperature caused by power plant coolant water discharges may significantly affect early developmental stages of fish. Critical thermal maximum tests indicate that the seawater heating rate is significantly negatively correlated with survival time and affects the critical thermal maximum value of L. crocea. On the basis of our determination of incipient lethal temperatures, emergency measures could be taken to avoid adverse economic and ecological impacts due to changes in seawater temperature caused by coolant water discharges.
Introduction
Sea surface temperature (SST) is affected by various factors, and changes in SST can affect recruitment in fish populations (Almodóvar et al., 2012; Cheung et al., 2013). Marine fishes are sensitive to temperature change, which affects their energy distribution (Trancart et al., 2016). Low amplitude in temperature rising greatly increases fish activity and affects their feeding behavior (Biro et al., 2010). If a physiologically optimum temperature is exceeded, then the increased metabolic rate and decreased energy intake can reduce growth rates (Killen et al., 2010). At high water temperatures, fish populations without adequate food sources can experience increased mortality (Biro et al., 2007; Munday et al., 2008).
Between 1950 and 1980, the large yellow croaker (Larimichthys crocea, Richardson) was a commercially important marine fish species in China; however, the fishery collapsed in the 1980s. Even after implementation of a fishing moratorium, stocks never fully recovered to satisfy market demands, and the population of L. crocea remained depressed (Chen and Zhang, 1984; Xu and Liu, 2007; Xu and Chen, 2011; Zhang et al., 2018). Conversely, according to the Chinese Fishery Statistical Yearbook, L. crocea has become the dominant species in mariculture, with a 9.46% growth rate in farming scale and a 14.11% increase in seeding from 2003 to 2020. Most farming of L. crocea occurs is in Fujian Province. The inadequacy of offshore farming models and a lack of data on the temperature tolerance of L. crocea at different life stages can be problematic for farmers when faced with sudden changes in SST due to external factors such as coolant water discharges from power plants (Cai et al., 2020).
Waste heat generated by power plants is generally removed using water, for which reason many power plants are built along coasts where coolant water is readily available (Rosen, 2001; Aminov et al., 2017). Affected by warm water discharged from these power plants, the offshore seawater temperatures increase. Discharge of heated coolant water from coastal power plants can exacerbate the rise in coastal water temperatures already caused by climate change, resulting in an increase of approximately 5°C in waters thousands of meters away from the discharge port, which can affect nearshore benthic, planktonic, and nektonic organisms (Ma et al., 2016; Lee et al., 2018). In addition, the temperature drop caused by stopping the discharge of coolant water can also have adverse effects. Power plants can shut down for several reasons such as electricity overproduction, material replacement, or maintenance, resulting in abrupt water temperature decreases in adjacent receiving waters (Zhao et al., 2006; Madden et al., 2013; Buhariwalla et al., 2016). For fish, such sudden cold shocks can increase the number of mitochondria in cells, increase respiratory and energy consumption rates, damage the respiratory system, or cause mortality (Guderley, 2004; Begriche et al., 2006; Kavanagh et al., 2010).
To understand the effects of temperature changes on fish populations, there are well-established methods of separately determining the incipient lethal temperature (ILT) and critical thermal maximum (CTMax) for a species (Beitinger et al., 2000; Golovanov, 2012). The ILT represents the tolerance limit of the species, which is the temperature at which half of the test animals die when exposed to a series of higher or lower temperatures after acclimation to the relevant temperature for a particular environment (Brett, 1952; Jobling, 1981). In this study, the upper and lower ILTs (ULIT and LLIT, respectively) were determined for different life stages of L. crocea to simulate the effects of temperature shock caused by coolant water discharges. CTMax represents the thermal resistance of a species, which is determined by increasing the water temperature at a constant rate until the fish lose equilibrium and opercular movement ceases (Otto, 1973; Becker and Genoway, 1979). CTMax was calculated to simulate the effects of coolant water discharged at different rates on fish populations. The hatching rate of eggs exposed to different temperatures was also determined to simulate the effects of coolant water discharge (Okamura et al., 2007). We report UILT, LILT, and CTMax values for different developmental stages of L. crocea and discuss the differences in temperature tolerance among different life history stages of this species. In addition, we suggest management measures that could be taken to avoid adverse economic and ecological impacts due to coolant water discharges.
Materials and methods
Preparation of test animals and seawater
Experiments were performed with four developmental stages of L. crocea (fertilized eggs, yolk-sac larvae, larvae, and juveniles). Test animals were provided by the Ningbo Marine and Fishery Research Institute, and their body sizes are shown in Table 1. The fish were kept in natural sand-filtered seawater with a normal saturation oxygen level, constant temperature (22°C), constant salinity (25.6‰), and a natural light regime.
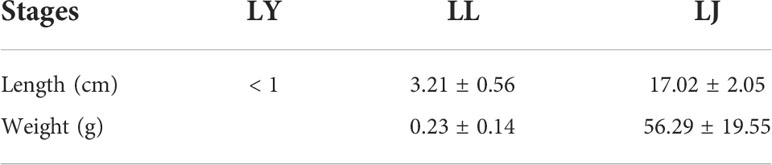
Table 1 Length and weight of Larimichthys crocea at different developmental stages: yolk-sac larvae (LY), larvae (LL), and juveniles (LJ).
Heat-shock seawater was heated using a titanium heating rod (Armaturenbau, 100–2,000 W) and calibrated using a precision thermometer (Korea A-MI 211H; range, 0°C–90°C; sensitivity, 0.1°C). Cold-shock seawater was prepared using a cooling incubator (Yi Heng LRH-150; range, 0°C–60°C; sensitivity, 0.1°C). Aeration and natural light were maintained, and temperature was measured at regular intervals with a mercury thermometer.
Fertilized egg hatching experiments
At the beginning of the experiment, healthy fertilized eggs were transferred directly from 22°C seawater to prepared seawater (15°C, 18°C, 21°C, 24°C, 27°C, 30°C, and 33°C) in 500-ml beakers. One hundred eggs were placed in each beaker, and each temperature test was replicated three times. We observed the eggs hatch and hatched larvae development until all eggs hatched or sank to the bottom. The hatching rate was calculated by dividing the number of eggs hatched by the total number of eggs in each beaker, and the deformity rate was calculated by determining the proportion of hatched larvae exhibiting spinal deformity, tail curvature, or fin membrane decay (Figure 1).
Temperature jump experiments
Yolk-sac larvae were removed from the acclimation environment to the 500-ml beakers, which contained 22°C, 24°C, 26°C, 28°C, 30°C, 32°C, and 34°C seawater for the heat-shock experiments, and 22°C, 19°C, 16°C, 13°C, and 10°C seawater for the cold-shock experiments. Experimental control groups maintained at 22°C were used in both heat-shock and cold-shock experiments to simulate natural ambient seawater unaffected by coolant water discharges.
Mortality of larvae and juveniles spiked in two sequential experimental groups (30°C and 32°C) in the heat-shock experiments, which required adding one intermediate temperature level at 31°C. Furthermore, in the cold-shock experiments, some larvae and juveniles exhibited no effects at 10°C, which required adding four lower experimental temperature levels (8°C, 6°C, 4°C, and 2°C). Consequently, larvae and juveniles were removed from the acclimation environment and placed in 5- and 50-L tanks, which contained seawater maintained at 22°C, 24°C, 26°C, 28°C, 30°C, 31°C, 32°C, and 34°C for the heat-shock experiments, and seawater maintained at 22°C, 20°C, 18°C, 16°C, 14°C, 12°C, 10°C, 8°C, 6°C, 4°C, and 2°C for the cold-shock experiments.
A total of 20 yolk-sac larvae, 30 larvae, and 20 juveniles were exposed to each temperature treatment for 48 h, and approximately100–300 rotifers (Brachionus plicatilis; Müller) and fish feed were provided daily as food. This frequency of feeding prevented starvation stress, as indicated by the food remaining in the water at the end of the experiment. Each temperature treatment was replicated three times for each of the three growth stages.
The behavioral responses of yolk-sac larvae, larvae, and juveniles were observed continuously over 48 h in each temperature treatment, and the number of dead individuals in each experimental group was recorded. Death was defined as the cessation of opercular movement or the absence of response to touch.
Critical thermal maximum experiments
We measured the reactions of larvae and juveniles exposed to different rates of increase in water temperature. The initial water temperature was 22°C for both larvae and juveniles, and a heating rod was used to control the rate of temperature increase (0.5°C, 3°C, 6°C, and 15°C h−1). For each temperature heating rate exposure, 30 larvae and 20 juveniles were used, and each exposure was replicated three times. Adequate fish feed was provided as food. Throughout the experiment, we noted any larval or juvenile movement disorders, abnormal behaviors, loss of balance, body turnover, or other reactions and recorded the experimental seawater temperature at the time any such observation was made as the CTMax value for that individual. The abnormal subjects were then removed and placed in buffered seawater maintained at 22°C until the CTMax values for all test organisms were observed and recorded.
Statistical analyses
Statistical analyses were performed using SPSS v. 20.0 (IBM Corp., Armonk, NY, USA) and visualized in Origin 2021 (OriginLab, Northampton, MA, USA). Normality and homogeneity of data were assessed using Shapiro–Wilk’s and Levene’s tests. For data with normal distribution and homogeneity of variance, one-way ANOVA and Duncan tests were used to evaluate differences for overall and pairwise comparisons. For data with non-normal distribution or non-homogeneity of variance, Kruskal–Wallis and Games–Howell tests were used, respectively. Spearman rank correlation analysis was performed to identify relationships between two groups of correlated data.
Results
Fertilized egg temperature tolerance
Hatching and deformity rates for fertilized eggs exposed to different temperatures over 48 h are shown in Figure 2. Significant differences in hatching rates were shown among different treatments (one-way ANOVA, F = 298.598, P < 0.05). The highest hatching rate occurred at 21°C and decreased significantly with increased temperature. The lowest larval deformity rates occurred at 21°C and 24°C (Kruskal–Wallis test, P < 0.05), and all larvae hatched at either 15°C or 33°C were deformed. Spearman rank correlation test results for hatching and deformity rates are shown in Table 2.
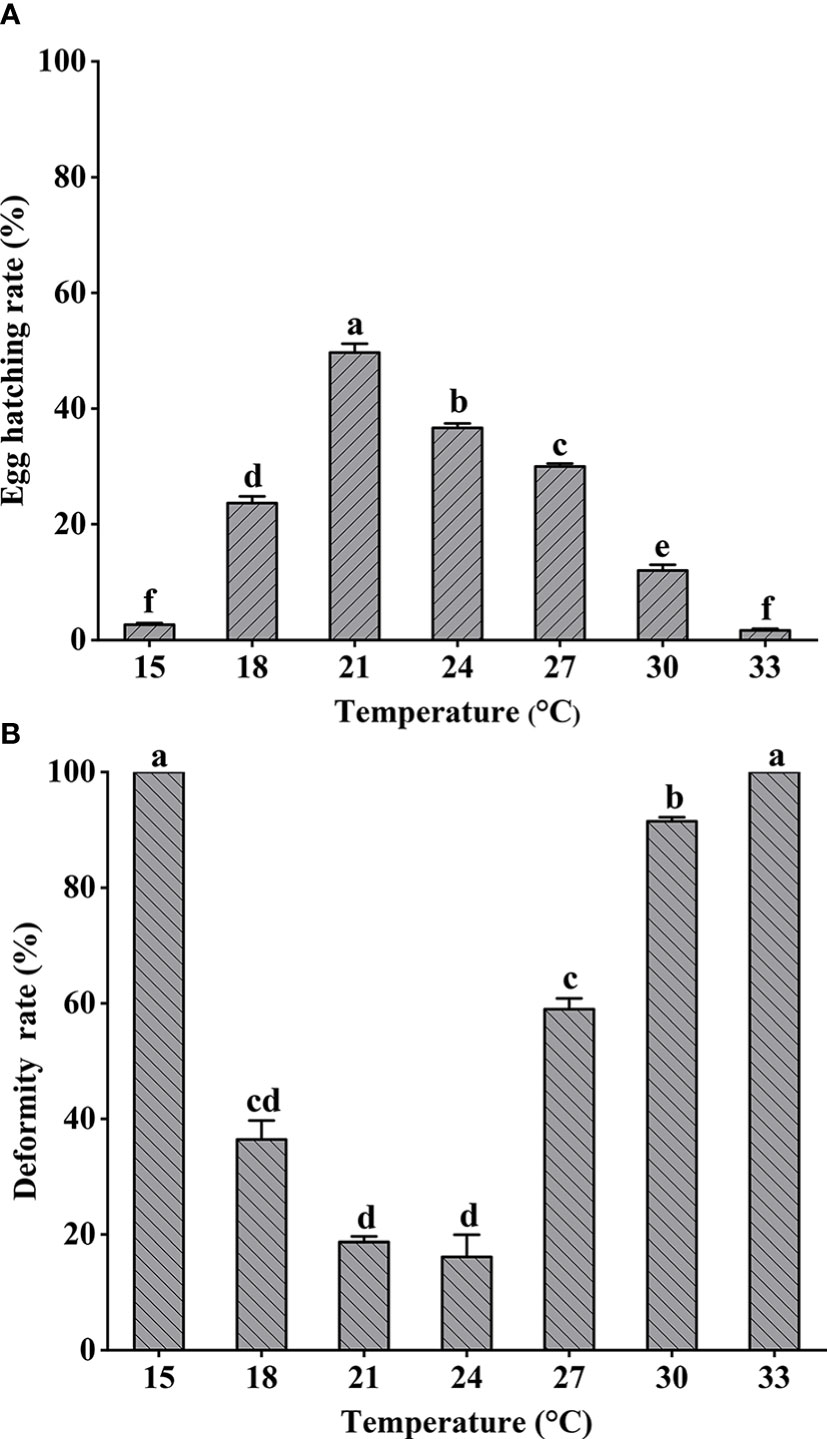
Figure 2 Larimichthys crocea fertilized eggs hatching rate (A) and hatched larvae deformity rate (B) exposed to different temperatures (mean ± SD). Labeled means without a common letter differ (P < 0.05).
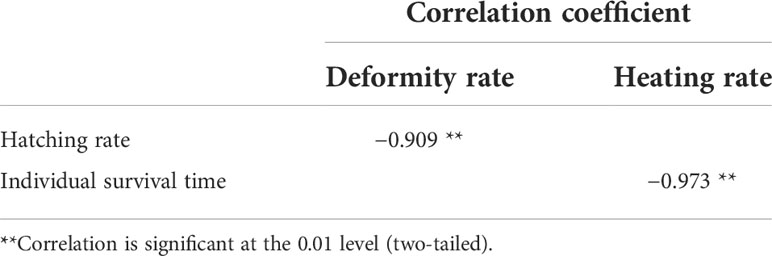
Table 2 Spearman rank correlation analysis results on hatching and deformity rates, individual survival time and heating rate.
Effects of temperature shock on large yellow croaker
Changes in the mortality of L. crocea exposed to cold shock are shown in Figure 3. Mortality rates of yolk-sac larvae increased significantly as temperature decreased (one-way ANOVA, F = 6.684, P < 0.05) but did not differ significantly at temperatures >10°C, the point at which 100% mortality occurred.
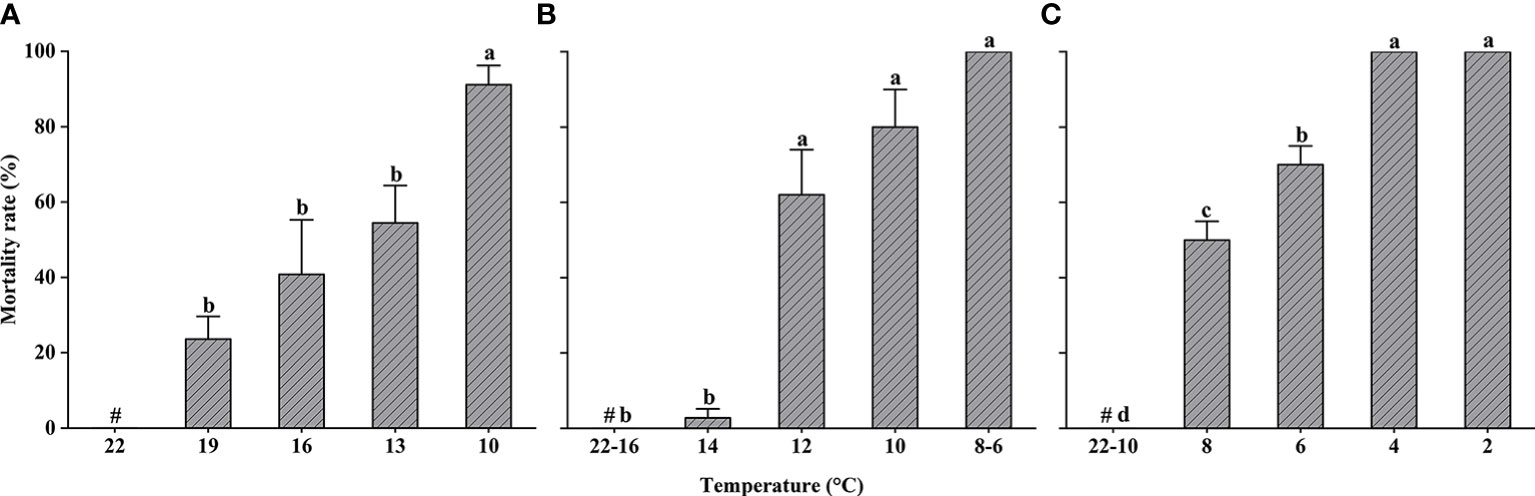
Figure 3 Larimichthys crocea yolk-sac larvae (A), larvae (B), and juvenile (C) mortality rate in cold-shock treatments (mean ± SD). Labeled means without a common letter differ (P < 0.05). #Control treatment.
Larvae were exposed to cold-shock temperatures as low as 6°C, and the mortality rate of larvae in both the 8°C and 6°C experimental groups was 100% (Kruskal–Wallis test, P < 0.05). To avoid unnecessary impacts to test organisms, larvae were not exposed to temperatures lower than 6°C. In contrast to the results of the yolk-sac larvae experiment, larval mortality did not occur until the temperature dropped to 14°C. The mortality rate of larvae exposed to temperatures ≤12°C was significantly higher than the mortality rate of larvae in the 14°C experimental group.
Juveniles were exposed to cold-shock temperatures as low as 2°C, and none exhibited mortality at temperatures ≥10°C.
Changes in the mortality of L. crocea exposed to heat shock are shown in Figure 4. After the yolk-sac larvae were acclimated at 22°C, they exhibited a significant increase in mortality in response to a sudden increase in temperature (Kruskal–Wallis test, P < 0.05) (Figure 4A). The mortality rate was less than 20% for yolk-sac larvae in the 24°C and 26°C experimental groups. All yolk-sac larvae died in the three replicate experimental groups at 34°C.
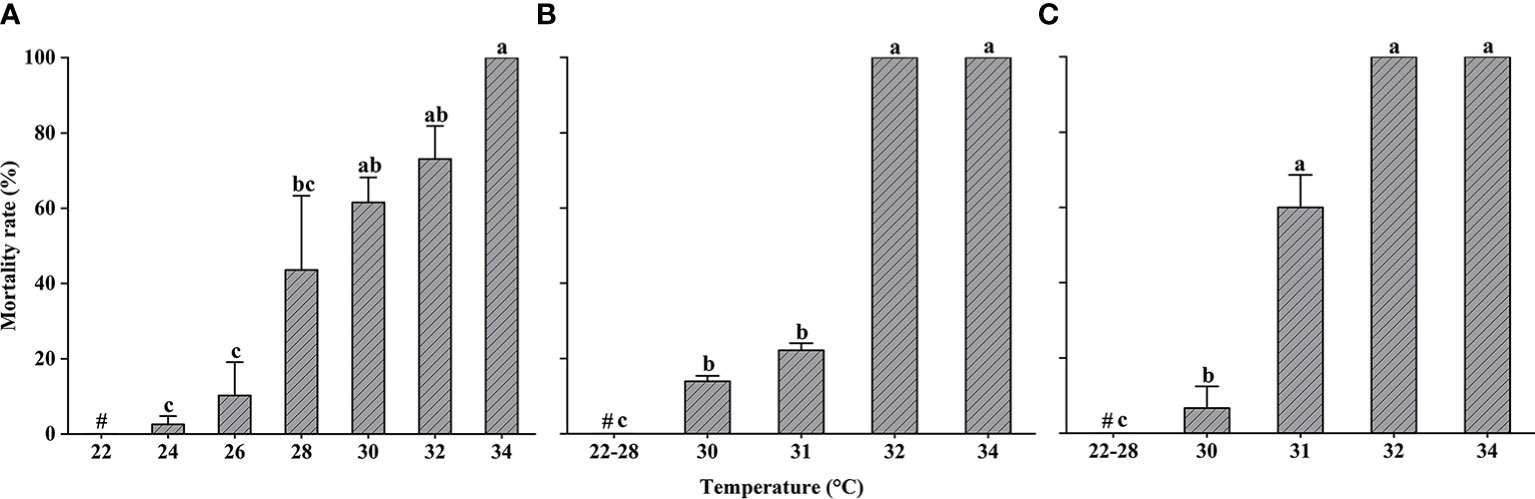
Figure 4 Larimichthys crocea yolk-sac larvae (A), larvae (B), and juvenile (C) mortality rate in heat-shock treatments (mean ± SD). Labeled means without a common letter differ (P < 0.05). #Control treatment.
Larvae did not exhibit any mortality at temperatures ≤28°C; however, larval mortality increased significantly at 30°C, 31°C, and 32°C (Kruskal–Wallis test, P < 0.05). Larval mortality at 34°C reached 100%.
Similar to larvae, juveniles did not exhibit any mortality at temperatures ≤28°C; however, at higher temperatures, there was a significant difference in mortality compared to the control group (Kruskal–Wallis test, P < 0.05).
We systematically described the mortality of yolk-sac larvae, larvae, and juveniles in different temperature shock experimental groups and used three calculation models to fit the mortality of fish at different life stages exposed to heat shock or cold shock after acclimation at 22°C (Cherry et al., 1977; Urquhart and Koetsier, 2013; Kir, 2020). UILT, LILT, model type, and input parameters are shown in Table 3.
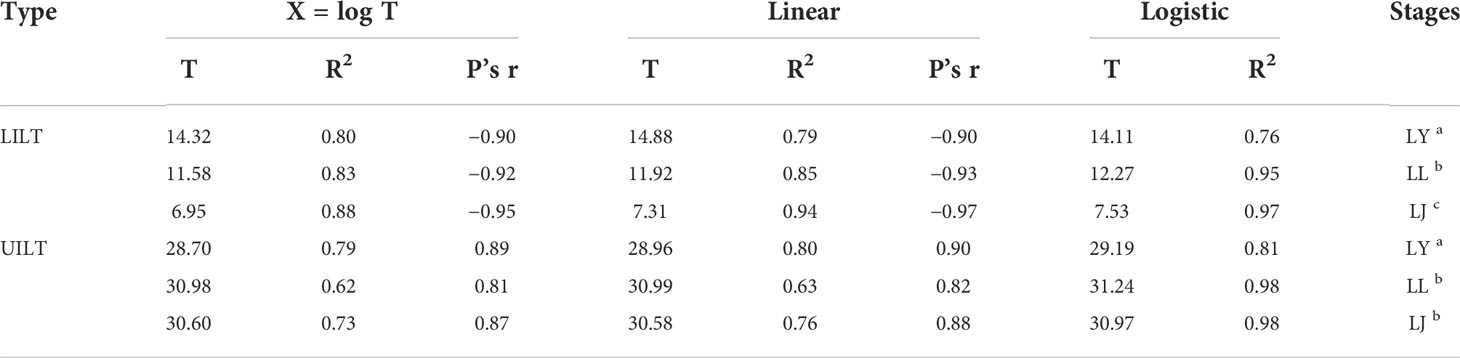
Table 3 Pearson’s r and R2 for Larimichthys crocea yolk-sac larvae (LY), larvae (LL), and juveniles (LJ) about upper and lower incipient lethal temperature (LILT and UILT). Labeled means without a common letter differ (P < 0.05).
Thermal resistance of larvae and juveniles at different heating rates
Larval and juvenile survival at different heating rates is shown in Figure 5. Average survival time differed significantly among treatments (Kruskal–Wallis test, P < 0.05); however, survival times for larvae and juveniles exposed to the same heating rate were not significantly different (paired sample T-test, P > 0.05).
CTMax values for larval and juvenile stages at a heating rate of 0.5°C h−1 were much higher than for other treatments (paired sample T-test, P < 0.05). There was no significant difference in CTMax among treatment groups exposed to heating rates >0.5°C h−1. The trend in CTMax change was similar for larval and juvenile stages (Figure 6).
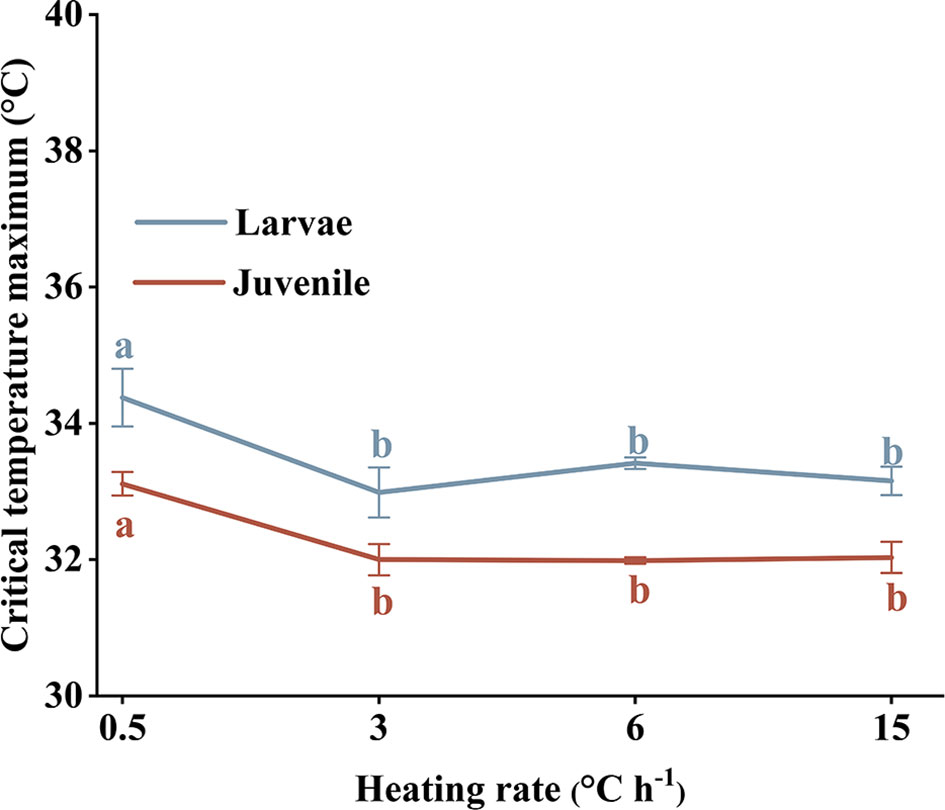
Figure 6 Larvae and juveniles Larimichthys crocea critical temperature maxima (CTMax) subjected to different heating rates. Labeled means without a common letter differ (P < 0.05).
Discussion
Responses of fertilized eggs to temperature fluctuations
Water temperature affects early fish development (Decuypere and Michels, 2019). Compared with salinity and dissolved oxygen, temperature is worthy of paying more attention during the incubation process (Alderdice and Forrester, 1968; Geist et al., 2011). Lower temperatures can prolong hatching time, whereas higher temperatures can accelerate development (Pauly and Pullin, 1988; Teletchea et al., 2009). We observed this phenomenon in our experiments, as well. During culture of L. crocea, Lin et al. (1991) found that the optimum temperature for fertilized eggs was 18°C–24°C. Consistent with this finding, we determined (by curve fitting hatching rate with temperature) that the optimum hatching temperature for L. crocea was 23.4°C, which is similar to seawater temperatures where L. crocea spawns (Wu et al., 2022). Therefore, discharging of heated coolant water should be avoided during the incubation period if possible. We found that the lowest rate of deformity in hatched larvae occurred at 23°C. In culturing L. crocea, a balance must be maintained between the hatching rate and the quality of the hatched larvae. High temperatures can accelerate hatching but can also reduce larval quality due to thermal stress (Ojanguren et al., 1999; Moran et al., 2007). Fluctuations in temperature are known to affect fertilized egg hatching time and rate; however, the impact of such fluctuations on malformation rates in hatched larvae was previously unknown (Koo and Johnston, 1978; Kurokawa et al., 2008). In this study, we found a significant negative correlation between rates of hatching and malformation (Table 2). We also found that temperatures producing the highest hatching rate and the highest quality hatched larvae were similar, indicating that this temperature regime could be successfully employed in L. crocea culture.
Tolerance of different life stages to heat and cold shock
Overfishing and lack of protection measures have depleted L. crocea stocks (Liu and De Mitcheson, 2008). The discharge of coolant water from power plants near L. crocea habitat is known to adversely affect population recruitment; however, few studies have investigated the tolerance of different life stages of L. crocea to temperature change (Wu et al., 2022). Understanding the causes of ILT and CTMax for different life stages is essential to restoring wild resources, guiding artificial breeding programs, and warning of likely impacts due to frequent, abnormal, or sudden temperature changes (Wernberg et al., 2012).
Among the multiple factors that affect ILT and CTMax, life stage is considered to be one of the most critical. Some studies have found that younger fish were more thermotolerant (Recsetar et al., 2012; Di Santo and Lobel, 2017), whereas others found that older fish were more tolerant to temperature changes (Charo-Karisa et al., 2005; Moyano et al., 2017). Some researchers have questioned the existence of a life stage effect, noting that the evidence for such an effect was inconclusive and finding little variation in temperature tolerance among fish of different stages (Ospina and Mora, 2004; Recsetar et al., 2012). Temperature tolerance has been linked to individual differences in metabolic rate and related to variations in key life history traits that could affect ecological patterns within animal populations (Norin et al., 2015). Therefore, a more likely explanation for changes in temperature tolerance may be related to individual life stage and bottlenecks during life stages. Older fish have more mature organs and physiological systems but require extra energy for activities such as spawning (Baroudy and Elliott, 1994; Dahlke et al., 2020). This could explain the increasing temperature range between LILT and UILT with the growth of L. crocea, as well as the lack of change in UILT between the larval and juvenile stages, a finding consistent with that of Baroudy and Elliott (1994).
Effect of heating rate on critical temperature maxima
Discharge of power plant coolant water is necessary for power production. Larvae and juvenile fish are strongly motile (Pavlov et al., 2008) and can escape elevated temperatures should they encounter coolant water (Crozier et al., 2008; Rijnsdorp et al., 2009). We describe a power function relationship between individual survival time and heating rate, with a statistically significant negative correlation (Table 2). The absence of any significant differences in larval and juvenile survival times suggests that the escape time for larvae and juveniles was determined more by the heating rate than the growth stage. Therefore, during the discharge of coolant water from power plants, the rate of increase in seawater temperature should be controlled to provide fish in early developmental stages with sufficient escape time to avoid suboptimal temperature conditions, thereby aiding wild population recovery.
When the heating rate exceeds a certain value, CTMax does not change and is generally greater for larvae than juveniles (Elliott and Elliott, 1995; Beitinger and Bennett, 2000; Das et al., 2004). Elliott and Elliott (1995) and Mora and Maya (2006) found that CTMax varied with heating rate, being greatest at 1°C h−1, with any change at increased rates being insignificant. We found that CTMax was highest at a heating rate of 0.5°C h−1 and trended downward at higher heating rates and, similarly, fluctuate insignificantly thereafter. Because CTMax does not change significantly at higher heating rates, rapid thermal shock probably causes irreversible impacts (Landsman et al., 2011). If CTMax experiments were performed on a variety of coastal fish species using different rates of temperature increase, then the results could be used to develop and implement appropriate treatment methods and discharge rates to minimize the damage to fish communities caused by heated coolant water.
Conclusion
In this paper, we described the responses of different life history stages of L. crocea to temperature change in heat-shock and cold-shock experiments. Fertilized egg hatching and malformation rates were negatively correlated, and the optimum hatching temperature was determined to be 23.4°C. Application of these findings to the culture of L. crocea could increase the survival of cultured fish. UILT and LILT values showed that the tolerance of L. crocea to temperature fluctuations increased during development from the yolk-sac larval stage to the juvenile stage. We discussed the impact of individual life stage and bottlenecks in the life cycle of L. crocea on ILT variation. As the thermotolerance mechanism in fish matures, the difference between the UILT of larvae and juveniles is less significant than the LILT. The discharge of coolant water and its interruption should be timed to avoid impacts on early life stages with poor tolerance to temperature change. We also found a significant negative correlation between survival time and heating rate. When coolant water is discharged, the rate of temperature increase should be monitored. Controlling the discharge rate of coolant water could provide early life stages of fishes in coastal waters with more time to react to elevated temperatures, thus reducing damage to coastal fisheries.
Data availability statement
The original contributions presented in the study are included in the article/supplementary material. Further inquiries can be directed to the corresponding author.
Ethics statement
The animal study was reviewed and approved by The Institutional Animal Care and Use Committee of Second Institute of Oceanography, Ministry of Natural Resources, China.
Author contributions
ST and KZ contributed equally to this work and share first authorship. ST, KZ: Formal analysis; Methodology; Writing - Original Draft; Writing - review and editing. YL: Resources; Data curation; Project administration. YT, QL, RZ: Writing - Review and Editing. LS, JZ: Supervision; Funding acquisition. All authors contributed to the article and approved the submitted version.
Funding
The present work was supported financially by the National Natural Science Foundation of China (41706125) and the Scientific Research Fund of the Second Institute of Oceanography, MNR, China (JG2209 and JG1914).
Acknowledgments
We thank wordvice (https://www.wordvice.com) for editing a draft of this manuscript.
Conflict of interest
The authors declare that the research was conducted in the absence of any commercial or financial relationships that could be construed as a potential conflict of interest.
Publisher’s note
All claims expressed in this article are solely those of the authors and do not necessarily represent those of their affiliated organizations, or those of the publisher, the editors and the reviewers. Any product that may be evaluated in this article, or claim that may be made by its manufacturer, is not guaranteed or endorsed by the publisher.
References
Alderdice D. F., Forrester C. R. (1968). Some effects of salinity and temperature on early development and survival of the English sole (Parophrys vetulus). J. Fisheries Res. Board Canada. 25, 495–521. doi: 10.1139/f68-043
Almodóvar A., Nicola G. G., Ayllón D., Elvira B. (2012). Global warming threatens the persistence of Mediterranean brown trout. Global Change Biol. 18, 1549–1560. doi: 10.1111/j.1365-2486.2011.02608.x
Aminov R. Z., Shkret A. F., Garievskii M. V. (2017). Thermal and nuclear power plants: Competitiveness in the new economic conditions. Thermal Eng 64, 319–328. doi: 10.1134/S0040601517050019
Baroudy E., Elliott J. M. (1994). The critical thermal limits for juvenile Arctic charr Salvelinus alpinus. J. Fish Biol. 45, 1041–1053. doi: 10.1111/j.1095-8649.1994.tb01071.x
Becker C. D., Genoway R. G. (1979). Evaluation of the critical thermal maximum for determining thermal tolerance of freshwater fish. Environ. Biol. Fish 4, 245–256. doi: 10.1007/bf00005481
Begriche K., Igoudjil A., Pessayre D., Fromenty B. (2006). Mitochondrial dysfunction in Nash: Causes, consequences and possible means to prevent it. Mitochondrion 6, 1–28. doi: 10.1016/j.mito.2005.10.004
Beitinger T. L., Bennett W. A. (2000). Quantification of the role of acclimation temperature in temperature tolerance of fishes. Environ. Biol. Fish 58, 277–288. doi: 10.1023/A:1007618927527
Beitinger T. L., Bennett W. A., Mccauley R. W. (2000). Temperature tolerances of north American freshwater fishes exposed to dynamic changes in temperature. Environ. Biol. Fish 58, 237–275. doi: 10.1023/a:1007676325825
Biro P. A., Beckmann C., Stamps J. A. (2010). Small within-day increases in temperature affects boldness and alters personality in coral reef fish. Proc. Biol. Sci. 277, 71–77. doi: 10.1098/rspb.2009.1346
Biro P. A., Post J. R., Booth D. J. (2007). Mechanisms for climate-induced mortality of fish populations in whole-lake experiments. Proc. Natl. Acad. Sci. U S A. 104, 9715–9719. doi: 10.1073/pnas.0701638104
Brett J. R. (1952). Temperature tolerance in young pacific salmon, genus Oncorhynchus. J. Fisheries Res. Board Canada. 9, 265–323. doi: 10.1139/f52-016
Buhariwalla C. F., Macmillan J. L., Gregoire M. J., Dadswell M. J., Stokesbury M. J. W. (2016). Population characteristics of striped bass killed by cold shock during winter shutdown of a power plant in Nova Scotia. Northeastern Naturalist. 23, 163–173. doi: 10.1656/045.023.0113
Cai X., Zhang J., Lin L., Li Y., Liu X., Wang Z. (2020). Study of a noninvasive detection method for the high-temperature stress response of the Large yellow croaker (Larimichthys crocea). Aquac Rep. 18:100514. doi: 10.1016/j.aqrep.2020.100514
Charo-Karisa H., Rezk M. A., Bovenhuis H., Komen H. (2005). Heritability of cold tolerance in Nile tilapia, Oreochromis niloticus, juveniles. Aquaculture 249, 115–123. doi: 10.1016/j.aquaculture.2005.04.029
Chen B., Zhang C. (1984). Preliminary study on the biological basis of Large yellow croaker fishery in southern fujian. J. Fujian Fish 4, 6–16.
Cherry D. S., Dickson K. L., Cairns J. Jr., Stauffer J. R. (1977). Preferred, avoided, and lethal temperatures of fish during rising temperature conditions. J. Fisheries Res. Board Canada. 34, 239–246. doi: 10.1139/f77-035
Cheung W. W., Watson R., Pauly D. (2013). Signature of ocean warming in global fisheries catch. Nature 497, 365–368. doi: 10.1038/nature12156
Crozier L. G., Hendry A. P., Lawson P. W., Quinn T. P., Mantua N. J., Battin J., et al. (2008). Potential responses to climate change in organisms with complex life histories: Evolution and plasticity in pacific salmon. Evol. Appl. 1, 252–270. doi: 10.1111/j.1752-4571.2008.00033.x
Dahlke F. T., Wohlrab S., Butzin M., Portner H. O. (2020). Thermal bottlenecks in the life cycle define climate vulnerability of fish. Science 369, 65–70. doi: 10.1126/science.aaz3658
Das T., Pal A. K., Chakraborty S. K., Manush S. M., Chatterjee N., Mukherjee S. C. (2004). Thermal tolerance and oxygen consumption of Indian major carps acclimated to four temperatures. J. Thermal Biol. 29, 157–163. doi: 10.1016/j.jtherbio.2004.02.001
Decuypere E., Michels H. (2019). Incubation temperature as a management tool: A review. World’s Poultry Sci. J. 48, 28–38. doi: 10.1079/wps19920004
Di Santo V., Lobel P. S. (2017). Body size and thermal tolerance in tropical gobies. J. Exp. Mar. Biol. Ecol 487, 11–17. doi: 10.1016/j.jembe.2016.11.007
Elliott J. M., Elliott J. A. (1995). The effect of the rate of temperature increase on the critical thermal maximum for parr of Atlantic salmon and brown trout. J. Fish Biol. 47, 917–919. doi: 10.1111/j.1095-8649.1995.tb06014.x
Geist D. R., Abernethy C. S., Hand K. D., Cullinan V. I., Chandler J. A., Groves P. A. (2011). Survival, development, and growth of fall Chinook salmon embryos, alevins, and fry exposed to variable thermal and dissolved oxygen regimes. Trans. Am. Fisheries Soc 135, 1462–1477. doi: 10.1577/T05-294.1
Golovanov V. K. (2012). Influence of various factors on upper lethal temperature (Review). Inland Water Biol. 5, 105–112. doi: 10.1134/s1995082911040079
Guderley H. (2004). Metabolic responses to low temperature in fish muscle. Biol. Rev. Camb Philos. Soc 79, 409–427. doi: 10.1017/S1464793103006328
Jobling M. (1981). Temperature tolerance and the final preferendum-rapid methods for the assessment of optimum growth temperatures. J. Fish Biol. 19, 439–455. doi: 10.1111/j.1095-8649.1981.tb05847.x
Kavanagh K. D., Haugen T. O., Gregersen F., Jernvall J., Vollestad L. A. (2010). Contemporary temperature-driven divergence in a Nordic freshwater fish under conditions commonly thought to hinder adaptation. BMC Evol. Biol. 10, 350. doi: 10.1186/1471-2148-10-350
Killen S. S., Atkinson D., Glazier D. S. (2010). The intraspecific scaling of metabolic rate with body mass in fishes depends on lifestyle and temperature. Ecol. Lett. 13, 184–193. doi: 10.1111/j.1461-0248.2009.01415.x
Kir M. (2020). Thermal tolerance and standard metabolic rate of juvenile gilthead seabream (Sparus aurata) acclimated to four temperatures. J. Therm Biol. 93, 102739. doi: 10.1016/j.jtherbio.2020.102739
Koo T. S. Y., Johnston M. L. (1978). Larva deformity in striped bass, Morone saxatilis (Walbaum), and blueback herring, Alosa aestivalis (Mitchill), due to heat shock treatment of developing eggs. Environ. pollut. (1970). 16, 137–149. doi: 10.1016/0013-9327(78)90128-3
Kurokawa T., Okamoto T., Gen K., Uji S., Murashita K., Unuma T., et al. (2008). Influence of water temperature on morphological deformities in cultured larvae of Japanese eel, Anguilla japonica, at completion of yolk resorption. J. World Aquac Soc 39, 726–735. doi: 10.1111/j.1749-7345.2008.00208.x
Landsman S. J., Gingerich A. J., Philipp D. P., Suski C. D. (2011). The effects of temperature change on the hatching success and larvae survival of largemouth bass Micropterus salmoides and smallmouth bass Micropterus dolomieu. J. Fish Biol. 78, 1200–1212. doi: 10.1111/j.1095-8649.2011.02927.x
Lee P. W., Tseng L. C., Hwang J. S. (2018). Comparison of mesozooplankton mortality impacted by the cooling systems of two nuclear power plants at the northern Taiwan coast, southern East China Sea. Mar. pollut. Bull. 136, 114–124. doi: 10.1016/j.marpolbul.2018.09.003
Lin D., Zhang J., Zheng Z., Weng Z., Su Y. (1991). Studies on the artificial propagation of the Large yellow croake, Pseudosciaena crocea (Richardson). J. Fujian Normal Univ(Natural Sci. Edition). 7, 71–79.
Liu M., De Mitcheson Y. S. (2008). Profile of a fishery collapse: Why mariculture failed to save the Large yellow croaker. Fish Fish 9, 219–242. doi: 10.1111/j.1467-2979.2008.00278.x
Ma P., Dai X., Guo Z., Wei C., Ma W. (2016). Detection of thermal pollution from power plants on china’s Eastern coast using remote sensing data. Stochastic Environ. Res. Risk Assess 31, 1957–1975. doi: 10.1007/s00477-016-1293-8
Madden N., Lewis A., Davis M. (2013). Thermal effluent from the power sector: An analysis of once-through cooling system impacts on surface water temperature. Environ. Res. Letters. 8:035006. doi: 10.1088/1748-9326/8/3/035006
Mora C., Maya M. F. (2006). Effect of the rate of temperature increase of the dynamic method on the heat tolerance of fishes. J. Thermal Biol. 31, 337–341. doi: 10.1016/j.jtherbio.2006.01.005
Moran D., Smith C. K., Gara B., Poortenaar C. W. (2007). Reproductive behaviour and early development in yellowtail kingfish (Seriola lalandi, valenciennes 1833). Aquaculture 262, 95–104. doi: 10.1016/j.aquaculture.2006.10.005
Moyano M., Candebat C., Ruhbaum Y., Alvarez-Fernandez S., Claireaux G., Zambonino-Infante J. L., et al. (2017). Effects of warming rate, acclimation temperature and ontogeny on the critical thermal maximum of temperate marine fish larvae. PloS One 12, e0179928. doi: 10.1371/journal.pone.0179928
Munday P. L., Kingsford M. J., O’callaghan M., Donelson J. M. (2008). Elevated temperature restricts growth potential of the coral reef fish Acanthochromis polyacanthus. Coral Reefs. 27, 927–931. doi: 10.1007/s00338-008-0393-4
Norin T., Malte H., Clark T. D., Konarzewski M. (2015). Differential plasticity of metabolic rate phenotypes in a tropical fish facing environmental change. Funct. Ecol 30, 369–378. doi: 10.1111/1365-2435.12503
Ojanguren A. F., Reyes-Gavilán F. G., Muñoz R. R. (1999). Effects of temperature on growth and efficiency of yolk utilisation in eggs and pre-feeding larvae stages of Atlantic salmon. Aquac Int. 7, 81–87. doi: 10.1023/A:1009214804949
Okamura A., Yamada Y., Horie N., Utoh T., Mikawa N., Tanaka S., et al. (2007). Effects of water temperature on early development of Japanese eel Anguilla japonica. Fisheries Sci 73, 1241–1248. doi: 10.1111/j.1444-2906.2007.01461.x
Ospina A. F., Mora C. (2004). Effect of body size on reef fish tolerance to extreme low and high temperatures. Environ. Biol. Fish 70, 339–343. doi: 10.1023/b:Ebfi.0000035429.39129.34
Otto R. G. (1973). Temperature tolerance of the mosquitofish, Gambmia affinis (Baird and girard). J. Fish Biol. 5, 575–585. doi: 10.1111/j.1095-8649.1973.tb04490.x
Pauly D., Pullin R. S. V. (1988). Hatching time in spherical, pelagic, marine fish eggs in response to temperature and egg size. Environ. Biol. Fish 22, 261–271. doi: 10.1007/BF00004892
Pavlov D. S., Mikheev V. N., Lupandin A. I., Skorobogatov M. A. (2008). Ecological and behavioural influences on juvenile fish migrations in regulated rivers: A review of experimental and field studies. Hydrobiologia 609, 125–138. doi: 10.1007/s10750-008-9396-y
Recsetar M. S., Zeigler M. P., Ward D. L., Bonar S. A., Caldwell C. A. (2012). Relationship between fish size and upper thermal tolerance. Trans. Am. Fisheries Soc 141, 1433–1438. doi: 10.1080/00028487.2012.694830
Rijnsdorp A. D., Peck M. A., Engelhard G. H., Möllmann C., Pinnegar J. K. (2009). Resolving the effect of climate change on fish populations. ICES J. Mar. Sci 66, 1570–1583. doi: 10.1093/icesjms/fsp056
Rosen M. A. (2001). Energy- and exergy-based comparison of coal-fired and nuclear steam power plants. Exergy Int. J. 1, 180–192. doi: 10.1016/S1164-0235(01)00024-3
Teletchea F., Gardeur J. N., Kamler E., Fontaine P. (2009). The relationship of oocyte diameter and incubation temperature to incubation time in temperate freshwater fish species. J. Fish Biol. 74, 652–668. doi: 10.1111/j.1095-8649.2008.02160.x
Trancart T., Feunteun E., Lefrançois C., Acou A., Boinet C., Carpentier A. (2016). Difference in responses of two coastal species to fluctuating salinities and temperatures: Potential modification of specific distribution areas in the context of global change. Estuarine Coast. Shelf Sci 173, 9–15. doi: 10.1016/j.ecss.2016.02.012
Urquhart A. N., Koetsier P. (2013). ). low-temperature tolerance and critical thermal minimum of the invasive oriental weatherfish Misgurnus anguillicaudatus Idaho, USA. Trans. Am. Fisheries Soc 143, 68–76. doi: 10.1080/00028487.2013.829124
Wernberg T., Smale D. A., Tuya F., Thomsen M. S., Langlois T. J., De Bettignies T., et al. (2012). An extreme climatic event alters marine ecosystem structure in a global biodiversity hotspot. Nat. Climate Change. 3, 78–82. doi: 10.1038/nclimate1627
Wu Y., Yu X., Suo N., Bai H., Ke Q., Chen J., et al. (2022). Thermal tolerance, safety margins and acclimation capacity assessments reveal the climate vulnerability of Large yellow croaker aquaculture. Aquaculture 561:738665. doi: 10.1016/j.aquaculture.2022.738665
Xu Z., Chen J. (2011). Analysis of migratory route of Larimichthys crocea in the East China Sea and yellow Sea. J. Fisheries China. 35, 429–437. doi: 10.3724/SP.J.1231.2011.17099
Xu K., Liu Z. (2007). The current stock of Large yellow croaker Pseudosciaena crocea in the East China Sea with respects of its stock decline. J. Dalian Fisheries Univ 22, 392–396.
Zhang K., Zhang J., Xu Y., Sun M., Chen Z., Yuan M. (2018). Application of a catch-based method for stock assessment of three important fisheries in the East China Sea. Acta Oceanol Sinica. 37, 102–109. doi: 10.1007/s13131-018-1173-9
Keywords: temperature tolerance, Larimichthys crocea, coolant water, heat shock, cold shock
Citation: Tian S, Zhou K, Liao Y, Tang Y, Liu Q, Zhang R, Shou L and Zeng J (2022) Effects of temperature shock on the survival of different life stages of large yellow croaker (Larimichthys crocea) by simulated power plant cooling water. Front. Mar. Sci. 9:1037137. doi: 10.3389/fmars.2022.1037137
Received: 05 September 2022; Accepted: 19 October 2022;
Published: 09 November 2022.
Edited by:
Huang Honghui, South China Sea Fisheries Research Institute (CAFS), ChinaReviewed by:
Anglu Shen, Shanghai Ocean University, ChinaSi Zhu, Ocean University of China, China
Yonghua Jiang, Jimei University, China
Zhiyong Wang, Jimei University, China
Copyright © 2022 Tian, Zhou, Liao, Tang, Liu, Zhang, Shou and Zeng. This is an open-access article distributed under the terms of the Creative Commons Attribution License (CC BY). The use, distribution or reproduction in other forums is permitted, provided the original author(s) and the copyright owner(s) are credited and that the original publication in this journal is cited, in accordance with accepted academic practice. No use, distribution or reproduction is permitted which does not comply with these terms.
*Correspondence: Yibo Liao, bGlhb3liQHNpby5vcmcuY24=
†These authors have contributed equally to this work and share first authorship