- 1Marine Biodiversity and Environmental Assessment Research Center (BioEnv), Research Institute for Global Change (RIGC), Japan Agency for Marine-Earth Science and Technology (JAMSTEC), Yokosuka, Japan
- 2Department of Marine and Earth Sciences, Marine Works Japan Ltd., Yokosuka, Japan
- 3Institute for Extra-cutting-edge Science and Technology Avant-garde Research (X-star), Japan Agency for Marine-Earth Science and Technology (JAMSTEC), Yokosuka, Japan
It is becoming obvious that the abundance of microplastics is increasing in worldwide oceans, raising concerns about their impact on marine ecosystems. Tiny plastic particles enter the body of marine organisms not only via oral ingestion but also through the body surface (e.g., gills or epidermis), but the mechanism of internalization into cells is poorly understood. In this study, we conducted experiments using deep-sea chemosynthetic mussels with limited feeding by exposing their gills to fluorescently labeled microplastic beads. We identified the gill cell types that preferentially internalized the beads and demonstrated the inhibitory effect of phagocytosis inhibitors on bead uptake. Furthermore, using correlative light-electron microscopy, we microhistologically verified that beads were enclosed within membrane-bound vacuoles. Our results indicated that microplastic particles were internalized into gill cells of deep-sea and coastal mussels by phagocytosis. This study highlights the need for further research on plastic contamination via the body surface to conserve the highly endemic and vulnerable deep-sea fauna and mitigate human health risks from consuming coastal bivalves.
Introduction
Plastics are now used in almost all aspects of human life because they are convenient, durable, and inexpensive to produce. However, they are often not properly processed after use and leak into the environment. Most plastics that have leaked into the environment end up in the ocean (Wayman and Niemann, 2021). Furthermore, natural disasters such as tsunamis and typhoons provoke episodic but massive plastic outflow from the land to the ocean (Matsuba et al., 2020; Nakajima et al., 2022). Once in the environment, plastic waste is affected by various environmental conditions, such as waves on the shore and ultraviolet radiation, eventually becoming small plastic particles (Andrady, 2011). In addition, some scrubbing agents are produced with very small plastic particles, most of which flow out into the sea through wastewater (Andrady, 2011). Plastic particles <5 mm in diameter are called microplastics (those <100 nm in diameter are called nanoplastics). As their recovery from the environment is difficult and they do not readily decompose naturally, even at smaller sizes, they are thought to persist in the environment for an extended period (Andrady, 2011; Wayman and Niemann, 2021). In other words, the amount of such microplastics is increasing in the oceans worldwide, raising concerns about their impact on marine ecosystems and human health (Thushari and Senevirathna, 2020).
As microplastics occupy a similar size range as some planktons, suspended particles, and marine sediments, they are potentially bioavailable to many organisms (Weinstein et al., 2019). The microplastics, upon ingestion into the bodies of these organisms, may lead to chemical impacts due to substances contained in or attached to the plastic and physical impacts as foreign substances (Andrady, 2011; Wright et al., 2013). During production, plastics are processed with various additives, such as plasticizers, flame retardants, pigments, antimicrobial agents, heat and UV stabilizers, and fillers, to give them specific properties. As plastics degrade in the environment, the ratio of surface area to volume increases, potentially leading to additive chemicals leaching out (Smith et al., 2018). Furthermore, microplastic particles are characterized by hydrophobicity in addition to the higher surface area to volume ratio, resulting in the adhesion of cooccurring hydrophobic persistent organic pollutants (POPs), some of which are highly toxic and recognized for their endocrine-disrupting, carcinogenic, mutagenic, and immunotoxic effects (Wright and Kelly, 2017; Smith et al., 2018). Thus, microplastics are now considered to act as vectors by sorbing pollutants, contributing to their bioaccumulation, particularly in marine organisms, and subsequently, entire food webs (Amelia et al., 2021). Moreover, microplastics pose various physical risks to marine organisms, such as gastrointestinal blockage, structural deterioration, inflammation, reduced feeding or satiation, inhibition of gastric enzyme secretion, decrease in steroid hormone levels, nutritional or growth complications, delay in ovulation, lack of reproduction, and alteration of metabolic profile (Kolandhasamy et al., 2018; Amelia et al., 2021).
Most studies that reported the abovementioned findings were based on microplastics ingested orally by animals. However, some reports have suggested that micro- and nanoplastics can enter the body through the body surface of marine bivalves (Al-Sid-Cheikh et al., 2018; Kolandhasamy et al., 2018). Therefore, microplastic contamination through means other than oral ingestion should be properly investigated. Bivalve mollusks, which are generally filter feeders, filter large quantities of seawater using their gills, resulting in large amounts of particles in the seawater, including food and microplastics, passing over the surface of the gills and being transported by the wafting of cilia to the digestive system (Inoue et al., 2021a). Some studies examining microplastic uptake in bivalves have presumed cellular internalization by endocytosis of gill epithelial cells as the initial step before the secondary uptake process occurring in the digestive tract (von Moos et al., 2012; Avio et al., 2015; Brate et al., 2018; Pittura et al., 2018). However, no physiological or histological evidence at the cellular level for microplastic uptake by endocytosis in gill cells has been reported.
Deep-sea epibenthic mussels belonging to the genus Bathymodiolus are endemic and dominant members of deep-sea chemosynthetic communities (Fisher, 1990). They harbor symbiotic chemosynthetic bacteria (Gammaproteobacteria) in the epithelial cells of their gills (DeChaine and Cavanaugh, 2006). As organic compounds produced by bacterial symbionts are the primary nutritional source for Bathymodiolus mussels, these mussels perform limited feeding, although they possess a functional gut (Page et al., 1991; Ponnudurai et al., 2017). Recently, polychlorinated biphenyls, persistent chemical pollutants, were detected in deep-sea chemosynthetic bivalves with limited or no filter feeding, including Bathymodiolus mussels, suggesting the possibility of contamination by absorbing organic particles such as pollutant-associated microplastics from the body surface (Ikuta et al., 2021). As the deep sea is a major sink for microplastic debris (Woodall et al., 2014), plastic pollution in deep-sea organisms, even those with nonfeeding or limited feeding habits, must be closely examined. Phagocytosis is a form of endocytosis through which cells internalize particulate matter larger than approximately 0.5 μm in diameter, including microorganisms and foreign solid substances, and is found in almost all cell types of multicellular organisms (Uribe-Querol and Rosales, 2020). A recent study has shown that gill epithelial cells of Bathymodiolus mussels nonselectively phagocytose exogenous bacteria and enclose them in membrane-bound vacuoles called phagosomes (Tame et al., 2022). Therefore, in the present study, we tested the hypothesis that the gill cells of Bathymodiolus mussels incorporate microplastics by phagocytosis. We exposed their gills to fluorescently labeled artificial microplastic beads to identify the cells that took up the beads and examined the effect of reagents known as phagocytosis inhibitors. Furthermore, we sequentially and correlatively performed fluorescence and electron microscopic observations of the fluorescent beads internalized by cells to microhistologically verify whether the beads were enclosed within membrane-bound vacuoles.
Methods
Faunal sampling
Specimens of Bathymodiolus japonicus were collected from the seep site off Hatsushima Island in Sagami Bay, Japan, at depths of 908 m (Dive #HPD2098) and 912 m (Dive #HPD2099) during the cruise KS-20-1 (Jan 07–Jan 11, 2020) using the ROV Hyper-Dolphin, operated by the R/V Shinsei-Maru of the Japan Agency of Marine-Earth Science and Technology (JAMSTEC), and at a depth of 910 m (Dive #6K1596) during the cruise YK21-08C (May 10–May 28, 2021) using the HOV Shinkai 6500, operated by the R/V Yokosuka of JAMSTEC. Before experiments, the collected B. japonicus specimens were kept in chilled seawater at in situ temperature (4°C).
Specimens of the coastal green mussel Perna viridis were collected from a pier in front of the Misaki Marine Biological Station (MMBS), The University of Tokyo, Miura City, Kanagawa, Japan, on Jun 28, 2022. All P. viridis specimens were stored in a stainless-steel tank filled with natural seawater and transported to the laboratory at the headquarters of JAMSTEC (Yokosuka City, Kanagawa, Japan) on the day of collection, where the experiment was conducted.
Exposing fluorescence-labeled plastic beads to gill filaments
The excised gills of B. japonicus are usable for 24 h of incubation, and exposure experiments were conducted as previously described (Tame et al., 2022) with some modifications. To identify the cell types that uptake microplastics in exposure experiments, pieces of the gills (approximately 20 mm in length) of three B. japonicus individuals (shell length 82–98 mm) were excised and incubated with 1 × 108 particles/mL of green-fluorescence labeled polystyrene beads (1 µm diameter; Fluoresbrite YG Microspheres, Polysciences, Warrington, PA, USA) in 6 cm plastic dishes containing 12 mL seawater filtered through a 0.22 μm filter (Nalgene, Rochester, NY, USA) (filtered seawater, FSW). As a previous study observed the phagocytosis of bacteria of approximately 1–2 µm in size by gill cells of B. japonicus (Tame et al., 2022), we selected beads 1 µm in diameter for our experiments. The concentration of beads (1 × 108), much higher than the concentration of plastic particles that can be assumed in the natural environment, was selected to secure substantial observations of internalization and inhibition processes. After 24 h of incubation in the dark at 4°C with gentle shaking, the gills were cut into 3–4 mm wide pieces, rinsed with FSW, transferred into 4% paraformaldehyde in FSW, and stored at 4°C. For correlative light-electron microscopy (CLEM), some pieces of the gills were fixed in 2.5% glutaraldehyde in FSW and stored at 4°C. Before fixation, the gill tissues were confirmed to be alive by observing the movement of cilia of the excised gills. In addition, the excised gills of three P. viridis individuals (shell length 66–70 mm) were incubated with beads as above but for 6 h at 18°C and fixed after confirming that the gill tissues were alive as described above. For negative controls, the gills fixed immediately after dissection in 4% paraformaldehyde for 16 h were incubated with beads and refixed as described above for both B. japonicus and P. viridis specimens.
For exposure experiments with phagocytosis inhibitors, pieces of the gills (approximately 20 mm) of another set of three B. japonicus individuals (shell length 86–98 mm) were incubated not only with beads but also with 0.1 µM wortmannin (Wako Pure Chemical, Osaka, Japan), 0.01 µM wortmannin, 1 µM latrunculin A (Wako Pure Chemical), 0.1 µM latrunculin A, or 0.1% DMSO. The concentration of DMSO, the solvent for wortmannin and latrunculin A, was prepared to be the same in all cultures. After 24 h of incubation in the dark, the gills were fixed and stored as described above.
Detection and counting of beads by microscopic observations
To identify the cell types that uptake microplastic beads, the gills of B. japonicus fixed with paraformaldehyde were embedded in Technovit 8100 resin (Kulzer, Hanau, Germany) and sectioned (2 µm thickness) as previously described (Tame et al., 2022). To avoid duplicate counting of beads in adjacent sections, 11 sections were cut every other section, followed by 11 more after 1 mm spacing, to obtain a total of 66 sections (22 sections × 3 mussels). The gill sections were stained with 1.25 µg/mL propidium iodide (PI; ImmunoChemistry Technologies, Davis, CA, USA) in PBS for 10 min at 24°C, rinsed in PBS, and mounted in ProLong Glass (Thermo Fisher Scientific, Waltham, MA, USA). Images were captured using an Olympus IX73 microscope (Evident, Tokyo, Japan) equipped with an Olympus DP73 camera (Evident). For 3D imaging of the cells that internalized the beads, z-stack images were captured using an A1RMP confocal scanning system (Nikon Instech, Tokyo, Japan) at 0.125 μm intervals and processed using the Nikon NIS-Elements software (Nikon Instech).
To compare the internalization of beads between cell types, images of PI staining (to identify cell nuclei and symbiotic bacteria) and autofluorescence (emission: 561 nm, excitation 595 nm) of gill tissues were simultaneously captured using a Keyence BZ-9000 microscope (Keyence, Osaka, Japan), and the positive region was binarized to determine the area using a BZ-II analyzer (Keyence). The number of beads detected in cells was counted under an Olympus IX73 microscope, and the proportion of beads in each cell type was determined and averaged for three mussels. In addition, the area of the examined gill tissue was multiplied by the thickness of the section (2 μm) to obtain the volume of the gill section; the average volume of gill tissues in each of the three B. japonicus individuals corresponded to 0.040 mm3. For negative control samples, at least 10 sections were examined in each individual, but no bead internalization was observed.
In the phagocytosis inhibition experiment, 10 thin sections (2 µm) were first cut every other section from gill tissue blocks prepared as above, followed by 10 more after 1 mm spacing for a total of 450 sections (30 sections × 5 experimental conditions × 3 mussels). Staining, imaging, and measuring were carried out as above, and the number of beads internalized in the host cell per gill tissue area was determined for each experimental condition, averaged for three mussels, and the ratio to the control condition was calculated. The Tukey–Kramer test (P = 0.05) was performed for statistical analyses using Excel 2011 (Microsoft, Redmond, WA, USA) and the add-on Statcel4 software (OMS publishers, Tokyo, Japan).
The fixed gills of P. viridis were embedded in resin as described above, and 10 thin sections (2 µm) were prepared for each mussel (n = 3). For negative control samples, 10 sections were observed in each individual (n = 3), but no bead internalization was observed.
Correlative light-electron microscopy
CLEM of gill sections of B. japonicus and P. viridis was conducted as previously described (Igawa-Ueda et al., 2021) with brief modifications. Thin sections (2 μm thick) of the gills incubated with beads (see above) were embedded in 0.1% p-phenylenediamine (Merck, Kenilworth, NJ, USA) in 50% glycerol and observed using an Olympus IX73 microscope (Olympus) to detect green-fluorescent signals of beads and autofluorescence (emission: 561 nm, excitation 595 nm) of gill tissues. After washing in PBS, sections were stained with 2.0% uranyl acetate solution for 20 min and 2.0% lead aqueous solution for 5 min at room temperature. Sections were coated with osmium using an OPC-80 osmium coater (Filgen, Nagoya, Japan). The areas examined using fluorescence microscopy were subsequently observed using a Quanta 450 FEG scanning electron microscope (FEI, Tokyo, Japan) with a backscattered electron detector operating at 5 kV. All micrographs were processed with Adobe Photoshop 2022 (Adobe Systems).
Results
Identification of cell types that internalize beads
After incubating the gills with fluorescence-labeled polystyrene beads, we detected some beads in gill cells (Figures 1A–P; Supplementary Figure 1). To compare the internalization of beads between cell types, we separated gill cells into frontal cells, bacteriocytes harboring methane-oxidizing symbionts, asymbiotic intercalary cells, cells in ciliated junction, secretory cells with mucus globules, abfrontal cells, and hemocytes (Fiala-Medioni et al., 1986; Raulfs et al., 2004), and examined the percentage of these cells that had internalized beads. We observed that beads were present in frontal cells, asymbiotic intercalary cells, cells in ciliated junction, abfrontal cells, and hemocytes, but not in bacteriocytes and secretory cells. In particular, we found that frontal cells had the highest percentage of bead uptake, with a mean value of 57.3%; however, the percentage of bead internalization among the other cells did not differ significantly (Figure 1Q; Supplementary Table 1). At the concentration of exposure used in this study, we calculated that an average of 1.19 × 106 beads per cm3 of gill tissue were incorporated into gill cells within 24 h.
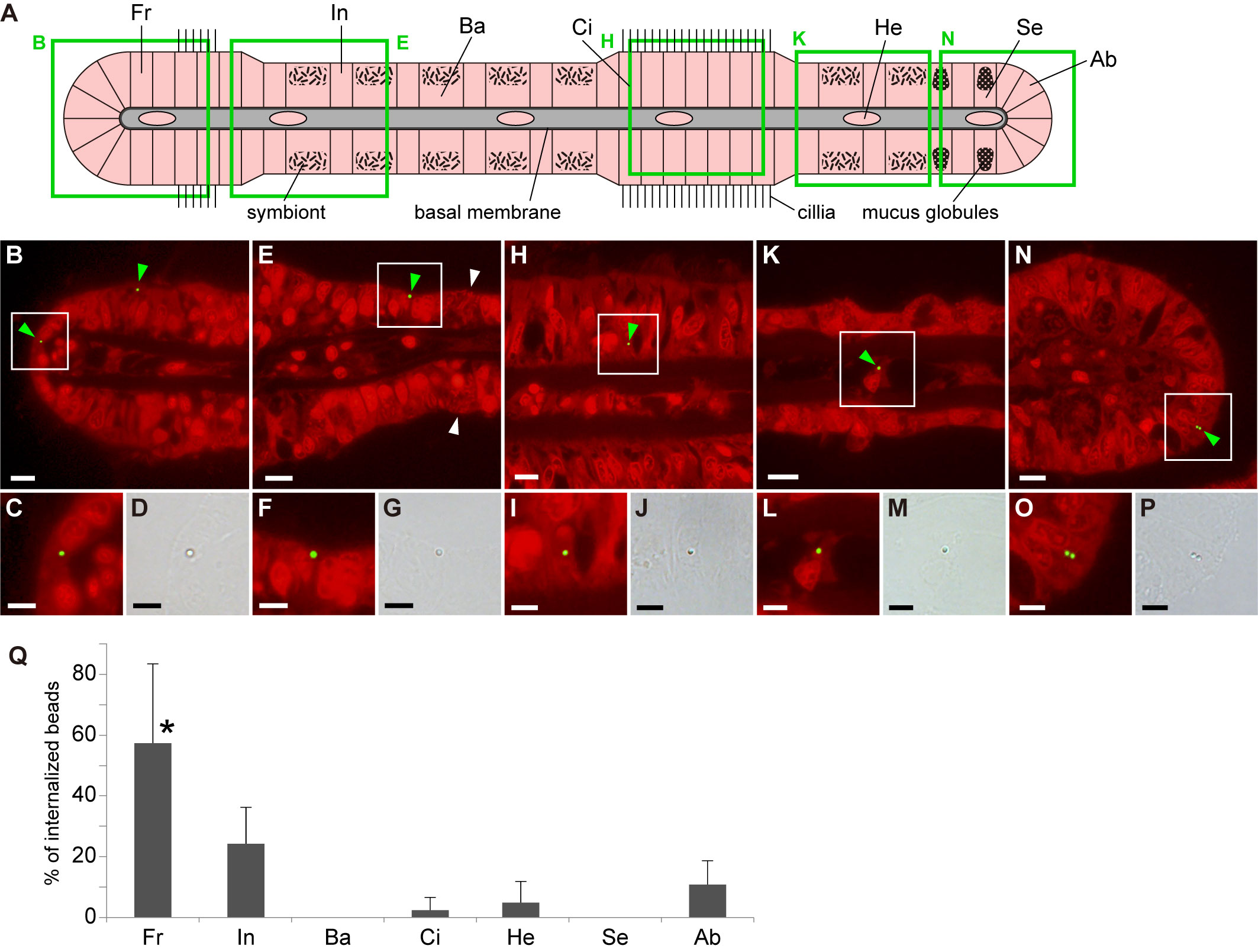
Figure 1 Distribution of internalized beads in various gill cell types of Bathymodiolus japonicus. (A) Schematic representation of a transverse section of a gill filament showing different cell types. Fr, frontal cell; In, asymbiotic intercalary cell; Ba, bacteriocytes harboring symbiotic bacteria; Ci, cell in ciliated junction; He, hemocyte; Se, secretory cell with mucus globules; Ab, abfrontal cell. Green squares indicate the areas shown in (B, E, H, K, N). (B–P) Fluorescent and bright-field micrographs showing the internalization of beads into the five cell types. In fluorescence images, propidium iodide-stained red fluorescence (strong signals from nuclei and symbiotic bacteria and weak signal from cytoplasm) and bead-stained green fluorescence were merged. Green and white arrowheads indicate internalized beads and symbiotic bacteria, respectively. White squares in (B, E, H, K, N) correspond to the locations of the magnified fluorescence and brightfield images shown below them. Scale bars: 10 µm in (B, E, H, K, N); 5 µm in (C, D, F, G, I, J, L, M, O, P). (Q) Average proportion of the number of beads internalized in each cell type per total number of internalized beads. Asterisk indicates a significant difference among cell types (P < 0.05), with error bars representing standard deviation (n = 3).
Effect of phagocytosis inhibitors on bead internalization
To investigate whether the internalization of beads was due to phagocytosis, we incubated the gills and beads in seawater containing two phagocytosis inhibitors (wortmannin and latrunculin A) at different concentrations and measured the number of beads internalized by cells (Supplementary Table 2). Both 0.1 and 0.01 µM wortmannin significantly inhibited bead uptake compared with that in the control group (solvent DMSO only) (Figure 2A); however, the results for 0.01 and 0.1 µM wortmannin did not differ significantly (Figure 2A). In addition, latrunculin A (1 µM) significantly inhibited bead uptake compared with the control (Figure 2B). However, no significant differences between the control and 0.1 µM latrunculin A or between 0.1 and 1 µM latrunculin A were observed (Figure 2B).
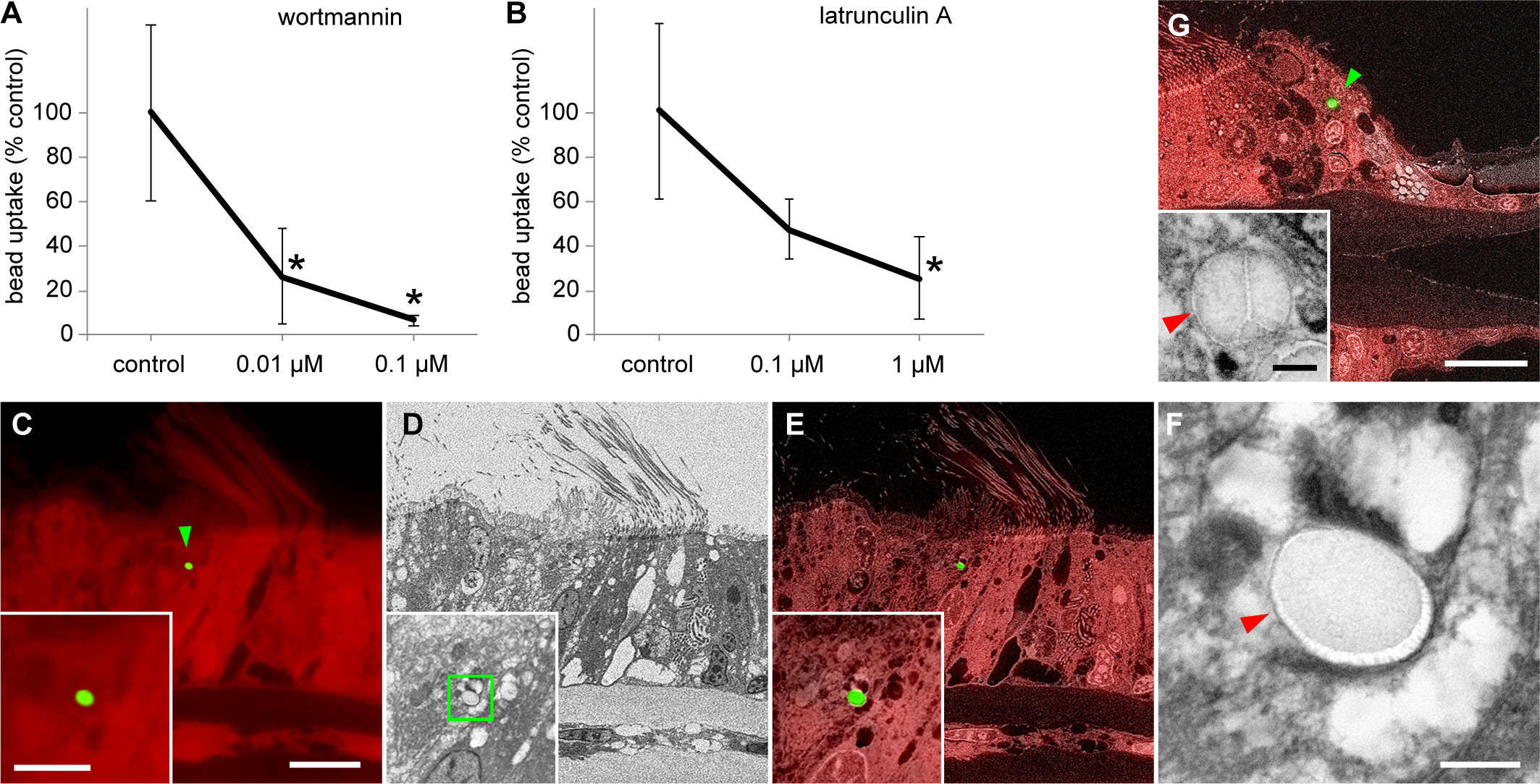
Figure 2 Cellular physiological and microhistological verification of phagocytosis of beads by gill cells. (A, B) Effects of wortmannin (A) and latrunculin A (B) on bead uptake by gill cells. After 24 h of bead exposure and simultaneous treatment with various concentrations of inhibitors, the number of beads internalized into cells was compared with that in control. Asterisks indicate a significant difference versus the control (P < 0.05), with error bars representing standard deviation (n = 3). (C–G) Correlative observations of the beads internalized into gill cells using optical and scanning electron microscopy (SEM) with a backscattered electron detector; green arrowheads indicate internalized beads. (C–F) Bathymodiolus japonicus, (G) Perna viridis. (C) Red autofluorescence of gill tissues of B. japonicus and green fluorescent signal of a bead (1 µm). (D) SEM image of the same area as (C). (E) Merged image of fluorescence microscopy and SEM. Insets in (C–E) show a higher magnification of the bead in the same area. (F) Magnified image that corresponds to the area indicated by the green square in the inset in (D) The bead is enclosed within a membrane-bound vacuole (red arrowhead). (G) Merged image of red autofluorescence of gill tissues of P. viridis and green fluorescent signal of a bead, and SEM image of the same area. The inset shows a magnified SEM image of this bead. The bead is enclosed within a membrane-bound vacuole (red arrowhead) in a frontal cell. Scale bars: 10 µm in (C) (applicable to D and E) and (G); 5 µm in inset in (C) [applicable to insets in (D, E)]; 0.5 µm in (F) and inset in (G).
Microhistological observation of beads enclosed within phagosomes
We performed detailed histological observations to verify that beads were internalized by gill cells through phagocytosis. The areas where beads were detected by fluorescence observation were subsequently examined by electron microscopy, which revealed that beads were enclosed within membrane-bound vacuoles in gill epithelial cells (Figures 2C–F). To check the generality of this phenomenon, we conducted a similar bead exposure experiment in the coastal green mussel P. viridis. Similar to the results in B. japonicus, the beads were enclosed within membrane-bound vacuoles in the gill epithelial cells of P. viridis (Figure 2G).
Discussion
This study showed that two different phagocytosis inhibitors inhibited the internalization of fluorescence-labeled polystyrene beads by the gill cells of the deep-sea chemosynthetic bivalve B. japonicus. Although the concentration dependence of the effects of inhibitors was not statistically clear, we still observed downward-sloping curves with higher concentrations of inhibitors. Wortmannin is a cell-permeable, potent inhibitor of phosphoinositide 3-kinase (PI3K) at nanomolar concentrations without significant interference to other signal transduction pathways (Wymann et al., 1996). In mammalian systems, PI3K regulates phagocytosis by modulating pseudopod extension and endoplasmic reticulum-dependent phagosome formation and activating many downstream signaling molecules. All phagocytic systems require PI3K activity to accomplish particle internalization (Rosales, 2005). Latrunculin A is a potent inhibitor of phagocytosis. It is a natural toxin molecule that binds to actin monomers and inhibits their polymerization, whose reorganization is required for phagocytosis (de Oliveira and Mantovani, 1988). Our results using these reagents indicated that the uptake of beads by the gill cells of B. japonicus was due to phagocytosis. Furthermore, using CLEM, we demonstrated that the beads internalized into cells were enclosed within membrane-bound vacuoles. In the case of tracking experiments using fluorescently labeled particles, concerns about artifacts caused by fluorescence leakage and the low resolution of optical microscopy have been pointed out (Triebskorn et al., 2019), however, using CLEM solves these problems. Phagocytosis is the process by which the cell uses its plasma membrane to ingest large particles, creating a membrane-bound vesicle called a phagosome (Vechetti et al., 2021). Our CLEM observations, therefore, have provided essential evidence to conclude that beads were internalized by phagocytosis.
Regarding the microplastics that enter the gastrointestinal tract via oral ingestion, a phenomenon called “translocation” has been reported in a variety of animals, suggesting that they pass through the gut epithelium and enter the circulatory system and other organs (Triebskorn et al., 2019). In mammals, translocation across the gut epithelium of various types and sizes of microparticles including microplastics has been demonstrated, with M-cells (microfold cells) in the Peyer’s patches of the ileum being considered the predominant site of uptake of particles by phagocytosis (Van Cauwenberghe and Janssen, 2014) and a route of entry into the lymphatic system or blood supply (Triebskorn et al., 2019). In the coastal mussel Mytilus edulis, ingested microplastics (3–9.6 µm) are accumulated in digestive tissues and translocated to the hemolymph (Browne et al., 2007). However, invertebrates do not have Peyer’s patches, and thus the findings in mammals cannot be easily adapted to invertebrates. In fact, very little is known about the mechanisms of microplastic uptake in the gastrointestinal tract and from the body surface, including the gills, in invertebrates. In this study, we showed that the exposed fluorescent beads (1 µm) were preferentially phagocytosed by the frontal cells of the gills of B. japonicus, followed by asymbiotic intercalary cells, cells in ciliated junction, abfrontal cells, and hemocytes but not by bacteriocytes and secretory cells. We observed that fluorescent beads were taken up in some hemocytes. The uptake of beads by the gill epithelial cells indicates that they could be one of the translocation routes into the circulatory system in the mussels, although the thick basal membrane exists on the basal side of the gill epithelium. Information on this mechanism is very limited in invertebrates; therefore, further detailed studies are necessary. However, as our study used excised gills, the possibility that beads entered the circulatory system through the amputation site during exposure experiments cannot be completely ruled out.
In contrast to our results, a recent study using the B. japonicus gills showed that experimentally exposed bacteria of approximately the same size as the beads used in this study were preferentially phagocytosed by bacteriocytes and asymbiotic intercalary cells, with frontal and abfrontal cells exhibiting significantly lower percentages of internalization, irrespective of bacterial type and viability status (live or dead) (Tame et al., 2022). These results suggested differences in the recognition between plastics and bacteria in the gills of B. japonicus and revealed some selectivity, albeit likely loose, for the phagocytosis of targets depending on cell type. In vertebrate systems, the phagocytosis process depends on the surface state of the target particle, that is, opsonization by antibodies or complement, and other physicochemical properties such as hydrophobicity, electric charge, and some molecular motifs on the microbial pathogen surface (Wright and Kelly, 2017; Uribe-Querol and Rosales, 2020). In the bivalve pacific oyster Crassostrea gigas, which has no antibodies or complement, hemocytes have been shown to exhibit phagocytic activity against latex beads, but its recognition mechanism remains unclear (Takahashi and Mori, 2000). In addition, particle size has also been reported to be a crucial factor for internalization (Triebskorn et al., 2019). A study reporting the translocation of 24 nm-sized nanoplastic particles through the body surface in scallops suggested the possibility of diffusion through the lipid bilayer (Rossi et al., 2014; Al-Sid-Cheikh et al., 2018). Future studies using various particle materials and sizes will be required to better understand the mechanisms of plastic uptake through the body surface in deep-sea bivalves.
It is expected that the amount of plastics in the ocean will continue to increase, which will be degraded and become finer and finer, leading to increased abundance of fine plastic particles in the environment (Wayman and Niemann, 2021). Considering that the lifespan of deep-sea mussels is estimated to be close to 20 years (Nedoncelle et al., 2013; Nedoncelle et al., 2015), the accumulation of plastic particle contamination by uptake through the body surface, as shown in this study, cannot be overlooked. Although the fate of plastic particles internalized into cells on the body surface is currently unknown, they may have chemical, physical, and physiological effects on the organisms that have absorbed them. In this study, B. japonicus was collected from Sagami Bay, which is located near a highly populated region that includes Tokyo (SBJ, 2020; Nakajima et al., 2021). In addition to the seas around Japan, hydrothermal vents and seeps with chemosynthetic communities are distributed near relatively densely populated areas, such as Monterey Bay, the Tyrrhenian Sea, and the Gulf of Mexico (German et al., 2011). To the best of our knowledge, no reports have been published on the concentration of microplastics in seawater at these deep-sea habitat sites. However, the present study suggested that highly endemic and vulnerable organisms living in such areas near dense human activities might be affected by plastic pollution, even if they are nonfeeding or have limited feeding activity. Additionally, we confirmed that fluorescent beads internalized by gill cells were enclosed within membrane-bound vacuoles also in P. viridis, providing strong evidence that microplastics are phagocytized via the surface of gill cells in coastal mussels. Coastal mussels have been the subject of environmental pollution studies as their body composition reflects environmental conditions and they are popular seafood that are consumed whole (Inoue et al., 2021b). In ascidians, another marine filter-feeding organism, fluorescent beads given experimentally were translocated from the gut to the circulatory system (Messinetti et al., 2019). Ascidians are also consumed as food in several countries. It is possible that these organisms may also take up plastic particles from their gills and other body surfaces. Therefore, the potential to vector microplastics to humans via contamination through the body surfaces of aquatic organisms would need to be examined more closely in the future.
In conclusion, this study showed that microplastic particles are phagocytosed in the gill cells of deep-sea mussels. Although the experimental conditions of this study did not allow us to directly infer the actual contamination situation at the habitat site, we have provided important insights that can serve as a basis for future studies. It will also be important to investigate the relationship between recently reported POP contamination in deep-sea nonfeeding or limited feeding bivalves collected at the same site (Ikuta et al., 2021) and plastic contamination via the body surface. Our study highlighted the need for further detailed investigations to address plastic contamination in deep-sea habitats, including that of nonfeeding or limited feeding chemosynthetic animals in order to protect these highly endemic and vulnerable deep-sea ecosystems. In addition, the study highlighted microplastic uptake from the body surface as a contamination pathway in coastal organisms.
Data availability statement
The original contributions presented in the study are included in the article/Supplementary Material. Further inquiries can be directed to the corresponding author.
Author contributions
TI: Conceptualization, Methodology, Formal analysis, Investigation, Writing - Original Draft, Visualization. AT: Methodology, Investigation, Writing - Review and Editing. TT: Investigation. HN: Resources, Writing - Review and Editing. RN: Supervision, Writing - Review and Editing. All authors contributed to the article and approved the submitted version.
Funding
This work was supported by the New Energy and Industrial Technology Development Organization (NEDO) under Project JPNP18016.
Acknowledgments
We are grateful to Dr Chong Chen (JAMSTEC) and the captains and crew of the V Shinsei-Maru and R/V Yokosuka, as well as the operation teams of ROV Hyper Dolphin and HOV Shinkai 6500, for their assistance with sample collection. We thank Mr. Hisanori Kohtsuka and the staff at MMBS for the sample collection. We also thank Editage [http://www.editage.com] for editing and reviewing this manuscript for English language
Conflict of interest
Author AT is employed by Marine Works Japan, Ltd.
The remaining authors declare that the research was conducted in the absence of any commercial or financial relationships that could be construed as a potential conflict of interest.
Publisher’s note
All claims expressed in this article are solely those of the authors and do not necessarily represent those of their affiliated organizations, or those of the publisher, the editors and the reviewers. Any product that may be evaluated in this article, or claim that may be made by its manufacturer, is not guaranteed or endorsed by the publisher.
Supplementary material
The Supplementary Material for this article can be found online at: https://www.frontiersin.org/articles/10.3389/fmars.2022.1034950/full#supplementary-material
References
Al-Sid-Cheikh M., Rowland S. J., Stevenson K., Rouleau C., Henry T. B., Thompson R. C. (2018). Uptake, whole-body distribution, and depuration of nanoplastics by the scallop pecten maximus at environmentally realistic concentrations. Environ. Sci. Technol. 52, 14480–14486. doi: 10.1021/acs.est.8b05266
Amelia T. S. M., Khalik W. M. A. W. M., Ong M. C., Shao Y. T., Pan H.-J., Bhubalan K. (2021). Marine microplastics as vectors of major ocean pollutants and its hazards to the marine ecosystem and humans. Prog. Earth Planet. Sci. 8, 12. doi: 10.1186/s40645-020-00405-4
Andrady A. L. (2011). Microplastics in the marine environment. Mar. pollut. Bull. 62, 1596–1605. doi: 10.1016/j.marpolbul.2011.05.030
Avio C. G., Gorbi S., Milan M., Benedetti M., Fattorini D., d’Errico G., et al. (2015). Pollutants bioavailability and toxicological risk from microplastics to marine mussels. Environ. Pollut. 198, 211–222. doi: 10.1016/j.envpol.2014.12.021
Brate I. L. N., Blazquez M., Brooks S. J., Thomas K. V. (2018). Weathering impacts the uptake of polyethylene microparticles from toothpaste in Mediterranean mussels (M. galloprovincialis). Sci. Total Environ. 626, 1310–1318. doi: 10.1016/j.scitotenv.2018.01.141
Browne M. A., Galloway T., Thompson R. (2007). Microplastic–an emerging contaminant of potential concern? Integr. Environ. Assess. Manage. 3, 559–561. doi: 10.1002/ieam.5630030412
DeChaine E. G., Cavanaugh C. M. (2006). Symbioses of methanotrophs and deep-sea mussels (Mytilidae: Bathymodiolinae). Prog. Mol. Subcell. Biol. 41, 227–249. doi: 10.1007/3-540-28221-1_11
de Oliveira C. A., Mantovani B. (1988). Latrunculin a is a potent inhibitor of phagocytosis by macrophages. Life Sci. 43, 1825–1830. doi: 10.1016/0024-3205(88)90282-2
Fiala-Medioni A., Metivier C., Herry A., Le Pennec M. (1986). Ultrastructure of the gill of the hydrothermal-vent mytilid Bathymodiolus sp. Mar. Biol. 92, 65–72. doi: 10.1007/Bf00392747
Fisher C. R. (1990). Chemoautotrophic and methanotrophic symbioses in marine invertebrates. Rev. Aquat. Sci. 2, 399–436.
German C. R., Ramirez-Llodra E., Baker M. C., Tyler P. A., ChEss Scientific Steering C. (2011). Deep-water chemosynthetic ecosystem research during the census of marine life decade and beyond: A proposed deep-ocean road map. PloS One 6, e23259. doi: 10.1371/journal.pone.0023259
Igawa-Ueda K., Ikuta T., Tame A., Yamaguchi K., Shigenobu S., Hongo Y., et al. (2021). Symbiont transmission onto the cell surface of early oocytes in the deep-sea clam Phreagena okutanii. Zool. Sci. 38, 140–147. doi: 10.2108/zs200129
Ikuta T., Nakajima R., Tsuchiya M., Chiba S., Fujikura K. (2021). Interdecadal distribution of persistent organic pollutants in deep-sea chemosynthetic bivalves. Front. Mar. Sci. 8, 1735. doi: 10.3389/fmars.2021.751848
Inoue K., Onitsuka Y., Koito T. (2021a). Mussel biology: from the byssus to ecology and physiology, including microplastic ingestion and deep-sea adaptations. Fish. Sci. 87, 761–771. doi: 10.1007/s12562-021-01550-5
Inoue K., Yoshioka Y., Tanaka H., Kinjo A., Sassa M., Ueda I., et al. (2021b). Genomics and transcriptomics of the green mussel explain the durability of its byssus. Sci. Rep. 11, 5992. doi: 10.1038/s41598-021-84948-6
Kolandhasamy P., Su L., Li J. N., Qu X. Y., Jabeen K., Shi H. H. (2018). Adherence of microplastics to soft tissue of mussels: A novel way to uptake microplastics beyond ingestion. Sci. Total Environ. 610–611, 635–640. doi: 10.1016/j.scitotenv.2017.08.053
Matsuba M., Tanaka Y., Yamakita T., Ishikawa Y., Fujikura K. (2020). Estimation of tsunami debris on seafloors towards future disaster preparedness: Unveiling spatial varying effects of combined land use and oceanographic factors. Mar. Pollut. Bull. 157, 111289. doi: 10.1016/j.marpolbul.2020.111289
Messinetti S., Mercurio S., Scari G., Pennati A., Pennati R. (2019). Ingested microscopic plastics translocate from the gut cavity of juveniles of the ascidian Ciona Intestinalis. Eur. Zool J. 86, 189–195. doi: 10.1080/24750263.2019.1616837
Nakajima R., Miyama T., Kitahashi T., Isobe N., Nagano Y., Ikuta T., et al. (2022). Plastic after an extreme storm: The typhoon-induced response of micro- and mesoplastics in coastal waters. Front. Mar. Sci. 8. doi: 10.3389/fmars.2021.806952
Nakajima R., Tsuchiya M., Yabuki A., Masuda S., Kitahashi T., Nagano Y., et al. (2021). Massive occurrence of benthic plastic debris at the abyssal seafloor beneath the Kuroshio Extension, the North West Pacific. Mar. Pollut. Bull. 166, 112188. doi: 10.1016/j.marpolbul.2021.112188
Nedoncelle K., Lartaud F., Contreira Pereira L., Yücel M., Thurnherr A. M., Mullineaux L., et al. (2015). Bathymodiolus growth dynamics in relation to environmental fluctuations in vent habitats. Deep Sea Res. 106, 183–193. doi: 10.1016/j.dsr.2015.10.003
Nedoncelle K., Lartaud F., de Rafelis M., Boulila S., Le Bris N. (2013). A new method for high-resolution bivalve growth rate studies in hydrothermal environments. Mar. Biol. 160, 1427–1439. doi: 10.1007/s00227-013-2195-7
Page H. M., Fiala-Medioni A., Fisher C. R., Childress J. J. (1991). Experimental evidence for filter-feeding by the hydrothermal vent mussel, Bathymodiolus thermophilus. Deep Sea Res. 38, 1455–1461. doi: 10.1016/0198-0149(91)90084-S
Pittura L., Avio C. G., Giuliani M. E., d’Errico G., Keiter S. H., Cormier B., et al. (2018). Microplastics as vehicles of environmental PAHs to marine organisms: Combined chemical and physical hazards to the Mediterranean mussels, Mytilus galloprovincialis. Front. Mar. Sci. 5. doi: 10.3389/fmars.2018.00103
Ponnudurai R., Kleiner M., Sayavedra L., Petersen J. M., Moche M., Otto A., et al. (2017). Metabolic and physiological interdependencies in the Bathymodiolus azoricus symbiosis. ISME J. 11, 463–477. doi: 10.1038/ismej.2016.124
Raulfs E. C., Macko S. A., Van Dover C. L. (2004). Tissue and symbiont condition of mussels (Bathymodiolus thermophilus) exposed to varying levels of hydrothermal activity. J. Mar. Biol. Assoc. U.K. 84, 229–234. doi: 10.1017/S0025315404009087h
Rossi G., Barnoud J., Monticelli L. (2014). Polystyrene nanoparticles perturb lipid membranes. J. Phys. Chem. Lett. 5, 241–246. doi: 10.1021/jz402234c
Smith M., Love D. C., Rochman C. M., Neff R. A. (2018). Microplastics in seafood and the implications for human health. Curr. Environ. Health Rep. 5, 375–386. doi: 10.1007/s40572-018-0206-z
Takahashi K. G., Mori K. (2000). Functional profiles of hemocytes in the bio-defense process of the pacific oyster, Crassostrea gigas. Tohoku J. Agric. Res. 51, 15–27.
Tame A., Maruyama T., Yoshida T. (2022). Phagocytosis of exogenous bacteria by gill epithelial cells in the deep-sea symbiotic mussel Bathymodiolus japonicus. R. Soc Open Sci. 9, 211384. doi: 10.1098/rsos.211384
Thushari G. G. N., Senevirathna J. D. M. (2020). Plastic pollution in the marine environment. Heliyon 6, e04709. doi: 10.1016/j.heliyon.2020.e04709
Triebskorn R., Braunbeck T., Grummt T., Hanslik L., Huppertsberg S., Jekel M., et al. (2019). Relevance of nano- and microplastics for freshwater ecosystems: A critical review. TrAC - Trends Anal. Chem. 110, 375–392. doi: 10.1016/j.trac.2018.11.023
Uribe-Querol E., Rosales C. (2020). Phagocytosis: Our current understanding of a universal biological process. Front. Immunol. 11. 1066 doi: 10.3389/fimmu.2020.01066
Van Cauwenberghe L., Janssen C. R. (2014). Microplastics in bivalves cultured for human consumption. Environ. Pollut. 193, 65–70. doi: 10.1016/j.envpol.2014.06.010
Vechetti I. J. Jr., Valentino T., Mobley C. B., McCarthy J. J. (2021). The role of extracellular vesicles in skeletal muscle and systematic adaptation to exercise. J. Physiol. 599, 845–861. doi: 10.1113/JP278929
von Moos N., Burkhardt-Holm P., Kohler A. (2012). Uptake and effects of microplastics on cells and tissue of the blue mussel Mytilus edulis l. after an experimental exposure. Environ. Sci. Technol. 46, 11327–11335. doi: 10.1021/es302332w
Wayman C., Niemann H. (2021). The fate of plastic in the ocean environment - a minireview. Environ. Sci.: Process. Impacts 23, 198–212. doi: 10.1039/d0em00446d
Weinstein J. E., Viado H. F., Leads R. R., Deloughry E., Schandera L., Liddy K., et al. (2019). “Microplastics: A global water pollution problem,” in Encyclopedia of water: Science, technology, and society, vol. 447–462. (Hoboken: Wiley).
Woodall L. C., Sanchez-Vidal A., Canals M., Paterson G. L., Coppock R., Sleight V., et al. (2014). The deep sea is a major sink for microplastic debris. R. Soc Open Sci. 1, 140317. doi: 10.1098/rsos.140317
Wright S. L., Kelly F. J. (2017). Plastic and human health: A micro issue? Environ. Sci. Technol. 51, 6634–6647. doi: 10.1021/acs.est.7b00423
Wright S. L., Thompson R. C., Galloway T. S. (2013). The physical impacts of microplastics on marine organisms: A review. Environ. pollut. 178, 483–492. doi: 10.1016/j.envpol.2013.02.031
Wymann M. P., Bulgarelli-Leva G., Zvelebil M. J., Pirola L., Vanhaesebroeck B., Waterfield M. D., et al. (1996). Wortmannin inactivates phosphoinositide 3-kinase by covalent modification of lys-802, a residue involved in the phosphate transfer reaction. Mol. Cell. Biol. 16, 1722–1733. doi: 10.1128/MCB.16.4.1722
Keywords: microplastic, phagocytosis, gill epithelial cell, deep-sea mussel, coastal mussel, correlative light-electron microscopy
Citation: Ikuta T, Tame A, Takahashi T, Nomaki H and Nakajima R (2022) Microplastic particles are phagocytosed in gill cells of deep-sea and coastal mussels. Front. Mar. Sci. 9:1034950. doi: 10.3389/fmars.2022.1034950
Received: 02 September 2022; Accepted: 04 October 2022;
Published: 26 October 2022.
Edited by:
Carlo Pretti, University of Pisa, ItalyReviewed by:
Camilla Della Torre, University of Milan, ItalyMatteo Oliva, Centro Interuniversitario di Biologia Marina ed Ecologia Applicata “G. Bacci” di Livorno (CIBM), Italy
Copyright © 2022 Ikuta, Tame, Takahashi, Nomaki and Nakajima. This is an open-access article distributed under the terms of the Creative Commons Attribution License (CC BY). The use, distribution or reproduction in other forums is permitted, provided the original author(s) and the copyright owner(s) are credited and that the original publication in this journal is cited, in accordance with accepted academic practice. No use, distribution or reproduction is permitted which does not comply with these terms.
*Correspondence: Tetsuro Ikuta, dGVpa3V0YUBqYW1zdGVjLmdvLmpw