- 1College of Environmental Science and Engineering, Ocean University of China, Qingdao, China
- 2Key Laboratory of Marine Environment and Ecology, Ocean University of China, Ministry of Education, Qingdao, China
The neurotoxin β-N-methylamino-L-alanine (BMAA) produced by cyanobacteria and diatoms can accumulate in diverse aquatic organisms through the food web. In the present study, embryos of mussel Mytilus galloprovincialis (Lamarck, 1819), oyster Magallana gigas (Thunberg, 1793), and marine medaka Oryzias melastigma (McClelland, 1839) were exposed to BMAA dissolved in seawater and monitored for early developmental effects. Results demonstrated that the embryonic development of mussels and oysters were significantly inhibited when BMAA concentrations were above 100 μg BMAA·HCl/L (0.65 µM) and 800 μg BMAA·HCl/L (5.18 µM), respectively. The shell growth of mussel embryos was also markedly inhibited by BMAA ≥ 100 μg BMAA·HCl/L (0.65 µM). Based on the dose-response curves related to the modified malformation rate of embryos, the median effective concentration (EC50) values of mussel (48 h) and oyster (24 h) embryos were 196 μg BMAA·HCl/L (1.27 µM) and 1660 μg BMAA·HCl/L (10.7 μM), respectively. A sustained and dose-dependent decrease in heart rate was apparent in marine medaka embryos at 9-days post fertilization following BMAA exposure. However, no obvious effect on ATP concentration was noted in these marine medaka embryos. The current study contributes to our understanding of the sublethal effects of BMAA on the early embryonic development of marine bivalves and medaka. Further research examining the long-term effects of BMAA on the early development of marine organisms is necessary to determine seawater quality criteria for protection.
Highlights
● Effect of BMAA on embryonic development of marine shellfish and fish were eplored.
● The 48 h-EC50 for the inhibition of embryonic development of mussels was 1.27 µM.
● The 24 h-EC50 for the inhibition of embryonic development of oysters was 10.7 µM.
● Heart rate of medaka embryos was dose-dependent and reduced by BMAA at 9 DPF.
● No obvious effect on the ATP content was found due to BMAA in marine medaka embryos.
1. Introduction
The non-protein amino acid β-N-methylamino-L-alanine (BMAA) is linked to the high incidence of Amyotrophic Lateral Sclerosis/Parkinsonism Dementia Complex (ALS/PDC) in Guam (Cox et al., 2016), and the adverse effects and toxicological mechanisms of BMAA have been considered (Delcourt et al., 2018; Dunlop et al., 2021). The establishment of animal models to evaluate the neurotoxicity of BMAA has been fundamental to our understanding of human risk. Various in vivo studies on non-human primates, chicks, rats, and mice document neurodegenerative symptoms including ataxia and convulsions, as well as the formation of neurofibrillary tangles, β-amyloid plaques, ALS-related proteinopathy, and microglia activation as a result of BMAA exposure (Chiu et al., 2011; Cox et al., 2016; Davis et al., 2020). Furthermore, in vitro studies suggest that BMAA can selectively damage motor neurons through multiple mechanisms including excitotoxicity mediated by the carbamate adduct (Rao et al., 2006; Lopicic et al., 2009). In recent years, multiple studies have examined the adverse effects of BMAA on different aquatic organisms. For example, BMAA inhibited the oxidative stress defense and biotransformation enzymes of Daphnia magna (Straus, 1820) (Esterhuizen-Londt et al., 2015), altered phototaxis in brine shrimp Artemia salina (Linnaeus, 1758) (Purdie et al., 2009a), and caused abnormal spinal axis formation and some degree short-term learning and memory deficit in zebrafish Danio rerio (Hamilton, 1822) (Purdie et al., 2009b; Wang et al., 2020a). The combined evidence of these animal studies suggests that BMAA causes disease consistent with ALS/PDC.
Multiple toxicological mechanisms for BMAA neurotoxicity have been proposed. The role of BMAA as a glutamate receptor agonist has been deemed a key toxicological mechanism affecting motor neurons. In humans, an over-stimulation of glutamate and glutamatergic pathways may contribute to the development of various neurodegenerative diseases such as Alzheimer’s disease, Parkinson’s disease, and Huntington’s disease (Salińska et al., 2005). BMAA has been shown to react with bicarbonate to produce a β-carbamate that, in turn, acts as an agonist due to its structural homology to glutamate (Weiss and Choi, 1988). Most glutamate receptors including NMDA, AMPA/kainate, and metabotropic receptors are sensitive to the β-carbamate of BMAA (Rao et al., 2006; Lopicic et al., 2009; Chiu et al., 2012). Subsequently, an increase in intracellular Ca2+ further damages the mitochondria of neurons, promotes endoplasmic reticulum stress, and induces cell apoptosis (Delcourt et al., 2018). In addition, the competition of BMAA with cystine at the cystine/glutamate antiporter (system Xc-) could also lead to glutathione depletion and aggravate oxidative stress (Albano and Lobner, 2018). In a rat model, neonatal exposure to BMAA increased protein ubiquitination in the cornus ammonis 1 region of the adult hippocampus indicating that BMAA may induce protein aggregation (Hanrieder et al., 2014).
The ubiquity of BMAA in marine seafood products increases the potential for human exposure to this neurotoxin (Lance et al., 2018; Li et al., 2018; Wang et al., 2021). Further, the health risk of BMAA to marine fauna is poorly documented. The embryonic development and early life stages (ELS) of mollusks and fish may be influenced by environmental BMAA released through diatom metabolism or from the broken cells of BMAA producers. It is important to assess the risk of BMAA exposure to marine animals in order to understand the risk to aquatic ecosystems.
The early life stages of marine bivalves have been used to develop toxicity bioassay models and assess the ecological effects of marine pollutants due to the transparency of embryo and larva, fast development, high-throughput screening format, and their sensitivity to contaminants (Capela et al., 2020). Mussels and oysters are commonly used as model organisms in marine/brackish ecotoxicology due to their economic and ecological significance for the near-shore ecosystem. In addition, the ELS of fish, such as marine medaka Oryzias melastigma, have been used as biologically significant endpoints to evaluate the effects of pollutants on aquatic teleosts (Wang et al., 2020b).
In this study, embryos of mussel (Mytilus galloprovincialis), Pacific oyster (Magallana gigas), and marine medaka (O. melastigma) were used to assess the ecotoxicological effects of the neurotoxin BMAA through exposure to dissolved toxin in seawater. The rate of development, malformation ratio, and heart rate were recorded along with morphological observations to evaluate the toxicological mechanisms of BMAA.
2. Materials and methods
2.1. Chemicals
Standard reference material of L-BMAA hydrochloride (B107, 50 mg) was obtained from Sigma-Aldrich (Oakville, ON, Canada). Hydrochloric acid (HCl) was obtained from Sinopharm Chemical Reagent. The BMAA·HCl was dissolved in 2 mM HCl at 5 g/L to create a stock solution. The assay kit for ATP concentration was obtained from Solarbio (Beijing, China). The water used in these experiments was purified by a Milli-Q ultrapure water system (Millipore SAS, Molsheim, France) to 18.2 MΩ cm.
2.2. Animal gametes and fertilization
Sexually mature mussels (M. galloprovincialis) obtained from a local breeding farm in Qingdao, Shandong Province, China, between March and May 2020, were transferred to the laboratory and acclimatized in tanks containing natural seawater for 48 h. The collection of mussel gametes was carried out within a 2-day window during the period of spontaneous spawning. Each spawning individual was placed in a 250 mL beaker containing 200 mL of aerated 0.45 μm filtered seawater (FSW) until complete gamete release. The sperm and eggs of mussels were sieved through 50 μm and 90 μm meshes, respectively, to remove impurities after spawning. Egg quality (shape, size) and sperm motility were checked using an inverted microscope. Eggs were fertilized by adding sperm (1:10 ratio) in 6-well plates (NEST, Naisi Life Technology Co., Ltd., Wuxi, China). After 30 min, successful fertilization (n. fertilized eggs/n. total eggs × 100) was checked by microscopic observation (>85%) (Balbi et al., 2018).
Sexually mature Pacific oysters (M. gigas) were collected from a local breeding farm in Qingdao, Shandong Province, China, between May and August 2020, and transferred to the laboratory and acclimatized using the same method descried above. The oyster gametes were collected by dissection within a 7-day period. Gametes were transferred from the oyster gonads to 0.45 μm FSW using a pipette. The washing and fertilization procedure for these gametes used the same method described above.
Five-month-old male and female marine medaka were quartered in 10-L glass aquaria with 6-L natural seawater at 28 ± 1°C, with a 14:10 h light-dark cycle. Marine medaka were fed with Artemia nauplii three times a day, and the excreta was removed daily. The males and females of medaka were paired with equal individuals in a breeding aquarium before spawning. Spawned eggs were collected the next morning (8:00-9:00 am), rinsed with FSW, and examined under a stereomicroscope (Zhongheng Instrument Ltd., Shanghai, China) to ensure fertilization.
2.3. BMAA exposure experiments for bivalve embryos
Mussel embryos exposed to BMAA were observed for 48 h in 48-well microwell plates containing 1 mL of liquid per well. A total of 25 embryos were exposed in each well with a range of different concentrations of BMAA including a zero blank control (FSW only), solvent control (2 mM HCl in FSW), 50 (0.32 μM), 100 (0.65 μM), 200 (1.29 μM), 400 (2.59 μM) and 800 (5.18 μM) μg BMAA·HCl/L in FSW. Each treatment was prepared in triplicate. Microplates were maintained at 16 ± 1°C for 48 h, with a 16 h: 8 h cycle of light: dark.
The bioassay for oyster embryos was carried out using the same method described above, except using higher concentrations of BMAA: 800 (5.18 μM), 1200 (7.77 μM), 1600 (10.36 μM), 2000 (12.94 μM) and 2400 (15.53 μM) μg BMAA·HCl/L. Each treatment was prepared in triplicate and the microplates were incubated at 24 ± 1°C for 24 h in the dark.
At the end of the incubation period, samples were fixed with a buffered formalin (4%) solution. All larvae in each well were examined using an inverted microscope (Olympus, Milano, Italy). A larva was considered normal when its shell was D-shaped (straight hinge) and the mantle did not protrude out of the shell. Meanwhile, a malformed embryo was defined as not reaching the normal 48 hours post fertilization (HPF) stage for mussels and not reaching the 24 HPF for oysters (trochophore or earlier stages) or considered malformed if other developmental defects were observed (concave, malformed or damaged shell, protruding mantle). The recorded endpoint was the percentage of normal D-larvae (D-veligers) in each well relative to the total number, including malformed larvae and pre-D stages. The blank control with only seawater and solvent control with HCl were run in parallel, and no obvious effects of HCl solvent on the embryo development were observed even at the highest concentration of HCl added in seawater.
To calculate the EC50 values of BMAA for mussel and oyster embryos, the percent of biological response was modified by the solvent control following the equation:
where P is the modified response (%), Po is the observed initial response (%) and Pc is the control response (%) (His et al., 1999).
In addition, the length (the anterior-posterior dimension of the shell parallel to the hinge line) and height (the dorsal-ventral dimension perpendicular to the hinge) of mussel D-veligers at 48 HPF in different experimental groups were also measured under an inverted microscope (Kurihara et al., 2008).
2.4. BMAA exposure experiments for marine medaka embryos
To evaluate the effects of BMAA on the embryonic development of fish, 15 embryos (3-5 HPF) of medaka were selected and exposed to FSW (blank control), FSW with 2 mM HCl (solvent control), and 20 (0.13 μM), 200 (1.29 μM), 2000 (12.9 μM), 20000 (129 μM) μg BMAA·HCl/L in each treatment until hatching. Embryonic development was examined every day under an inverted microscope (Olympus, Milano, Italy) equipped with a color CCD camera. Several sublethal endpoints were observed during the exposure period, including developmental rate (occurrence/percentage of head bud at 24 HPF and eye pigmentation at 48 HPF), heart beat at 6 days post fertilization (DPF) and 9 DPF, hatching time-course and hatching success rate, cumulative mortality and mortality ratio at four developmental periods, morphological abnormality, malformed characteristic (Wang et al., 2020b). Embryos were judged as dead if they turned opaque and/or white in early developmental stages and if a heart beat could not be observed in the later stages. Dead embryos were counted and removed daily. The solutions were renewed once after three days. A hatching failure was recorded if the embryo did not hatch within 18 days.
2.5. Effect of BMAA on the ATP concentration of marine medaka embryos
The ATP concentration of medaka embryos was determined using an ATP assay kit (Solarbio, Beijing, China). Embryos (3-5 HPF) were exposed to FSW (blank control), FSW with 2 mM HCl (solvent control), and 20000 μg BMAA·HCl/L. Based on previous experiments (tested in section 2.4), embryos started to hatch at 10 DPF. Thus, embryos were collected at 9 DPF, i.e. one day before hatching, to test for ATP. Embryos and ATP extract solvent were mixed at a ratio of 1:10 (embryo weight: ATP extraction volume, m/v) and were homogenized within an ice bath. The homogenates were centrifuged at 8000 ×g, 4°C for 10 min. Supernatants were collected and chloroform was added. The mixed liquid was centrifuged at 10000 ×g, 4°C for 3 min. Supernatants were collected and 20 μL were transferred to a 96-well plate. The ATP reaction solution was added to these samples in each well. The ATP concentration was analyzed at 340 nm using a Synergy MX microplate reader after gently shaking the mixture.
2.6. Statistical analysis
All data were expressed as means ± standard deviations (SDs). The EC50 values for BMAA were calculated by probability unit conversion and analysis (Giannuzzi et al., 2021). Statistical analysis was performed using the IMB SPSS Statistics V25.0 software (IBM Corporation, New York, USA). Results were initially tested for normality and equality of variance (Levene’s test, 5% risk). Percentage data were arcsine transformed to achieve normality and equality of variance criteria. If assumptions of homogeneity of variances were satisfied, one-way analysis of variance (ANOVA) followed by LSD’s test was used. A p-value< 0.05 indicated a statistically significant result.
3. Results
3.1. Survival
The embryo mortality of mussel and oysters were less than 20% and 10%, respectively, in all experimental groups (Figure S1). No acute lethal effect of BMAA on either bivalve was observed in this study. Accumulative mortality of marine medaka did not significantly differ between the solvent controls and BMAA exposure treatments (Figure S2). The LC50 value of marine medaka embryo was higher than 20000 μg BMAA·HCl/L.
3.2. Effects of BMAA on the development of bivalve embryos
The effects of BMAA exposure on the embryonic development of mussel and oysters are summarized in Figure 1. The percentage of normal D-veligers in the mussel embryo bioassay decreased with rising concentrations of BMAA (Figure 1A), and no normal D-veligers were found in the BMAA exposure treatments at 400 and 800 μg BMAA·HCl/L. The percentage of mussel trochophores gradually increased with the concentration of BMAA in the exposure groups, and all embryos were blocked at the trochophore stage in the 400 and 800 μg BMAA·HCl/L treatments. Furthermore, the percentage of malformed D-veligers also positively increased as the BMAA increased from 0 to 200 μg BMAA·HCl/L.
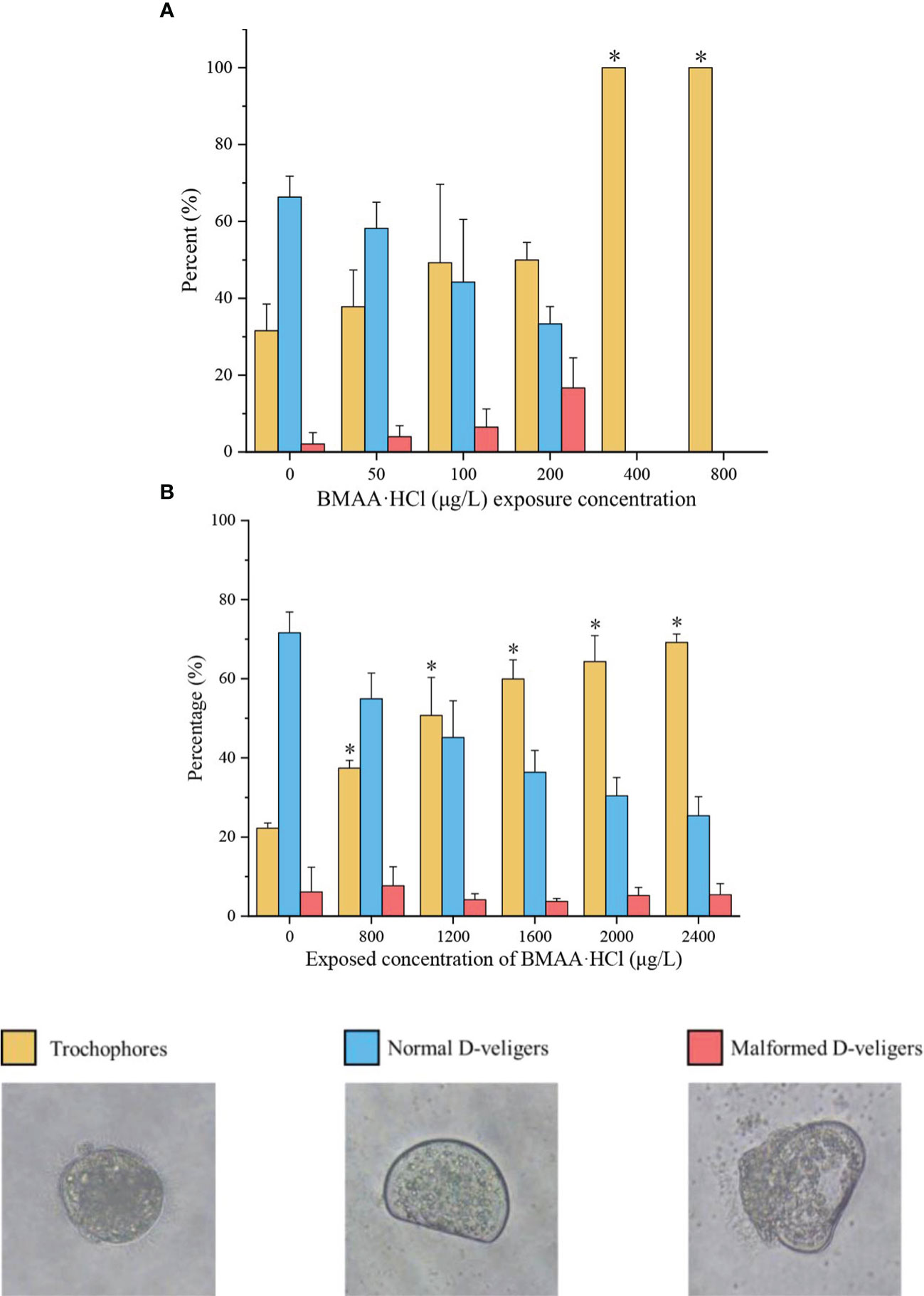
Figure 1 Percentage of trochophores, normal D-veligers, and malformed D-veligers of mussel (Mytilus galloprovincialis) at 48 hours post fertilization (HPF) (A) and oyster (Magallana gigas) at 24 HPF (B) in the BMAA exposure experiments. Note: An equivalent volume of 2 mM HCl used in the highest BMAA exposure treatments was added into the culture wells as a solvent control, which was labeled as a zero concentration of BMAA. Only the percentage of trochophores between control group and BMAA exposure treatments were statistically analyzed. An asterisk indicates the results were significantly difference at p< 0.05.
Within the oyster embryo bioassay (Figure 1B), the percent variation of normal D-veligers and trochophores of oysters demonstrated similar trends as those seen with the mussel embryos from 0 to 2400 μg BMAA·HCl/L treatments. However, the percentage of malformed oyster D-veligers was less than 10% and did not significantly change between different BMAA treatments.
The modified responses of mussel and oyster embryos demonstrated that the mussel embryos were more sensitive to BMAA exposure than oyster embryos (Figure S3). The dose-response curves for mussel and oyster embryos exposed to BMAA were shown in Figure 2. Finally, the 48 h-EC50 for mussel embryos was 196 μg BMAA·HCl/L (1.27 µM), and the 24 h-EC50 for oyster embryos was 1660 μg BMAA·HCl/L (10.7 µM) (Table 1).
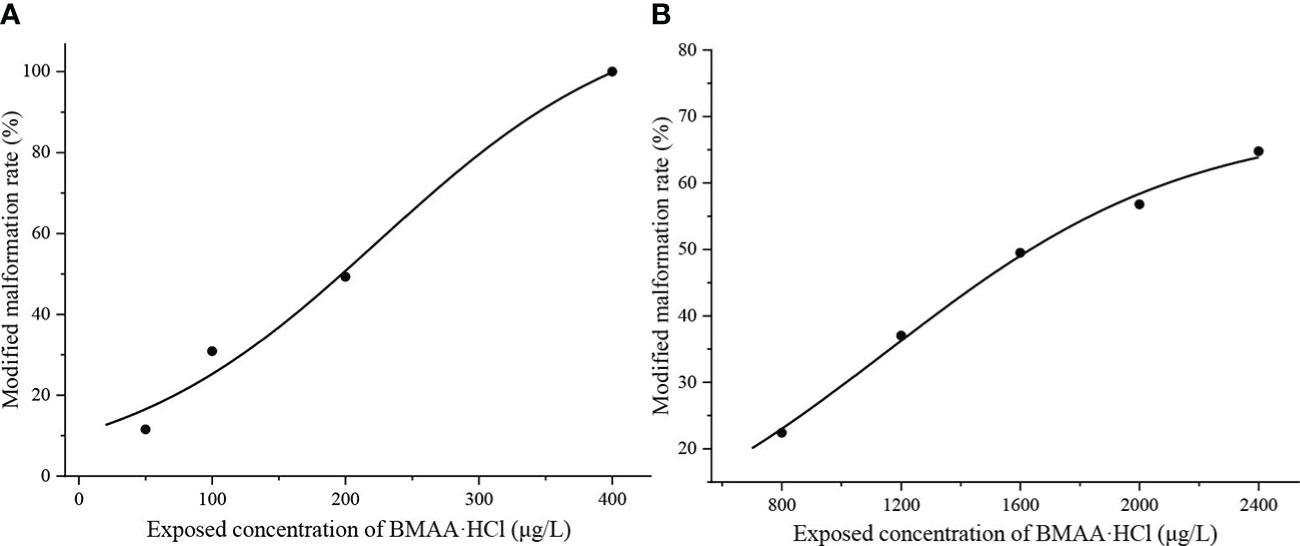
Figure 2 Modified malformation ratios of mussel (Mytilus galloprovincialis) embryos at 48 hours post fertilization (HPF) (A) and oyster (Magallana gigas) embryos at 24 HPF (B) during the BMAA exposure experiments.
To assess the impact of BMAA on the growth and development of mussel embryos, the shell length and height of normal D-veligers at 48 HPF were calculated (Figure 3). The mussel shell size significantly reduced when the embryos were exposed to BMAA at 100 and 200 μg BMAA·HCl/L.
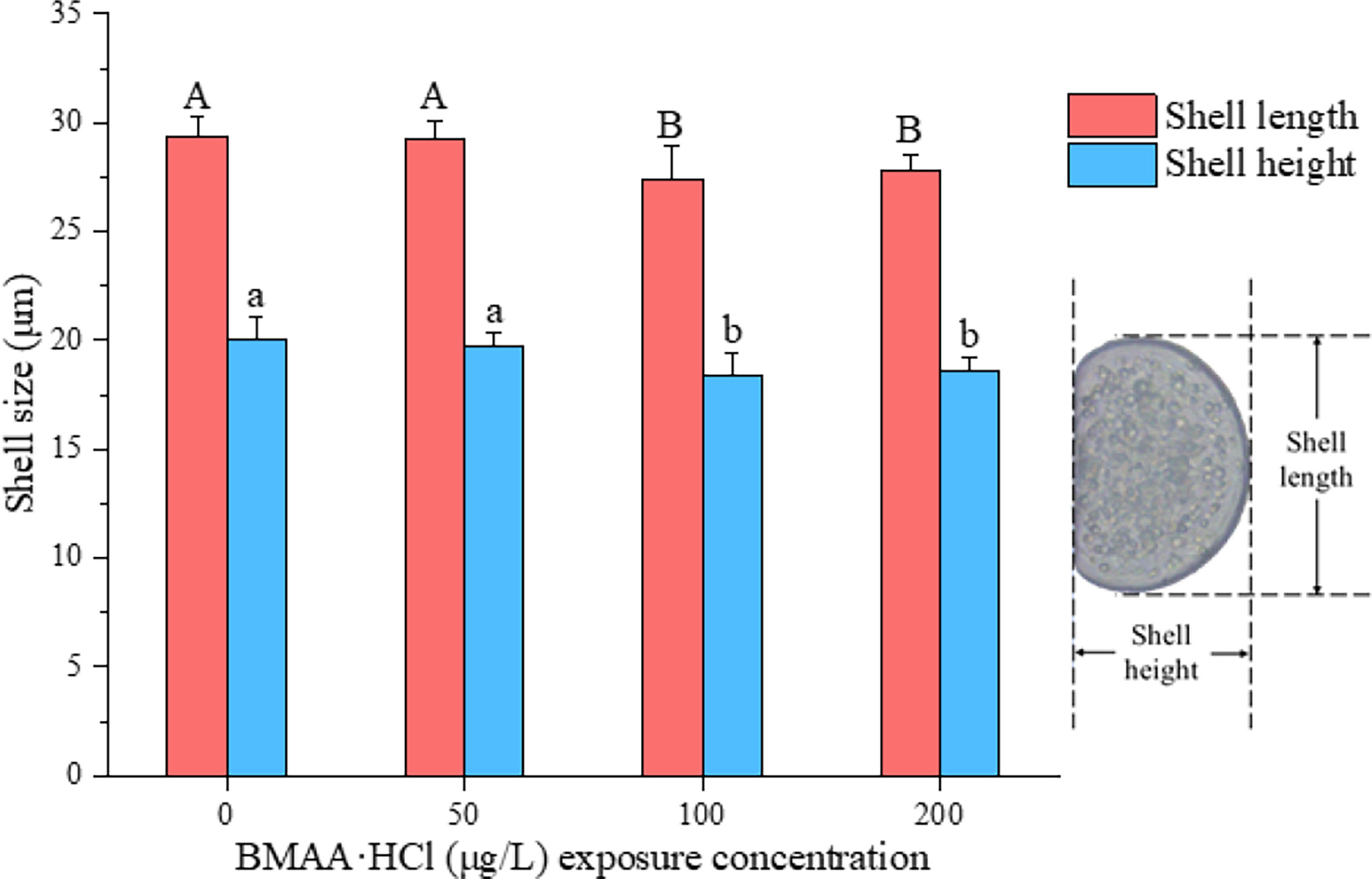
Figure 3 Shell size of normal D-veligers at 48 HPF in the mussel (Mytilus galloprovincialis) embryos following exposure to BMAA. Note: An equivalent volume of 2 mmol/L HCl used in the highest BMAA exposure treatments was added into the culture wells as a solvent control group and labeled as a zero concentration of BMAA. The different letters indicate a significantly different result between treatments (p< 0.05).
3.3. Effects of BMAA on development and ATP concentration within marine medaka embryos
The development of head bud (1 DPF) and eye pigmentation (2 DPF) were above 90% in all treatments (Figure S4). No significant difference between the developmental rates of medaka embryos occurred in the solvent control and BMAA treatments. The heart rate of embryos recorded at 6 DPF indicated no differences between treatment groups (Figure 4). However, the heart rate of embryos at 9 DPF were reduced in a dose-dependent manner when exposed to BMAA at 200, 2000 and 20000 μg BMAA·HCl/L.
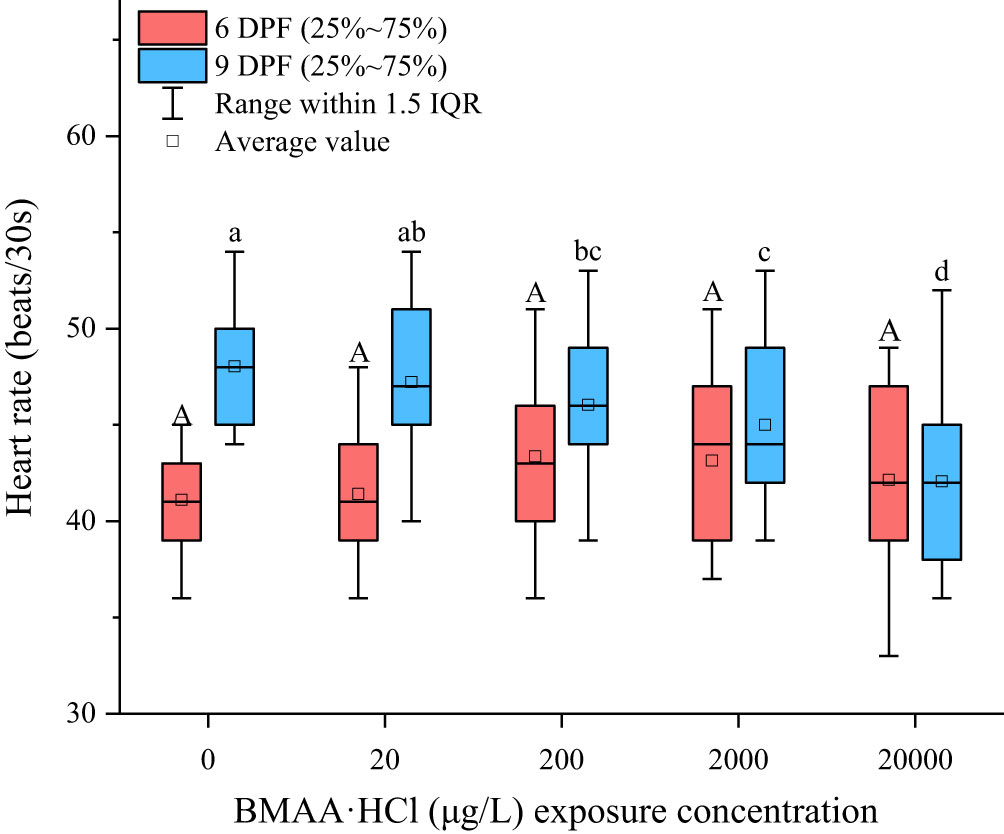
Figure 4 Heart rates measured at 6 days post fertilization (DPF) and 9 DPF in marine medaka (Oryzias melastigma) embryos exposed to BMAA. Note: An equivalent volume of 2 mM HCl used in the highest BMAA exposure treatments was added into the culture wells as solvent control group and labeled as a zero concentration of BMAA. The different letters indicate a significantly different result between treatments (p< 0.05).
The hatching rates of marine medaka embryos slightly increased with rising BMAA concentrations but no statistical difference between solvent control and exposure treatments were found (Figure S5A). We note that the error bars between replicates showed that variation in the hatching rate in BMAA exposure treatments were higher than that in the solvent control group. The hatching time-course of medaka embryos was not obviously affected by BMAA exposure in this study (Figure S5B). In addition, no observed changes for the development of eye melanin in medaka embryos were found in the BMAA exposure treatments.
No significant differences were found in the ATP concentrations between blank controls and solvent controls used with the medaka embryos, which demonstrated that the additional low concentration of HCl did not affect the ATP concentration of medaka embryos. Although the result was not statistically significant, the ATP concentration of medaka embryos exposed to 20000 μg BMAA·HCl/L decreased and expressed relatively large variation within the replicated groups (Figure 5).
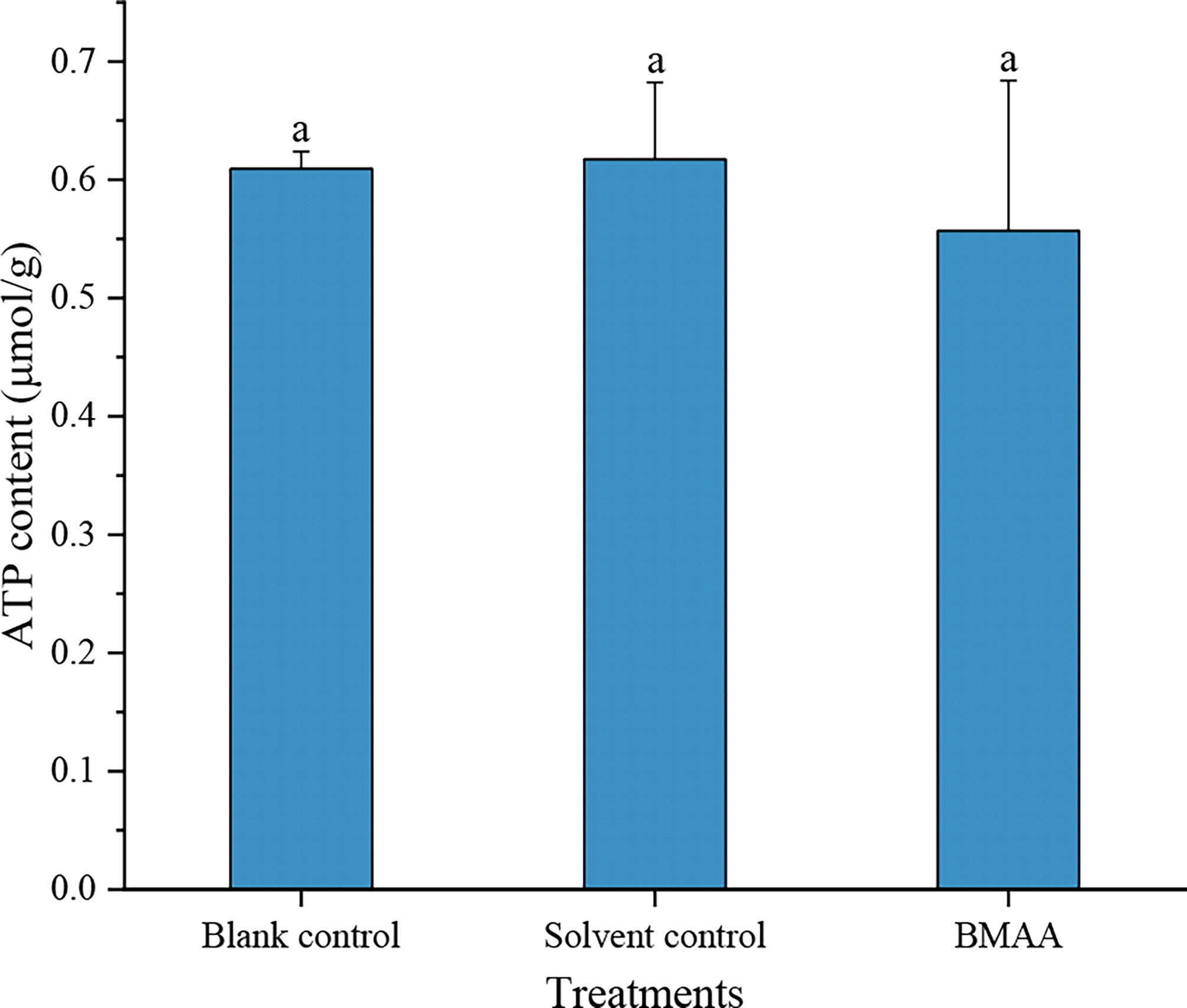
Figure 5 The ATP concentrations in marine medaka (Oryzias melastigma) embryos at 9 days post fertilization (DPF) in different treatments. An equivalent volume of 2 mM HCl was added into the culture well as a solvent control group.
4. Discussion
BMAA is hydrophilic and can be accumulated by shellfish and marine opossum shrimp (Neomysis awatschensis) through water filtration (Baptista et al., 2015; Wang et al., 2020a). Prior research has demonstrated that soluble BMAA was directly transferred to marine organisms from a seawater medium (Baptista et al., 2015; Wang et al., 2020a). Exogenous BMAA has been shown to disrupt the antioxidant system and cause significant DNA damage in the hemocytes of freshwater bivalves (Contardo-Jara et al., 2014; Lepoutre et al., 2018).
To further explore the toxicological effects of BMAA dissolved in seawater environments on marine bivalves and fish, we examined the external fertilization and early development of bivalve and fish embryos exposed to exogenous BMAA. We did not find a lethal effect on mussel and oyster embryos exposed to BMAA at the experimental dose used here, but demonstrated that embryo development was markedly inhibited (Figure 1). The development of all mussel embryos was delayed at the trochophore stage (48-hours post fertilization, HPF) by exposure to BMAA concentrations of 400 and 800 μg BMAA·HCl/L (Figure 1A). Comparatively, the oyster embryos were not sensitive to BMAA exposure as 25% of the embryos entered the D-veliger stage from the trochophore stage at 24 HPF while exposed to 2400 μg BMAA·HCl/L (Figure 1B). This discrepancy suggests that sensitivity to BMAA exposure during embryogenesis was interspecific in bivalves. Due to the delayed development process, the malformation rate of bivalve embryos was modified and used to calculate the EC50 values for mussel (48 h) and oyster (24 h) embryos at 1.27 and 10.7 µM BMAA, respectively (Table 1). In our previous study, the fertilization and development of sea urchin embryos were also significantly inhibited by BMAA above 300 μg/L (2.54 μM), and EC50 value calculated by the percent of active swimming larvae was 1.4 µM BMAA (Li et al., 2020). Therefore, the EC50 values for mussel and sea urchin embryos were similar, which was significantly lower than that for oyster embryos. Similar sensitivities during embryogenesis were noted among sea urchins, mussels, and copepods exposed to aromatic hydrocarbons found in heavy fuel oil (Saco-Álvarez et al., 2008). The sensitivity discrepancy we noted in bivalve embryos exposed to BMAA suggests that oysters have a relatively high resistivity to environmental contaminants. In this current study, we did not observe significant changes in the fertilization efficiency of oyster sperm exposed to a high concentration of BMAA 3200 μg BMAA·HCl/L (20.7 µM) (Figure S6). This is in contrast to our previous observations of the fertilization ability of sea urchin sperm which were significantly reduced after exposure to 500 μg BMAA/L (4.24 µM) for 10 min (Li et al., 2020). This difference further supports the conclusion that both gametes and embryos of oysters were more resistant to BMAA than those of sea urchins.
In addition to the delayed bivalve embryogenesis, as discussed above, the shell size of normal D-veliger also decreased with increasing BMAA concentrations. In recent studies, the larval shell formation of oysters was shown to be modulated by monoamine neurotransmitters, 5-HT and dopamine (DA), through the TGF-β smad pathway, triggering the expression of tyrosinase to form the initial shell which further inhibited the expression of chitinase at the trochophore stage (Liu et al., 2018; Liu et al., 2020). Interestingly, DA levels were altered in the central nervous system of adult rats and reduced in the substantia nigra of the rat brain due to the loss of tyrosine hydroxylase (TH)-positive dopaminergic neurons following BMAA exposure (Santiago et al., 2006; Scott and Downing, 2019). For aquatic animals, the expression of dopamine D4 receptor (DRD4) and monoamine oxidase A (MAOA) were also differentially down-regulated in the brain of mangrove rivulus fish (Kryptolebias marmoratus) exposed to BMAA at 15 mg/L (Carion et al., 2020). The inhibition of DA and serotonin biosynthesis were hypothesized to explain the destruction of swimming ability of sea urchin larva (Li et al., 2020). We here hypothesize that disruption or inhibition of 5-HT and/or DA biosynthesis were possibly responsible for the inhibitory effects of BMAA on shell formation and growth of shellfish embryos in this current study.
The heart development is a key process for successful embryogenesis of fish. The delivery of nutrients necessary for growth would be reduced if cardiac function was impaired by either heart malformations or a reduced heartbeat. The heart rate of marine medaka larvae was significantly reduced in a dose-dependent manner at 9 DPF, in this study (Figure 4). This same effect has been noted in previous studies using the freshwater zebrafish larvae model exposed to BMAA (Purdie et al., 2009b; Wang, 2015). The embryonic heartbeat of zebrafish was linked to several ionic currents including sodium, T-type and L-type calcium and several potassium currents (Rottbauer et al., 2001). Increased Ca2+ in the sarcoplasmic reticulum caused delayed post depolarization and arrhythmia in this model (Zhang et al., 2016). Likewise, the β-carbamate adduct of BMAA could act on glutamate receptors to activate Ca2+ influx into cells through NMDA receptors and result in the accumulation of intracellular Ca2+ (Salińska et al., 2005). Recently, it has been confirmed that the endoplasmic reticulum releases Ca2+ in rat primary motor neurons (Petrozziello et al., 2022). Glutamate receptors exist not only in the CNS, but also in other non-neuronal tissues such as the heart, pancreas and skin (Skerry and Genever, 2001; Mueller et al., 2003). In the current study, the decreased embryonic heart rate of medaka was possibly caused by the abnormal activation of glutamate receptors resulting in an increase of intracellular Ca2+ in embryonic cardiomyocytes as a direct consequence of BMAA exposure.
Mitochondrial dysfunction could lead to cardiac developmental defects because the cardiac contraction and relaxation processes relies on ATP biosynthesis by the mitochondria. Our results demonstrated that the average content of ATP in medaka embryos treated with BMAA at 9 DPF was slightly lower than that of the control group but with greater variation (Figure 5). However, an increase of intracellular ATP levels occurred in a fish immune cell line (CLC) exposed to 1.5 mM BMAA without dose-dependence, which indicated that the BMAA exposure could alter ATP biosynthesis (Sieroslawska and Rymuszka, 2019). It is possible that BMAA could affect mitochondrial function via multiple mechanisms in different species (Chiu et al., 2012).
The indexes of eye, heart and hatching rate are commonly used to assess the embryogenesis development of fish in toxicological tests. The eyes of medaka embryos begin to develop at about 28 HPF, and the optic choroidea darken because of melanin pigmentation at about 50 HPF (González-Doncel et al., 2005). In the present study, the development of eye melanin in medaka embryos was not significantly affected by BMAA. Reportedly, BMAA binds to melanin and neuromelanin cells in animals, which may lead to changes in the structure and characteristics of melanin and neuromelanin. Long term exposure to BMAA possibly caused the disintegration of melanin polymers in cells containing melanin and neuromelanin (Karlsson et al., 2009). Although no obvious effect of BMAA on melanin synthesis in medaka embryos was found, its possible impact on the later life development of medaka embryos could not be excluded.
The hatching rate of medaka embryos was not affected by BMAA. The majority of embryos in all treatments hatched in the time interval from 10 to 14 DPF. No effect of BMAA on the incubation time of zebrafish embryos was observed in previous studies using even higher concentrations of BMAA (50 and 100 mg/L) (Purdie et al., 2009b; Wang, 2015). The BMAA exposure did not cause abnormal death of medaka embryos in this study, which is similar to a previous study on freshwater fish (Wang, 2015). We observed a reduction in the heart rate of medaka embryos at 9 DPF, which could have an effect on the later developmental stages of medaka embryos. In prior in vivo experiments, almost no acute lethal effect was recorded and abnormal biological behaviors were observed only following the injection of BMAA at even higher concentrations (Chiu et al., 2011; Delcourt et al., 2018; Wang et al., 2020a). Long-term observations of aquatic organisms exposed to BMAA are needed to assess its full ecological impact.
5. Conclusions
The adverse effects of BMAA on the early embryo development of marine bivalves and marine medaka were explored and discussed in this study. We documented the sublethal effects of BMAA on the embryogenesis and growth of bivalve and fish embryos. The embryonic development and growth of mussel and oyster larvae, as well as the shell formation and growth of mussel larvae, were inhibited by BMAA exposure. Comparatively, mussel embryos were more sensitive to BMAA than oyster embryos. Arrhythmias and a slight reduction of average ATP concentration occurred in marine medaka embryos exposed to BMAA. Long-term effects of BMAA on the production and development of aquatic organisms should be explored in future research.
Data availability statement
The original contributions presented in the study are included in the article/Supplementary Material. Further inquiries can be directed to the corresponding author.
Author contributions
YF: Experimental design, Data acquisition and processing, Manuscript draft. AL: Experimental design, Supervisor, Funding acceptance, Manuscript review and editing. JQ: Project supervision, Manuscript review and editing. WY: Experimental animal maintenance. CY: Experimental animal maintenance. LZ: Experimental design. ML: Experimental design. All authors contributed to the article and approved the submitted version.
Acknowledgments
This work was funded by the National Natural Science Foundation of China (U2106205, 41676093). We appreciate Dr. Sandra Banack at the Brain Chemistry Laboratories, Institute for Ethnomedicine, USA editing this manuscript before resubmission.
Conflict of interest
The authors declare that the research was conducted in the absence of any commercial or financial relationships that could be construed as a potential conflict of interest.
Publisher’s note
All claims expressed in this article are solely those of the authors and do not necessarily represent those of their affiliated organizations, or those of the publisher, the editors and the reviewers. Any product that may be evaluated in this article, or claim that may be made by its manufacturer, is not guaranteed or endorsed by the publisher.
Supplementary material
The Supplementary Material for this article can be found online at: https://www.frontiersin.org/articles/10.3389/fmars.2022.1033851/full#supplementary-material
References
Albano R., Lobner D. (2018). Transport of BMAA into neurons and astrocytes by system xc-. Neurotox. Res. 33, 1–5. doi: 10.1007/s12640-017-9739-4
Balbi T., Montagna M., Fabbri R., Carbone C., Franzellitti S., Fabbri E., et al. (2018). Diclofenac affects early embryo development in the marine bivalve Mytilus galloprovincialis. Sci. Total Environ. 642, 601–609. doi: 10.1016/j.scitotenv.2018.06.125
Baptista M. S., Vasconcelos R. G., Ferreira P. C., Almeida C. M., Vasconcelos V. M. (2015). Assessment of the non-protein amino acid BMAA in Mediterranean mussel Mytilus galloprovincialis after feeding with estuarine cyanobacteria. Environ. Sci. Pollut. Res. 22, 12501–12510. doi: 10.1007/s11356-015-4516-5
Capela R., Garric J., Castro L. F. C., Santos M. M. (2020). Embryo bioassays with aquatic animals for toxicity testing and hazard assessment of emerging pollutants: A review. Sci. Total Environ. 705, 135740. doi: 10.1016/j.scitotenv.2019.135740
Carion A., Markey A., Hetru J., Carpentier C., Suarez-Ulloa V., Denoel M., et al. (2020). Behavior and gene expression in the brain of adult self-fertilizing mangrove rivulus fish (Kryptolebias marmoratus) after early life exposure to the neurotoxin β-N-methylamino-L-alanine (BMAA). Neurotoxicol 79, 110–121. doi: 10.1016/j.neuro.2020.04.007
Chiu A. S., Gehringer M. M., Braidy N., Guillemin G. J., Welch J. H., Neilan B. A. (2012). Excitotoxic potential of the cyanotoxin β-methyl-amino-L-alanine (BMAA) in primary human neurons. Toxicon 60, 1159–1165. doi: 10.1016/j.toxicon.2012.07.169
Chiu A. S., Gehringer M. M., Welch J. H., Neilan B. A. (2011). Does α-amino-β-methylaminopropionic acid (BMAA) play a role in neurodegeneration? Int. J. Environ. Res. Public Health 8, 3728–3746. doi: 10.3390/ijerph8093728
Contardo-Jara V., Otterstein S. K. B., Downing S., Downing T. G., Pflugmacher S. (2014). Response of antioxidant and biotransformation systems of selected freshwater mussels (Dreissena polymorpha, anadonta cygnea, unio tumidus, and Corbicula javanicus) to the cyanobacterial neurotoxin β-N-methylamino-L-alanine. Toxicol. Environ. Chem. 96, 451–465. doi: 10.1080/02772248.2014.945452
Cox P. A., Davis D. A., Mash D. C., Metcalf J. S., Banack S. A. (2016). Dietary exposure to an environmental toxin triggers neurofibrillary tangles and amyloid deposits in the brain. Proc. Biol. Sci. 283, 20152397. doi: 10.1098/rspb.2015.2397
Davis D. A., Cox P. A., Banack S. A., Lecusay P. D., Garamszegi S. P., Hagan M., et al. (2020). L-serine reduces spinal cord pathology in a vervet model of preclinical ALS/MND. J. Neuropathol. Exp. Neurol. 79, 393–406. doi: 10.1093/jnen/nlaa002
Delcourt N., Claudepierre T., Maignien T., Arnich N., Mattei C. (2018). Cellular and molecular aspects of the β-N-methylamino-L-alanine (BMAA) mode of action within the neurodegenerative pathway: Facts and controversy. Toxins 10, 6. doi: 10.3390/toxins10010006
Dunlop R. A., Banack S. A., Bishop S. L., Metcalf J. S., Murch S. J., Davis D. A., et al. (2021). Is exposure to BMAA a risk factor for neurodegenerative diseases? a response to a critical review of the BMAA hypothesis. Neurotox. Res. 39, 81–106. doi: 10.1007/s12640-020-00302-0
Esterhuizen-Londt M., Wiegand C., Downing T. G. (2015). β-N-methylamino-L-alanine (BMAA) uptake by the animal model, Daphnia magna and subsequent oxidative stress. Toxicon 100, 20–26. doi: 10.1016/j.toxicon.2015.03.021
Giannuzzi L., Lombardo T., Juárez I., Aguilera A., Blanco G. (2021). A stochastic characterization of hydrogen peroxide-induced regulated cell death in Microcystis aeruginosa. Front. Microbiol. 12, 636157. doi: 10.3389/fmicb.2021.636157
González-Doncel M., Okihiro M. S., Villalobos S. A., Hinton D. E., Tarazona J. V. (2005). A quick reference guide to the normal development of Oryzias latipes (Teleostei, adrianichthyidae). J. Appl. Ichthyol. 21, 39–52. doi: 10.1111/j.1439-0426.2004.00615.x
Hanrieder J., Gerber L., Sandelius Å. P., Brittebo E. B., Ewing A. G., Karlsson A. (2014). High resolution metabolite imaging in the hippocampus following neonatal exposure to the environmental toxin BMAA using ToF-SIMS. Neurosci 5, 568–575. doi: 10.1021/cn500039b
His E., Beiras R., Seaman M. N. L. (1999). The assessment of marine pollution - bioassays with bivalve embryos and larvae. Adv. Mar. Biol 37, 1–178. doi: 10.1016/S0065-2881(08)60428-9
Karlsson O., Berg C., Brittebo E. B., Lindquist N. G. (2009). Retention of the cyanobacterial neurotoxin β-N-methylamino-L-alanine in melanin and neuromelanin-containing cells - a possible link between Parkinson-dementia complex and pigmentary retinopathy. Pigment Cell Melanoma Res. 22, 120–130. doi: 10.1111/j.1755-148X.2008.00508.x
Kurihara H., Asai T., Kato S., Ishimatsu A. (2008). Effects of elevated pCO2 on early development in the mussel Mytilus galloprovincialis. Aquat. Biol. 4, 225–233. doi: 10.3354/ab00109
Lance E., Arnich N., Maignien T., Bire R. (2018). Occurrence of β-N-methylamino-L-alanine (BMAA) and isomers in aquatic environments and aquatic food sources for humans. Toxins 10, 83. doi: 10.3390/toxins10020083
Lepoutre A., Milliote N., Bonnard M., Palos-Ladeiro M., Rioult D., Bonnard I., et al. (2018). Genotoxic and cytotoxic effects on the immune cells of the freshwater bivalve Dreissena polymorpha exposed to the environmental neurotoxin BMAA. Toxins 10, 106. doi: 10.3390/toxins10030106
Li A., Espinoza J., Hamdoun A. (2020). Inhibitory effects of neurotoxin β-N-methylamino-L-alanine on fertilization and early development of the Sea urchin Lytechinus pictus. Aquat. Toxicol. 221, 105425. doi: 10.1016/j.aquatox.2020.105425
Li A., Hu Y., Song J., Wang S., Deng L. (2018). Ubiquity of the neurotoxin β-N-methylamino-L-alanine and its isomers confirmed by two different mass spectrometric methods in diverse marine mollusks. Toxicon 151, 129–136. doi: 10.1016/j.toxicon.2018.07.004
Liu Z. Q., Wang L. L., Yan Y. C., Zheng Y., Ge W. J., Li M. J., et al. (2018). D1 dopamine receptor is involved in shell formation in larvae of pacific oyster Crassostrea gigas. Dev. Comp. Immunol. 84, 337–342. doi: 10.1016/j.dci.2018.03.009
Liu Z., Zhou Z., Zhang Y., Wang L., Song X., Wang W., et al. (2020). Ocean acidification inhibits initial shell formation of oyster larvae by suppressing the biosynthesis of serotonin and dopamine. Sci. Total Environ. 735, 139469. doi: 10.1016/j.scitotenv.2020.139469
Lopicic S., Nedeljkov V., Cemerikic D. (2009). Augmentation and ionic mechanism of effect of β-N-methylamino-L-alanine in presence of bicarbonate on membrane potential of retzius nerve cells of the leech Haemopis sanguisuga. Comp. Biochem. Physiol. A. Mol. Integr. Physiol. 153, 284–292. doi: 10.1016/j.cbpa.2009.02.038
Mueller R. W., Gill S. S., Pulido O. M. (2003). The monkey (Macaca fascicularis) heart neural structures and conducting system: An immunochemical study of selected neural biomarkers and glutamate receptors. Toxicol. Pathol. 31, 227–234. doi: 10.1080/01926230390183724
Petrozziello T., Boscia F., Tedeschi V., Pannaccione A., de Rosa V., Corvino A., et al. (2022). Na+/Ca2+ exchanger isoform 1 takes part to the Ca2+-related prosurvival pathway of SOD1 in primary motor neurons exposed to beta-methylamino-L-alanine. Cell Commun. Signal. 20, 8. doi: 10.1186/s12964-021-00813-z
Purdie E. L., Metcalf J. S., Kashmiri S., Codd G. A. (2009a). Toxicity of the cyanobacterial neurotoxin β-N-methylamino-L-alanine to three aquatic animal species. Amyotroph. Lateral Scler. 2, 67–70. doi: 10.3109/17482960903273551
Purdie E. L., Samsudin S., Eddy F. B., Codd G. A. (2009b). Effects of the cyanobacterial neurotoxin β-N-methylamino-L-alanine on the early-life stage development of zebrafish (Danio rerio). Aquat. Toxicol. 95, 279–284. doi: 10.1016/j.aquatox.2009.02.009
Rao S. D., Banack S. A., Cox P. A., Weiss J. H. (2006). BMAA selectively injures motor neurons via AMPA/Kainate receptor activation. Exp. Neurol. 201, 244–252. doi: 10.1016/j.expneurol.2006.04.017
Rottbauer W., Baker K., Wo Z. G., Mohideen M. P. K., Cantiello H. F., Fishman M. C. (2001). Growth and function of the embryonic heart depend upon the cardiac-specific l-type calcium channel α1 subunit. Dev. Cell 1, 265–275. doi: 10.1016/s1534-5807(01)00023-5
Saco-Álvarez L., Bellas J., Nieto Ó., Bayona J. M., Albaigés J., Beiras R. (2008). Toxicity and phototoxicity of water-accommodated fraction obtained from Prestige fuel oil and marine fuel oil evaluated by marine bioassays. Sci. Total Environ. 394, 275–282. doi: 10.1016/j.ecoenv.2015.09.002
Salińska E., Danysz W., Łazarewicz J. W. (2005). The role of excitotoxicity in neurodegeneration. Folia Neuropathol. 43, 322–339. doi: 10.1016/s0163-7258(98)00042-4
Santiago M., Matarredona E. R., Machado A., Cano J. (2006). Acute perfusion of BMAA in the rat’s striatum by in vivo microdialysis. Toxicol. Lett. 167, 34–39. doi: 10.1016/j.toxlet.2006.08.005
Scott L., Downing T. (2019). Dose-dependent adult neurodegeneration in a rat model after neonatal exposure to β-N-methylamino-L-alanine. Neurotox. Res. 35, 711–723. doi: 10.1007/s12640-019-9996-5
Sieroslawska A., Rymuszka A. (2019). Assessment of the cytotoxic impact of cyanotoxin beta-N-methylamino-L-alanine on a fish immune cell line. Aquat. Toxicol. 212, 214–221. doi: 10.1016/j.aquatox.2019.05.012
Skerry T. M., Genever P. G. (2001). Glutamate signalling in non-neuronal tissues. Trends Pharmacol. Sci. 22, 174–181. doi: 10.1016/S0165-6147(00)01642-4
Wang J. (2015). Acute toxicity of β-N-methylamino-L-alanine (BMAA) to fathead minnow (Pimephales promelas) and zebrafish (Danio rerio). (Master dissertation, Digital Commons, University of Nebraska, Lincoln, Nebraska, USA.).
Wang S., Qiu J., Zhao M., Li F., Yu R., Li A. (2020a). Accumulation and distribution of neurotoxin BMAA in aquatic animals and effect on the behavior of zebrafish in a T-maze test. Toxicon 173, 39–47. doi: 10.1016/j.toxicon.2019.11.005
Wang C., Yan C., Qiu J., Liu C., Yan Y., Ji Y., et al. (2021). Food web biomagnification of the neurotoxin β-N-methylamino-L-alanine in a diatom-dominated marine ecosystem in China. J. Hazard. Mater. 404, 124217. doi: 10.1016/j.jhazmat.2020.124217
Wang R. F., Zhu L. M., Zhang J., An X. P., Yang Y. P., Song M., et al. (2020b). Developmental toxicity of copper in marine medaka (Oryzias melastigma) embryos and larvae. Chemosphere 247, 125923. doi: 10.1016/j.chemosphere.2020.125923
Weiss J. H., Choi D. W. (1988). Beta-N-methylamino-L-alanine neurotoxicity: Requirement for bicarbonate as a cofactor. Sci 241, 973–975. doi: 10.1126/science.3136549
Keywords: β-N-methylamino-L-alanine (BMAA), embryonic development, Mytilus galloprovincialis, Magallana gigas, Oryzias melastigma
Citation: Fu Y, Li A, Qiu J, Yan W, Yan C, Zhang L and Li M (2022) Effects of the neurotoxin β-N-methylamino-L-alanine (BMAA) on the early embryonic development of marine shellfish and fish. Front. Mar. Sci. 9:1033851. doi: 10.3389/fmars.2022.1033851
Received: 01 September 2022; Accepted: 06 December 2022;
Published: 21 December 2022.
Edited by:
Simon Mitrovic, University of Technology Sydney, AustraliaReviewed by:
Haiyan Wu, Yellow Sea Fisheries Research Institute, Chinese Academy of Fishery Sciences (CAFS), ChinaJunhui Chen, First Institute of Oceanography, China
Copyright © 2022 Fu, Li, Qiu, Yan, Yan, Zhang and Li. This is an open-access article distributed under the terms of the Creative Commons Attribution License (CC BY). The use, distribution or reproduction in other forums is permitted, provided the original author(s) and the copyright owner(s) are credited and that the original publication in this journal is cited, in accordance with accepted academic practice. No use, distribution or reproduction is permitted which does not comply with these terms.
*Correspondence: Aifeng Li, bGFmb3VjQG91Yy5lZHUuY24=