- Red Sea Research Center (RSRC), King Abdullah University of Science and Technology (KAUST), Thuwal, Saudi Arabia
The light-dependent zooxanthellate jellyfish Cassiopea sp. (the upside-down jellyfish) is invasive/exotic in many shallow and clear marine habitats, where the jellyfish might be exposed to high levels of ultraviolet radiation (UVR). Compared to other reef organisms, the sensitivity/resilience of the semi-transparent jellyfish to UVR exposure is overlooked. Therefore, we experimentally investigated the metabolic and physiological responses of Cassiopea sp. from the Red Sea to natural levels of underwater UVR following 16 days of exposure to three light treatments: 1) control group with only photosynthetically active radiation (PAR), 2) PAR+UV-B, and 3) PAR+UV-B+UV-A. While jellyfish body mass increased (by 40%) significantly in the control group, it did not increase in either of the UV treatments. However, both UV-exposed jellyfish had higher (98% to 120%) mitochondrial electron transport system (ETS) activity than the control group. Therefore, the results indicate elevated aerobic respiration rates in UV-exposed jellyfish (i.e., reflecting a higher energy cost of UVR exposure). Neither the lactate dehydrogenase (LDH) activity nor the available energy (Ea) exhibited different levels among UVR treatments compared to the control group. In contrast, pyruvate kinase activity was significantly lower (by 46%) in all UV-exposed jellyfish compared to the control group. Unchanged Ea and LDH activity combined with higher ETS activity indicates a high aerobic capacity of jellyfish, which might explain their ability to cope with UVR exposure-induced higher energy demands without inducing the onset of anaerobiosis. The results indicated that UV-A does not amplify or modulate jellyfish physiology and growth under UV-B exposure. In conclusion, the findings suggest that the jellyfish is more resilient (i.e., in terms of survival) to UVR than other cnidarians. This study on Cassiopea is the first to address its metabolic and physiological responses to UVR. Therefore, it could be used as a framework for further studies aiming to better understand jellyfish physiology.
1. Introduction
The upside-down jellyfish (genus Cassiopea) has displayed signs of being exotic/invasive in many shallow marine coastal systems (Nabipour et al., 2015; Özbek and Oztürk, 2015; Thé et al., 2021). Unlike many other jellyfish, Cassiopea sp. is epibenthic and has symbiotic dinoflagellates (endosymbiont algae). This peculiarity (i.e., association with photosynthetic dinoflagellates) is shared with few other jellyfish (e.g. Linuche unguiculata and Mastigias sp.; Kremer et al., 1990; McCloskey et al., 1994). Therefore, it is a photosynthetic mixotrophic cnidarian, analogous to coral. Thus, Cassiopea sp. requires sufficient doses of photosynthetically active radiation (PAR) to grow optimally. Depending on light availability, the jellyfish might act as a functional photoautotroph (Cates, 1975; Verde and McCloskey, 1998), where it gains most of energy needs from photosynthesis. In Cassiopea, the symbiotic dinoflagellates live in oral arm and the bell, and can reach high densities (i.e., 1.52 × 106 to 2.68 × 106 cells mg–1 protein; Verde and McCloskey, 1998). These symbionts synthesize mycosporine-like amino acids (MAAs) and transport them to the host, protecting the holobiont from excessive ultraviolet radiation (UVR) in shallow water habitats (Banaszak and Trench, 1995). Furthermore, Cassiopea has a special photoprotective dimeric protein pigment known as Cassio Blue (Blanquet and Phelan, 1987; Phelan et al., 2005). While this pigment allows the passage of PAR, it shields against the damaging solar radiation (Blanquet and Phelan, 1987; Phelan et al., 2005). In coral reefs, the jellyfish plays critical roles in food web and nutrient cycling and the food web (Jantzen et al., 2010; Niggl et al., 2010; Zarnoch et al., 2020). Furthermore, Cassiopea medusae are mass-cultured as food for medusivores in captivity in aquaculture industry (Pierce, 2005). The jellyfish usually prefers calm and shallow marine habitat such as mangrove forest and lagoons, however, it is also common in shallow seagrass beds and coral reefs (Fleck and Fitt, 1999; Arai, 2001; Todd et al., 2006; Niggl and Wild, 2009; Jantzen et al., 2010). Cassiopea, temporarily, fixes its bell to the substrate (i.e., in an upside-down orientation) with help of its beating motion and mucus (Gohar and Eisawy, 1960). This unusual orientation maximizes the exposure of the symbiotic dinoflagellates to light for proper photosynthesis.
In general, scyphozoans grow rapidly and die en masse (Pitt et al., 2009). Therefore, jellyfish blooms are usually associated with the rapid release of massive amounts of organic matter, which could, temporally and spatially, change the physical and biological properties of confined water bodies (Pitt et al., 2009; Tinta et al., 2016). Jellyfish’s feeding menu varies, ranging from zooplankton to fish and their larvae (Breitburg et al., 1997; Mills, 2001). Scyphozoans are generally known to be robust and tolerate wild range of environmental stresses, such as temperature and pollutants (Aljbour et al., 2018; Aljbour et al., 2019). Furthermore, global warming and anthropogenic activities (e.g. eutrophication and coastal constructions) are proposed as the main drivers of jellyfish blooms (Arai, 2001; Mills, 2001; Purcell, 2005; Aljbour et al., 2018; Aljbour et al., 2019).
The subtropical Red Sea marine ecosystem, like most subtropical and tropical ecosystems, is oligotrophic and characterized by highly transparent waters due to low concentrations of ultraviolet radiation (UVR)-absorbing substances in the water column (Kheireddine et al., 2017; Overmans and Agustí, 2019). Furthermore, this ecosystem receives intense incident solar and UVR (Khogali and Al-Bar, 1992). Moreover, Overmans and Agustí (2020) demonstrated that UVR deeply penetrates the Red Sea water in moderately high doses, even at depths of 3 to 5 m. Therefore, marine organisms living in the shallow waters of this area are usually overexposed to harmful doses of UVR (Overmans and Agustí, 2019).
The UVR reaching the sea surface may represent, depending on the location, about the 6% of the incident solar radiation and is distributed in ~95% and ~5% in the UVR A (UV-A, 315 to 400 nm) and UVR B (UV-B, 280 to 315 nm) bands, respectively (Moan, 2001). Furthermore, UV-B and UV-A differ markedly in biological activity, water penetration, abundance in solar radiation, energy content, and damaging effects. While UV-B has significantly less penetration underwater, it has more damaging effects due to the higher energy content of its shorter wavelengths compared to UV-A. Moreover, UV-B is one of the most potent agents that adversely affect cellular homeostasis and genomic stability by inducing a variety of cytotoxic DNA lesions (Rastogi et al., 2010) and triggering reactive oxygen species (ROS) formation (Lesser, 1996; Lesser and Farrell, 2004; Lu and Wu, 2005). In contrast, UV-A, which is less damaging but more abundant than UV-B (i.e., 10 to 100 times more; Moan, 2001), contributes in activating the photoreactivation enzymes to repair UVR-damaged DNA (Hearst, 1995; Sancar, 1996; Rastogi et al., 2010).
Therefore, organisms have developed a variety of highly conserved defensive repair mechanisms to counteract the deleterious DNA-damaging effects of UVR. For example, the synthesis or acquisition of photoprotective UV-B-absorbing pigments (e.g., carotenoid, melanin, and mycosporine-like amino acids; Roy, 2000; Rossbach et al., 2020), and the evolution of DNA repair mechanisms such as base excision repair, and photoreactivation (using photolyases “alternatively called photoenzymes”; Sinha and Häder, 2002; Rastogi et al., 2010). In DNA, cyclobutane pyrimidine dimers (CPDs) and pyrimidine (6–4) pyrimidone photoproducts (6–4PPs) are the two major classes of UVR induced DNA helix distorting lesions (Sancar, 1996). DNA photoreactivating phosphorylases contain chromophores (i.e., as light harvesting antenna) and flavin adenine dinucleotide (FAD) molecule as a catalytic cofactor (Thoma, 1999). Interestingly, photolyases binds to UVR-damaged DNA and make use of light in the range of 350-450 nm (i.e., near UV-A and blue light) as an energy source to split the cyclobutane ring of CPDs, restoring the altered nucleotides to their native form (Hearst, 1995; Sancar, 1996). In nature, organisms exposed to UV-B are also exposed to UV-A and blue light. Thus while UVR (mainly UV-B) could cause DNA damage, the co-exposure to UV-A and blue light activate photoreactivating enzymes, alleviating the harmful impacts of shorter UVR to certain extent (Hearst, 1995; Sancar, 1996). Nevertheless, the ability of organisms to tolerate UVR varies considerably across various life stages, taxonomic groups, and prevailing environmental conditions (Alves et al., 2021; Alves et al., 2020; Leech and Williamson, 2000; Bancroft et al., 2007).
Compared to UV-A, UV-B has received more attention and is recognized to be harmful to the majority of marine animals, including fish and benthic invertebrates (Lesser, 1996; Häder et al., 2011; Torres-Pérez and Armstrong, 2012; Häder et al., 2015; Donner et al., 2017; Nordborg et al., 2021). Furthermore, the detrimental effects of UV-B are usually dose-dependent (Alves and Agustí, 2020). For example, Stylophora pistillata coral exhibited photoinhibition (Winters et al., 2003) and DNA breakages (Baruch et al., 2005) following UV-B exposure at levels comparable to those received at <5 m depths in the Red Sea. Furthermore, UV-B induced oxidative stress and cell death in the host tissue of shallow water coral while impairing the photosynthesis in symbiotic dinoflagellates and resulting in reduced fecundity of the holobiont (Lesser, 1996; Lesser and Farrell, 2004; Torres-Pérez and Armstrong, 2012). In sea urchins, 30 min of exposure to UV-A negatively affected sperm motility and fertilization and induced ROS production (Lu and Wu, 2005). According to Rijstenbil (2001), 4 h of daily exposure to UV-A for 4 weeks significantly increased superoxide dismutase and caused lipid peroxidation in Ditylum brightwellii diatoms. In Caenorhabditis elegans nematodes, UV-A triggered photoaging in DAF-16 dependent pathway (Prasanth et al., 2016). In contrast, in coral larvae and Symbiodiniaceae, UV-A alleviated the adverse effects of UV-B on photosynthesis (Zhou et al., 2016). The variation in UV sensitivity/tolerance in organisms could imply important consequences for community and ecosystem responses to the present and future predicted levels of UVR (associated with climate change and the stratospheric ozone depletion; Anderson et al., 2012).
In animal physiology, the onset of anaerobic metabolism indicates energy stress (i.e., elevated energy demand beyond organismal aerobic capacity; Bagwe et al., 2015). Therefore, transition to anaerobiosis is crucial to cope with accelerated energy demands under stressful conditions (Pörtner, 2002). Under normal physiological conditions (i.e., sufficient oxygen supply and undisturbed homeostasis), aerobic mitochondrial ATP production is sufficient to meet cellular energy requirements. Aerobic respiration is the primary and most efficient pathway of ATP synthesis in the animal kingdom. The maximum aerobic respiration potential (i.e., that supported by the existing enzymatic machinery) is estimated by measuring the activity of the mitochondrial electron transport system (ETS) in organisms carrying out aerobic respiration (Packard, 1971; Owens and King, 1975).
In contrast, anaerobic metabolism could be estimated by measuring the activity of pyruvate kinase (PK) and lactate dehydrogenase (LDH; Hochachka et al., 1983). In most animals, PK and LDH are the two primary enzymes controlling the glycolysis rate. In addition, PK mediates the conversion of phosphoenolpyruvate and ADP to pyruvate and ATP. Therefore, it sits at the crossroads between anabolism and catabolism of glucose carbons (Mazurek et al., 2002). In contrast, LDH channels pyruvate into anaerobic metabolism to maintain a sustained supply of ATP under anoxic/hypoxic conditions. Furthermore, the enzyme is a universally used biomarker of anaerobiosis because it correlates well with cellular anaerobic capacity (Hochachka et al., 1983).
There is little information on the effect of UV-B and UV-A radiation on the metabolic rate and biochemical composition of Cassiopea jellyfish. However, few studies investigated the effect of UVR on MAA synthesis by symbiotic dinoflagellates in Cassiopea (Banaszak and Trench, 1995), and scyphistomae proliferation and survival (Klein et al., 2016). While Banaszak and Trench (1995) demonstrated an elevated synthesis of MAA in symbiotic Cassiopea in response to UVR, Klein et al. (2016) found that UV-B adversely affected the scyphistomae health and survival. Nevertheless, both studies did not address differential effects of UV-A and UV-B on the metabolic rate and biochemical composition of Cassiopea medusae. In general, some jellyfish are robust and tolerant of many environmental disturbances. For example, the jellyfish acclimated well under high temperatures (32°C) incubations (Aljbour et al., 2017; Aljbour et al., 2018). Furthermore, the jellyfish were abundant in polluted areas with no signs of oxidative stress (Aljbour et al., 2018).
This study aims to develop a better understanding of the metabolic and physiological performance of Cassiopea at the cellular level in response to UVR. Therefore, we asked the following questions: Does UVR (i.e., UV-B alone and UV-B+UV-A combined) affect aerobic respiration and glycolytic potential in jellyfish? Do the defined conditions of UVR in this experiment affect the survival and growth of jellyfish? Do the UVR doses used in this experiment induce changes in available energy (Ea) resources (i.e., carbohydrates, lipids, and proteins)? Finally, does UV-A affect or modulate the jellyfish response to UV-B under the defined conditions in this experiment?
To answer these questions, we measured the activity of PK and LDH (i.e., the key regulatory enzymes of glycolysis), ETS activity (i.e., a proxy to evaluate the aerobic cellular respiration), and the total content of carbohydrates, lipids, and proteins to evaluate the available energy (Ea) in jellyfish. Therefore, we exposed Cassiopea sp. Medusae to three different light scenarios (i.e., PAR only as a control treatment, PAR + UV-B treatment, and PAR +UV-B and UV-A treatment) to investigate the differential effects of UV-B and UV-A on jellyfish. Overall, this is the first study on Cassiopea of its kind that rigorously addresses the metabolic and physiological performance of Cassiopea at the cellular level in response to UVR.
2. Materials and methods
2.1. Experimental animals
Medusae of Cassiopea sp. jellyfish were collected by gently scooping them (using a bucket) from shallow water at King Abdullah University of Science and Technology (KAUST) in August and September 2021. We immediately transferred the jellyfish to the aquarium facilities in the Coastal and Marine Resources Core Lab (CMR, in KAUST). In CMR, we maintained the jellyfish in a 300 L temperature-controlled (27.5 ± 0.7°C) aquarium tank supplied with filtered seawater (20 μm, salinity: 40.5 ± 0.5‰) until the commencement of the experiment in November 2021. The aquarium was lighted with PAR using radion light-emitting diodes (EcoTech Marine, USA) on a 12 h/12 h light/dark cycle. The PAR intensity ranged from 500 μmol·m-2·s-1 (high PAR) at the water surface to 171 μmol·m-2·s−1 (low PAR) at the aquarium bottom (water depth = 0.40 m). Three days before starting the experiment, we carefully selected 20 jellyfish and kept them at a depth of 0.10 m in a perforated tray in the same aquarium (i.e., receiving an average of 370 μmol·m-2·s-1; refer to Figure 1). We applied the selection criteria used by (Aljbour et al., 2017; Aljbour et al., 2019) to ensure that the selected jellyfish were morphologically healthy (i.e., no lost arms, pitched umbrella, or perforated bells). One day before the experiment (Day 0), we measured jellyfish size parameters (i.e., bell diameter = 3.5 ± 0.3 cm, wet body mass (WM) = 5.0 ± 0.5 g) to avoid putting extra stress on the organism on Day 1 of the experiment. The jellyfish bell diameter and body mass were measured according to the protocol used by (Aljbour et al., 2017; Aljbour et al., 2019).
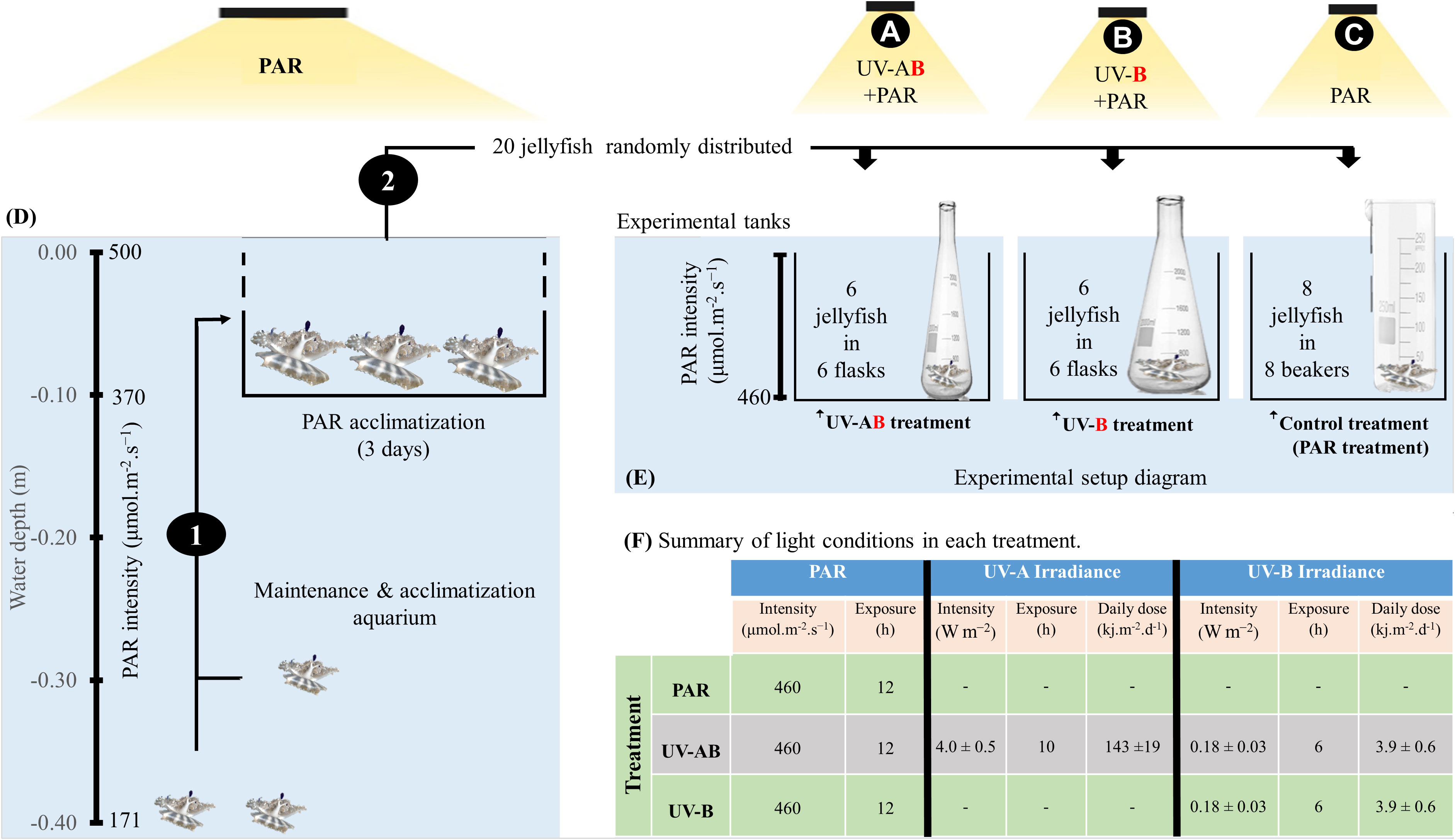
Figure 1 Diagram illustrating the jellyfish rearing and experimental design (D, E). (1) Jellyfish were transferred to an elevated tray at 0.10 m depth for 3 days to acclimatize them for higher photosynthetically active radiation (PAR) values. (2) Jellyfish were randomly distributed into three treatments (i.e., indicated by the letters A–C. Each organism represented a biological replica and was kept in a separate incubation chamber. (F) summarizes the light exposure conditions in each treatment. Refer to the materials and methods section for more detailed explanations.
2.2. UVR exposure
To investigate jellyfish responses to UV-B alone and highlight the role of UV-A in jellyfish responses to UV-B, we ran three UV-exposure treatments (refer to Figure 1). Treatment 1 was the control, alternatively called the PAR treatment. In this treatment (N = 8), jellyfish were only exposed to PAR (average intensity of 460 μmol·m-2·s-1; ca. 25% of natural PAR at sea surface) for 12 h a day on a 12 h/12 h light/dark cycle using radion light-emitting diodes (EcoTech Marine, USA). The PAR lights were automatically switched on at 6:00 am and off at 6:00 pm. Treatment 2 was the UV-AB treatment (i.e., UVA+UVB). In this treatment (N = 6), in addition to PAR exposure, the jellyfish were exposed to UV-A using Philips TL20W/12RS UV-B and UV-A broadband lamps (average intensity = 4.0 ± 0.5 W·m-2; dose = 143 ± 19 kJ·m−2·d-1) on a 10 h/14 h light/dark photoperiod and to UV-B (average intensity = 0.18 ± 0.03 W·m−2; dose = 3.9 ± 0.6 kJ·m-2·d-1) on a 6 h/16 h light/dark photoperiod. We switched UV−A lamps on and off at 8:00 am and 6:00 pm, respectively. In contrast, UV-B lamps were switched on and off at 10:00 am and 4:00 pm, respectively. Therefore, jellyfish were exposed to a combination of UV-A and UV-B light for 6 h a day (in addition to PAR). Furthermore, the delay in switching on the UV-B light might partially mimic the fact that UV-B reaches the earth at later hours than UV-A. Treatment 3 was the UV-B treatment (N = 6), where in addition to PAR exposure, the jellyfish were only exposed to UV-B (average intensity = 0.18 ± 0.03 W·m-2; dose = 3.9 ± 0.6 kJ·m-2·d-1) on a 6 h/16 h light/dark photoperiod only (refer to Figure 1F). Undetectable underwater UV-C levels were confirmed before the start of the experiment using the PMA2100 radiometer fitted with a UV Germicidal PMA2122-WP sensor (Solar LightTM, USA). When considering the averaged doses measured by Overmans and Agustí (2020) in the coastal Red Sea (34.6 and 1270 KJ m-2 d-1, for UVB and UVA respectively), the UVA and UVB doses provided in the experiment represented ~11.5% of the doses received at the surface.
All physical factors were held constant in all treatments (i.e., temperature, 27.5 ± 0.5°C, salinity, 40.5 ± 0.5‰). In addition, 0.8 L glass beakers were used as incubation chambers for jellyfish in PAR treatment, whereas 2.0 L wide mouth (7 cm) quartz flasks were used in both UV treatments. The quartz flasks were used because quartz allows UVR penetration, whereas glass does not allow UVR penetration. Jellyfish were fed (to repletion) every second day during the morning with freshly hatched Artemia nauplii. Water exchange (i.e., after 12 h of feeding) was achieved by removing about 90% of the incubation water and replacing it with filtered seawater at the same incubation temperature. To avoid stressing the jellyfish, we did not feed jellyfish on Days 1 and 16 (i.e., 24 h before sampling). Body mass was measured (according to the protocol from Aljbour et al., 2019) on Days 0 and 16 (i.e., 24 h before sampling).
2.3. Tissue sampling and homogenization
From each jellyfish (i.e., on day 17), the oral arms (four per tube) and the bell (i.e., devoid of gonadal tissue) were sampled in preweighed tubes and immediately snap-frozen in liquid nitrogen. Then, they were stored at -80°C until analyzed.
Oral samples were solely used for enzymatic assays and protein quantification (i.e., for enzyme activity normalization). For both ETS and glycolytic enzyme (PK and LDH) assays, we used the following common homogenization practice except for the type of the homogenization buffer specific for each enzyme set (buffer ingredients were described in detail by Aljbour et al., 2019). Oral arms (assigned for different enzymes) were double homogenized using the MicroDisTecTM MDT125 homogenizer (Thermo Fisher Scientific, USA) for <40 s and QIAGEN TissueLyser II (i.e., using about 0.5-g glass bead mixture; 0.4 and 1.0 mm diameter) and applying the following program (TN: 12×15, for 15 s) and immediately kept on ice to avoid heat build-up. Afterward, the resulting homogenates were centrifuged (5,000 x g for 5 min at 4°C), and the supernatants were distributed into different tubes for enzymatic activity measurement. All aliquots were snap-frozen in liquid nitrogen and kept at -80°C until analyzed.
Bell samples were double homogenized (without buffers) using the approach mentioned above. Therefore, the resultant homogenates (called crude homogenate hence after) were divided into aliquots (in preweighed tubes) and immediately snap-frozen in liquid nitrogen and stored at -80°C until analyzed. Bell crude homogenates were exclusively used for Ea estimation.
All enzymatic assays were performed at 28°C using a microplate spectrometer (SpectraMax, USA). Moreover, when we mention the word “supernatant” in the subsequent sections, we mean the above-described supernatant aliquots collected from the oral arms following the homogenization process. Similarly, the word “crude homogenate” refers to the nonbuffered bell homogenate aliquots described in the bell homogenization section.
2.4. Metabolic Enzyme Activity Assays
2.4.1. Electron transport system activity
We measured ETS activity using the common iodonitrotetrazolium (INT) reduction assay (Packard, 1971; Owens and King, 1975), following the methodology described in detail by Aljbour et al. (2019). This assay assumes that the INT reduction rate reflects the oxygen consumption rate. Technically, ETS enzymes mediate the reduction of INT to INT-formazan in the reaction mixture containing electron donors (i.e., nicotinamide adenine dinucleotide or NADH). We estimated the ETS-mediated oxygen consumption using the theoretical stoichiometric relationship that the formation of 2.0 µmol INT-formazan is equivalent to 1.0 µmol of O2 consumed. The increase in absorbance at 490 nm was followed spectrophotometrically. Then, the results were calculated based on the corrected slopes and presented in milligrams of O2 per hour per milligram of protein. Protein concentration was determined spectrophotometrically at 595 nm following the Bradford (1976) method, using the bovine serum albumin to build the standard curve.
2.4.2. Glycolytic Enzyme Activity
We measured the activity of PK (EC 2.7.1.40) according to Hickey and Clements (2003), following the methodology described in detail by Aljbour et al. (2018). Technically, pyruvate conversion to lactate is associated with NADH oxidation, which changes the absorbance at 340 nm. The results were calculated based on the corrected slopes and presented as milliunits per milligram of protein (mU·mg protein-1.) In addition, LDH (EC 1.1.1.27) activity was measured according to (Lushchak et al., 1998; Lushchak et al., 2001), following the methodology described in detail by Aljbour et al. (2018).
2.5. Biochemical composition quantification (available energy resources)
We measured the total soluble protein and carbohydrate content of the bell tissue following the methods described by De Coen and Janssen (1997). Bell crude homogenates were deproteinated with 12% Trichloroacetic acid (0.2 mL per ca. 0.08 g of bell tissue) aided by incubation at -20°C for 10 min. Following centrifugation (1000 x g for 10 min), supernatants were recovered in new tubes, and the carbohydrates were quantified with anthrone (Van Handel, 1965), using glucose as a standard with absorbance at 630 nm. The anthrone reagent was prepared fresh right before analysis. The results are presented as milligrams of glucose per gram of WM. The pellets were resuspended in 1.0 N of NaOH and incubated at 60°C for 30 min. Finally, they were neutralized with 2.0 M of HCl, and the protein concentration was determined spectrophotometrically at 595 nm following the Bradford (1976) method, using the bovine serum albumin to build the standard curve. Results are presented as milligrams of protein per gram of WM.
Total lipids were extracted following Bligh and Dyer (1959), following the methods outlined by De Coen and Janssen (1997). Bell crude homogenates were mixed with a 2.0 volume of chloroform and methanol and a 0.85 volume of H2O. Following centrifugation (1000 x g for 5 min), 0.13 mL of the organic phase was transferred to a capped glass vial containing 0.65 mL of H2SO4 and was charred at 200°C for 15 min. Then, 0.5 mL of the charred mixture was mixed with 1.13 mL of H2O and centrifuged at 15,000 x g for 15 min. The total lipid was determined by measuring the absorbance at 375 nm. Tripalmitin was used as a standard to build the standard curve. The results are presented as milligrams of tripalmitin per gram of WM.
2.6. Statistical analysis
Before conducting the analysis, all data were tested for compliance with the statistical test assumptions, normality, and homoscedasticity. Nonparametric tests were applied when assumptions were violated. The Kruskal–Wallis test and Dunn’s Kruskal–Wallis multiple comparisons were applied to test for significant variation between groups. Spearman’s rank correlation test was applied to test for the correlation between variables. We used the R software (R Foundation for Statistical Computing) to conduct the statistical analysis. A p-value <.05 was used as the borderline of statistical significance to reject the null hypothesis for each test, and the word “significant” was used only if the p-value of the test was <.05.
3. Results
3.1. Changes in jellyfish body mass
While the jellyfish in the control treatment significantly increased their WM by about 40% (Wilcoxon signed-rank test with continuity correction: V = 0, p = .01), neither the UV-B-exposed nor UV-AB-exposed jellyfish demonstrated any significant change in body mass (Figure 2A). However, we did not notice any abnormal behavioural or morphological changes (i.e., bell perforation or oral arm complex separation) during the experiment in either treatment.
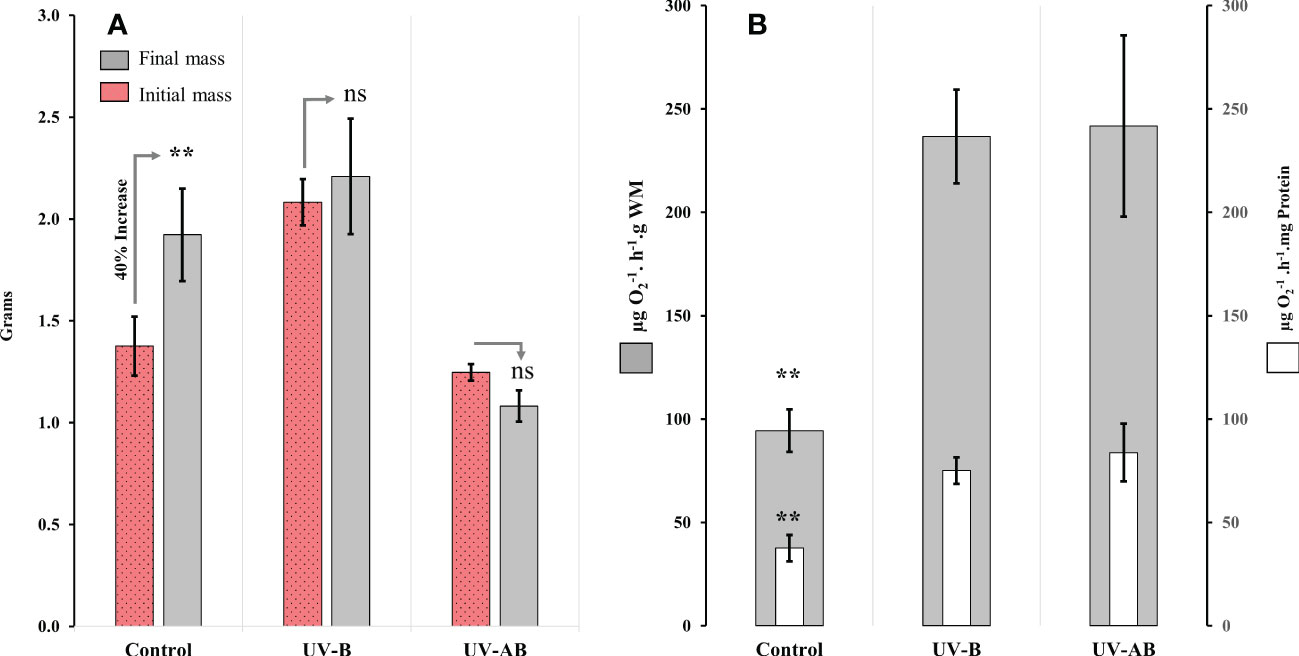
Figure 2 Body mass and aerobic respiration response of Cassiopea to UVR. (A) Wet body mass at the beginning and the end of the experiment; N = 8 (control), 6 (UV-B), and 6 (UV-AB). Statistical significance was calculated using Wilcoxon signed-rank test. (B) Mitochondrial electron transport system (ETS) activity in oral arms at the end of the experiment; N = 8 (control), 6 (UV-B), and 5 (UV-AB). Bars present the data in two normalization methods: 1- activity per wet body mass (i.e., external bars), 2- activity per total protein content (internal bars). Statistical significance was tested using Dunn (1964) Kruskal–Wallis multiple comparison test. In both panels, Data represent the mean ± SE. An asterisk above bars means statistical significance (∗p ≤ 0.05, ∗∗p ≤ 0.01). An “ns” above the bars indicates nonsignificant differences.
3.2. Enzymatic activity responses
The ANOVA using the Kruskal–Wallis test revealed that UVR exposure significantly affected ETS activity in jellyfish in at least one treatment (χ2 = 9.9 df = 2, p <.01; Figure 2B). The results of the post hoc analysis using the Dunn (1964) Kruskal–Wallis multiple comparisons indicated that the ETS activity was significantly higher (i.e., 98% to 120%) in UV-B and UV-AB-exposed jellyfish when compared pairwise to the control group (p <.01; Figure 2B). However, the ETS activity in the UV-B and UV-AB-exposed jellyfish was not significantly different when compared pairwise to each other (Dunn (1964) Kruskal–Wallis multiple comparison test: Z = 0.2398773, p = .81; Figure 2B).
Moreover, UV exposure significantly affected the activity of PK in jellyfish in at least one treatment (Kruskal–Wallis test: χ2 = 10.517, df = 2, p <.01). The PK activity was significantly lower (by 46%) in both UV-B and UV-AB-exposed jellyfish when compared pairwise to the control group (Dunn’s (1964) Kruskal–Wallis multiple comparison test: p <.01; Figure 3A). However, pairwise comparison of PK activity between UV-exposed jellyfish did not detect any significant differences between UV-B and UV-AB-treated jellyfish when compared pairwise to each other (Dunn’s Kruskal–Wallis multiple comparison test: Z = 0.098, p = .92; Figure 3A). In contrast, UV exposure did not have any significant effect on LDH activity in either treatment (Kruskal–Wallis rank sum test: χ2 = 3.137, df = 2, p >.05; Figure 3B).
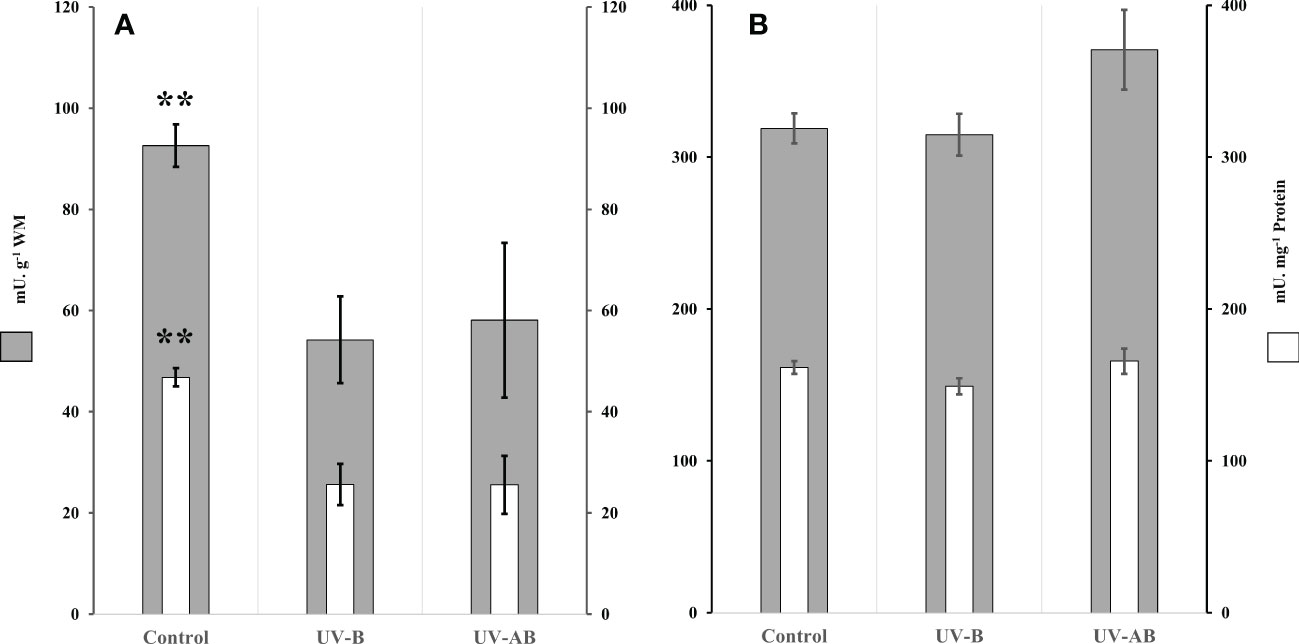
Figure 3 Glycolytic enzymes activity in Cassiopea oral arms in response to UVR. (A) Pyruvate kinase activity (PK); N = 8 (control), 6 (UV-B), and 6 (UV-AB). (B) Lactate dehydrogenase (LDH) activity; N = 8 (control), 5 (UV-B), and 6 (UV-AB). Data represent the mean ± SE; an asterisk above bars means statistical significance (∗p ≤ 0.05, ∗∗p ≤ 0.01). Statistical significance was tested using Dunn (1964) Kruskal–Wallis multiple comparison test. Bars present the data in two normalization methods: 1- activity per wet body mass (i.e., external bars), 2- activity per total protein content (internal bars).
Using the enzymatic ratio analysis, we found that the PK/LDH ratio was significantly different in at least one treatment (one-way ANOVA: df = 2, F = 9.05, p = .002). The ratio was significantly lower in both UV-B and UV-AB-exposed jellyfish when compared pairwise to the control group (Tukey’s HSD test for multiple comparison test: p <.05; Figure 4A). However, the ratio did not differ between UV-B and UV-AB-exposed jellyfish when compared pairwise to each other (Tukey’s HSD test for multiple comparison test: 95% confidence interval [0.032, 0.132], p = .70). We did not find any correlation between PK and LDH. In contrast, when the data from all treatments were combined, the PK was inversely correlated to the ETS activity (Spearman’s rank correlation rho: p <.002, rho = -0.66). However, at the treatment levels, PK did not correlate with ETS. The ETS activity was negatively correlated with the PK/LDH ratio when data from all treatments were combined (Spearman’s rank correlation rho: -0.58, p = .002; Figure 4B).
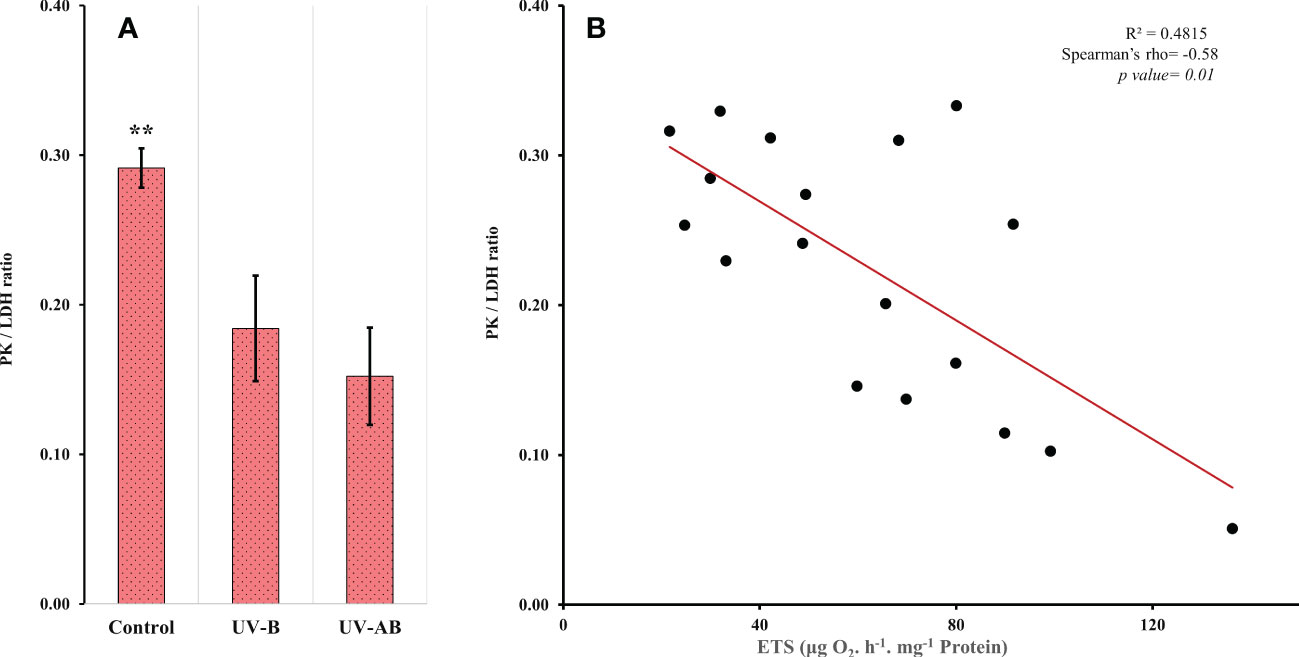
Figure 4 (A) Pyruvate kinase (PK)/lactate dehydrogenase (LDH) activity ratio. N = 8 (control), 5 (UV-B), and 6 (UV-AB). Data represent the mean ± SE; an asterisk above bars means statistical significance (∗p ≤ 0.05, ∗∗p ≤ 0.01). Statistical significance was tested using Tukey HSD test for multiple comparisons. (B) Scatter plot showing the correlation between the ETS and PK/LDH ratio. Spearman’s test.
3.3. Energy allocation parameters (carbohydrates, lipids, and total protein content)
The ANOVA using the Kruskal–Wallis test did not reveal any significant effect of UVR on the jellyfish Ea parameters in any treatment (Kruskal–Wallis rank sum test: p >.2 for all parameters; Figure 5). When we performed the correlation analysis, the correlation type (if present) between enzymatic activity and Ea parameters was always a negative correlation. For example, LDH negatively correlated with the lipid content in UV-B-exposed jellyfish (Spearman’s test: rho = -1, p = .017), and PK negatively correlated with carbohydrates content in the same treatment (Spearman’s test: rho = -0.93, p = .008). The ETS, LDH, and PK did not correlate with any Ea parameter in any other treatment except for the mentioned cases. We measured Ea and enzymatic activities in different tissue types (umbrella (bell) vs. oral arms).
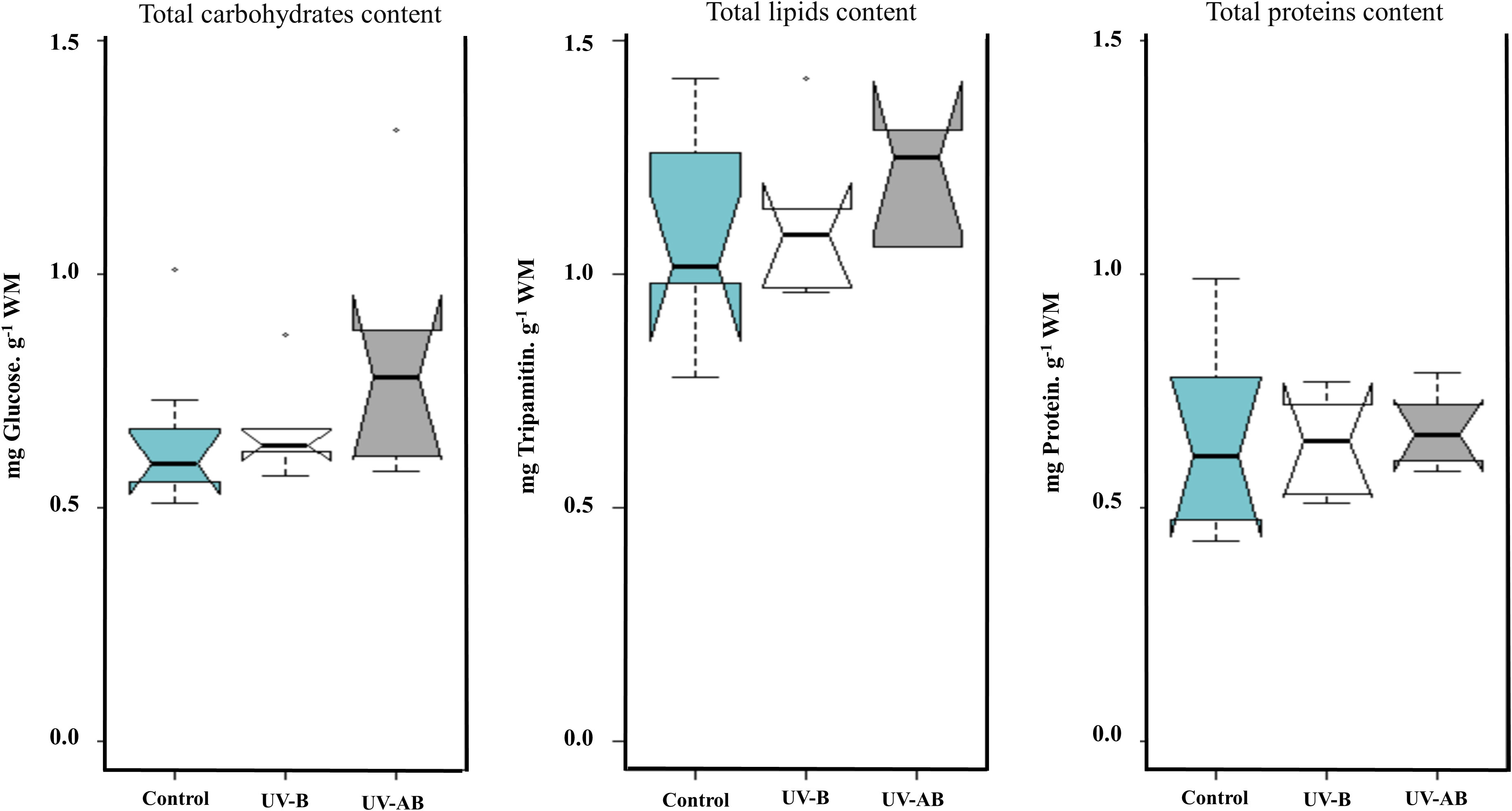
Figure 5 Cellular available energy (Ea) content in Cassiopea umbrella (bell) in response to UVR. Treatments were pairwise-compared to test for any significant differences in Ea (i.e., carbohydrates, lipids, and soluble protein). N = 8 (control), 6 (UV-B), and 6 (UV-AB). Statistical significance was tested using Kruskal–Wallis rank sum test.
4. Discussion
In this study, while aerobic metabolism increased significantly in terms of ETS activity in UV-exposed jellyfish compared to the control group, we did not detect signs of anaerobiosis (e.g., unchanged LDH) in any treatment. We discuss the findings considering the effect of UVR on other organisms due to the lack of research on jellyfish addressing this topic.
In general, organisms vary greatly in their response to UVR. For example, while a 2-h exposure to high PAR supplemented with UV-A + UV-B significantly stimulated the aerobic respiration rate in the green alga Selenastrum capricornutum, it significantly inhibited the respiration rate in the cyanobacterium Aphanizomenon flos-aquae (Beardall et al., 1997). According to Chuang et al. (2006), UV-A or UV-B significantly decreased the respiration rate in the earthworm Amynthas gracilis. In contrast to earthworms, in the present study, the ETS activity was significantly higher (98% to 120%) in all UV-treated jellyfish compared to the control group, which indicates a higher aerobic cellular respiration rate and oxygen uptake. However, these highly respiring jellyfish did not display equivalent increases in body mass (i.e., compared to about a 40% increase in body mass of the control group). Therefore, this mismatch between a high respiration rate and a reduced growth rate might indicate an elevated energy demand associated with UVR exposure (i.e., more energy is allocated to maintain cellular homeostasis due to ROS and DNA repair, for example). According to Aljbour et al. (2019), the ETS activity was significantly high in Cassiopea medusae incubated at a cold temperature (20°C) for two weeks. Moreover, high ETS activity was associated with oxidative stress-mediated cellular damage and significant body mass losses. Furthermore, the medusae were morphologically unhealthy, with shrunk oral arms and perforated bells (Aljbour et al., 2019). In the present study, however, the survival rate of jellyfish was 100%, and they appeared morphologically healthy, suggesting that the jellyfish is more resilient to UVR than to temperature drops.
Many scyphozoan jellyfish might thrive/bloom in response to climate change-driven changing environmental factors (e.g., a rising seawater temperature and eutrophication; Graham, 2001; Purcell et al., 2007; Gambill and Peck, 2014). According to (Aljbour et al., 2017; Aljbour et al., 2019), Cassiopea sp. attained larger sizes and enhanced performance when incubated at 32°C for 2 weeks. In the oligotrophic subtropical/tropical marine reef ecosystem (the Red Sea), a high SWT is usually associated with high doses of UVR, even at depths of 3 to 5 m (Overmans and Agustí, 2020). Therefore, marine organisms living in shallow waters of this area might be overexposed to harmful doses of UVR (Overmans and Agustí, 2019). According to Zhou et al. (2016), a high seawater temperature exacerbated the effect of UVR exposure on coral. Furthermore, UVR decreased the concentration of chlorophyll only at higher SWTs or higher UVR doses/intensities (Zhou et al., 2016). Compared to the findings of (Aljbour et al., 2017; Aljbour et al., 2019), the results from the present study might indicate that Cassiopea sp. is more sensitive to UVR than to rising seawater temperature. However, this conclusion is beyond the goals of this study and requires further investigation.
Under normal physiological conditions, aerobic respiration generates enough ATP to suffice the cellular energy demands. However, when the aerobic scope declines severely and the Krebs cycle is about to shut down due to an insufficient O2 supply, the onset of anaerobiosis becomes essential to sustain cellular energy demands (Pörtner). When the Krebs cycle shuts down, NADH builds up and exerts negative feedback inhibitory actions on glycolysis. However, the LDH-mediated re-oxidation of NADH to NAD+ frees glycolysis from the inhibitory action of NADH. Therefore, energy production from glycolysis can continue.
In invertebrate toxicological studies, LDH is commonly used as a proxy for anaerobiosis (Hochachka et al., 1983). For example, in adductor muscles of the green mussel Perna viridis, copper treatment caused higher activity and the onset of anaerobiosis (Aanand et al., 2010). In the present study, UV-treated Cassiopea sp. did not exhibit changes in LDH activity or Ea (carbohydrates, lipids, and protein content) compared to the control group, which might rule out the onset of anaerobic metabolism under the defined experimental conditions. In a recent study, Enrique-Navarro et al. (2022) demonstrated that the umbrella tissue of Cotylorhiza tuberculate jellyfish could effectively absorb light in the UV region and attenuate the light intensity inside the jellyfish. Furthermore, they suggested a protective role of the jellyfish tissue itself, demonstrating an added reason to explain the success of the Cotylorhiza tuberculata population (Enrique-Navarro et al., 2022). The jellyfish seems to have high resilience (i.e., compared to coral) to UV and a higher ability to aerobically cope with elevated energy demands without switching their metabolism into anaerobic mode.
Interestingly, PK activity unexpectedly decreased in UV-exposed jellyfish. According to Aljbour et al. (2018), PK activity was significantly higher in jellyfish from heavy-metal polluted aquatic habitats. The authors related this increase to the enhanced anaerobic metabolism of the jellyfish. Furthermore, Dailianis and Kaloyianni (2004) observed the same pattern of PK activity change in Cd-treated mollusks. While a low PK/LDH ratio usually indicates enhanced anaerobic glycolysis, the unchanged LDH activities combined with the decreased PK activities in UV-exposed jellyfish rule out this possibility in the present study.
Furthermore, we found that ETS activity is inversely correlated to PK and the PK/LDH ratio when data from all treatments were combined. However, at the treatment levels, PK did not correlate with the ETS. However, these data do not imply causality and require more experiments with a higher number of organisms to decipher the correlation at treatment levels.
The UVR (mainly UV-B) could initiate oxidative stress (Lesser, 1996; Lesser and Farrell, 2004; Lu and Wu, 2005) and induce DNA damage (Rastogi et al., 2010) in marine organisms. Therefore, it adversely affects genomic stability and cellular homeostasis. In coral, UV-B impaired photosynthesis initiated DNA damage and triggered ROS formation (Lesser, 1996; Torres-Pérez and Armstrong, 2012; Donner et al., 2017; Nordborg et al., 2021). In contrast, UV-A might induce varying effects in different organisms. For example, UV-A adversely affected sea urchin productivity (Lu and Wu, 2005). Furthermore, UV-A increased superoxide dismutase activity and lipid peroxidation rate in the Distyle brightwellii diatom. In contrast, UV-A minimizes the adverse effects of UV-B on photosynthesis in coral larvae and Symbiodinium (Zhou et al., 2016). In Cassiopea sp., all UV-B and (UV-B+UV-A)-treated jellyfish responded similarly (i.e., in terms of all response parameters tested in this study). Therefore, it seems that the presence of UV-A did not synergize or hinder the effects of UV-B in jellyfish under the defined conditions in this experiment.
5. Conclusion
The results demonstrate that Cassiopea sp. was aerobically poised to deal with UVR-mediated elevated energy demands. Furthermore, the jellyfish did not switch to anaerobic metabolism, as Ea and LDH activity remained unchanged in UVR-treated jellyfish. The UVR did not affect jellyfish survival while reducing their growth rate. Although UV-A might exacerbate/modulate the effect of UV-B on different marine organisms, the results indicate that UV-A did not seem to amplify or modulate UV-B effects on jellyfish physiology and growth. However, the findings suggest that the jellyfish is more resilient (i.e., concerning unaffected survival) to UVR than other cnidarians.
Data availability statement
The raw data supporting the conclusions of this article will be made available by the authors, without undue reservation.
Author contributions
SMA, RA, and SA conceived and designed the study. SMA conducted the jellyfish sampling from the field and performed enzymatic and biochemical analyses. All authors contributed to the writing of the manuscript and critically read and approved the final version. All authors contributed to the article and approved the submitted version.
Funding
This work was supported by the King Abdullah University of Science and Technology through baseline funding to Prof. SA.
Acknowledgments
We would like to acknowledge the effort of the CMR team at KAUST. I would like to extend my sincere thanks to Aussama Alzeem for his excellent assistance in the field.
Conflict of interest
The authors declare that the research was conducted in the absence of any commercial or financial relationships that could be construed as a potential conflict of interest.
Publisher’s note
All claims expressed in this article are solely those of the authors and do not necessarily represent those of their affiliated organizations, or those of the publisher, the editors and the reviewers. Any product that may be evaluated in this article, or claim that may be made by its manufacturer, is not guaranteed or endorsed by the publisher.
References
Aanand S., Purushothaman C. S., Pal A. K., Rajendran K. V. (2010). Toxicological studies on the effect of copper lead and zinc on selected enzymes in the adductor muscle and intestinal diverticula of the green mussel perna viridis. Indian J. Mar. Sci. 39 (2), 299–302.
Aljbour S. M., Al-Horani F., Kunzmann A. (2018) Metabolic and oxidative stress responses of the jellyfish cassiopea to pollution in the gulf of aqaba Jordan. Mar. pollut. Bull 130, 271–278. doi: 10.1016/j.marpolbul.2018.03.044
Aljbour S. M., Zimmer M., Al-Horani F., Kunzmann A. (2019). Metabolic and oxidative stress responses of the jellyfish cassiopea sp. to changes in seawater temperature. J. Sea. Res. 145, 1–7. doi: 10.1016/j.seares.2018.12.002
Aljbour S. M., Zimmer M., Kunzmann A. (2017). Cellular respiration oxygen consumption and trade-offs of the jellyfish cassiopea sp. in response to temperature change. J. Sea. Res. 128, 92–97. doi: 10.1016/j.seares.2017.08.006
Alves R., Agustí S. (2020). Effect of ultraviolet radiation (UVR) on the life stages of fish. Rev. Fish. Biol. Fisher. 30 (2), 335–372. doi: 10.1007/s11160-020-09603-1
Alves R. N., Mahamed A. H., Alarcon J. F., Al Suwailem A., Agustí S. (2020). Adverse effects of ultraviolet radiation on growth, behavior, skin condition, physiology, and immune function in gilthead seabream (sparus aurata). Front. Mar. Sci. 7:306. doi: 10.3389/fmars.2020.00306
Alves R. N., Justo M. S. S., Laranja J. L. Q., Alarcon J. F., Al Suwailem A., Agustí S. (2021). Exposure to natural ultraviolet b radiation levels has adverse effects on growth behavior physiology and innate immune response in juvenile European seabass (Dicentrarchus labrax). Aquaculture 533, 736215. doi: 10.1016/j.aquaculture.2020.736215
Anderson J. G., Wilmouth D. M., Smith J. B., Sayres D. S. (2012). UV Dosage levels in summer: Increased risk of ozone loss from convectively injected water vapor. Science 337 (6096), 835–839. doi: 10.1126/science.1222978
Arai M. N. (2001). Pelagic coelenterates and eutrophication: a review. Hydrobiologia 451, 69–87. doi: 10.1023/A:1011840123140
Bagwe R., Beniash E., Sokolova I. M. (2015). Effects of cadmium exposure on critical temperatures of aerobic metabolism in eastern oysters crassostrea virginica (Gmelin 1791). Aquat. Toxicol. 167, 77–89. doi: 10.1016/j.aquatox.2015.07.012
Banaszak A. T., Trench R. K. (1995). Effects of ultraviolet (UV) radiation on marine microalgal-invertebrate symbioses. II. the synthesis of mycosporine-like amino acids in response to exposure to UV in anthopleura elegantissima and cassiopea xamachana. J. Exp. Biol. Ecol. 194, 233–250. doi: 10.1016/0022-0981(95)00073-9
Bancroft B., Baker N., Blaustein A. (2007). Effects of UVB radiation on marine and freshwater organisms: a synthesis through meta-analysis. Ecol. Lett. 10 (4), 332–345. doi: 10.1111/j.1461-0248.2007.01022.x
Baruch R., Avishai N., Rabinowitz C. (2005). UV Incites diverse levels of DNA breaks in different cellular compartments of a branching coral species. J. Exp. Biol. 208 (5), 843–848. doi: 10.1242/jeb.01496
Beardall J., Berman T., Markager S., Martinez R., Montecino V. (1997). The effects of ultraviolet radiation on respiration and photosynthesis in two species of microalgae. Can. J. Fisher. Aquat. Sci. 54 (3), 687–696. doi: 10.1139/f96-320
Blanquet R. S., Phelan M. A. (1987). An unusual blue mesogleal protein from the mangrove jellyfish cassiopea xamachana. Mar. Biol. 94, 423–430. doi: 10.1007/BF00428249
Bligh E. G., Dyer W. J. (1959). A rapid method of total lipid extraction and purification. Can. J. Biochem. Physiol. 37 (8), 911–917. doi: 10.1139/y59-099
Bradford M. M. (1976). A rapid and sensitive method for the quantitation of microgram quantities of protein utilizing the principle of protein-dye binding. Analytical. Biochem. 72 (1-2), 248–254. doi: 10.1016/0003-2697(76)90527-3
Breitburg D. L., Loher T., Pacey C. A., Gerstein A. (1997). Varying effects of low dissolved oxygen on trophic interactions in an estuarine food web. Ecol. Monogr. 67 (4), 489–507. doi: 10.1890/0012-9615(1997)067[0489:VEOLDO]2.0.CO;2
Cates N. (1975). Productivity and organic consumption in cassiopea and conductylus. J. Exp. Biol. Ecol. 18, 55–59. doi: 10.1016/0022-0981(75)90016-7
Chuang S. C., Lai W. S., Chen J. H. (2006). Influence of ultraviolet radiation on selected physiological responses of earthworms. J. Exp. Biol. 209 (21), 4304–4312. doi: 10.1242/jeb.02521
Dailianis S., Kaloyianni M. (2004). Cadmium induces both pyruvate kinase and Na+/H+ exchanger activity through protein kinase c mediated signal transduction in isolated digestive gland cells of mytilus galloprovincialis (L.). J. Exp. Biol. 207 (10), 1665–1674. doi: 10.1242/jeb.00925
De Coen W., Janssen C. (1997). The use of biomarkers in Daphnia magna toxicity testing. IV. cellular energy allocation: a new methodology to assess the energy budget of toxicant-stressed daphnia populations. J. Aquat. Ecosyst. Stress Recovery. 6, 43–55. doi: 10.1023/A:1008228517955
Donner S., Rickbeil G., Heron S. (2017). A new high-resolution global mass coral bleaching database. PloS One 12 (4), e0175490. doi: 10.1371/journal.pone.0175490
Dunn O. J. (1964). Multiple comparisons using rank sums. Technometrics 6(3), 241–252. doi: 10.1080/00401706.1964.10490181
Enrique-Navarro A., Huertas E., Flander-Putrle V., Bartual A., Navarro G., Ruiz J., et al. (2022). Living inside a jellyfish: The symbiosis case study of host-specialized dinoflagellates “Zooxanthellae” and the scyphozoan cotylorhiza tuberculataFront. Mar. Sci.9, 817312. doi: 10.3389/fmars.2022.817312
Fleck J., Fitt W. K. (1999). Degrading mangrove leaves of rhizophora mangle linne provide a natural cue for settlement and metamorphosis of the upside-down jellyfish Cassiopea xamachana Bigelow. J. Exp. Biol. Ecol. 234, 83–94. doi: 10.1016/S0022-0981(98)00140-3
Gambill M., Peck M. (2014). Respiration rates of the polyps of four jellyfish species: Potential thermal triggers and limits. J. Exp. Mar. Biol. Ecol. 459, 17–22. doi: 10.1016/j.jembe.2014.05.005
Gohar H. A. F., Eisawy A. M. (1960). The development of Cassiopea andromeda. Publ. Mar. Biol. Stat. 11, 148–190.
Graham W. M. (2001). Numerical increases and distributional shifts of chrysaora quinquecirrha (Desor) and aurelia aurita (Linnè) (Cnidaria: Scyphozoa) in the northern gulf of Mexico. Hydrobiologia 451, 97–111. doi: 10.1023/A:1011844208119
Häder D., Helbling E., Williamson C., Worrest R. (2011). Effects of UV radiation on aquatic ecosystems and interactions with climate change. Photochem. Photobiolog. Sci. 10 (2), 242–260. doi: 10.1039/c0pp90036b
Häder D., Williamson C., Wängberg S., Rautio M., Rose K., Gao K., et al. (2015). Effects of UV radiation on aquatic ecosystems and interactions with other environmental factors. Photochem. Photobiolog. Sci. 14 (1), 108–126. doi: 10.1039/c4pp90035a
Hearst J. (1995). The structure of photolyase: Using photon energy for DNA repair. Science 268 (5219), 1858–1859. doi: 10.1126/science.7604259
Hickey A. J. R., Clements K. D. (2003). Key metabolic enzymes and muscle structure in triplefin fishes (Tripterygiidae): a phylogenetic comparison. J. Comp. Physiol. B. 173 (2), 113–123. doi: 10.1007/s00360-002-0313-9
Hochachka P. W., Stanley C., Merkt J., Sumar-Kalinowski J. (1983). Metabolic meaning of elevated levels of oxidative enzymes in high altitude adapted animals: an interpretive hypothesis. Respir. Physiol. 52 (3), 303–313. doi: 10.1016/0034-5687(83)90087-7
Jantzen C., Wild C., Rasheed M., El-Zibdah M., Richter C. (2010). Enhanced pore-water nutrient fluxes by the upside-down jellyfish cassiopea sp. in a red Sea coral reef. Mar. Ecol. Prog. Ser. 411, 117–125. doi: 10.3354/meps08623
Kheireddine M., Ouhssain M., Claustre H., Uitz J., Gentili B., Jones B. (2017). Assessing pigment-based phytoplankton community distributions in the red Sea. Front. Mar. Sci. 4. doi: 10.3389/fmars.2017.00132
Khogali A., Al-Bar O. F. (1992). A study of solar ultraviolet-radiation at makkah solar station. Solar. Energy 48, 79–87. doi: 10.1016/0038-092X(92)90036-A
Klein S. G., Pitt K. A., Carroll A. R. (2016). Surviving but not thriving: inconsistent responses of zooxanthellate jellyfish polyps to ocean warming and future UV-b scenarios. Sci. Rep. 6 (1), e28859. doi: 10.1038/srep28859
Kremer P., Costello J., Kremer J., Canino M. (1990). Significance of photosynthetic endosymbionts to the carbon budget of the scyphomedus linuche unguiculata. Limnol. Oceanogr. 35, 609–624. doi: 10.4319/lo.1990.35.3.0609
Leech D., Williamson C. (2000). Is tolerance to uv radiation in zooplankton related to body size taxon or lake transparency? Ecol. Appl. 10 (5), 1530–1540. doi: 10.1890/1051-0761(2000)010[1530:itturi]2.0.co;2
Lesser M. P. (1996). Elevated temperatures and ultraviolet radiation cause oxidative stress and inhibit photosynthesis in symbiotic dinoflagellates. Limnol. Oceanogr. 41 (2), 271–283. doi: 10.4319/lo.1996.41.2.0271
Lesser M. P., Farrell J. H. (2004). Exposure to solar radiation increases damage to both host tissues and algal symbionts of corals during thermal stress. Coral. Reefs. 23 (3), 367–377. doi: 10.1007/s00338-004-0392-z
Lushchak V. I., Bagnyukova T. V., Storey J. M., Storey K. B. (2001). Influence of exercise on the activity and the distribution between free and bound forms of glycolytic and associated enzymes in tissues of horse mackerel. Braz. J. Med. Biol. Res. 34 (8), 1055–1064. doi: 10.1590/S0100-879X2001000800013
Lushchak V. I., Bahnjukova T. V., Storey K. B. (1998). Effect of hypoxia on the activity and binding of glycolytic and associated enzymes in sea scorpion tissues. Braz. J. Med. Biol. Res. 31 (8), 1059–1067. doi: 10.1590/S0100-879X1998000800005
Lu X. Y., Wu R. S. S. (2005). UV Induces reactive oxygen species damages sperm and impairs fertilisation in the sea urchin anthocidaris crassispina. Mar. Biol. 148 (1), 51–57. doi: 10.1007/s00227-005-0049-7
Mazurek S., Grimm H., Boschek C. B., Vaupel P., Eigenbrodt E. (2002). Pyruvate kinase type M2: a crossroad in the tumor metabolome. Br. J. Nutr. 87 (1), 23–29. doi: 10.1079/BJN2001454
McCloskey L. R., Muscatine L., Wilkerson F. P. (1994). Daily photosynthesis, respiration, and carbon budgets in a tropical marine jellyfish (mastigias sp). Marine Biology 119 (1), 3–22. doi: 10.1007/bf00350101
Mills C. E. (2001). Jellyfish blooms: are populations increasing globally in response to changing ocean conditions? Hydrobiologia 451, 55–68. doi: 10.1007/978-94-010-0722-1_6
Moan J. (2001). “Visible light and UV radiation,” in Radiation at home outdoors and in the workplace. Eds. Brune A., Hellborg R., Persson B. R. R., Pääkkönen R. (Oslo: Scandinavian Science Publisher), p 69–p 85.
Nabipour I., Moradi M., Mohebbi G. H. (2015). A first record on population of the alien venomous jellyfish Cassiopea andromeda (Forsskål 1775) (Cnidaria: Scyphozoa: Rhizostomea) in the nayband lagoon from bushehr-Iran (Persian gulf). J. Chem. Pharm. Res. 7, 1710–1713.
Niggl W., Naumann M. S., Struck U., Manasrah R., Wild C. (2010). Organic matter release by the benthic upside-down jellyfish cassiopea sp. fuels pelagic food webs in coral reefs. J. Exp. Mar. Biol. Ecology. 384, 99–106. doi: 10.1016/j.jembe.2010.01.011
Niggl W., Wild C. (2009). Spatial distribution of the upsidedown jellyfish cassiopea sp. within fringing coral reef environments of the northern red Sea: implications for its life cycle. Helgoland. Mar. Res. 64, 281–287. doi: 10.1007/s10152-009-0181-8
Nordborg F., Brinkman D., Ricardo G., Agustí S., Negri A. (2021). Comparative sensitivity of the early life stages of a coral to heavy fuel oil and UV radiation. Sci. Total. Environ. 781, e146676. doi: 10.1016/j.scitotenv.2021.146676
Overmans S., Agustí S. (2019). Latitudinal gradient of UV attenuation along the highly transparent red Sea basin. Photochem. Photobiol. 95 (5), 1267–1279. doi: 10.1111/php.13112
Overmans S., Agustí S. (2020). Unraveling the seasonality of UV exposure in reef waters of a rapidly warming (Sub-)tropical Sea. Front. Mar. Sci. 7. doi: 10.3389/fmars.2020.00111
Owens T. G., King F. D. (1975). The measurement of respiratory electron-transport-system activity in marine zooplankton. Mar. Biol. 30 (1), 27–36. doi: 10.1007/BF00393750
Özbek E.Ö., Oztürk B. (2015). The new location record of Cassiopea andromeda (Forsskå) from asin bay gulf of güllük muğla Aegean coast of Turkey. J. Black. Sea. /. Mediterr. Environ. 21 (1), 96–101.
Packard T. T. (1971). The measurement of respiratory electron transport activity in marine plankton. J. Mar. Res. 29, 235–244.
Phelan M., Matta J., Reyes Y., Fernando R., Boykins R., Blanquet R. (2005). Associations between metals and the blue mesogleal protein of cassiopea xamachana. Mar. Biol. 149 (2), 307–312. doi: 10.1007/s00227-005-0189-9
Pierce J. (2005). A system for mass culture of upside-down jellyfish Cassiopea spp as a potential food item for medusivores in captivity. Int. Zoo Yearbook 39, 62–69. doi: 10.1111/j.1748-1090.2005.tb00005.x
Pitt K. A., Welsh D. T., Condon R. H. (2009) Influence of jellyfish blooms on carbon nitrogen and phosphorus cycling and plankton productionHydrobiolog 616, 133–149 doi: 10.1007/s10750-008-9584-9
Pörtner H. O. (2002). Climate variations and the physiological basis of temperature dependent biogeography: systemic to molecular hierarchy of thermal tolerance in animals. Comp. Biochem. Physiol. Part A.: Mol. Integr. Physiol. 132 (4), 739–761. doi: 10.1016/S1095-6433(02)00045-4
Prasanth M. I., Santoshram G. S., Bhaskar J. P., Balamurugan K. (2016). Ultraviolet-a triggers photoaging in model nematode Caenorhabditis elegans in a DAF-16 dependent pathway. Age. (Dordr). 38 (1), 27. doi: 10.1007/s11357-016-9889-y
Purcell J. (2005). Climate effects on formation of jellyfish and ctenophore blooms: a review. J. Mar. Biol. Assoc. United. Kingdom. 85 (3), 461–476. doi: 10.1017/S0025315405011409
Purcell J., Uye S., Lo W. (2007). Anthropogenic causes of jellyfish blooms and their direct consequences for humans: a review. Mar. Ecol. Prog. Ser. 350, 153–174. doi: 10.3354/meps07093
Rastogi R., Richa, Kumar A., Tyagi M., Sinha R. (2010). Molecular mechanisms of ultraviolet radiation-induced DNA damage and repair. J. Nucleic Acids. 2010, 1–32. doi: 10.4061/2010/592980
Rijstenbil J. (2001). Effects of periodic low UVA radiation on cell characteristics and oxidative stress in the marine planktonic diatom ditylum brightwellii. Eur. J. Phycology. 36 (1), 1–8. doi: 10.1080/09670260110001735138
Rossbach S., Overmans S., Kaidarova A., Kosel J., Agustí S., Duarte C. M. (2020). Giant clams in shallow reefs: UV-resistance mechanisms of Tridacninae in the Red Sea. Coral Reefs. 39 (5), 1345–1360. doi: 10.1007/s00338-020-01968-w.
Roy S. (2000). “Strategies for the minimization of UV-induced damage,” in The effects of UV radiation in the marine environment, vol. 10 . Eds. De Mora S., Demers S., Vernet M. (Cambridge: Cambridge University Press), 177–205.
Sancar A. (1996). No "End of history" for photolyases. Science 272 (5258), 48–49. doi: 10.1126/science.272.5258.48
Sinha R., Häder D. (2002). UV-Induced DNA damage and repair: a review. Photochem. Photobiolog. Sci. 1 (4), 225–236. doi: 10.1039/b201230h
Thé J., Gamero-Mora E., Chagas da Silva M. V., Morandini A. C., Rossi S., d Soares M. O. (2021). Non-indigenous upside-down jellyfish cassiopea andromeda in shrimp farms (Brazil). Aquaculture. 532, 735999. doi: 10.1016/j.aquaculture.2020.735999
Thoma F. (1999). Light and dark in chromatin repair: repair of UV-induced DNA lesions by photolyase and nucleotide excision repair. EMBO J. 18 (23), 6585–6598. doi: 10.1093/emboj/18.23.6585
Tinta T., Kogovšek T., Turk V., Shiganova T. A., Mikaelyan A. S., Malej A. (2016). Microbial transformation of jellyfish organic matter affects the nitrogen cycle in the marine water column-a black Sea case study. J. Exp. Mar. Biol. Ecol. 475, 19–30. doi: 10.1016/j.jembe.2015.10.018
Todd B. D., Thornhill D. J., Fitt W. K. (2006). Patterns of inorganic phosphate uptake in Cassiopea xamachana: a bioindicator species. Mar. pollut. Bull. 52, 515–552. doi: 10.1016/j.marpolbul.2005.09.044
Torres-Pérez J., Armstrong R. (2012). Effects of UV radiation on the growth photosynthetic and photoprotective components and reproduction of the Caribbean shallow-water coral porites furcata. Coral. Reefs. 31, 1077–1091. doi: 10.1007/s00338-012-0927-7
Van Handel E. (1965). Microseparation of glycogen sugars and lipids. Analytical. Biochem. 11 (2), 266–271. doi: 10.1016/0003-2697(65)90014-X
Verde E. A., McCloskey L. R. (1998). Production respiration and photophysiology of the mangrove jellyfish cassiopea xamachana symbiotic with zooxanthellae: effect of jellyfish size and season. Mar. Ecol. Prog. Ser. 168, 147–162. doi: 10.3354/meps168147
Winters G., Loya Y., Röttgers R., Beer S. (2003) Photoinhibition in shallow-water colonies of the coral stylophora pistillata as measured in situ. Limnol. Oceanogr. 48 (4), 1388–1393. doi: 10.4319/lo.2003.48.4.1388
Zarnoch C. B., Hossain N., Fusco E., Alldred M., Hoellein T. J., Perdikaris S. (2020). Size and density of upside-down jellyfish cassiopea sp. and their impact on benthic fluxes in a Caribbean lagoon. Mar. Environ. Res. 154, 104845. doi: 10.1016/j.marenvres.2019.104845
Keywords: jellyfish, Cassiopea sp., mitochondrial respiration, pyruvate kinase, lactate dehydrogenase, energy allocation, medusae, anaerobic respiration
Citation: Aljbour SM, Alves RN and Agustí S (2023) Aerobic respiration, biochemical composition, and glycolytic responses to ultraviolet radiation in jellyfish Cassiopea sp. Front. Mar. Sci. 9:1031977. doi: 10.3389/fmars.2022.1031977
Received: 30 August 2022; Accepted: 14 December 2022;
Published: 10 January 2023.
Edited by:
Zulin Zhang, The James Hutton Institute, United KingdomReviewed by:
Tania Islas-Flores, National Autonomous University of Mexico, MexicoWilliam Fitt, University of Georgia, United States
Copyright © 2023 Aljbour, Alves and Agustí. This is an open-access article distributed under the terms of the Creative Commons Attribution License (CC BY). The use, distribution or reproduction in other forums is permitted, provided the original author(s) and the copyright owner(s) are credited and that the original publication in this journal is cited, in accordance with accepted academic practice. No use, distribution or reproduction is permitted which does not comply with these terms.
*Correspondence: Samir M. Aljbour, U2FtaXIuYWxqYm91ckBrYXVzdC5lZHUuc2E=