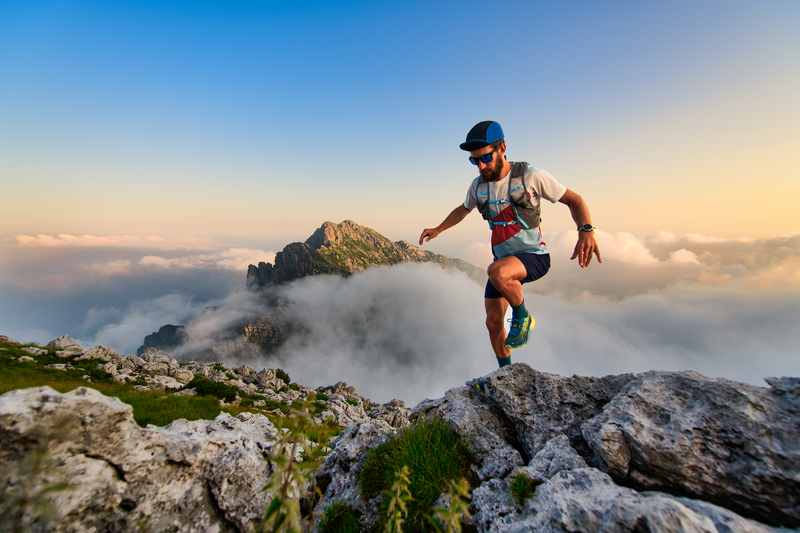
95% of researchers rate our articles as excellent or good
Learn more about the work of our research integrity team to safeguard the quality of each article we publish.
Find out more
ORIGINAL RESEARCH article
Front. Mar. Sci. , 20 October 2022
Sec. Marine Fisheries, Aquaculture and Living Resources
Volume 9 - 2022 | https://doi.org/10.3389/fmars.2022.1029483
This article is part of the Research Topic Aquaculture Environment Regulation and System Engineering View all 18 articles
A three-month culture experiment was designed to assess the effects of photoperiod and feeding regime on growth, feed utilization, and food anticipatory activity (FAA) of juvenile rainbow trout. The experiment included two photoperiods: 24L:0D (LL) and 12L:12D (LD); three feeding regimes: random feeding (R), mid-dark stage feeding (D), and mid-light stage feeding (L). A total of six treatment groups (R-LL, D-LL, L-LL, R-LD, D-LD, L-LD) were defined. The experimental results showed that the growth and feed utilization of the scheduled feeding groups (D and L groups) were significantly higher than those of the R group under both photoperiods, and there was no statistical difference between the D and L groups. A typical FAA was observed in the L group, independent of the photoperiod. Also, the digestive enzyme activity of the L group was synchronized by the feeding time under both photoperiods. There were rhythms in serum levels of glucose (GLU), triglyceride (TG), and total-cholesterol (T-CHO) in the D and L groups. Serum GLU also had a rhythm in the R group, but the peaks occurred at the feeding point (LL group) or after the feeding point (LD group), reflecting a possible passive rise in GLU after feeding. Serum cortisol was higher in the R group than in the scheduled feeding group, indicating that random feeding caused stress to juvenile rainbow trout. Serum insulin levels were found to increase before feeding in all three feeding regimes, probably reflecting the anticipation of food induced by the last meal. Serum melatonin levels were suppressed by the LL group. Serum 5-HT levels were synchronized by meal time in the R and L groups. Finally, rhythms of appetite-related genes were observed under all three feeding regimes, and more genes were rhythmic under LL, suggesting that food can strongly synchronize the feeding rhythm of juvenile rainbow trout when lacking light zeitgeber. In summary, this study concluded that diurnal feeding (L group) independent of photoperiod induced typical FAA in juvenile rainbow trout and that the LD (12L:12D) photoperiod and L (mid-light phase) feeding were recommended in the juvenile rainbow trout aquaculture.
Light (main characteristics include: spectrum, light intensity, and photoperiod) is an important abiotic factor that plays a vital role in the entire life cycle of fish, from embryonic development to gonadal maturation (Villamizar et al., 2011; Imsland et al., 2020). Photoperiod is the most primary zeitgeber of the fish biological clock system, affecting its growth (Wei et al., 2019; Singh and Zutshi, 2020), reproduction (Foss et al., 2020; Imsland et al., 2020), locomotion (Almazán-Rueda et al., 2004), metabolism (Paredes et al., 2014; Wei et al., 2019), and immunity (Leonardi and Klempau, 2003; Esteban et al., 2006). Aquaculture has widely used photoperiod manipulation to improve productivity (Imsland et al., 2020; Ruchin, 2021). It has been observed in most intensively farmed fish that extended light periods promote somatic growth (Boeuf and Le Bail, 1999; Aride et al., 2021; Li et al., 2022), while extended darkness periods promote gonadal development and maturation (Hermelink et al., 2017; Lundova et al., 2019). Constant light (LL) has been implemented in commercial production in freshwater recirculation aquaculture systems (RAS) of Atlantic salmon smolts (Lorgen-Ritchie et al., 2022; Ytrestøyl et al., 2022). Several studies have shown that LL does not negatively affect behavior (Hamilton et al., 2022), survival (Nemova et al., 2020), flesh quality (Imsland et al., 2019), and immune gene expression (Ytrestøyl et al., 2022) in salmonids. However, LL exposure is considered a stressor in some fish, such as black sea turbot (Psetta maeotica) (Turker, 2005), Atlantic cod (Gadus morhua) (Giannetto et al., 2014), and largemouth bass (Micropterus salmoides) (Malinovskyi et al., 2022), etc. More importantly, light, as the most important zeitgeber, plays a crucial role in the regulation of biological circadian rhythms, and the absence of photoperiod can cause metabolic disorders and further develop into metabolic diseases (Bass, 2012; Coomans et al., 2015; Kopp et al., 2016; Hillyer et al., 2021).
Like photoperiod, feeding is another crucial zeitgeber, providing the organism with a temporal cue to its environment (Stephan, 2002; López-Olmeda, 2017). Moreover, feeding appears to be preferential to photoperiod in regulating biological clock oscillations in peripheral tissues such as the liver and intestine (Stephan, 2002; Heyde and Oster, 2019). Feeding has been shown to reset the peripheral biological clock and affect metabolic processes (Bass, 2012). For fish, it is difficult to obtain food continuously in the natural environment, thus exhibiting different feeding rhythms such as diurnal and nocturnal predators (López-Olmeda and Sánchez-Vázquez, 2010; Kousoulaki et al., 2015). Further, feeding time preference was influenced by the season in some fishes, e.g., European sea bass (Dicentrarchus labrax L.) showed diurnal feeding in spring, summer, and autumn nocturnal feeding in winter (Sánchez-Vázquez et al., 1998). Matching feeding times to feeding rhythms can significantly improve growth and feed utilization in many fish species (López-Olmeda and Sánchez-Vázquez, 2010), such as channel catfish (Ictalurus punctatus) (Noeske-Hallin et al., 1985), rainbow trout (Oncorhynchus mykiss) (Boujard et al., 1995), European sea bass (Azzaydi et al., 2000), African catfish (Clarias gariepinus) (Hossain et al., 2001), and Senegalese sole (Solea senegalensis) (Marinho et al., 2014). Conversely, misaligned feeding (e.g., feeding during the resting phase) can lead to the decoupling of the central and peripheral biological clock oscillations of the organism, resulting in metabolic disturbances (Opperhuizen et al., 2016; Oishi and Hashimoto, 2018; Challet, 2019).
The positive effects of scheduled feeding were mainly based on food anticipatory activity (FAA), a behavior present in almost all fish (López-Olmeda, 2017), manifested by an increase in locomotion from a few minutes or hours before mealtime, as well as an increase in feeding-related endocrine and digestive and metabolic enzyme activity (Stephan, 2002; Vera et al., 2007). In addition, FAA could persist for several days when deprived of the food zeitgeber, suggesting the presence of endogenous oscillators guiding the FAA, known as food entrainment oscillators (FEOs, Davidson, 2006; López-Olmeda and Sánchez-Vázquez, 2010; Patton and Mistlberger, 2013). Despite numerous studies in model animals such as mice, the exact organization or localization of FEOs remains unknown, and many findings support that FEOs can work independently of the traditional biological clock system (feedback loops) (Davidson, 2006; Page et al., 2020). In fish, the understanding and working pathways of FEOs are still very vague and fragmented, and some hormones are thought to play an important role, such as ghrelin (Nisembaum et al., 2014a) and orexin (Hoskins and Volkoff, 2012; Nisembaum et al., 2014b).
The effects of light and food on fish circadian rhythms have been studied in several species. In zebrafish (Danio rerio) (Paredes et al., 2015) and gilthead sea bream (Sparus aurata) (Paredes et al., 2014), the rhythm of lipid metabolism genes in the liver was found to be pulled by the photoperiod independent of feeding time. In contrast, in rainbow trout (Hernández-Pérez et al., 2015), food was found to affect the rhythm of hepatic glucose and lipid metabolism gene expression more than light. Vera et al. (2013) also observed liver biological clock gene expression rhythms in the gilthead sea bream entrained by food independent of light. In addition, the expression of different biological clock genes in rainbow trout liver (Hernández-Pérez et al., 2017) and goldfish (Carassius auratus) gut (Nisembaum et al., 2012) was pulled by light and food, respectively, suggesting an interaction between these two zeitgebers. In zebrafish, neither regular feeding (diurnal feeding) nor altered feeding (nocturnal feeding) affected the expression rhythm of gut biological clock genes, but altered feeding shifted the expression rhythm of gut melatonin synthase genes, indicating that gut biological clock genes are entrained by photoperiod and melatonin synthesis is synchronized by feeding time (Mondal et al., 2022). On the other hand, food and light also impacted the locomotor activity and FAA of the fish. In zebrafish (Blanco-Vives and Sánchez-Vázquez, 2009), feeding was found to be synchronized with locomotor activity and independent of photoperiod. It was also observed in goldfish that scheduled feeding under LL synchronized the rhythm of biological clock genes in the optic tectum and hypothalamus and elicited FAA, while rhythmic expression of biological clock genes in the liver was also observed in the random feeding group, suggesting that the biological clock in the liver is synchronized by the last meal time, and FAA independent of the liver biological clock system (Feliciano et al., 2011). However, in tambaqui (Colossoma macropomum), mid-dark phase feeding caused FAA, while mid-light phase feeding was futile (Fortes-Silva et al., 2018). Notably, as a strictly nocturnal species, tench (Tinca tinca) elicited FAA in the light phase feeding under a constant dark but not LD (12L:12D) environment (Herrero et al., 2005). Whether scheduled feeding can induce FAA in fish seems closely related to feeding time and photoperiod.
Rainbow trout is a high-value economically farmed fish widely farmed worldwide (especially in European countries) (Öz, 2018). Global aquaculture production reached 848,051 tons, with a value of $3.88 billion in 2018 (FAO, 2020). Rainbow trout is famous among consumers as a high-grade aquatic product in China due to its bright color, delicious taste, and rich nutrition. However, there is still a vast gap between production and market demand due to the lack of cold-freshwater resources. Previous studies found that rainbow trout farming in seawater can achieve faster growth rates (Landless, 1976; Shepherd et al., 2005), higher feed conversion efficiencies (Landless, 1976), and stronger immunity (Yada et al., 2001). Based on this discovery, in recent years, some researchers have attempted to aquaculture rainbow trout using offshore fish cages (such as “Shenlan 1”) in the cold-water masses of the Yellow Sea in China (Dong, 2019; Chu et al., 2020). Nowadays, rainbow trout has emerged as a promising candidate fish for deep-sea aquaculture (Dong, 2019; Huang et al., 2021).
Currently, the “mountain-sea transfer” culturing method has been used to culture rainbow trout in seawater (Dong, 2019). In brief, rainbow trout larvae are hatched in low-temperature freshwater nurseries. The fry (about 5 g) is transferred to mountainous areas with abundant cold-freshwater resources for large-size juvenile culture. After growing to approximately 150 g, the juveniles are transported to seawater domestication systems for one week and finally transferred to the offshore fish cage for culture to commercial fish (weight above 4 kg). During this process, cultivating fish from fry to juveniles is a crucial step, which directly affects the later mariculture success rate. Whether light and food can be manipulated to promote rainbow trout growth during the freshwater culture is vital to the success of the “mountain-sea transfer” culturing method. Therefore, the objectives of this study were 1) to investigate whether the photoperiod/feeding regimes can induce FAA and promote growth in juvenile rainbow trout and 2) the effects of different photoperiod/feeding regimes on behavioral, digestive, metabolic, endocrine, and appetite rhythms in juvenile rainbow trout.
This study was approved by the Animal Care and Use Committee of Ningbo University.
This research was conducted in the Intelligent Aquaculture Laboratory, School of Marine Sciences, Ningbo University. The juvenile rainbow trout were obtained from a commercial nursery (Shandong, China) and cultured in a recirculating aquaculture system (RAS) (HISHING, Qingdao, China) from July to November 2020. All fish were acclimatized in the RAS for one month before the culture experiment. During the acclimation period, the fish were randomly fed a commercial diet (Tech-Bank, Ningbo, China, Supplementary Table 1) at about 2% of body weight, with a photoperiod of 12L:12D (lights-on at 6:00, the light intensity on the water surface is 100-200 lx), water temperature controlled at 15.5-17.5°C, dissolved oxygen was kept saturated, and ammonia nitrogen < 0.05mg/L.
A total of 840 healthy rainbow trout were weighed and randomly distributed to 42 tanks (1.0m× H 0.8m, Supplementary Figure 1), with 20 fish per tank. The experiment included two photoperiods: 24L:0D (LL, the light intensity on the water surface is 100-200 lx) and 12L:12D (LD, lights-on at 6:00, light-off at 18:00, the light intensity on the water surface is 100-200 lx); three feeding regimes: random feeding (R), mid-dark stage feeding (D), and mid-light stage feeding (L). Briefly, a random feeding schedule for the R group was obtained using a random number generator software according to the method described by Nisembaum et al. (2012); In group D, we fed at 24:00 daily; in group L, we fed at 12:00 daily. A total of six experimental treatments were combined: R-LL, D-LL, L-LL, R-LD, D-LD, and L-LD, and each treatment included seven tanks. The growth experiment lasted three months, and the cultural management and environmental conditions were consistent with the acclimation.
Figure 1 Growth performance, feed utilization, and locomotion of juvenile Oncorhynchus mykiss under different experimental treatment. (A). IBW, Initial body weight, (B). FBW, Final body weight, (C). WG, Weight gain (%) = 100 × (final weight − initial weight)/initial weight, (D). SGR, Specific growth rate (%/day) = 100 × (ln final weight − ln initial weight)/94 days, (E). FCR, Feed conversion ratio = dry feed consumed/wet weight gain, (F). EF, Exercise frequency. Different lowercase letters and capital letters indicate significant difference among different feeding strategy at the LL (constant light) and LD (12L: 12D, lights-on at 6:00), respectively (P < 0.05). Asterisks denote significant differences between photoperiods at the same feeding regime (*, P < 0.05; **, P < 0.01).
In this experiment, an underwater infrared camera (SLD-EX300Q, Hengdun Shunli Da electronic technology Co., Ltd., Jinan, China) was used to observe the exercise frequency (EF) of juvenile rainbow trout. The number of times the fish swam through the observation area was recorded as EF. Fish locomotion data were collected from October 2nd to 4th, 2020 (2 months after the growth experiment was conducted) for 72 hours. As Mistlberger (1994) described, a typical FAA is usually defined as a rapid increase in activity from minutes to hours before the feeding point, reaching more than twice the basal activity and lasting at least 30 minutes.
Following the 3-month growth experiment, all fish were starved for 24 hours, while samples were taken afterward. The 24th hour after food deprivation was ZT0 (feeding point), and samples were taken every 4 hours for 24 hours, for a total of 7 sampling points (ZT0, ZT4, ZT8, ZT12, ZT16, ZT20, ZT24), corresponding to 7 tanks in each treatment, to avoid the stimulation of fish by sampling. Each sampling point was operated as follows: first, the fish in the tank were anesthetized using MS-222 (50mg/L, Shanghai Macklin Biochemical Co., Ltd., Shanghai, China), and then 12 fish were randomly taken from the tank for weight and body length measurement (to calculate the condition factor (CF)); the blood was immediately collected from each fish by the tail vein method and stored overnight at 4°C; then, the fish were dissected immediately after blood collection, the brain, liver (weighing to calculate the hepatosomatic index (HSI)), and digestive tract (scraping off intestinal fat to calculate the intraperitoneal fat ratio (IPF)) were isolated, quick frozen in liquid nitrogen and stored at -80°C.
First, the digestive tract was homogenized in saline (0.9% NaCl, 4°C) in a ratio of 1:9 (W: V). The homogenate was centrifuged at 3000 rpm for 10 min at 4°C to obtain the supernatant. Amylase (C016-1-1), lipase (A054-2-1), pepsin (A080-1-1), and chymotrypsin (A080-3-1) activity in the digestive tract were assayed using commercial kits (Nanjing Jiancheng Bioengineering Institute, Nanjing, China) according to the instructions.
Fish blood was left overnight at 4°C and then centrifuged at 4°C for 10 minutes at 2500 rpm, and the supernatant was taken as serum. Serum glucose (Glu, A154-2-1), triglyceride (TG, A110-1-1), and total-cholesterol (T-CHO, A111-1-1) were measured by commercial kits (Nanjing Jiancheng Bioengineering Institute, Nanjing, China) according to the instructions. Serum cortisol, insulin, melatonin, and serotonin (5-HT) levels were measured using commercial enzyme linked immunosorbent assay (ELISA) kits (Shanghai Qiaodu Biotechnology Co., Ltd., Shanghai, China) according to the instructions.
The total RNA of the brain and digestive tract was extracted with a commercial kit RNA isolator (R401-01, Vazyme, Nanjing, China). The quality of total RNA was checked by ultramicro-spectrophotometer (Nanodrop 2000, Thermo Scientific, Waltham, USA) and 1% gel electrophoresis. The RNA was reverse transcribed into cDNA using a HiFiScript cDNA synthesis kit (CW2569M, CWBIO, Beijing, China).
The real-time PCR was used to analyze the relative expression of appetite-related genes in the brain (agouti-related peptide (agrp), cocaine- and amphetamine-related transcript (cart), neuropeptide Y (npy), orexin, pro-opio melanocortin (pomc)) and digestive tract (Ghrelin/Obestatin prepropeptide (ghrl) and leptin). The total reaction volume includes 10 μl of 2 × MagicSYBR Mixture (CW3008H, CWBIO, Beijing, China), 2 μl of cDNA, 0.4 μl of each primer (10 μM), and 7.2 μl of ddH2O. A Real-Time PCR System (QuantStudio™ 6 Flex, Life Technologies, Carlsbad, USA) was used with the program as follows: 95°C for 30 s; 45 cycles at 95°C for 5 s, 60°C for 30 s; and 95°C for 15 s. The specific primers used in this study are shown in Supplementary Table 2 and synthesized by a commercial company (Youkang Biological Technology Co., Ltd, Hangzhou, China). The relative expression level of target genes is normalized by β-actin and elongation factor-1α (ef1α) and calculated by the comparative CT method (2-ΔΔCT method) (Livak and Schmittgen, 2001).
All statistical analyses were performed on SPSS 22.0 and R 4.1.2 software. First, all data were checked for homogeneity and normal distribution through Levene’s test and Kolmogorov-Smirnov test, respectively. Then a two-way ANOVA was performed with photoperiod and feeding regime. Meanwhile, t-tests were performed for photoperiod, and one-way ANOVA followed by Duncan’s multiple range test was performed for the feeding regime to check the effect on IBW, FBW, WG, SGR, FCR, EF, HSI, IPF, and CF. P < 0.05 and P < 0.01 were considered significant differences and extremely significant differences, respectively. In addition, cosinor analysis [performed using the circacompare R package (Parsons et al., 2020)] was performed to detect the circadian rhythms in digestive enzyme activity, serum metabolite and hormone levels, and appetite-related gene expression. P < 0.05 indicates the presence of circadian rhythms.
In the present study, photoperiod did not affect the growth and feed utilization of juvenile rainbow trout (Figures 1A–E and Supplementary Table 3). Scheduled feeding (D and L groups) significantly increased the final body weight (FBW) and decreased the feed conversion ratio (FCR) of juvenile rainbow trout (P<0.05). The scheduled feeding (D and L groups) significantly increased specific growth rate (SGR) under LL (P<0.05), although there was no statistical difference, and higher weight gain (WG) and SGR were also observed with scheduled feeding (D and L groups) under LD (P>0.05). There was no interaction between photoperiod and feeding regime on growth and feed utilization of juvenile rainbow trout (Supplementary Table 3). Feeding time (D or L groups) did not affect the growth and feed utilization of juvenile rainbow trout (Figures 1A–E). Morphological parameters (HSI, IPF, and CF) were not affected by the experimental treatment (Supplementary Table 3 and Supplementary Figure 2, P>0.05).
Figure 2 Exercise frequency of juvenile Oncorhynchus mykiss under different experimental treatment. The bright bars indicate the light period and the dark bars indicate the dark period.
The EF of juvenile rainbow trout was significantly influenced by photoperiod and feeding regime (Figures 1F, 2, and Supplementary Table 3). The EF of juvenile rainbow trout in the LL group was higher than that of the LD group (P<0.05); the EF of juvenile rainbow trout in the L group was significantly higher than that of the other groups under both photoperiods (P<0.05). There was an interaction between photoperiod and feeding regime on the locomotion of juvenile rainbow trout (Supplementary Table 3). Under LL, the EF of the L group was significantly higher than that of the R and D groups; under LD, EF showed L>R>D.
Under both photoperiods, the EF of juvenile rainbow trout in the L group was significantly higher before feeding and then decreased, a typical FAA (Figure 2). However, no typical FAA was observed in both R and D groups under both photoperiods (Figure 2).
In this study, amylase activity showed a significant rhythm under LL for all three feeding regimes, while no rhythm was observed under LD (Figures 3A, 6). Under LL, the amylase activity of the L and D groups increased before feeding and peaked at ZT2 and ZT4, respectively. In comparison, the amylase activity of the R group peaked before feeding (ZT18).
Figure 3 Digestive enzyme activity of juvenile Oncorhynchus mykiss under different experimental treatment. (A). Amylase, (B). Lipase, (C). Pepsin, (D) Chymotrypsin. The cosine curve denotes the presence of rhythmic oscillations (P < 0.05).
Lipase activity showed significant rhythms in R and L groups under both photoperiods (Figures 3B, 6). In the L group, lipase activity increased before the feeding point, peaked at the feeding point (ZT0), and then decreased. However, lipase activity in the R group increased after feeding and then decreased.
There was a significant rhythm of pepsin activity in the L group under both photoperiods (Figures 3C, 6). Pepsin activity increased before feeding, peaked at the feeding point (ZT0), and then decreased.
The chymotrypsin activity was significantly rhythmic under LL for all three feeding regimes (Figures 3D, Figure 6). The L and D groups showed increased chymotrypsin activity before feeding, peaking at the feeding point (ZT0) and then decreasing. Chymotrypsin activity in the R group increased after feeding. Under LD, chymotrypsin activity of L and R groups increased before feeding, peaked after feeding (ZT7), and then decreased.
In the present study, the experimental treatments affected serum levels of metabolites and hormones (Figures 4, 6). Serum GLU levels increased before feeding and decreased after feeding in the D (peaked at ZT20 under LD) and L (peaked at ZT0 under LL, peaked at ZT23 under LD) groups. In the R group, serum GLU levels peaked at the ZT0 (LL) and ZT3 (LD), respectively (Figures 4A, 6). Serum TG levels were significantly higher in the scheduled feeding group (D and L groups) than in the R group and were found to peak after feeding (ZT4) under all three feeding regimes (Figures 4A, 6). Serum T-CHO levels in the scheduled feeding group also increased and peaked after feeding (Figures 4A, 6).
Figure 4 Serum metabolites (A) and hormones (B) of juvenile Oncorhynchus mykiss under different experimental treatment. The cosine curve denotes the presence of rhythmic oscillations (P < 0.05).
Serum cortisol levels were not affected by photoperiod, but random feeding caused a significant increase in serum cortisol levels (Figure 4B). Serum insulin levels were significantly higher in the R and D groups than in the L group, and peaked before feeding (ZT20-ZT23) under all three feeding regimes (Figures 4B, 6). Serum melatonin levels were significantly lower in the LL group than in the LD group. There was no circadian rhythm observed in serum melatonin levels under LD. Serum melatonin levels showed R>D>L under both photoperiods (Figure 4B). Serum serotonin levels were significantly higher in the LL group than in the LD group. Serum serotonin levels peaked before feeding (ZT23-ZT0) in both the R and L groups and then decreased (Figures 4B, 6).
Overall, more appetite-related genes showed circadian rhythms under LL compared to LD. And all appetite-related genes showed significant circadian rhythms under different feeding regimes (Figure 5).
Figure 5 Expression of feeding-related genes of juvenile Oncorhynchus mykiss under different experimental treatment. Asterisks denote the presence of diurnal oscillations (*, P < 0.05; **, P < 0.01). The cosine curve denotes the presence of rhythmic oscillations (P < 0.05).
Under LL, all appetite-related genes showed circadian rhythms in the R group, and the peaks were mostly concentrated around ZT15-ZT18 (except leptin and npy); in the L group, all genes except orexin showed significant circadian rhythms with peaks around ZT10-ZT13; in the D group, only leptin and orexin genes showed circadian rhythms with peaks around ZT11 and ZT14, respectively (Figures 5, 6).
Figure 6 The peak time of the indicator with diurnal oscillation in this study. The distance from the center of the clock indicates significance, with closer to the edge of the clock indicating stronger rhythmic oscillations (smaller P values).
Under LD, significant circadian rhythms were observed only in the cart, ghrl, leptin, and npy genes. In the R group, circadian rhythms were observed in ghrl (peak at ZT23) and leptin (peak at ZT5) genes; in the L group, circadian rhythms were observed in cart (peak at ZT0), ghrl (peak at ZT14), and leptin (peak at ZT13) genes; in the D group, circadian rhythms were observed in cart (peak at ZT22), ghrl (peak at ZT15), leptin (peak at ZT1), and npy (peak at ZT3) genes (Figures 5, 6).
Rainbow trout mortality was not observed in this study, indicating that our aquaculture environment was suitable for the survival of juvenile rainbow trout. In the present study, photoperiod did not affect growth performance (FBW, WG, SGR) and feed utilization (FCR) of juvenile rainbow trout. Similar results have been reported in other fish species, such as lenok (Brachymystax lenok) (Liu et al., 2015), pacamã catfish (Lophiosilurus alexandri) (Kitagawa et al., 2015), Amazonian ornamental (Heros severus) (Veras et al., 2016), zebrafish (Abdollahpour et al., 2020), and largemouth bass (Malinovskyi et al., 2022). However, in some fish, compared to LD, LL promoted somatic growth and feed utilization, e.g., Caspian roach (Rutilus rutilus caspicus) (Shahkar et al., 2015), gibel carp (Carassius auratus) (Wei et al., 2019), winter flounder (Pseudopleuronectes americanus) (Casey et al., 2020), Nile tilapia (Oreochromis niloticus) (Martínez-Chávez et al., 2021). LL promotes somatic growth in fish mainly attributed to the suppression of gonadal development (Doyle et al., 2021), increased feed intake (Wei et al., 2019; Martínez-Chávez et al., 2021), enhanced digestive enzyme activity (Shan et al., 2008; Ramzanzadeh et al., 2016), and upregulation of gene expression of growth-related hormones (growth hormone (GH) and insulin-like growth factor (IGF-1)) (Li et al., 2022). Conversely, some studies have found that compared to LD, LL inhibited the growth of some fish, such as anemonefish (Amphiprion melanopus) (Arvedlund et al., 2000), wild carp (Cyprinus carpio) (Ghomi et al., 2011), and European sea bass (Li et al., 2021). Li et al. (2021) found that feed intake and digestion were elevated in European sea bass under LL exposure. However, antioxidant enzyme activity was elevated and accompanied by more frequent locomotion leading to an increase in non-growth energy consumption, which ultimately inhibited fish growth (Li et al., 2021). Similarly, Valenzuela et al. (2022) found that LL exposure caused stress in rainbow trout and impacted immune system function. In this study, the growth performance and feed utilization of juvenile rainbow trout did not show statistical differences between LL and LD groups. This result may be attributed to several reasons: 1) fixed feeding rates in both groups; 2) only amylase and lipase activity was increased in the LL group; 3) more frequent activity in the LL group; 4) no significant stress was observed in the LL group. In this study, fish feeding was fixed at about 2% of body weight for the different treatments. In addition, only significantly elevated amylase and lipase activities were observed in the LL group, while pepsin and chymotrypsin activities were not affected by photoperiod. On the other hand, the EF of rainbow trout in the LL group was significantly higher than that in the LD group. Also, although the serum GLU levels were significantly higher in the LL group than in the LD group, there was no significant difference in serum cortisol levels between the photoperiods. In summary, it is hypothesized that digestion and absorption of feeds were improved in rainbow trout under LL. However, it may not have caused significant stress, the more frequent locomotion also increased energy expenditure under LL, so LL did not promote growth and feed utilization in juvenile rainbow trout. It is also noteworthy that compared to LD, LL significantly increased the WG of largemouth bass for the first 8 weeks; however, after 12 weeks, the WG difference disappeared between these two groups (Malinovskyi et al., 2022). The culture period in this study was 3 months, so further studies are needed to determine whether short-term LL exposure would promote the growth of juvenile rainbow trout.
Scheduled feeding (D and L groups) significantly improved juvenile rainbow trout’s growth and feed utilization independent of photoperiod. Scheduled feeding induced FAA within almost all fish species (López-Olmeda, 2017). Similarly, this study observed the typical FAA behavior in the L group and was independent of photoperiod. Under both photoperiods, the locomotion of rainbow trout in the L group increased rapidly before the feeding point and decreased after feeding. Surprisingly, the D group did not exhibit FAA under both photoperiods, and rainbow trout are typically diurnal fish (Iigo and Tabata, 1997; Sánchez-Vázquez and Tabata, 1998; Bolliet et al., 2001), suggesting that shift feeding does not induce FAA in the rainbow trout. Similar results were reported in tambaqui, as nocturnal fish (Fortes-Silva et al., 2016), where typical FAA was found in the mid-dark feeding group rather than the mid-light feeding group under an LD environment (Fortes-Silva et al., 2018). Fortes-Silva et al. (2018) concluded that daytime feeding generally does not alter the locomotion of nocturnal fish. Another study in tench (Tinca tinca) (strictly nocturnal species) also found that daytime feeding failed to elicit FAA under an LD environment (Herrero et al., 2005). However, daytime feeding elicited FAA in a constant dark environment (Herrero et al., 2005). Herrero et al. (2005) concluded that mealtime became the dominant zeitgeber for synchronous locomotion in the absence of photoperiod. Similarly, an earlier study found that the demand-feeding rhythm of rainbow trout under LL was synchronized by dark period feeding (Bolliet et al., 2001). Unexpectedly, in this study, under LL, the typical FAA was still not present in the D group, although there was a higher EF at the feeding point. This result may be related to the different observation methods, as Bolliet et al. (2001) directly recorded the baiting behavior of fish through electronic self-feeders connected to a computer, whereas in the present study, the locomotion of fish was recorded through a video camera. In fish, the exact location and mechanism of FEOs that cause FAA are still poorly understood (Nisembaum et al., 2014a), and the present study also cannot explain why the D group did not cause typical FAA. However, the results of this study showed that rainbow trout have strong diurnal behavior, which could not be altered by shift feeding. The nocturnal feeding also inhibited the locomotion of rainbow trout, which reduced energy consumption and may partially explain the improved growth performance of the D group.
FAA is not only an increase in locomotion before feeding but also accompanies the preparation of the digestive and endocrine systems for the upcoming meal (Stephan, 2002; Vera et al., 2007). First, in the L group, all four digestive enzymes showed significant rhythms under both photoperiods (except for amylase in the L-LD group), and in the L-LL group, the peak of all four digestive enzyme activities was concentrated around the feeding point (ZT0), while in the L-LD group, the peak of the three digestive enzymes was moved to around ZT4. This result indicated that scheduled diurnal feeding under LL could fully synchronize the rhythm of digestive enzyme activity; however, under LD, photoperiod affected the rhythm of digestive enzyme activity (Lazado et al., 2017), delaying the peak of digestive enzyme activity. In the D group, although only rhythms in amylase and chymotrypsin activities were observed under LL, the trend for both was consistent with the L group, with both rising before the feeding point and peaking at ZT4. This result suggested that scheduled nocturnal feeding can partially synchronize the rhythm of digestive enzyme activity in rainbow trout in the absence of a photoperiod.
Noteworthy, significant rhythms were also observed for amylase, lipase, and chymotrypsin in the R group. However, these peaks in enzyme activity were not clustered but rather scattered at different points in the day. In permit (Trachinotus falcatus), Lazado et al. (2017) also found a rhythm in digestive enzyme activity and diversity of peaks between different digestive enzymes. This result indicated that there is a rhythm of digestive enzyme activity in the digestive tract of rainbow trout, but random feeding could not synchronize the rhythm of digestive enzymes. In other words, rainbow trout could not anticipate the feeding point under random feeding and failed to increase the digestive enzyme activity before the feeding point to improve the digestion and utilization of feed. Similar results have been widely reported in different fish species. Regardless of the photoperiod, scheduled feeding induced a significant increase in digestive enzyme activity before the feeding point, but this phenomenon disappeared under random feeding (Vera et al., 2007; Montoya et al., 2010; Lazado et al., 2017).
Further analysis of serum metabolite levels revealed significant rhythms in serum GLU, TG, and T-CHO in the scheduled feeding groups (D and L groups). First, the L group serum GLU showed a rapid increase and peak before the feeding point and a decrease after feeding under both photoperiods, a trend consistent with locomotion. GLU is a substrate for energy metabolism (Haman et al., 1997), which showed that an elevated energy requirement accompanies FAA before the feeding point. The D group observed a similar serum GLU rhythm under LD, but the peak was advanced. In the R group, significant rhythms in GLU were also present, but the peaks occurred at the feeding point (LL group) and after feeding (LD group), respectively, which may reflect a passive rise in GLU caused by feeding rather than anticipation of food.
Wagner and Congleton (2004) suggested that serum TG and T-CHO levels in salmonids represent lipid metabolism and nutritional status, respectively. Overall, TG and T-CHO levels were significantly lower in the R group than in the scheduled feeding groups (D and L groups), reflecting a poor metabolic and nutritional status. On the other hand, under LL, a TG peak was observed around ZT4 for all three feeding strategies, which is consistent with an increase in lipid metabolism levels after feeding, and the same rhythmic trend was observed in the R group, implying that the last meal also induced a rhythm in lipid metabolism. Similarly, Feliciano et al. (2011) found in goldfish that the biological clock gene expression rhythm of the liver, a central metabolic organ, was synchronized by the last meal. Daily metabolic oscillations were also driven by the last meal in the randomly fed rat (Escobar et al., 2007). As a nutritional indicator, the rhythm of serum T-CHO was only found in the scheduled feeding group. The serum T-CHO levels peaked at ZT4 (D group) and ZT8 (L group). This result revealed the differential effect of feeding time on the rhythm of lipid metabolism in rainbow trout.
Insulin is a peptide hormone that widely exists in vertebrates and plays a fundamental role in growth, development, and metabolic regulation (Hernández-Sánchez et al., 2006). The best-known function of insulin is to regulate blood GLU levels (Caruso and Sheridan, 2011). In general, serum insulin levels rise after a meal to suppress the rise in blood glucose and then quickly return to normal levels. Carnivorous fish are considered non-insulin-dependent diabetes mellitus (Salgado et al., 2004), and insulin and blood glucose levels take longer to recover after meals (Navarro et al., 1993). In the present study, serum insulin levels were significantly higher in the R and D groups compared to the L group, reflecting the increased insulin resistance in rainbow trout. Further analysis of insulin rhythms showed that serum insulin levels increased and peaked before feeding and decreased after feeding for all three feeding regimes. The rainbow trout were starved for 24 h before sampling. They were also starved during the sampling period, so the rise in serum insulin levels in this study was not a passive rise caused by feeding. The results of this study may suggest that rainbow trout can release insulin into serum earlier to maintain blood GLU homeostasis based on the timing of the last meal.
In fish, cortisol can increase the rate of energy metabolism in response to the high energy demands of stress (Mommsen et al., 1999). In the present study, high cortisol levels were detected in the serum of the R group. Random feeding as a stressor has been found to cause increased serum cortisol levels in other teleosts, such as gilthead seabream (Sánchez et al., 2009) and goldfish (Saiz et al., 2021). A recent study found that long-term treatment with high cortisol resulted in atrophy of the digestive tissues of rainbow trout, hindering nutrient assimilation and energy absorption while increasing routine energy expenditure, ultimately impeding growth (Pfalzgraff et al., 2021). Therefore, the significantly poor growth performance and feed utilization of the R group in this study were strongly associated with high cortisol levels. On the other hand, LL appears to be an acceptable light environment for rainbow trout and does not cause serum cortisol rises. Similar results have been reported in juvenile red sea bream (Biswas et al., 2006). Consistent with the results of this study, Saiz et al. (2021) found that random feeding resulted in higher cortisol levels compared to the absence of photoperiod in goldfish, suggesting that food is a more critical zeitgeber for fish welfare.
Melatonin is an important mediator for organisms to receive environmental time cues (Mondal et al., 2022). In fish, the pineal gland receives external light signals and converts time cues into biological signals via melatonin, which regulates endogenous biological clock rhythms (Yasmin et al., 2021). In the present study, melatonin levels were significantly lower in the LL group than in the LD group. The inhibition of melatonin synthesis and secretion by light has been widely reported in fish (Masuda et al., 2003; Ziv et al., 2007). The feeding regime also affected serum melatonin levels. In the present study, serum melatonin levels were found to show R>D>L. Studies in recent years have found that many peripheral tissues are also critical sources of serum melatonin, such as the intestine (Mukherjee and Maitra, 2015). Moreover, melatonin production was found to be regulated by feeding independent of photoperiod in the intestine of rainbow trout (Lepage et al., 2005), carp (Catla catla) (Mukherjee et al., 2014a; Mukherjee et al., 2014b), and zebrafish (Mondal et al., 2022). Surprisingly, no circadian rhythm of serum melatonin levels was observed under LD in the present study, and it is speculated that serum melatonin comes from different tissues, including the pineal gland and intestine, regulated by light and food, respectively, so that these two zeitgebers may mask the rhythm of serum melatonin. In addition, no serum melatonin rhythms were also observed in the R-LL group, and similar results have been reported in Atlantic salmon (Salmo salar), where Huang et al. (2010) suggested that LL treatment may mask serum melatonin fluctuations.
Serotonin, also known as 5-hydroxytryptamine (5-HT), is an essential neurotransmitter and a precursor of melatonin distributed in the nervous system and many other peripheral tissues (Lillesaar, 2011). Many physiological functions and behaviors of animals are affected by 5-HT, including locomotion, sleep, appetite, and reproduction (Lucki, 1998). In zebrafish, 5-HT levels in the brainstem and spinal cord correlate with locomotion, and 5-HT increased swimming in early juvenile stages (Brustein et al., 2003). Similarly, the present study observed higher locomotion and serum 5-HT levels under LL. In vertebrates, tryptophan is synthesized into serotonin by tryptophan hydroxylase-1 (Tph1) and decarboxylation (Borjigin et al., 1999; Mondal et al., 2022). In zebrafish, tph1 gene expression was guided by the feeding time, independent of photoperiod, with the highest expression at the point of feeding (Mondal et al., 2022). An increase in intestinal 5-HT levels after feeding was also found in Atlantic salmon (Mardones et al., 2022). 5-HT promotes intestinal peristalsis and secretion and thus regulates the digestive process (Kindt and Tack, 2007; Hasler, 2009). In the present study, serum 5-HT levels in the L group increased rapidly and peaked before feeding, suggesting that rainbow trout in the L group were able to prepare for the upcoming meal to improve digestibility. Similar rhythms were also found in the R-LD group, suggesting that the last meal can also synchronize the rhythm of serum 5-HT. Surprisingly, no rhythm of serum 5-HT was observed in the D group, implying that scheduled nocturnal feeding could not synchronize the serum 5-HT rhythm of rainbow trout.
The circadian system plays an important role in the temporal regulation of metabolic processes and food intake to ensure energy efficiency (Page et al., 2020). Many areas of the brain are involved in the regulation of food intake, with the hypothalamus being the central area (Page et al., 2020). The arcuate nucleus (ARC) in the hypothalamus contains two distinct opposing populations of nuclei (Delgado et al., 2017; Page et al., 2020): neuropeptide Y (NPY) and agouti-related peptide (AgRP) (Broberger et al., 1998) neurones are orexigenic with their activation increasing food intake, while the pro-opio melanocortin (POMC) (Joseph et al., 1985) and cocaine- and amphetamine-related transcript (CART) (Kristensen et al., 1998) neurones are considered anorexigenic with their activation reducing food intake. NPY/AgRP can be stimulated by ghrelin (ghrl) and inhibited by leptin (Hahn et al., 1998). In contrast, POMC/CART can be stimulated by leptin and inhibited by ghrl (Broberger, 2005). Therefore, we further analyzed the effects of light and food on appetite-related gene expression rhythms.
The rhythmic expression of ghrl and leptin genes was observed in the L group under both photoperiods. The ghrl gene expression peaked about 2 h later than leptin, and LD delayed the peak expression of both genes by about 2 h, suggesting that photoperiod affected the rhythm of appetite-related hormones. Further, in the L-LL group, the expression of both pomc and cart genes rose after feeding and reached a trough around the next feeding point, consistent with the observation of FAA. Similarly, previous studies have also observed an increase in rainbow trout pomc gene expression after feeding (Gong and Björnsson, 2014; Naderi et al., 2018). A significant increase in cart gene expression was also observed in Siberian sturgeon (Acipenser baerii) after 3 h of feeding (Zhang et al., 2018). In the present study, significant rhythms were observed in the expression of both npy and agrp genes in L-LL, and the trends were consistent with pomc and cart genes. Further, npy gene expression in the L-LL group peaked at ZT14 (10 h before feeding) and reached a trough at ZT2 (2 h after feeding). Similarly, a previous study on rainbow trout found that the npy gene peaked at ZT16 (8 h before feeding) and reached a trough at ZT4 (4 h after feeding) (Naderi et al., 2018). However, the cart gene expression rhythm showed an opposite trend under L-LD, peaking at the feeding point (ZT0). The same results were reported in rainbow trout under LD photoperiod (Naderi et al., 2018), and Mercer et al. (2003) also found that this gene expression was regulated by photoperiod.
Although no typical FAA was observed in the D group under both photoperiods in this study, some appetite-related genes showed significant rhythms. Under LL, leptin and orexin gene expression was observed to be rhythmic. The expression trend of the leptin gene was similar to that of the L group, which may indicate that leptin gene expression was synchronized by scheduled feeding when lacking a photoperiod. Interestingly, in the D group, the rhythms of leptin gene expression were reversed between the LD and LL photoperiods, reflecting the complex effect of shifting feeding on fish feeding rhythms, and further studies are needed to explain this phenomenon. Significant cart, ghrl, and npy gene expression rhythms were also observed under LD. The gene expression trend of the ghrl gene was similar to that of the L group, while the peak of cart and npy gene expression was before and after feeding, respectively. As mentioned above, CART suppresses appetite, and NPY enhances appetite in fish (Delgado et al., 2017), and the disruption of the peak expression of both genes may suggest that shifting feeding disrupts the normal feeding rhythm of rainbow trout.
In addition, rhythms in the expression of some appetite-related genes were also observed in the R group. All genes measured under LL exhibited significant rhythms, indicating that feeding rhythms in rainbow trout can be elicited by just one meal when lacking light zeitgeber. Similarly, last meal induced metabolic rhythms has been reported in other species (Escobar et al., 2007; Feliciano et al., 2011).
Orexin is also a feeding regulatory neuropeptide secreted by the hypothalamus (Matsuda et al., 2012) but is not involved in the integrative core described above (Delgado et al., 2017). Some studies in mice have speculated that orexin could be involved in regulating FAA (Page et al., 2020). However, different results have been reported in some studies, Kaur et al. (2008) and Clark et al. (2009) observed a decrease in FAA in orexin-deficient mice; in contrast, Gunapala et al. (2011) found no change in FAA in orexin knockout mice. In the present study, the rhythm of orexin gene expression was observed only in R-LL and D-LL groups. Therefore, the results of this study suggest that the expression of the orexin gene in rainbow trout does not seem to be directly associated with FAA.
In this study, growth and feed utilization of juvenile rainbow trout were enhanced by scheduled feeding (D and L groups) and were independent of photoperiod. Typically FAA was observed in the L group, where locomotion rose before the feeding point, accompanied by rhythmic synchronization of digestive enzyme activity, serum metabolites and hormones, and appetite-related gene expression. Although typical FAA behavior was not observed in the D group, an increase in digestive enzyme activity and serum GLU levels before feeding was detected, suggesting the presence of food anticipation. Notably, although FAA was not present in the R group, rhythms were observed in digestive enzyme activity, serum metabolite and hormone levels, and appetite-related gene expression. Overall, this study found that only mid-light stage feeding induced typical FAA in rainbow trout independent of photoperiod.
The raw data supporting the conclusions of this article will be made available by the authors, without undue reservation.
The animal study was reviewed and approved by Animal Care and Use Committee of Ningbo University.
Conceptualization, CS and HX; methodology, HX; formal analysis, HX; resources, CS; investigation, HX; writing—original draft preparation, HX; writing—review and editing, HX, CS, YY, CM and CW; supervision, CS and CW; project administration, CW; funding acquisition, CS All authors have read and agreed to the published version of the manuscript.
This study was supported by the National Key Research and Development Program of China (Project No. 2019YFD0901000), The Province Key Research and Development Program of Zhejiang (Project No. 2021C02047), and K.C. Wong Magna Fund from Ningbo University.
The authors also thank Professor Herve Migaud for his constructive comments on this research.
The authors declare that the research was conducted in the absence of any commercial or financial relationships that could be construed as a potential conflict of interest.
All claims expressed in this article are solely those of the authors and do not necessarily represent those of their affiliated organizations, or those of the publisher, the editors and the reviewers. Any product that may be evaluated in this article, or claim that may be made by its manufacturer, is not guaranteed or endorsed by the publisher.
The Supplementary Material for this article can be found online at: https://www.frontiersin.org/articles/10.3389/fmars.2022.1029483/full#supplementary-material
Abdollahpour H., Falahatkar B., Lawrence C. (2020). The effect of photoperiod on growth and spawning performance of zebrafish, danio rerio. Aquaculture. Rep. 17, 100295. doi: 10.1016/j.aqrep.2020.100295
Almazán-Rueda P., Schrama J. W., Verreth J. A. J. (2004). Behavioural responses under different feeding methods and light regimes of the African catfish (Clarias gariepinus) juveniles. Aquaculture 231 (1-4), 347–359. doi: 10.1016/j.aquaculture.2003.11.016
Aride P. H. R., Gomes M. F. S., Azevedo D. G., Sangali G. R., Silva A. C. F., Lavander H. D., et al. (2021). Dusky grouper Epinephelus marginatus growth and survival when exposed to different photoperiods. Fishes 6 (3), 31. doi: 10.3390/fishes6030031
Arvedlund M., McCormick M. I., Ainsworth T. (2000). Effects of photoperiod on growth of larvae and juveniles of the anemonefish amphiprion melanopus Vol. 23 (Australia: Naga, the ICLARM Quarterly), 18–23. Available at: http://hdl.handle.net/1834/25680.
Azzaydi M., Martinez F. J., Zamora S., Sánchez-Vázquez F. J., Madrid J. A. (2000). The influence of nocturnal vs. diurnal feeding under winter conditions on growth and feed conversion of European sea bass (Dicentrarchus labrax l.). Aquaculture 182 (3-4), 329–338. doi: 10.1016/S0044-8486(99)00276-8
Bass J. (2012). Circadian topology of metabolism. Nature 491 (7424), 348–356. doi: 10.1038/nature11704
Biswas A. K., Seoka M., Tanaka Y., Takii K., Kumai H. (2006). Effect of photoperiod manipulation on the growth performance and stress response of juvenile red sea bream (Pagrus major). Aquaculture 258 (1-4), 350–356. doi: 10.1016/j.aquaculture.2006.03.048
Blanco A. M., Velasco C., Bertucci J. I., Soengas J. L., Unniappan S. (2018). Nesfatin-1 regulates feeding, glucosensing and lipid metabolism in rainbow trout. Front. Endocrinol. 9. doi: 10.3389/fendo.2018.00484
Boeuf G., Le Bail P. Y. (1999). Does light have an influence on fish growth? Aquaculture 177 (1-4), 129–152. doi: 10.1016/S0044-8486(99)00074-5
Bolliet V., Aranda A., Boujard T. (2001). Demand-feeding rhythm in rainbow trout and European catfish: Synchronisation by photoperiod and food availability. Physiol. Behav. 73 (4), 625–633. doi: 10.1016/S0031-9384(01)00505-4
Borjigin J., Li X. D., Snyder S. H. (1999). The pineal gland and melatonin: Molecular and pharmacologic regulation. Annu. Rev. Pharmacol. Toxicol. 39, 53–65. doi: 10.1146/annurev.pharmtox.39.1.53
Boujard T., Gélineau A., Corraze G. (1995). Time of a single daily meal influences growth performance in rainbow trout (Oncorhynchus mykiss). Aquaculture. Res. 26 (5), 341–349. doi: 10.1111/j.1365-2109.1995.tb00922.x
Broberger C. (2005). Brain regulation of food intake and appetite: molecules and networks. J. Internal Med. 258 (4), 301–327. doi: 10.1111/j.1365-2796.2005.01553.x
Broberger C., Johansen J., Johansson C., Schalling M., Hokfelt T. (1998). The neuropeptide y agouti gene-related protein (AGRP) brain circuitry in normal, anorectic, and monosodium glutamate-treated mice. Proc. Natl. Acad. Sci. United. States America 95 (25), 15043–15048. doi: 10.1073/pnas.95.25.15043
Brustein E., Chong M., Holmqvist B., Drapeau P. (2003). Serotonin patterns locomotor network activity in the developing zebrafish by modulating quiescent periods. J. Neurobiol. 57 (3), 303–322. doi: 10.1002/neu.10292
Caruso M. A., Sheridan M. A. (2011). New insights into the signaling system and function of insulin in fish. Gen. Comp. Endocrinol. 173 (2), 227–247. doi: 10.1016/j.ygcen.2011.06.014
Casey P., Butts I. A. E., Zadmajid V., Sorensen S. R., Litvak M. K. (2020). Prolonged photoperiod improves the growth performance for a hatchery reared right-eyed flatfish. Aquacultural. Eng. 90, 102089. doi: 10.1016/j.aquaeng.2020.102089
Challet E. (2019). The circadian regulation of food intake. Nat. Rev. Endocrinol. 15 (7), 393–405. doi: 10.1038/s41574-019-0210-x
Chu Y. I., Wang C. M., Parka J. C., Lader P. F. (2020). Review of cage and containment tank designs for offshore fish farming. Aquaculture 519, 734928. doi: 10.1016/j.aquaculture.2020.734928
Clark E. L., Baumann C. R., Cano G., Scammell T. E., Mochizuki T. (2009). Feeding-elicited cataplexy in orexin knockout mice. Neuroscience 161 (4), 970–977. doi: 10.1016/j.neuroscience.2009.04.007
Coomans C. P., Lucassen E. A., Kooijman S., Fifel K., Deboer T., Rensen P. C. N., et al. (2015). Plasticity of circadian clocks and consequences for metabolism. Diabetes Obes. Metab. 17 (1), 65–75. doi: 10.1111/dom.12513
Davidson A. J. (2006). Search for the feeding-entrainable circadian oscillator: a complex proposition. Am. J. Physiol.-Regul. Integr. Comp. Physiol. 290 (6), R1524–R1526. doi: 10.1152/ajpregu.00073.2006
Delgado M. J., Cerdá-Reverter J. M., Soengas J. L. (2017). Hypothalamic integration of metabolic, endocrine, and circadian signals in fish: involvement in the control of food intake. Front. Neurosci. 11. doi: 10.3389/fnins.2017.00354
Dong S. L. (2019). Researching progresses and prospects in large salmonidae farming in cold water mass of yellow Sea. Periodical. Ocean. Univ. China 49 (03), 1–6. doi: 10.16441/j.cnki.hdxb.20180303
Doyle A., Cowan M. E., Migaud H., Wright P. J., Davie A. (2021). Neuroendocrine regulation of reproduction in Atlantic cod (Gadus morhua): Evidence of Eya3 as an integrator of photoperiodic cues and nutritional regulation to initiate sexual maturation. Comp. Biochem. Physiol. A-Molecular. Integr. Physiol. 260, 111000. doi: 10.1016/j.cbpa.2021.111000
Escobar C., Martinez-Merlos M. T., Angeles-Castellanos M., Minana M. D., Buijs R. M. (2007). Unpredictable feeding schedules unmask a system for daily resetting of behavioural and metabolic food entrainment. Eur. J. Neurosci. 26 (10), 2804–2814. doi: 10.1111/j.1460-9568.2007.05893.x
Esteban M.Á., Cuesta A., Rodríguez A., Meseguer J. (2006). Effect of photoperiod on the fish innate immune system: a link between fish pineal gland and the immune system. J. Pineal. Res. 41, 261–266. doi: 10.1111/j.1600-079X.2006.00362.x
FAO (2020). FAO yearbook. Fishery and Aquaculture Statistics 2018 (Rome) Food and Agriculture Organization of the United Nations. doi: 10.4060/cb1213t
Feliciano A., Vivas Y., de Pedro N., Delgado M. J., Velarde E., Isorna E. (2011). Feeding time synchronizes clock gene rhythmic expression in brain and liver of goldfish (Carassius auratus). J. Biol. Rhythms. 26 (1), 24–33. doi: 10.1177/0748730410388600
Fortes-Silva R., Do Valle S. V., Lopez-Olmeda J. F. (2018). Daily rhythms of swimming activity, synchronization to different feeding times and effects on anesthesia practice in an Amazon fish species (Colossoma macropomum). Chronobiology. Int. 35 (12), 1713–1722. doi: 10.1080/07420528.2018.1509078
Fortes-Silva R., Oliveira I. E., Vieira V. P., Winkaler E. U., Guerra-Santos B., Cerqueira R. B. (2016). Daily rhythms of locomotor activity and the influence of a light and dark cycle on gut microbiota species in tambaqui (Colossoma macropomum). Biol. Rhythm. Res. 47 (2), 183–190. doi: 10.1080/09291016.2015.1094972
Foss A., Siikavuopio S. I., Imsland A. K. D. (2020). Effects of altered photoperiod regimes during winter on growth and gonadosomatic index in Arctic charr (Salvelinus alpinus) reared in freshwater. Aquaculture Res. 51 (4), 1365–1371. doi: 10.1111/are.14481
Ghomi M. R., Zarei M., Sohrabnejad M. (2011). Effect of photoperiod on growth and feed conversion of juvenile wild carp, Cyprinus carpio. Acta Biologica. Hungarica. 62 (2), 215–218. doi: 10.1556/ABiol.62.2011.2.12
Giannetto A., Fernandes J. M. O., Nagasawa K., Mauceri A., Maisano M., De Domenico E., et al. (2014). Influence of continuous light treatment on expression of stress biomarkers in Atlantic cod. Dev. Comp. Immunol. 44 (1), 30–34. doi: 10.1016/j.dci.2013.11.011
Gong N. P., Björnsson B. T. (2014). Leptin signaling in the rainbow trout central nervous system is modulated by a truncated leptin receptor isoform. Endocrinology 155 (7), 2445–2455. doi: 10.1210/en.2013-2131
Gunapala K. M., Gallardo C. M., Hsu C. T., Steele A. D. (2011). Single gene deletions of orexin, leptin, neuropeptide y, and ghrelin do not appreciably alter food anticipatory activity in mice. PloS One 6 (3), e18377. doi: 10.1371/journal.pone.0018377
Hahn T. M., Breininger J. F., Baskin D. G., Schwartz M. W. (1998). Coexpression of agrp and NPY in fasting-activated hypothalamic neurons. Nat. Neurosci. 1 (4), 271–272. doi: 10.1038/1082
Haman F., Powell M., Weber J. M. (1997). Reliability of continuous tracer infusion for measuring glucose turnover rate in rainbow trout. J. Exp. Biol. 200 (19), 2557–2563. doi: 10.1242/jeb.200.19.2557
Hamilton T. J., Szaszkiewicz J., Krook J., Richards J. G., Stiller K., Brauner C. J. (2022). Continuous light (relative to a 12:12 photoperiod) has no effect on anxiety-like behaviour, boldness, and locomotion in coho salmon (Oncorhynchus kisutch) post-smolts in recirculating aquaculture systems at a salinity of either 2.5 or 10 ppt. Comp. Biochem. Physiol. A-Molecular. Integr. Physiol. 263, 111070. doi: 10.1016/j.cbpa.2021.111070
Hasler W. L. (2009). Serotonin and the GI tract. Curr. Gastroenterol. Rep. 11, 383–391. doi: 10.1007/s11894-009-0058-7
Hermelink B., Kleiner W., Schulz C., Kloas W., Wuertz S. (2017). Photo-thermal manipulation for the reproductive management of pikeperch Sander lucioperca. Aquaculture. Int. 25, 1–20. doi: 10.1007/s10499-016-0009-x
Hernández-Pérez J., Míguez J. M., Librán-Pérez M., Otero-Rodiño C., Naderi F., Soengas J. L., et al. (2015). Daily rhythms in activity and mRNA abundance of enzymes involved in glucose and lipid metabolism in liver of rainbow trout, Oncorhynchus mykiss. influence of light and food availability. Chronobiology. Int. 32 (10), 1391–1408. doi: 10.3109/07420528.2015.1100633
Hernández-Pérez J., Míguez J. M., Naderi F., Soengas J. L., López-Patiño M. A. (2017). Influence of light and food on the circadian clock in liver of rainbow trout, Oncorhynchus mykiss. Chronobiology. Int. 34 (9), 1259–1272. doi: 10.1080/07420528.2017.1361435
Hernández-Sánchez C., Mansilla A., de la Rosa E. J., de Pablo F. (2006). Proinsulin in development: new roles for an ancient prohormone. Diabetologia 49 (6), 1142–1150. doi: 10.1007/s00125-006-0232-5
Herrero M. J., Pascual M., Madrid J. A., Sánchez-Vázquez F. J. (2005). Demand-feeding rhythms and feeding-entrainment of locomotor activity rhythms in tench (Tinca tinca). Physiol. Behav. 84 (4), 595–605. doi: 10.1016/j.physbeh.2005.02.015
Heyde I., Oster H. (2019). Differentiating external zeitgeber impact on peripheral circadian clock resetting. Sci. Rep. 9, 20114. doi: 10.1038/s41598-019-56323-z
Hillyer K. E., Beale D. J., Shima J. S. (2021). Artificial light at night interacts with predatory threat to alter reef fish metabolite profiles. Sci. Total. Environ. 769, 144482. doi: 10.1016/j.scitotenv.2020.144482
Hoskins L. J., Volkoff H. (2012). Daily patterns of mRNA expression of two core circadian regulatory proteins, Clock2 and Per1, and two appetite-regulating peptides, OX and NPY, in goldfish (Carassius auratus). Comp. Biochem. Physiol. A-Molecular. Integr. Physiol. 163 (1), 127–136. doi: 10.1016/j.cbpa.2012.05.197
Hossain M. A. R., Haylor G. S., Beveridge M. C. M. (2001). Effect of feeding time and frequency on the growth and feed utilization of African catfish Clarias gariepinus (Burchell 1822) fingerlings. Aquaculture. Res. 32, 999–1004. doi: 10.1046/j.1365-2109.2001.00635.x
Huang T. S., Ruoff P., Fjelldal P. G. (2010). Effect of continuous light on daily levels of plasma melatonin and cortisol and expression of clock genes in pineal gland, brain, and liver in Atlantic salmon postsmolts. Chronobiology. Int. 27 (9-10), 1715–1734. doi: 10.3109/07420528.2010.521272
Huang M., Yang X. G., Zhou Y. G., Ge J., Davis D. A., Dong Y. W., et al. (2021). Growth, serum biochemical parameters, salinity tolerance and antioxidant enzyme activity of rainbow trout (Oncorhynchus mykiss) in response to dietary taurine levels. Mar. Life Sci. Technol. 3 (4), 449–462. doi: 10.1007/s42995-020-00088-2
Iigo M., Tabata M. (1997). Circadian rhythms of locomotor activity in the rainbow trout Oncorhynchus mykiss. Fisheries. Sci. 63 (1), 77–80. doi: 10.2331/fishsci.63.77
Imsland A. K. D., Gunnarsson S., Thorarensen H. (2020). Impact of environmental factors on the growth and maturation of farmed Arctic charr. Rev. Aquaculture. 12 (3), 1689–1707. doi: 10.1111/raq.12404
Imsland A. K. D., Roth B., Doskeland I., Fjelldal P. G., Stefansson S. O., Handeland S., et al. (2019). Flesh quality of Atlantic salmon smolts reared at different temperatures and photoperiods. Aquaculture. Res. 50 (7), 1795–1801. doi: 10.1111/are.14058
Joseph S. A., Pilcher W. H., Knigge K. M. (1985). Anatomy of the corticotropin-releasing factor and opiomelanocortin systems of the brain. Fed. Proc. 44, 100–107.
Kaur S., Thankachan S., Begum S., Blanco-Centurion C., Sakurai T., Yanagisawa M., et al. (2008). Entrainment of temperature and activity rhythms to restricted feeding in orexin knock out mice. Brain Res. 1205, 47–54. doi: 10.1016/j.brainres.2008.02.026
Kindt S., Tack J. (2007). Mechanisms of serotonergic agents for treatment of gastrointestinal motility and functional bowel disorders. Neurogastroenterol. Motil. 19 (2), 32–39. doi: 10.1111/j.1365-2982.2007.00966.x
Kitagawa A. T., Costa L. S., Paulino R. R., Luz R. K., Rosa P. V., Guerra-Santos B., et al. (2015). Feeding behavior and the effect of photoperiod on the performance and hematological parameters of the pacamã catfish (Lophiosilurus alexandri). Appl. Anim. Behav. Sci. 171, 211–218. doi: 10.1016/j.applanim.2015.08.025
Kopp R., Billecke N., Legradi J., den Broeder M., Parekh S. H., Legler J. (2016). Bringing obesity to light: Rev-erb alpha, a central player in light-induced adipogenesis in the zebrafish? Int. J. Obes. 40 (5), 824–832. doi: 10.1038/ijo.2015.240
Kousoulaki K., Saether B. S., Albrektsen S., Noble C. (2015). Review on European sea bass (Dicentrarchus labrax, Linnaeus 1758) nutrition and feed management: a practical guide for optimizing feed formulation and farming protocols. Aquaculture. Nutr. 21, 129–151. doi: 10.1111/anu.12233
Kristensen P., Judge M. E., Thim L., Ribel U., Christjansen K. N., Wulff B. S., et al. (1998). Hypothalamic CART is a new anorectic peptide regulated by leptin. Nature 393 (6680), 72–76. doi: 10.1038/29993
Landless P. J. (1976). Acclimation of rainbow trout to sea water. Aquaculture 7 (2), 173–179. doi: 10.1016/0044-8486(76)90006-5
Lazado C. C., Pedersen P. B., Nguyen H. Q., Lund I. (2017). Rhythmicity and plasticity of digestive physiology in a euryhaline teleost fish, permit (Trachinotus falcatus). Comp. Biochem. Physiol. A-Molecular. Integr. Physiol. 212, 107–116. doi: 10.1016/j.cbpa.2017.07.016
Leonardi M. O., Klempau A. E. (2003). Artificial photoperiod influence on the immune system of juvenile rainbow trout (Oncorhynchus mykiss) in the southern hemisphere. Aquaculture 221, 581–591. doi: 10.1016/S0044-8486(03)00032-2
Lepage O., Larson E. T., Mayer I., Winberg S. (2005). Tryptophan affects both gastrointestinal melatonin production and interrenal activity in stressed and nonstressed rainbow trout. J. Pineal. Res. 38 (4), 264–271. doi: 10.1111/j.1600-079X.2004.00201.x
Lillesaar C. (2011). The serotonergic system in fish. J. Chem. Neuroanat. 41 (4), 294–308. doi: 10.1016/j.jchemneu.2011.05.009
Liu Y., Li X., Xu G. F., Bai S. Y., Zhang Y. Q., Gu W., et al. (2015). Effect of photoperiod manipulation on the growth performance of juvenile lenok, Brachymystax lenok (Pallas 1773). J. Appl. Ichthyology. 31 (1), 120–124. doi: 10.1111/jai.12632
Livak K. J., Schmittgen T. D. (2001). Analysis of relative gene expression data using real-time quantitative PCR and the 2-ΔΔCT method. Methods 25 (4), 402–408. doi: 10.1006/meth.2001.1262
Li X., Wei P. P., Liu S. T., Tian Y., Ma H., Liu Y. (2021). Photoperiods affect growth, food intake and physiological metabolism of juvenile European Sea bass (Dicentrachus labrax l.). Aquaculture. Rep. 20, 100656. doi: 10.1016/j.aqrep.2021.100656
Li Y., Zhu Q. F., Huang Y., Xu Q., Dai X. L., Ju C. X. (2022). Cloning and characterization of two types of growth hormone receptors in tomato clownfish (Amphiprion frenatus), and their expression under different light spectra and photoperiods. Aquaculture. Int. 30 (1), 483–500. doi: 10.1007/s10499-021-00814-2
López-Olmeda J. F. (2017). Nonphotic entrainment in fish. Comp. Biochem. Physiol. 203, 133–143. doi: 10.1016/j.cbpa.2016.09.006
López-Olmeda J. F., Sánchez-Vázquez F. J. (2010). “Feeding rhythms in fish: From behavioural to molecular approach,” in Biological clock in fish. Eds. Kulczykowska E., Popek W., Kapoor B. G. (Enfield, USA: Science Publishers), 155–184.
Lorgen-Ritchie M., Clarkson M., Chalmers L., Taylor J. F., Migaud H., Martin S. A. M. (2022). Temporal changes in skin and gill microbiomes of Atlantic salmon in a recirculating aquaculture system - why do they matter? Aquaculture 558, 738352. doi: 10.1016/j.aquaculture.2022.738352
Lucki I. (1998). The spectrum of behaviors influenced by serotonin. Biol. Psychiatry 44 (3), 151–162. doi: 10.1016/S0006-3223(98)00139-5
Lundova K., Matousek J., Prokesova M., Sebesta R., Policar T., Stejskal V. (2019). The effect of timing of extended photoperiod on growth and maturity of brook trout (Salvelinus fontinalis). Aquaculture. Res. 50, 1697–1704. doi: 10.1111/are.14053
Malinovskyi O., Rahimnejad S., Stejskal V., Bonko D., Stara A., Velisek J., et al. (2022). Effects of different photoperiods on growth performance and health status of largemouth bass (Micropterus salmoides) juveniles. Aquaculture 548, 737631. doi: 10.1016/j.aquaculture.2021.737631
Mardones O., Oyarzún-Salazar R., Labbé B. S., Miguez J. M., Vargas-Chacoff L., Muñoz J. L. P. (2022). Intestinal variation of serotonin, melatonin, and digestive enzymes activities along food passage time through GIT in Salmo salar fed with supplemented diets with tryptophan and melatonin. Comp. Biochem. Physiol. A-Molecular. Integr. Physiol. 266, 111159. doi: 10.1016/j.cbpa.2022.111159
Marinho G., Peres H., Carvalho A. P. (2014). Effect of feeding time on dietary protein utilization and growth of juvenile Senegalese sole (Solea senegalensis). Aquaculture. Res. 45, 828–833. doi: 10.1111/are.12024
Martínez-Chávez C. C., Navarrete-Ramírez P., Parke D. V., Migaud H. (2021). Effects of continuous light and light intensity on the growth performance and gonadal development of Nile tilapia. Rev. Bras. Zootecnia-Brazilian. J. Anim. Sci. 50, e20180275. doi: 10.37496/rbz5020180275
Masuda T., Iigo M., Mizusawa K., Naruse M., Oishi T., Aida K., et al. (2003). Variations in plasma melatonin levels of the rainbow trout (Oncorhynchus mykiss) under various light and temperature conditions. Zoological. Sci. 20 (8), 1011–1016. doi: 10.2108/zsj.20.1011
Matsuda K., Azuma M., Kang K. S. (2012). Orexin system in teleost fish. Vitamins. Hormones. 89, 341–361. doi: 10.1016/B978-0-12-394623-2.00018-4
Mercer J. G., Ellis C., Moar K. M., Logie T. J., Morgan P. J., Adam C. L. (2003). Early regulation of hypothalamic arcuate nucleus CART gene expression by short photoperiod in the Siberian hamster. Regul. Peptides. 111 (1-3), 129–136. doi: 10.1016/S0167-0115(02)00263-X
Mistlberger R. E. (1994). Circadian food-anticipatory activity: Formal models and physiological mechanisms. Neurosci. Biobehav. Rev. 18 (2), 171–195. doi: 10.1016/0149-7634(94)90023-X
Mommsen T. P., Vijayan M. M., Moon T. W. (1999). Cortisol in teleosts: dynamics, mechanisms of action, and metabolic regulation. Rev. Fish. Biol. Fisheries. 9 (3), 211–268. doi: 10.1023/A:1008924418720
Mondal G., Devi S. D., Khan Z. A., Yumnamcha T., Rajiv C., Devi H. S., et al. (2022). The influence of feeding on the daily rhythm of mRNA expression on melatonin bio-synthesizing enzyme genes and clock associated genes in the zebrafish (Danio rerio) gut. Biol. Rhythm. Res. 53 (7), 1073–1090. doi: 10.1080/09291016.2021.1905989
Montoya A., López-Olmeda J. F., Yúfera M., Sánchez-Muros M. J., Sánchez-Vázquez F. J. (2010). Feeding time synchronises daily rhythms of behaviour and digestive physiology in gilthead seabream (Sparus aurata). Aquaculture 306, 315–321. doi: 10.1016/j.aquaculture.2010.06.023
Mukherjee S., Maitra S. K. (2015). Gut melatonin in vertebrates: chronobiology and physiology. Front. Endocrinol. 6. doi: 10.3389/fendo.2015.00112
Mukherjee S., Moniruzzaman M., Maitra S. K. (2014a). Impact of artificial lighting conditions on the diurnal profiles of gut melatonin in a surface dwelling carp (Catla catla). Biol. Rhythm. Res. 45 (6), 831–848. doi: 10.1080/09291016.2014.923618
Mukherjee S., Moniruzzaman M., Maitra S. K. (2014b). Daily and seasonal profiles of gut melatonin and their temporal relationship with pineal and serum melatonin in carp Catla catla under natural photo-thermal conditions. Biol. Rhythm. Res. 45 (2), 301–315. doi: 10.1080/09291016.2013.817139
Naderi F., Hernández-Pérez J., Chivite M., Soengas J. L., Míguez J. M., López-Patiño M. A. (2018). Involvement of cortisol and sirtuin1 during the response to stress of hypothalamic circadian system and food intake-related peptides in rainbow trout, Oncorhynchus mykiss. Chronobiology. Int. 35 (8), 1122–1141. doi: 10.1080/07420528.2018.1461110
Navarro I., Carneiro M. N., Párrizas M., Maestro J. L., Planas J., Gutiérrez J. (1993). Post-feeding levels of insulin and glucagon in trout (Salmo trutta fario). Comp. Biochem. Physiol. Part A.: Physiol. 104 (2), 389–393. doi: 10.1016/0300-9629(93)90335-2
Nemova N. N., Nefedova Z. A., Pekkoeva S. N., Voronin V. P., Shulgina N. S., Churova M. V., et al. (2020). The effect of the photoperiod on the fatty acid profile and weight in hatchery-reared underyearlings and yearlings of Atlantic salmon Salmo salar l. Biomolecules 10 (6), 845. doi: 10.3390/biom10060845
Nisembaum L. G., de Pedro N., Delgado M. J., Isorna E. (2014a). Crosstalking between the "gut-brain" hormone ghrelin and the circadian system in the goldfish. effects on clock gene expression and food anticipatory activity. Gen. Comp. Endocrinol. 205, 287–295. doi: 10.1016/j.ygcen.2014.03.016
Nisembaum L. G., de Pedro N., Delgado M. J., Sanchez-Bretano A., Isorna E. (2014b). Orexin as an input of circadian system in goldfish: Effects on clock gene expression and locomotor activity rhythms. Peptides 52, 29–37. doi: 10.1016/j.peptides.2013.11.014
Nisembaum L. G., Velarde E., Tinoco A. B., Azpeleta C., de Pedro N., Alonso-Gomez A. L., et al. (2012). Light-dark cycle and feeding time differentially entrains the gut molecular clock of the goldfish (Carassius auratus). Chronobiology. Int. 29 (6), 665–673. doi: 10.3109/07420528.2012.686947
Noeske-Hallin T. A., Spieler R. E., Parker N. C., Suttle M. A. (1985). Feeding time differentially affects fattening and growth of channel catfish. J. Nutr. 115 (9), 1228–1232. doi: 10.1093/jn/115.9.1228
Oishi K., Hashimoto C. (2018). Short-term time-restricted feeding during the resting phase is sufficient to induce leptin resistance that contributes to development of obesity and metabolic disorders in mice. Chronobiology. Int. 35 (11), 1576–1594. doi: 10.1080/07420528.2018.1496927
Opperhuizen A. L., Wang D. W., Foppen E., Jansen R., Boudzovitch-Surovtseva O., de Vries J., et al. (2016). Feeding during the resting phase causes profound changes in physiology and desynchronization between liver and muscle rhythms of rats. Eur. J. Neurosci. 44 (10), 2795–2806. doi: 10.1111/ejn.13377
Öz M. (2018). Effects of garlic (Allium sativum) supplemented fish diet on sensory, chemical and microbiological properties of rainbow trout during storage at -18 °C. LWT. - Food Sci. Technol. 92, 155–160. doi: 10.1016/j.lwt.2018.02.030
Page A. J., Christie S., Symonds E., Li H. (2020). Circadian regulation of appetite and time restricted feeding. Physiol. Behav. 220, 112873. doi: 10.1016/j.physbeh.2020.112873
Paredes J. F., López-Olmeda J. F., Martínez F. J., Sánchez-Vázquez F. J. (2015). Daily rhythms of lipid metabolic gene expression in zebra fish liver: response to light/dark and feeding cycles. Chronobiology. Int. 32 (10), 1438–1448. doi: 10.3109/07420528.2015.1104327
Paredes J. F., Vera L. M., Martinez-Lopez F. J., Navarro I., Vázquez F. J. S. (2014). Circadian rhythms of gene expression of lipid metabolism in gilthead sea bream liver: synchronisation to light and feeding time. Chronobiology. Int. 31 (5), 613–626. doi: 10.3109/07420528.2014.881837
Parsons R., Parsons R., Garner N., Oster H., Rawashdeh O. (2020). CircaCompare: a method to estimate and statistically support differences in mesor, amplitude and phase, between circadian rhythms. Bioinformatics 36 (4), 1208–1212. doi: 10.1093/bioinformatics/btz730
Patton D. F., Mistlberger R. E. (2013). Circadian adaptations to meal timing: neuroendocrine mechanisms. Front. Neurosci. 7. doi: 10.3389/fnins.2013.00185
Pfalzgraff T., Lund I., Skov P. V. (2021). Cortisol affects feed utilization, digestion and performance in juvenile rainbow trout (Oncorhynchus mykiss). Aquaculture 536, 736472. doi: 10.1016/j.aquaculture.2021.736472
Ramzanzadeh F., Yeganeh S., JaniKhalili K., Babaei S. S. (2016). Effects of different photoperiods on digestive enzyme activities in rainbow trout (Oncorhynchus mykiss) alevin and fry. Can. J. Zoology. 94 (6), 435–442. doi: 10.1139/cjz-2015-0180
Ruchin A. B. (2021). Effect of illumination on fish and amphibian: development, growth, physiological and biochemical processes. Rev. Aquaculture. 13 (1), 567–600. doi: 10.1111/raq.12487
Saiz N., Gomez-Boronat M., De Pedro N., Delgado M. J., Isorna E. (2021). The lack of light-dark and feeding-fasting cycles alters temporal events in the goldfish (Carassius auratus) stress axis. Animals 11 (3), 669. doi: 10.3390/ani11030669
Salgado M. C., Metón I., Egea M., Baanante I. V. (2004). Transcriptional regulation of glucose-6-phosphatase catalytic subunit promoter by insulin and glucose in the carnivorous fish, Sparus aurata. J. Mol. Endocrinol. 33 (3), 783–795. doi: 10.1677/jme.1.01552
Sánchez J. A., López-Olmeda J. F., Blanco-Vives B., Sánchez-Vázquez F. J. (2009). Effects of feeding schedule on locomotor activity rhythms and stress response in sea bream. Physiol. Behav. 98 (1-2), 125–129. doi: 10.1016/j.physbeh.2009.04.020
Sánchez-Vázquez F. J., Azzaydi M., Martinez F. J., Zamora S., Madrid J. A. (1998). Annual rhythms of demand-feeding activity in seabass: evidence of a seasonal phase inversion of the diel feeding pattern. Chronobiology. Int. 15, 607–622. doi: 10.3109/07420529808993197
Sánchez-Vázquez F. J., Tabata M. (1998). Circadian rhythms of demand-feeding and locomotor activity in rainbow trout. J. Fish. Biol. 52 (2), 255–267. doi: 10.1111/j.1095-8649.1998.tb00797.x
Shahkar E., Kim D., Mohseni M., Khara H. (2015). Effects of photoperiod manipulation on growth performance and hematological responses of juvenile Caspian roach Rutilus rutilus caspicus. Fisheries. Aquat. Sci. 18 (1), 51–56. doi: 10.5657/FAS.2015.0051
Shan X. J., Xiao Z. Z., Huang W., Dou S. Z. (2008). Effects of photoperiod on growth, mortality and digestive enzymes in miiuy croaker larvae and juveniles. Aquaculture 281 (1-4), 70–76. doi: 10.1016/j.aquaculture.2008.05.034
Shepherd B. S., Drennon K., Johnson J., Nichols J. W., Playle R. C., Singer T. D., et al. (2005). Salinity acclimation affects the somatotropic axis in rainbow trout. Am. J. Physiology-Regulatory. Integr. Comp. Physiol. 288, R1385–R1395. doi: 10.1152/ajpregu.00443.2004
Singh A., Zutshi B. (2020). Photoperiodic effects on somatic growth and gonadal maturation in mickey mouse platy, Xiphophorus maculatus (Gunther 1866). Fish. Physiol. Biochem. 46 (4), 1483–1495. doi: 10.1007/s10695-020-00806-8
Stephan F. K. (2002). The "other" circadian system: food as zeitgeber. J. Biol. Rhythms. 17 (4), 284–292. doi: 10.1177/074873002129002591
Turker A. (2005). Effects of photoperiod on growth and feed utilization of juvenile black Sea turbot (Psetta maeotica). Israeli. J. Aquaculture-Bamidgeh. 57 (3), 156–163. doi: 10.46989/001c.20413
Valenzuela A., Rodriguez I., Schulz B., Cortes R., Acosta J., Campos V., et al. (2022). Effects of continuous light (LD24:0) modulate the expression of lysozyme, mucin and peripheral blood cells in rainbow trout. Fishes 7 (1), 28. doi: 10.3390/fishes7010028
Vera L. M., De Pedro N., Gomez-Milán E., Delgado M. J., Sánchez-Muros M. J., Madrid J. A., et al. (2007). Feeding entrainment of locomotor activity rhythms, digestive enzymes and neuroendocrine factors in goldfish. Physiol. Behav. 90 (2-3), 518–524. doi: 10.1016/j.physbeh.2006.10.017
Vera L. M., Negrini P., Zagatti C., Frigato E., Sanchez-Vazquez F. J., Bertolucci C. (2013). Light and feeding entrainment of the molecular circadian clock in a marine teleost (Sparus aurata). Chronobiology. Int. 30 (5), 649–661. doi: 10.3109/07420528.2013.775143
Veras G. C., Paixão D. J. D. R., Brabo M. F., Soares L. M. O., Sales A. D. (2016). Influence of photoperiod on growth, uniformity, and survival of larvae of the Amazonian ornamental Heros severus (Heckel 1840). Rev. Bras. Zootecnia-Brazilian. J. Anim. Sci. 45 (7), 422–426. doi: 10.1590/S1806-92902016000700010
Villamizar N., Blanco-Vives B., Migaud H., Davie A., Carboni S., Sánchez-Vázquez F. J. (2011). Effects of light during early larval development of some aquacultured teleosts: A review. Aquaculture 315 (1-2), 86–94. doi: 10.1016/j.aquaculture.2010.10.036
Wagner T., Congleton J. L. (2004). Blood chemistry correlates of nutritional condition, tissue damage, and stress in migrating juvenile chinook salmon (Oncorhynchus tshawytscha). Can. J. Fisheries. Aquat. Sci. 61 (7), 1066–1074. doi: 10.1139/F04-050
Wei H., Cai W. J., Liu H. K., Han D., Zhu X. M., Yang Y. X., et al. (2019). Effects of photoperiod on growth, lipid metabolism and oxidative stress of juvenile gibel carp (Carassius auratus). J. Photochem. Photobiol. B-Biology. 198, 111552. doi: 10.1016/j.jphotobiol.2019.111552
Yada T., Azuma T., Takagi Y. (2001). Stimulation of non-specific immune functions in seawater-acclimated rainbow trout, Oncorhynchus mykiss, with reference to the role of growth hormone. Comp. Biochem. Physiol. Part B.: Biochem. Mol. Biol. 129, 695–701. doi: 10.1016/S1096-4959(01)00370-0
Yasmin F., Sutradhar S., Das P., Mukherjee S. (2021). Gut melatonin: A potent candidate in the diversified journey of melatonin research. Gen. Comp. Endocrinol. 303, 113693. doi: 10.1016/j.ygcen.2020.113693
Ytrestøyl T., Hjelle E., Kolarevic J., Takle H., Rebl A., Afanasyev S., et al. (2022). Photoperiod in recirculation aquaculture systems and timing of seawater transfer affect seawater growth performance of Atlantic salmon (Salmo salar). J. World Aquaculture. Soc. doi: 10.1111/jwas.12880
Zhang X., Gao Y. D., Tang N., Qi J. W., Wu Y. B., Hao J., et al. (2018). One evidence of cocaine- and amphetamine-regulated transcript (CART) has the bidirectional effects on appetite in Siberian sturgeon (Acipenser baerii). Fish. Physiol. Biochem. 44 (1), 411–422. doi: 10.1007/s10695-017-0444-2
Keywords: photoperiod, feeding regime, food anticipatory activity, rainbow trout oncorhynchus mykiss, digestive enzyme
Citation: Xu H, Shi C, Ye Y, Mu C and Wang C (2022) Photoperiod-independent diurnal feeding improved the growth and feed utilization of juvenile rainbow trout (Oncorhynchus mykiss) by inducing food anticipatory activity. Front. Mar. Sci. 9:1029483. doi: 10.3389/fmars.2022.1029483
Received: 27 August 2022; Accepted: 06 October 2022;
Published: 20 October 2022.
Edited by:
Jinghui Fang, Yellow Sea Fisheries Research Institute (CAFS), ChinaReviewed by:
Yiran Hou, Freshwater Fisheries Research Center (CAFS), ChinaCopyright © 2022 Xu, Shi, Ye, Mu and Wang. This is an open-access article distributed under the terms of the Creative Commons Attribution License (CC BY). The use, distribution or reproduction in other forums is permitted, provided the original author(s) and the copyright owner(s) are credited and that the original publication in this journal is cited, in accordance with accepted academic practice. No use, distribution or reproduction is permitted which does not comply with these terms.
*Correspondence: Ce Shi, c2hpY2UzMjEwQDEyNi5jb20=; Chunlin Wang, d2FuZ2NodW5saW5AbmJ1LmVkdS5jbg==
Disclaimer: All claims expressed in this article are solely those of the authors and do not necessarily represent those of their affiliated organizations, or those of the publisher, the editors and the reviewers. Any product that may be evaluated in this article or claim that may be made by its manufacturer is not guaranteed or endorsed by the publisher.
Research integrity at Frontiers
Learn more about the work of our research integrity team to safeguard the quality of each article we publish.