- 1Mueller Lab, Department of Biology, The Pennsylvania State University, University Park, State College, PA, United States
- 2Center for Marine and Environmental Studies, University of the Virgin Islands, St. Thomas, VI, United States
- 3Laboratory of Photobiology, Unidad Académica de Sistemas Arrecifales Puerto Morelos, Instituto de Ciencias del Mar y Limnología, Universidad Nacional Autónoma de Mexico (UNAM), Puerto Morelos, Cancún, QR, Mexico
The upregulation of animal chromoproteins (CPs) during thermal stress produces “colorful” bleached corals that facilitate coral recovery after bleaching. In situ measurements indicate that animal CPs present in coral tissues reduce the elevated internal light environment of the remaining symbionts in bleached or low-pigmented stressed corals. However, there is still a lack of understanding regarding the extent to which animal CPs contribute to modifying the internal light environment of the symbionts in hospite. In this study, we evaluate the effect of three animal CPs on the optical properties of the coral tissue and their internal light environment using a numerical model. The model allows estimations of the absorbance spectra of corals as a function of changes in symbiont and animal pigmentation, as well as descriptions of the light environment in hospite of the symbionts. These descriptions were derived from the quantification of the contribution of each pigment component to light absorption, together with the contribution of the coral skeleton’s reflectance. Simulations indicate that animal CPs upregulation modifies the spectral distribution and the intensity of the internal light field. Animal CPs can reduce up to 11% of the light intensity in hospite when present individually, and up to 24% when present in combination. Such reduction may play a critical role in preventing the full development of the bleached phenotype when irradiance rises to excessive levels at low coral pigmentation, facilitating coral recovery and symbiont tissue re-colonization after bleaching. Accordingly, coral’s CPs components need to also be considered when selecting coral species for future restoration efforts.
1 Introduction
The light environment inside the tissue of scleractinian corals is mainly determined by the incident irradiance on the coral surface and the optical properties of the tissue (Salih et al., 2000; Enríquez et al., 2005; Wangpraseurt et al., 2014; Wangpraseurt et al., 2016) and the skeleton (Enríquez et al., 2005; Terán et al., 2010; Marcelino et al., 2013; Swain et al., 2016; Enríquez et al., 2017). In the tissue, the presence of animal chromoproteins (CPs) (Salih et al., 2000; Smith et al., 2013) and photosynthetic pigments from the symbiotic dinoflagellates (Enríquez et al., 2005; Scheufen et al., 2017b) increases the absorption capacity of the coral pigments. Meanwhile, multiple light scattering by the white skeleton produces diffuse light, reducing pigments’ self-shading while enlarging the optical path, increasing the probability of light absorption by the pigments (Enríquez et al., 2005). However, the reduction of coral skeleton reflectivity due to the presence of endolithic organisms such as the chlorophyte Ostreobium spp. near the skeletal surface strongly affects pigment absorption and modifies the light environment of the symbionts inside the tissue, i.e., in hospite (Galindo-Martínez et al., 2022).
During thermal stress, reductions in the symbiotic dinoflagellate population within the coral tissue result in progressive increases in the light environment in hospite (Wangpraseurt et al., 2017). The remaining symbionts respond to these internal increases in the local illumination by reducing photosynthetic pigment content to photoacclimate to the new light environment (Iglesias-Prieto and Trench, 1994; Enríquez et al., 2005; Gómez-Campo et al., 2022). However, such typical algal response, which is intended to regulate the light dose absorbed by the symbionts, is not an appropriate response for organisms living inside one of the most efficient light collectors in nature (Enríquez et al., 2005; Scheufen et al., 2017b), as it exposes the symbionts in hospite to further increases in local irradiance, activating a positive feedback loop. Under these circumstances, dinoflagellates experience dramatic increases in photodamage that can compromise the symbiotic relationship when all other photoprotective mechanisms are overwhelmed, and the bleached phenotype is finally induced (Scheufen et al., 2017a; Scheufen et al., 2017b; Gómez-Campo et al., 2022). Hence the dramatic increases in the light environment of the symbionts in hospite due to the loss of coral pigmentation (i.e., loss of symbionts and reduction in symbiont pigmentation) is critical for understanding the limits of tolerance of this symbiotic association coral-Symbiodinacea. An important implication of this process is that coral recovery from bleaching remains a challenging task for the symbionts when the external stress is removed because internal light levels are still too high, even when the external irradiance remains constant (Swain et al., 2016; Wangpraseurt et al., 2017). However, corals can recover after bleaching, and populations have the capacity to restore their pigmentation and functionality, surviving the heat stress event (Rodríguez-Román et al., 2006; Bollati et al., 2020; Galindo-Martínez et al., 2022).
The upregulation of animal chromoproteins (CPs) in the animal tissue, which is responsible for creating “colorful” bleached corals (Bollati et al., 2020; Bollati et al., 2022), has been proposed as one of the mechanisms for coral bleaching recovery. The most common group of animal CPs found in corals are the Green Fluorescent Proteins (GFP) (Matz et al., 1999; Salih et al., 2000; Alieva et al., 2008; Smith et al., 2013). They are primarily found in populations of shallow areas, exposed to high-light environments, and their upregulation can be promoted by blue light (Salih et al., 2000; D’Angelo et al., 2008), nutrient stress (Bollati et al., 2022), and exposure to mild heat stress (Bollati et al., 2020). Animal CPs generally accumulate in parts of the coral colony with low symbiont density composition, such as the areas with the highest growth rate, or parts that are particularly damaged (Smith et al., 2013; Bollati et al., 2020). Animal CPs’ function in corals is not clear; however, it has been suggested that animal CPs can help in coral photoprotection to high levels of UVR or PAR (Salih et al., 2000; Smith et al., 2013; Gittins et al., 2015; Quick et al., 2018), antioxidant activity (Bou-Abdallah et al., 2006), and camouflage (Matz et al., 2006). They have also been proposed to amplify light scattering and increase the temperature within coral tissue (Lyndby et al., 2016). It also has been suggested that animal CPs could help corals attract symbionts (Aihara et al., 2019) and prey such as plankton (Ben-Zvi et al., 2022).
Due to their chemical composition, animal CPs can absorb light in the visible and UV range. Based on their optical properties, animal CPs can be classified as fluorescent proteins (FPs) and non-fluorescent chromoproteins (CPs) (Alieva et al., 2008). FPs can reemit light at particular wavelengths, a process known as photoconversion (Dove et al., 2001; D’Angelo et al., 2008; Smith et al., 2013; Bollati et al., 2022). Hence, it has been suggested that animal CPs could enhance algal photosynthesis in low light environments by modifying the light spectrum and intensity (Schlichter et al., 1994; Salih et al., 2000; Bollati et al., 2022). Nevertheless, considering that the number of photons generated through photoconversion is minimal compared to ambient light, its contribution to photosynthesis may be negligible (Ben-Zvi et al., 2021; Bollati et al., 2022). In situ spectral scalar irradiance in corals indicate that the presence of animal CPs reduces the light environment in hospite at particular wavelengths (Bollati et al., 2022) due to the CPs’ light absorption capacity. However, there is still a limited understanding of the animal CPs’ contribution to the modification of the light environment in the PAR range of the coral symbionts in hospite.
In this study, we analyzed the damping effect of three non-fluorescent animal CPs (asulCP562, amilCP580, and amilCP604) in the internal light environment of coral tissues using a numerical model for light transmission in corals. The model assumes light attenuation in the coral tissue due to light absorption by symbiotic photosynthetic pigments and animal CPs, and the additional effect of skeleton light scattering. The portion of light reaching the skeleton is scattered back to the tissue in different ratios depending on the optical properties of the skeleton. Simulations indicate that upregulation of animal CPs in the coral tissue can reduce internal irradiance up to 24% when present in combination.
2 Materials and methods
2.1 Numerical model
The numerical model for light transmission in corals previously reported (Galindo-Martínez et al., 2022) was modified to further explore the effects of animal CPs in the internal light environment of coral tissue. Briefly, the model assumes a flat coral where polyps are retracted, and the transparent coral tissue, containing the spherical photosynthetic symbionts, sits on top of the calcium carbonate skeleton. In the model, the skeleton reflectance can be modified due to the presence of the endolithic algae Ostreobium near the skeletal surface (Galindo-Martínez et al., 2022) (Figure 1A).
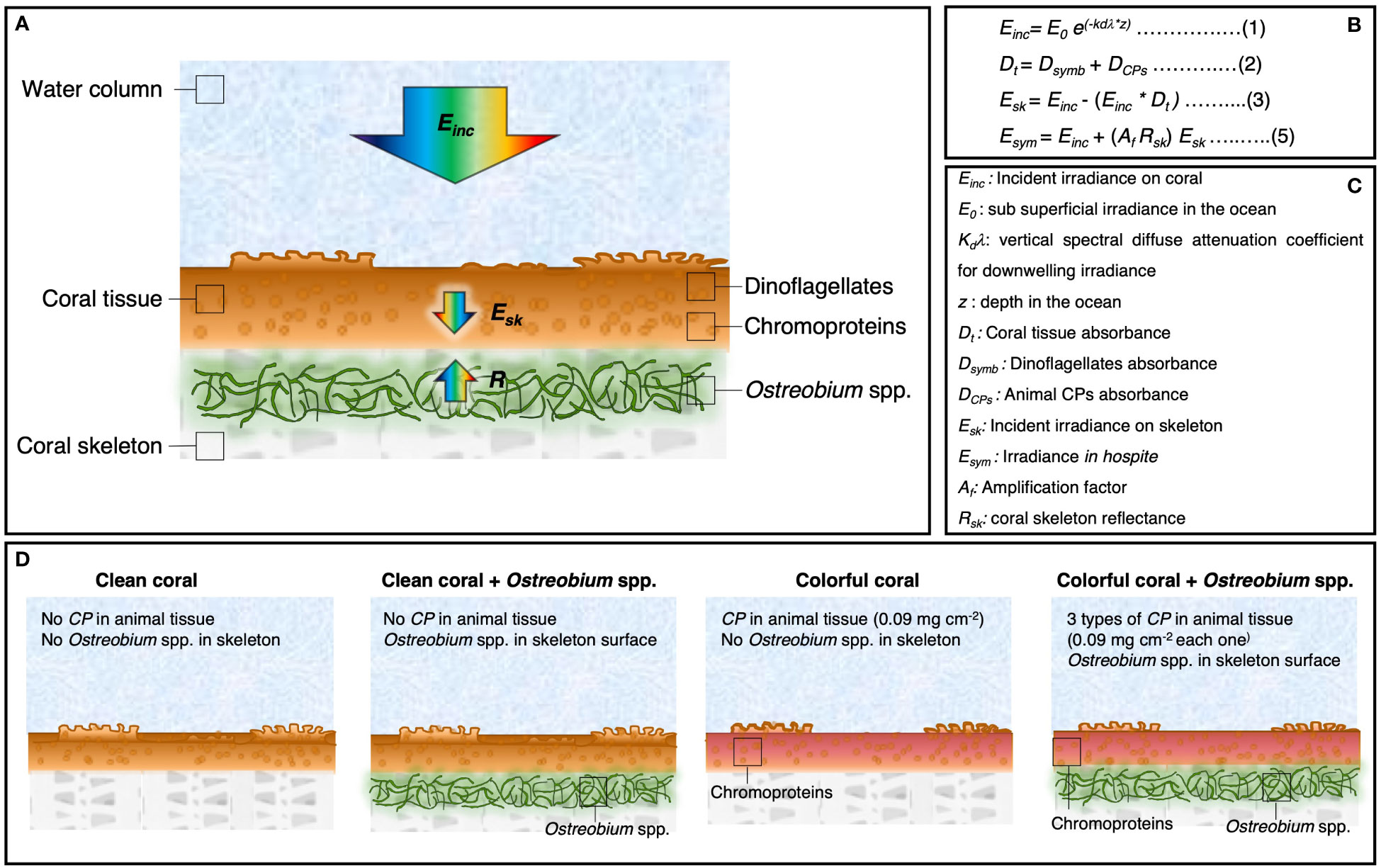
Figure 1 Description of the numerical model for light absorption in corals. (A) Schematic representation of the coral model. Arrows indicate the parameters used to determine the light environment in hospite for the symbionts. (B) Equations used in the numerical model for the calculation of (1) the incident irradiance on the coral, (2) the coral tissue absorbance, (3) the incident irradiance in the skeleton, and (5) and the light environment in hospite for the symbionts. (C) Description of the variables used in the model. (D) Different scenarios of light absorption in corals: a clean coral with a white skeleton (no chromoproteins, no Ostreobium spp.), clean coral with an Ostreobium spp. bloom near the skeletal surface, a colorful coral with one of the three chromoproteins upregulated in the tissue: asulCP562, amilCP580, or amilCP604 (0.09 mg cm-2), and a colorful coral with an Ostreobium spp. bloom near the skeletal surface.
The model considers that 1) the incident light on the coral surface is attenuated inside the tissue by the photosynthetic pigments of the symbiotic dinoflagellates and by the animal CPs when present, and 2) that the light that is not attenuated and reaches the skeletal surface is scattered back to the coral tissue as a function of coral skeleton reflectance (R) (Figure 1A). By doing so, the model can predict the coral absorbance spectra and the light environment in hospite for the symbionts with 0.2 nm resolution in the PAR range (400 to 700 nm) as a function of the Chla density due to the presence of the symbiotic dinoflagellates, the animal CP density when upregulated in the animal tissue, and the skeleton reflectance (Figures 1B, C). See detailed equations in supplementary materials.
To predict the light environment for the symbionts inside the coral tissue, the numerical model was designed in the software Excel version 16.66.1 based on data previously reported: 1) the vertical spectral diffuse attenuation coefficient (KdΛ) for downwelling irradiance in the ocean reported by López-Londoño et al. (2021), 2) the light absorption spectra of a coral measured in transmittance mode (Enríquez et al., 2005), 3) the in vitro absorption spectra of three animal CPs isolated from the coral tissue (asulCP562, amilCP580, and amilCP604) (Smith et al., 2013), 4) the light transmission properties of coral skeletons (Vásquez-Elizondo et al., 2017), and 5) the in vivo absorption spectra of Ostreobium spp. in coral skeletons (Galindo-Martínez et al., 2022). Transmission absorption spectra of coral tissues (Enríquez et al., 2005) and animal CPs (Smith et al., 2013) were digitized using the software PlotDigitizer (Version 2.6.9). Each animal CP considered for the model presents a particular absorption peak at 562, 580, and 604 nm for asulCP562, amilCP580, and amilCP604, respectively (Figure 2) (Smith et al., 2013).
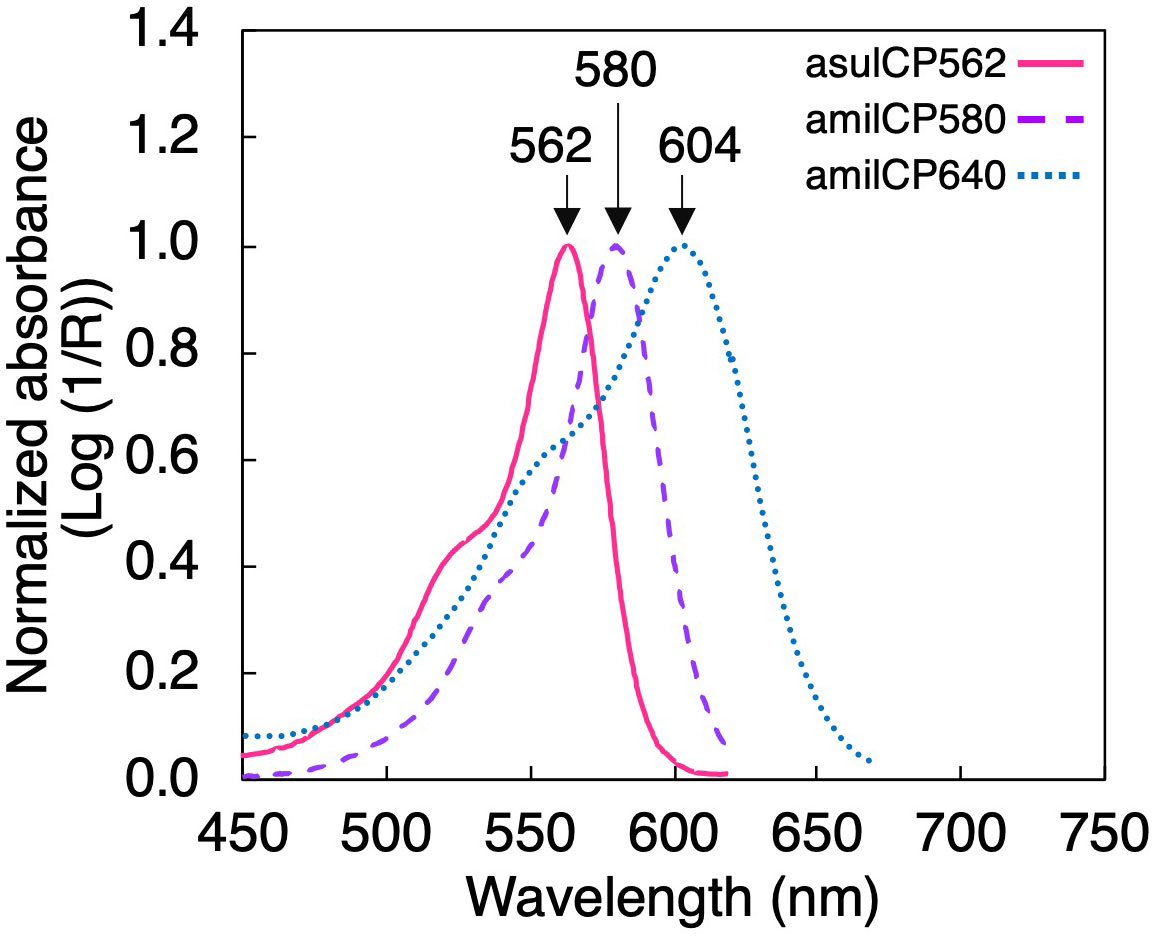
Figure 2 In vitro light absorption spectra for the three coral chromoproteins used in the numerical model: asulCP562 (pink solid line), amilCP580 (purple dashed line), and amilCP604 (blue dotted line) extracted from coral tissue (after Smith et al., 2013).
For the model’s components, equations were designed to predict absorption with 0.2 nm resolution in the PAR range (400 to 700 nm) (Figures 1B, C) (See Supplementary materials). The first component, KdΛ (López-Londoño et al., 2021), allows quantification of the incident irradiance in the coral (Einc) as a function of depth (z). Where E0 is the sub-superficial irradiance in the ocean
The second and third components enable the prediction of light absorption in the coral tissue (Dt) by adding the absorbance of the dinoflagellates (Dsym) and the animal chromoproteins (DCP). These absorbance spectra were predicted using equations based on an exponential attenuation as a function of algal and animal pigment densities. Dt was later used for the calculation of the incident irradiance in the skeleton (Esk).
The fourth and fifth components, coral skeleton transmittance (Tsk) and Ostreobium spp. absorption (Dost), allow us to calculate the skeleton’s reflectance (Rskos) considering the effects of the endolithic algae Ostreobium spp. when present in the skeleton. The absorbance spectrum of Ostreobium spp. was calculated using equations based on an exponential attenuation as a function of Ostreobium spp. density and its position in the skeleton (Galindo-Martínez et al., 2022).
Finally, using the previous parameters, the light environment in hospite for the symbionts (Esym) was calculated as
In this expression, Einc is the incident irradiance on the coral surface, Af is the amplification factor for a flat surface as Chla density changes (Enríquez et al., 2005), Rskos is the coral skeleton reflectance, and Esk is the incident downwelling irradiance on the coral skeleton (Figure 1B). The amplification factor indicates the enhancement of the light absorption by the symbionts in hospite due to the presence of the coral skeleton in comparison with symbionts in suspension with the same amount of pigmentation (Enríquez et al., 2005).
2.2 Numerical model simulations
To quantify the damping effect of animal CPs in the light environment in hospite, simulations were run in the numerical model assuming different coral scenarios with different Chla densities (100, 75, 50, 25, 12, and 6 mg Chla m-2) that correspond to variations in coral pigmentation from a well-pigmented coral (100 mg Chla m-2), to a bleached coral (less than 6 mg Chla m-2). The scenarios proposed represent corals with variations in the optical properties of the coral tissue and/or the coral skeleton, such as 1) a “clean” coral, representing a coral with no animal CPs in the tissue and with a white skeleton, 2) a “clean” coral with an Ostreobium spp. bloom located near the skeletal surface (less than 0.1 mm from the skeletal surface), 3-5) a “colorful” coral, representing a coral with one of the three animal CPs (asulCP562 (3), amilCP580 (4), and amilCP604 (5) (Smith et al., 2013)) upregulated in its tissue, assuming an animal CPs density of 0.09 mg cm-2 as reported by Smith et al., 2013, and with a white coral skeleton, and lastly, 6) a “colorful” coral with the three animal CP’s (asulCP562, amilCP580 and, amilCP604) upregulated in its tissue and with an Ostreobium spp. bloom located near the skeletal surface (Figures 1B–D). Simulations were run in the software Excel version 16.66.1. For each scenario, coral absorption spectra, percentage of light absorbed by each model component, and variation in the light environment in hospite were predicted.
2.3 Estimations of ACPs specific absorption coefficient
For the calculation of the specific absorption coefficient (Morel and Bricaud, 1981) of each animal CP (a*CP562, a*CP580, and a*CP604 for asulCP562, amilCP580, and amilCP604, respectively; m2 Chla-1), we used the predicted coral absorbance from the simulations run in the scenarios previously mentioned. In the simulations, the CPs’ density was maintained constant (0.09 mg cm-2) and only the Chla density in the coral was modified (100, 75, 50, 25, 12, and 6 mg Chla m-2). For the calculation of the a*CP we used the equation:
Where ρ is the Chla content per projected area in the coral (mg Chla m-2) (Enríquez and Sand‐Jensen, 2003; Enríquez et al., 2005) and D is the absorbance value predicted for the coral by the numerical model at 562 nm, 580 nm, and 604 nm, for a*CP562, a*CP580, and a*CP604 respectively. This parameter, a*CPs, was calculated only for the absorbance value at the peak of absorption of each animal CPs. It can be considered a descriptor of the pigment light absorption efficiency of the animal CPs in hospite. An ANCOVA test was used to identify significant differences among animal chromoproteins. The statistical analysis was conducted using the software SPSS Statistics (Version 28).
3 Results
3.1 Light absorption
The numerical model indicates that the coral tissue of bleached/pale corals is able to absorb three times less incident light in the PAR range than pigmented corals (Figures 3A, G), allowing more incident light to reach the coral skeleton. The accumulation of chromoproteins in the coral tissue increases light absorption (Figures 3B-D). However, each chromoprotein contributes in a different proportion; asulCP562, amilCP604, and amilCP580 can absorb up to 13%, 15%, and 24%, respectively, of the incident light in a bleached/pale coral (3.3 mg Chla m-2) (Figures 3B-D). On the contrary, a pigmented coral (100 mg Chla m-2) can only absorb up to 5%, 6%, and 7%, respectively (Figures 3H-J). When Ostreobium spp. bloom in the skeletal surface, they can absorb up to 54% of the incident light in a bleached/pale coral (Figure 3E, F), and only up to 10% in a pigmented organism (Figure 3K, L). However, if Ostreobium spp. is located near the skeletal surface of a “colorful” bleached coral, it can absorb only 14% of the incident light because the three animal CPs absorb up to 52% of the incident light (Figure 3F).
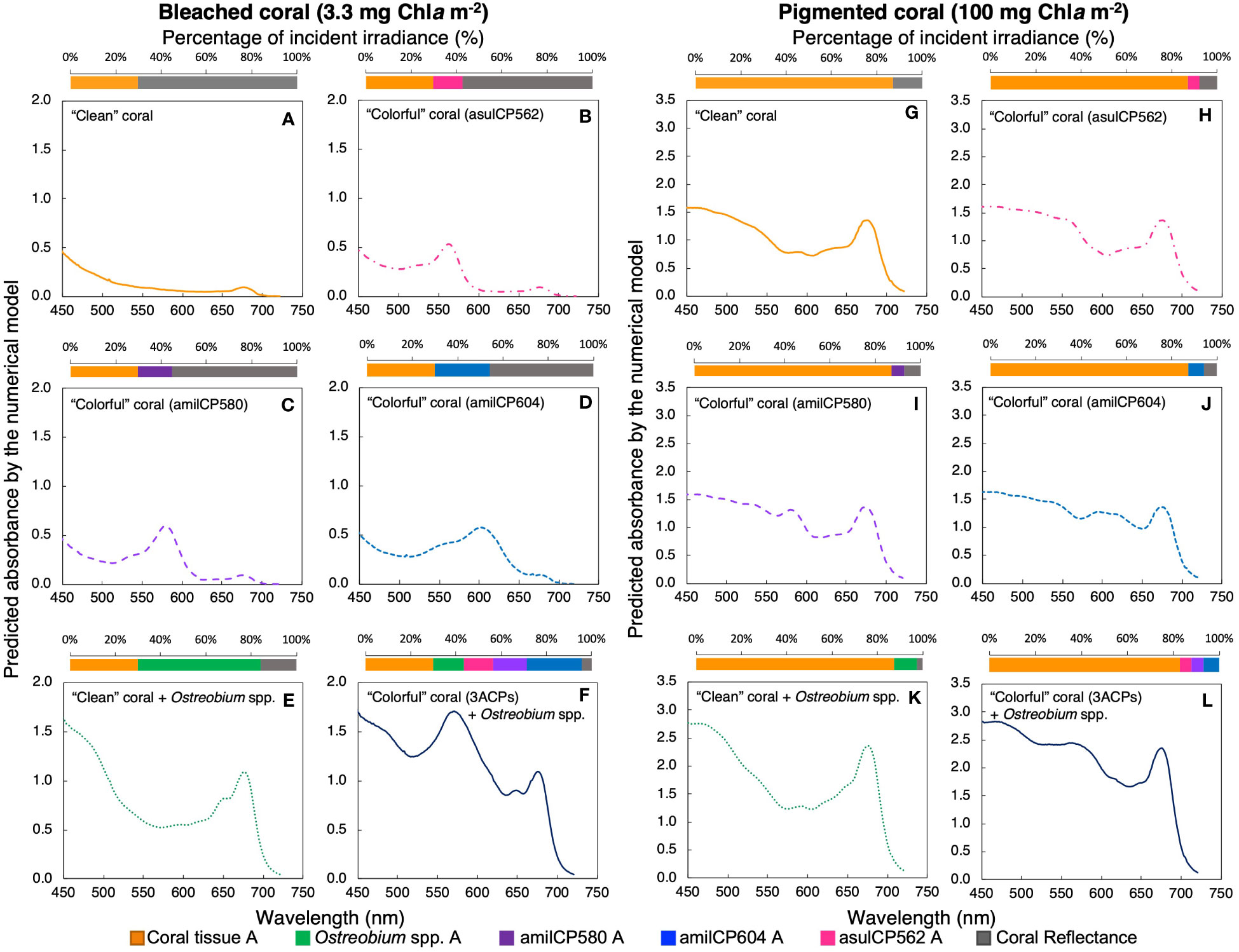
Figure 3 Predicted absorption spectra for a bleached (A-F, 3.3 mg Chla m-2) and a pigmented coral (G-L, 100 mg Chla m-2) under different scenarios: a coral with a white skeleton (yellow solid line, A, G), a coral with the chromoprotein asulCP562 upregulated in its tissue (pink dotted line, B, H), a coral with the chromoprotein amilCP580 upregulated in its tissue (purple dashed line, C, I), a coral with the chromoprotein amilCP604 upregulated in its tissue (clear blue dotted line, D, J), a coral with an Ostreobium bloom in the skeletal surface (green dotted line, E, K), and a coral with the three chromoproteins upregulated in its tissue and an Ostreobium bloom in the skeletal surface (navy blue solid line, F, L). Superior panels indicate the percentage of incident PAR utilized by each pigmented layer: coral tissue (yellow), chromoprotein asulCP562 (pink), chromoprotein amilCP580 (purple), chromoprotein amilCP604 (blue), Ostreobium spp. (green), and coral reflectance (gray).
To quantify the variability in animal CPs’ light absorption efficiency, differences in the specific absorption coefficient were estimated for each chromoprotein (a*CP), as well as its variability as a function of Chla density for corals of different pigmentation. Model simulations indicate that a*CP increases with reductions in pigment density in the coral. However, they did not show significant differences between chromoproteins (ANCOVA, f(15)=0.015, p>0.05) (Figure 4). The a*CP varied from a minimum of 0.029 m2 mg Chla -1 for a pigmented coral (100 mg Chla m-2) to a maximum of 0.284 m2 mg Chla -1 for a bleached coral (6 mg Chla m-2) (Figure 4).
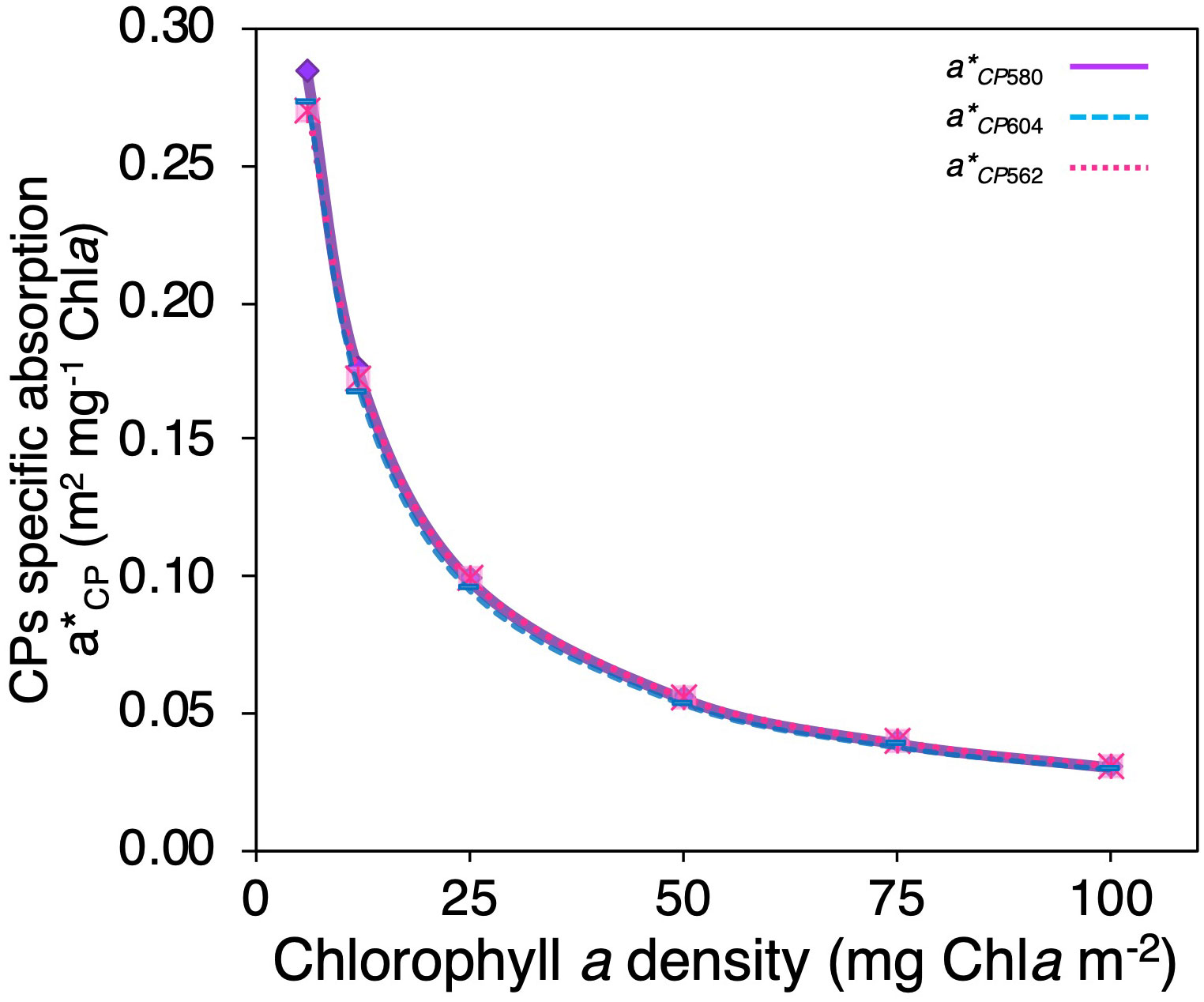
Figure 4 Comparison of changes in animal CP specific absorption coefficient (a*CP) as a function of Chla density of “colorful” corals for the three animal CPs used in the numerical model asulCP562 (pink dotted line), amilCP580 (purple solid line), and amilCP604 (clear blue dashed line).
3.2 Light environment in hospite
Our simulations indicate that reductions in Chla density in the coral allow more incident light to reach the skeleton, producing dramatic increases in the light environment in hospite of the symbionts due to the extraordinary skeleton’s capacity to scatter light (Figure 5). Under the “clean” coral scenario, the model estimated a 2.8-fold increase in light levels in hospite for bleached corals compared to a pigmented coral (Figures 5A, G). However, when chromoproteins were present in the tissue of “colorful” bleached corals, the in hospite light environment was amplified between 2.3 to 2.6 times (Figures 5B-D), relative to the amplification estimated for “colorful” pigmented corals (Figures 5H-J). Combining the presence of the three chromoproteins in the same bleached coral, a 2.1-fold increase in the light levels in hospite was estimated. Finally, for the last scenario, the combined presence of Ostreobium spp. and the three chromoproteins resulted in only a 1.3-fold increase in the light environment in hospite of the symbionts for “colorful” bleached corals (Figure 5F).
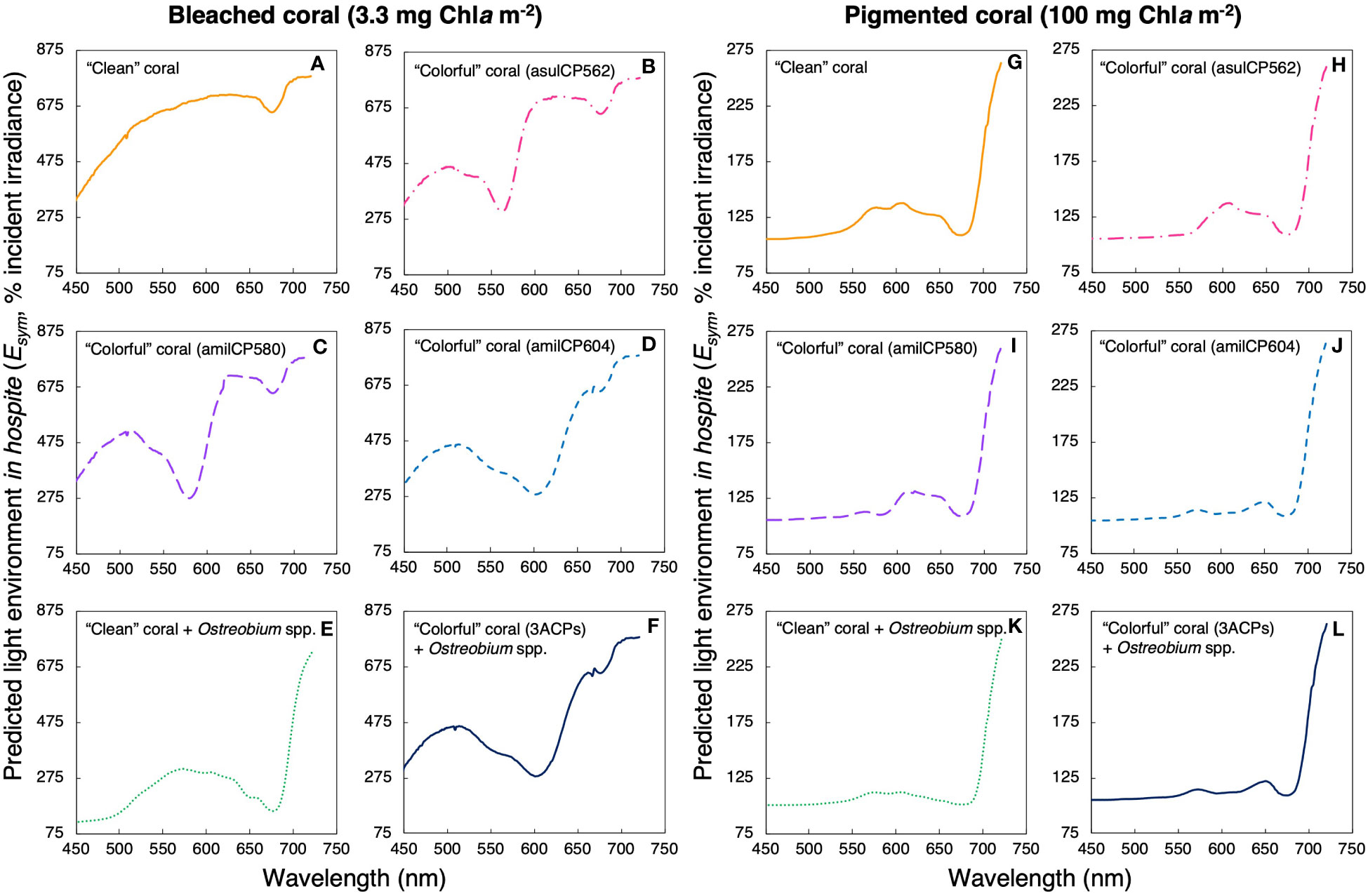
Figure 5 Predicted light environment in hospite for a bleached coral (A-F, 3.3 mg Chla m-2) and a pigmented coral (G-L, 100 mg Chla m-2) under different scenarios: a coral with a white skeleton (yellow solid line, A, G), a coral with the chromoprotein asulCP562 upregulated in its tissue (pink dotted line, B, H), a coral with the chromoprotein amilCP580 upregulated in its tissue (purple dashed line, C, I), a coral with the chromoprotein amilCP604 upregulated in its tissue (clear blue dotted line, D, J), a coral with an Ostreobium bloom in the skeletal surface (green dotted line, E, K), and, a coral with the three chromoproteins upregulated in its tissue and an Ostreobium bloom in the skeletal surface (navy blue solid line, F, L).
These simulations showed that the presence of optical dampers, such as animal CPs in coral tissues or Ostreobium spp. in the skeleton, reduces the light levels in hospite for the symbionts regardless of the variation in coral pigmentation (i.e., Chla density) (Figure 6). Nevertheless, corals with less Chla density showed more considerable reductions of the light environment in hospite for the symbionts when dampers were present, relative to regular pigmented corals. In bleached corals, the presence of animal CPs reduces between 11 to 24% of the light environment in hospite of the symbionts (Figures 5B-D; Figure 6) relative to tissues of “clean” bleached corals (Figure 5A; Figure 6). Meanwhile, in pigmented corals, the presence of animal CPs in the tissue leads to reductions of 2-6% (Figures 5H-J; Figure 6) in comparison to “clean” pigmented corals (Figure 5G; Figure 6). In contrast, the presence of Ostreobium spp. near the skeletal surface can reduce up to 53% of the light environment in hospite of the symbionts (Figure 5E; Figure 6).
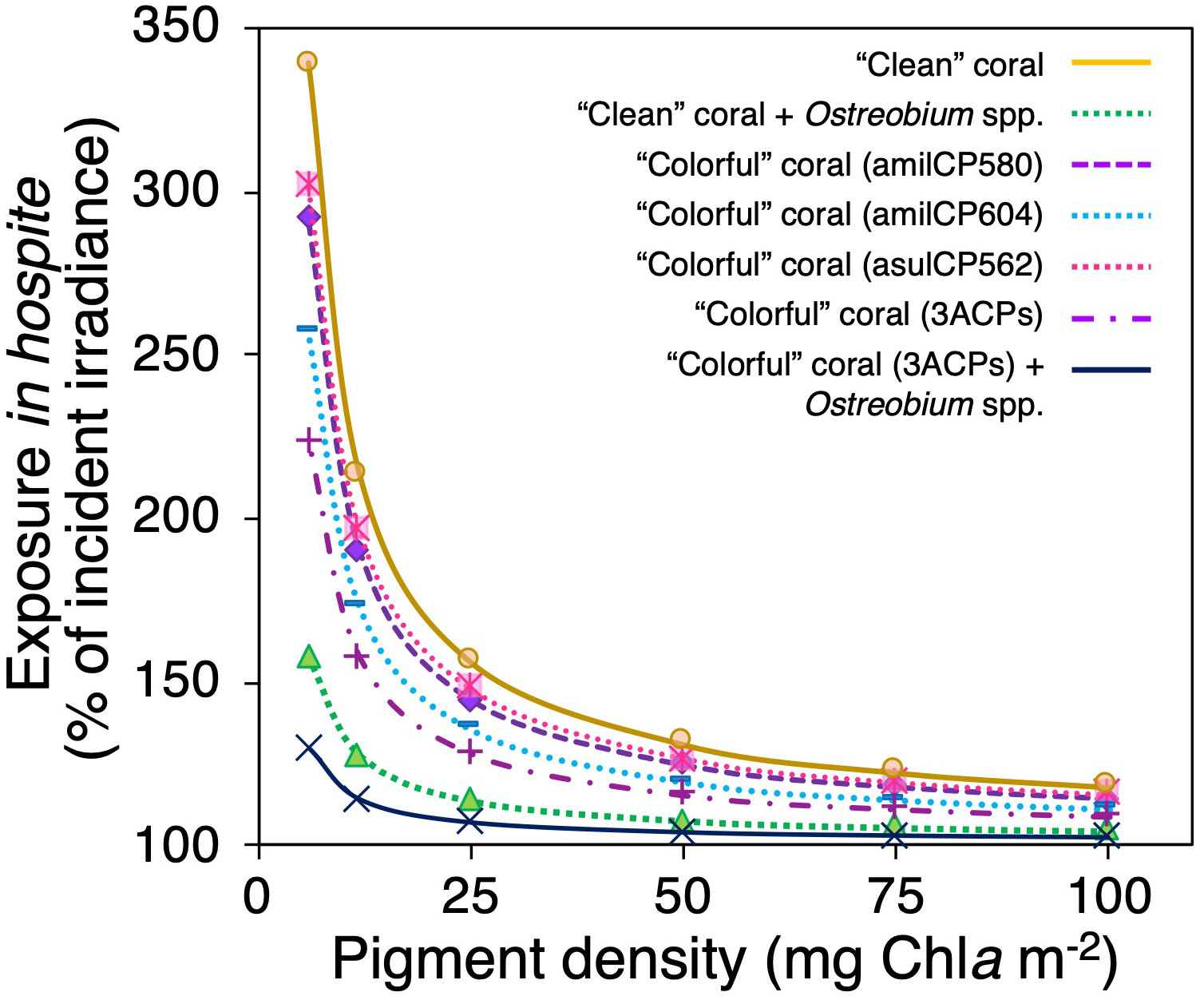
Figure 6 Modification of the light environment in hospite as a function of the pigment content for different coral scenarios, a “clean” coral with a white skeleton (yellow solid line), a coral with an Ostreobium bloom in the skeletal surface (green dotted line), a “colorful” coral with the chromoprotein asulCP562 upregulated in its tissue (pink dotted line), a “colorful” coral with the chromoprotein amilCP580 upregulated in its tissue (purple dashed line), a “colorful” coral with the chromoprotein amilCP604 upregulated in its tissue (clear blue dotted line), a “colorful” coral with the three chromoproteins upregulated in its tissue (purple dotted-dashed line), and a “colorful” coral with the three chromoproteins upregulated in its tissue and with an Ostreobium bloom in the skeletal surface (navy blue solid line).
4 Discussion
The use of numerical models allows us to predict light absorbance spectra in corals (Figure 3) under different scenarios of pigment composition in their tissue and/or skeleton (Figure 1). Models determine the percentage of incident light absorbed by each component and variations in coral skeleton’s reflectance, allowing estimation of potential changes in the light environment in hospite of the symbiotic dinoflagellates (Figures 5, 6). Our simulations indicated that reductions in light absorption in bleached corals, due to decreases in Chla density, may increase up to three times the internal light field of bleached corals, and that the main determinant is the presence of a highly reflective coral skeleton (Figures 5A, G). However, the presence of optical dampers in the tissue and/or the coral skeleton allows for significant reductions in the light environment in hospite (Figure 5). When corals upregulate the production of CPs in their tissue, the light environment in hospite of the symbionts reduces between 11- 24% in colorful bleached corals relative to “clean” bleached corals. (Figures 5B-D). Interestingly, when Ostreobium spp. increase in abundance near the skeletal surface, “colorful” bleached corals can reduce up to 53% of the light environment in hospite of the symbionts (Figure 5F).
Measurements of in vivo scalar irradiances within tissues of bleached corals have documented 20% reductions in irradiances at the animal CP absorption peaks when they are present (Bollati et al., 2022). Our model predicts similar reductions for the CPs reported by Bollati et al. (2022). It also supports the critical contribution of animal CPs to the regulation of the spectral distribution and intensity of the light environment of the symbionts in hospite (Figure 6), according to their light absorption characteristics (Salih et al., 2000; Dove et al., 2001). Photoconversion has been suggested as another possible role of animal CPs (Wiedenmann et al., 2004; Bollati et al., 2022). This phenomenon denotes the capacity of a particular group of green fluorescent pigments (GFP), known as photoconvertibles RFPs (pcRFPs), to absorb light in the blue-green range and reemit it in the orange-red range (Wiedenmann et al., 2004; Bollati et al., 2022). However, considering that dinoflagellates have poor light absorption in the orange range, animal CPs photoconversion may not be as relevant in enhancing their photosynthetic activity (Ben-Zvi et al., 2021). Indeed, the number of photons generated through photoconversion is insignificant compared to those provided by ambient light (Bollati et al., 2022).
The effect of animal CPs on the light environment within coral tissues varies depending on the type and density of the CPs and symbiont pigment density (Figure 6). A combination of CPs has a more substantial effect damping the light environment within bleached corals than the presence of a single type of CPs (Figure 6). In this sense, the combination of animal CPs in addition with a bloom of the endolithic algae Ostreobium spp. near the skeletal surface, produce the largest light attenuation in our simulations (Figure 5; Figure 6) due to the synergistic effect of the increases in light absorption and reductions in skeleton light scattering. Moreover, model predictions indicate that the combination of the three animal CPs used in our simulations and coral pigmentation values above 50 mg Chla m-2 allow the coral to absorb most of the light available in the tissue (Figure 3L). This condition minimizes the amount of incident light reaching the skeleton, with the consequent control of Ostreobium spp. blooms. Therefore, the last scenario simulated in the model could only occur in low-pigmented corals (<40 mg Chla m-2).
Multiple light scattering on coral skeleton together with reductions in Chla density during stressful conditions could affect both the animal CPs upregulation as well their efficiency of light absorption. As the upregulation of CPs in corals can be stimulated with light around 450 nm (D’Angelo et al., 2008; Bollati et al., 2020), the amplification of the light environment due to reductions in coral Chla density also involves higher levels of blue light within the coral tissue (Figure 5), and in consequence, facilitates animal CPs upregulation before the bleached phenotype is expressed (Gómez-Campo et al., 2022). Accordingly, bleaching may not be necessary for inducing animal CPs upregulation (Bollati et al., 2020) as a small reduction in pigment density could be enough to enhance blue light levels within coral tissues. In addition, the amplification of the light environment enhances light availability in all wavelengths in the PAR range, increasing the probability of light absorption by both pigment types, symbiotic and animal. This effect can be quantified by each pigment’s specific absorption coefficients (a*). Significant enhancement in light absorption efficiency of Chla (a*Chla) at low pigmentation has already been documented (Enríquez et al., 2005; Scheufen et al., 2017a; Scheufen et al., 2017b), and our analysis estimated similar enhancements for animal CPs pigments (a*CP) in bleached/paled coral tissue (Figure 4). This finding supports the capacity of animal CPs to act as optical dampers, even at small concentrations.
The presence of animal CPs in the coral tissue could simply be considered as a mechanism that facilitates coral recovery after bleaching (Bollati et al., 2020; Bollati et al., 2022). However, our results also support their utility in preventing the induction of the optical feedback loop that leads to the development of the bleached coral phenotype (Gómez-Campo et al., 2022). Contrary to CPs upregulation, Ostreobium spp. require a substantial reduction in Chla density to bloom near the skeletal surface. Once Ostreobium spp. blooms, its presence near the skeletal surface can also modify the light environment in hospite of the symbionts (Galindo-Martínez et al., 2022). This coral condition facilitates coral recovery after bleaching and may help in future bleaching events. Consequently, the effect of the combination of both mechanisms (CPs upregulation and Ostreobium spp. blooms) on the optical properties of coral tissue and skeleton creates less efficient corals for light absorption but more robust under heat stress and with a higher capacity of recovery after bleaching.
Finally, the light absorption properties of each CP determine its specific characteristics as a light-damper. Consequently, differences in coral vulnerability to bleach under thermal stress or the coral’s ability for recovery after bleaching may also be regulated by the coral’s ability to maintain/enhance animal CPs production and/or the type of animal pigment upregulated. More research is still needed to understand further possible trade-offs in coral physiology associated with animal CPs production. However, their presence and variability in coral tissues could be an excellent criterion when considering species for coral restoration efforts.
Data availability statement
The original contributions presented in the study are included in the article/Supplementary Material. Further inquiries can be directed to the corresponding authors.
Author contributions
CG-M and RI-P designed the research; CG-M and AC participated in data collection; CG-M analyzed data and created the numerical model; CG-M performed numerical model simulations; CG-M, SE, and RI-P wrote the paper. All authors contributed to the article and approved the submitted version.
Funding
The research leading to these results has received funding from Penn State University startup funds to RI-P. The Mexican Consejo Nacional de Ciencia y Tecnología (CONACyT) is acknowledged by providing one year Ph.D. fellowship to support CG-M (Conv-377983/246185). CG-M, AC, and RI-P were funded by National Science Foundation’s INCLUDES program.
Acknowledgments
We acknowledge Penn State University, and the NSF INCLUDES Alliance Collaborative Research program for MMES students that allow the collaboration between CG-M, RI-P, and AC. The PASPA program of DGAPA-UNAM is acknowledged for providing a scholarship to SE to support a sabbatical period at Penn State University. We want to thank the two reviewers for their help in improving the original submitted manuscript.
Conflict of interest
The authors declare that the research was conducted in the absence of any commercial or financial relationships that could be construed as a potential conflict of interest.
Publisher’s note
All claims expressed in this article are solely those of the authors and do not necessarily represent those of their affiliated organizations, or those of the publisher, the editors and the reviewers. Any product that may be evaluated in this article, or claim that may be made by its manufacturer, is not guaranteed or endorsed by the publisher.
Supplementary material
The Supplementary Material for this article can be found online at: https://www.frontiersin.org/articles/10.3389/fmars.2022.1029201/full#supplementary-material
References
Aihara Y., Maruyama S., Baird A. H., Iguchi A., Takahashi S., Minagawa J. (2019). Green fluorescence from cnidarian hosts attracts symbiotic algae. Proc. Natl. Acad. Sci. 116 (6), 2118–2123. doi: 10.1073/pnas.1812257116
Alieva N. O., Konzen K. A., Field S. F., Meleshkevitch E. A., Hunt M. E., Beltran-Ramirez V., et al. (2008). Diversity and evolution of coral fluorescent proteins. PloS One 3 (7), e2680. doi: 10.1371/journal.pone.0002680
Ben-Zvi O., Wangpraseurt D., Bronstein O., Eyal G., Loya. Y. (2021). Photosynthesis and bio-optical properties of fluorescent mesophotic corals. Front. Mar. Sci. 8. doi: 10.3389/fmars.2021.651601
Ben-Zvi O., Lindemann Y., Eyal G., Loya Y. (2022). Coral fluorescence: a prey-lure in deep habitats. Commun. Biol. 5 (1), 537. doi: 10.1038/s42003-022-03460-3
Bollati E., D´Angelo C., Alderdice R., Pratchett M., Zielgler M., Wiedenmann J. (2020). Optical feedback loop involving dinoflagellate symbiont and scleractinian host drives colorful coral bleaching. Curr. Biol 30, 1-13. doi: 10.1016/j.cub.2020.04.055
Bollati E., Lyndby N. H., D´Angelo C., Kühl M., Wiedenmann J., Wangpraseurt D., et al. (2022). Green fluorescent protein-like pigments optimise the internal light environment in symbiotic reef-building corals. eLife 11, e73521. doi: 10.7554/eLife.73521
Bou-Abdallah F., Chasteen N. D., Lesser M. P. (2006). Quenching of superoxide radicals by green fluorescent protein. Biochim. Biophys. Acta (BBA) - Gen. Subj. 1760 (11), 1690–1695. doi: 10.1016/j.bbagen.2006.08.014
D’Angelo C., Denzel A., Vogt A., Matz Mv., Oswald F., Salih A., et al. (2008). Blue light regulation of host pigment in reef-building corals. Mar. Ecol. Prog. Ser. 364, 97–106. doi: 10.3354/meps07588
Dove S. G., Hoegh-Guldberg O., Ranganathan S. (2001). Major colour patterns of reef-building corals are due to a family of GFP-like proteins. Coral Reefs 19 (3), 197–204. doi: 10.1007/PL00006956
Enríquez S., Méndez E. R., Hoegh-Guldberg O., Iglesias-Prieto R. (2017). Key functional role of the optical properties of coral skeletons in coral ecology and evolution. Proc. R. Soc. B: Biol. Sci. 284 (1853), 20161667. doi: 10.1098/rspb.2016.1667
Enríquez S., Méndez E. R., Iglesias-Prieto R. (2005). Multiple scattering on coral skeletons enhances light absorption by symbiotic algae. Limnol. Oceanogr. 50 (4), 1025–1032. doi: 10.4319/lo.2005.50.4.1025
Enríquez S., Sand-Jensen K. (2003). Variation in light absorption properties of Mentha aquatica l. as a function of leaf form: Implications for plant growth. Int. J. Plant Sci. 164 (1), 125–136. doi: 10.1086/344759
Galindo-Martínez C. T., Weber M., Ávila-Magaña V., Enríquez S., Kitano H., Medina M., et al. (2022). The role of the endolithic alga Ostreobium spp. during coral bleaching recovery. Sci. Rep. 12 (1), 2977. doi: 10.1038/s41598-022-07017-6
Gittins J. R., D’Angelo C., Oswald F., Edwards R. J., Wiedenmann J. (2015). Fluorescent protein-mediated colour polymorphism in reef corals: multicopy genes extend the adaptation/acclimatization potential to variable light environments. Mol. Ecol. 24 (2), 453–465. doi: 10.1111/mec.13041
Gómez-Campo K., Enríquez S., Iglesias-Prieto R. (2022). A road map for the development of the bleached coral phenotype. Front. Mar. Sci. 9. doi: 10.3389/fmars.2022.806491
Iglesias-Prieto R., Trench R. K. (1994). Acclimation and adaptation to irradiance in symbiotic dinoflagellates. i. responses of the photosynthetic unit to changes in photon flux density. Mar. Ecol. Prog. Ser. 113 (1), 163–175. doi: 10.3354/meps113136
López-Londoño T., Galindo-Martínez C. T., Gómez-Campo K., González-Guerrero L. A., Roitman S., Pollock F. J., et al. (2021). Physiological and ecological consequences of the water optical properties degradation on reef corals. Coral Reefs 40 (4), 1243–1256. doi: 10.1007/s00338-021-02133-7
Lyndby N. H., Kühl M., Wangpraseurt D. (2016). Heat generation and light scattering of green fluorescent protein-like pigments in coral tissue. Sci. Rep. 6 (1), 26599. doi: 10.1038/srep26599
Marcelino L. A., Westeat M. W., Stoyneva V., Henss J., Rogers J. D., Radosevich A., et al. (2013). Modulation of light-enhancement to symbiotic algae by light-scattering in corals and evolutionary trends in bleaching. PloS One 8 (4), e61492. doi: 10.1371/journal.pone.0061492
Matz M. V., Fradkov A. F., Labas Y. A., Savitsky A. P., Zaraisky A. G., Markelov M. L., et al. (1999). Fluorescent proteins from nonbioluminescent anthozoa species. Nat. Biotechnol. 17 (10), 969–973. doi: 10.1038/13657
Matz M. V., Marshall N. J., Vorobyev M. (2006). Are corals colorful? Photochem. Photobiol. 82 (2), 345–350. doi: 10.1562/2005-08-18-RA-653
Morel A., Bricaud A. (1981). Theoretical results concerning light absorption in a discrete medium, and application to specific absorption of phytoplankton. Deep Sea Res. Part A. Oceanogr. Res. Pap. 28 (11), 1375–1393. doi: 10.1016/0198-0149(81)90039-X
Quick C., D’Angelo C., Wiedenmann J. (2018). Trade-offs associated with photoprotective green fluorescent protein expression as potential drivers of balancing selection for color polymorphism in reef corals. Front. Mar. Sci. 5. doi: 10.3389/fmars.2018.00011
Rodríguez-Román A., Hernández-Pech X., Thome E P., Enríquez S., Iglesias-Prieto R. (2006). Photosynthesis and light utilization in the Caribbean coral Montastraea faveolata recovering from a bleaching event. Limnol. Oceanogr. 51 (6), 2702–2710. doi: 10.4319/lo.2006.51.6.2702
Salih A., Larkum A., Cox G., Kühl M., Hoegh-Guldberg O. (2000). Fluorescent pigments in corals are photoprotective. Nature 408 (6814), 850–853. doi: 10.1038/35048564
Scheufen T., Krämer W. E., Iglesias-Prieto R., Enríquez S. (2017a). Seasonal variation modulates coral sensibility to heat-stress and explains annual changes in coral productivity. Sci. Rep. 7 (1). doi: 10.1038/s41598-017-04927-8
Scheufen T., Iglesias-Prieto R., Enríquez S. (2017b). Changes in the number of symbionts and Symbiodinium cell pigmentation modulate differentially coral light absorption and photosynthetic performance. Front. Mar. Sci. 4. doi: 10.3389/fmars.2017.00309
Schlichter D., Meier U., Fricke H. W. (1994). Improvement of photosynthesis in zooxanthellate corals by autofluorescent chromatophores. Oecologia 99 (1/2), 124–131. doi: 10.1007/BF00317092
Smith E. G., D’Angelo C., Salih A., Wiedenmann J. (2013). Screening by coral green fluorescent protein (GFP)-like chromoproteins supports a role in photoprotection of zooxanthellae. Coral Reefs 32 (12), 463-474. doi: 10.1007/s00338-012-0994-9
Swain T. D., DuBois E., Gomes A., Stoyneva V. P., Radosevich A. J., Henss J., et al. (2016). Skeletal light-scattering accelerates bleaching response in reef-building corals. BMC Ecol. 16 (1). doi: 10.1186/s12898-016-0061-4
Terán E., Méndez E. R., Enríquez S., Iglesias-Prieto R. (2010). Multiple light scattering and absorption in reef-building corals. Appl. Optics 49 (27), 5032. doi: 10.1364/AO.49.005032
Vásquez-Elizondo R. M., Legaria-Moreno L., Pérez-Castro M. A., Krämer W. E., Scheufen T., Iglesias-Prieto R., et al. (2017). Absorptance determinations on multicellular tissues. Photosyn. Res. 132 (3), 311–324. doi: 10.1007/s11120-017-0395-6
Wangpraseurt D., Larkum A. W. D., Franklin Szabo J. M., Ralph P. J., Kühl M. (2014). Lateral light transfer ensures efficient resource distribution in symbiont-bearing corals. J. Exp. Biol. 217 (4), 489–498. doi: 10.1242/jeb.091116
Wangpraseurt D., Jacques S. L., Petrie T., Kühl. M. (2016). Monte Carlo Modeling of photon propagation reveals highly scattering coral tissue. Front. Plant Sci. 7. doi: 10.3389/fpls.2016.01404
Wangpraseurt D., Holm J. B., Larkum A. W. D., Pernice M., Ralph P. J., Sugget D. J., et al. (2017). In vivo microscale measurements of light and photosynthesis during coral bleaching: Evidence for the optical feedback loop? Front. Microbiol. 8. doi: 10.3389/fmicb.2017.00059
Keywords: coral bleaching, coral recovery, animal chromoproteins, light environment in hospite, a*CP
Citation: Galindo-Martínez CT, Chaparro A, Enríquez S and Iglesias-Prieto R (2022) Modulation of the symbionts light environment in hospite in scleractinian corals. Front. Mar. Sci. 9:1029201. doi: 10.3389/fmars.2022.1029201
Received: 26 August 2022; Accepted: 05 December 2022;
Published: 22 December 2022.
Edited by:
Eric J. Armstrong, Université de Perpignan Via Domitia, FranceReviewed by:
Dakota McCoy, Stanford University, United StatesNetanel Kramer, Tel Aviv University, Israel
Copyright © 2022 Galindo-Martínez, Chaparro, Enríquez and Iglesias-Prieto. This is an open-access article distributed under the terms of the Creative Commons Attribution License (CC BY). The use, distribution or reproduction in other forums is permitted, provided the original author(s) and the copyright owner(s) are credited and that the original publication in this journal is cited, in accordance with accepted academic practice. No use, distribution or reproduction is permitted which does not comply with these terms.
*Correspondence: Claudia Tatiana Galindo-Martínez, Y2dhbGluZG9tYXJ0aW5lekB1Y3NkLmVkdQ==; Roberto Iglesias-Prieto, cnppM0Bwc3UuZWR1
†Present address: Claudia Tatiana Galindo-Martínez,Scripps Institution of Oceanography, University of San Diego, La Jolla, CA, United States