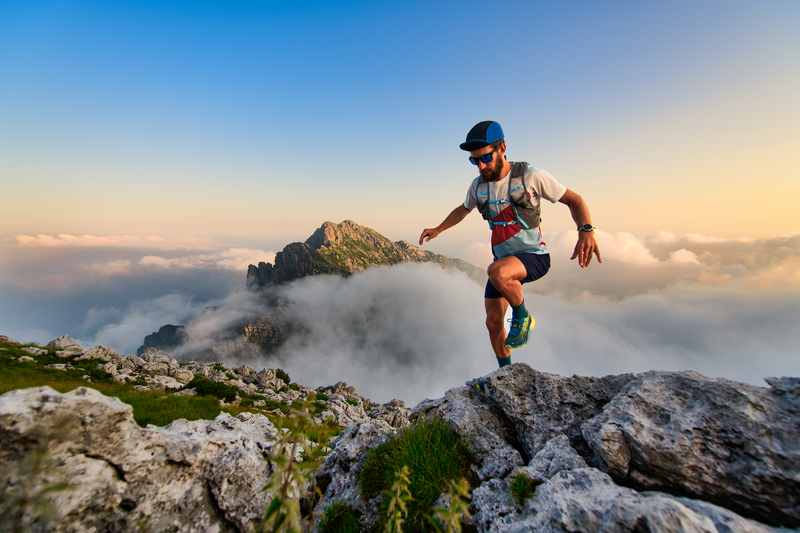
95% of researchers rate our articles as excellent or good
Learn more about the work of our research integrity team to safeguard the quality of each article we publish.
Find out more
ORIGINAL RESEARCH article
Front. Mar. Sci. , 17 October 2022
Sec. Marine Ecosystem Ecology
Volume 9 - 2022 | https://doi.org/10.3389/fmars.2022.1026736
This article is part of the Research Topic Degradation, Ecological Restoration and Adaptive Management of Estuarine Wetlands under Intensifying Global Changes View all 20 articles
Human activities have profoundly affected coastal wetlands. Apart from direct occupation, indirect influences are usually caused by modifications to environmental conditions, which are various and complex. Saltmarsh bare patches might be related to these artificial modifications. They have little or no vegetation cover and lose some important functions. Hence, the mechanisms underlying saltmarsh bare patches and the effects of artificial modifications should be studied. We took the Yellow River Delta as our research object and explored the characteristics of saltmarsh bare patches in the Yellow River Estuary Natural Reserve. Our results show that artificial modifications limit some key plant life stages and thus hinder the natural regeneration process. Once suffering from fatal events, such as long-term inundation or artificial damage, this limitation will lead to the formation of persistent bare patches in saltmarshes. First, a shortage of local seed sources will be induced by the death of local plants when they are affected by a fatal event. Second, the replenishment of external seeds is often constrained by dikes and dams. Third, construction activities and the prolonged high-water inundation events can lead to soil compaction and the lack of microtopographic heterogeneity, which will hinder the retention and anchorage of seeds. Overall, it is essential to realize the underlying mechanisms of persistent bare patches and their potential to be included in cost-effective restoration or management plans.
Coastal wetlands lie in the transition zone between the land and the sea. They defend us from storms and flooding (Barbier et al., 2011; Leonardi et al., 2016; Perillo et al., 2018), and provide important ecosystem services, such as carbon capture (Mcleod et al., 2011) and biodiversity maintenance (Worm et al., 2006; Ramus et al., 2017). However, coastal wetlands are also vulnerable, as they are always in hydrodynamic flux due to the interactions among tides, waves, runoff, and sediment. As such, they are one of the most physically disturbed ecosystems on Earth (Tessler et al., 2015; Perillo et al., 2018). In addition, human activities and climate change have placed coastal wetlands under various, complex stresses across the globe (Gedan et al., 2009; Fagherazzi et al., 2012; Kirwan and Megonigal, 2013; Schuerch et al., 2018; FitzGerald and Hughes, 2019). Between 1999 and 2019, the net global tidal wetland area decreased by 4,000 km2 and about 74.1% of the area is in Asia, including 20.6% in China (Murray et al., 2022). Tidal marshes have similarly been severely degraded by human activities (Murray et al., 2022). In China, approximately 59% of coastal saltmarshes were lost between 1980 and the 2010s due to land reclamation (Gu et al., 2018).
The profound impact of human activities not only lies in the direct occupation of coastal wetlands (He and Silliman, 2019; Newton et al., 2020), but is also due to human modification of the environment (Kirwan and Megonigal, 2013; Auerbach et al., 2015; Tessler et al., 2015). Apart from direct occupation, the environment has also been modified by the construction of dikes and roads, which cut down the tidal flow interaction between the enclosed area and the open marsh, and shift seed flows and hydrological and sediment fluxes at the local scale (Syvitski et al., 2009; Jones et al., 2017; Xie et al., 2017; Van Coppenolle and Temmerman, 2020). This modification might result in increased inundation, sediment deposition, and hydrodynamic forces in front of the dikes. There may also be reduced seed, water, sediment, and nutrient flows behind the dikes. Thus, most of the natural ecological process will be affected, especially plant life cycles (Xie et al., 2017; Wang et al., 2018). This might be destructive to saltmarsh plants and could generate bare patches.
Fatal events, such as long-term inundation or construction activity (eg., dike or road construction), commonly affect saltmarsh plants and they may occur randomly. Inundation is the main stressor to saltmarsh plants and is thought to regulate plant patterns. It can form anoxic low redox potentials and a reductive ion toxic environment (Crooks, 1998; Crooks et al., 2002; Blackwell et al., 2004). Saltmarsh plants adapt to inundation, but can die when the inundation duration is too long or the water depth is too high (Davy et al., 2011; Mossman et al., 2012). Thus, a low-elevation or poor-drainage area is more likely to suffer fatal inundation stress (Crooks et al., 2002; Davy et al., 2011; Krauss et al., 2018; Lagomasino et al., 2021). The construction of dikes and dams can make the drainage conditions in some places worse and increase local plant vulnerability to inundation. Furthermore, temporary artificial occupation or utilization will also cause the death of local plants. It means that, without the help of restoration measures, areas affected by fatal events will remain bare for a long time.
In ecosystems, natural regeneration is an essential process (Figure 1A). Usually, vegetation is highly self-organized and can recover from slight disturbances. This is important to an ecosystem because it maintains its stability. Therefore, a randomly occurring fatal event might not always produce persistent bare patches. If the regeneration process is not hindered, then the bare area often recovers naturally under suitable conditions (Figure 1B). In conclusion, a persistent bare state does not depend on the initial cause of plant death, but on the loss of natural regeneration capacity (Figure 1C). This loss is mainly caused by the blocking of some key stages during the plant life cycle, such as the hindering of seed dispersal (Wolters et al., 2005; Dausse et al., 2008; Xie et al., 2017; Wang et al., 2018) or reduced emergence and growth caused by stressors (i.e., inundation or drought, sediment, and hydrodynamic forces) (Ivajnšič and Kaligarič, 2014; Xie et al., 2017; Wang et al., 2019). Therefore, it is difficult to identify the drivers and mechanisms underlying the creation of persistent bare patches. They might look similar in appearance, but the underlying mechanism might be different.
Figure 1 Modes of disturbance during the natural regeneration process for annual plants. (A) Natural regeneration process: a photograph of a natural saltmarsh. (B) Vegetation that has suffered from disturbance, but can still regenerate: a photograph of naturally recovering areas. (C) Vegetation that has suffered from disturbance and where the natural regeneration process has been blocked. The photograph shows a degraded area that remained bare over a number of years.
These persistent saltmarsh bare patches have lost many functions, such as acting as primary production, habitats for species, carbon storage, and buffering capacity (Gedan et al., 2009; Barbier et al., 2011). They look like “deserts” on the marsh and are disconnected from the existing vegetation. These bare patches reduce the connectivity of the whole coastal marsh and weaken some positive feedbacks (i.e. the positive relations between plants and soil, and seed yield and vegetation patterns) in the ecosystem (Taylor et al., 2015). They also increase the risk of wetland fragmentation and vegetation degradation. However, persistent bare patches could be potential restoration areas that can be used to offset the loss of other saltmarsh areas. They are cost-effective restoration areas compared to other beneficial reclamation areas. Because they were abandoned area with no economic benefit. Understanding their driving forces and mechanisms means that a targeted adjustment plan to restore the regeneration process can be formulated.
In China, the coastal saltmarsh is mainly distributed in the north, and the Yellow River Delta (YRD) is the largest and most complete coastal wetland ecosystem in China. In the YRD, some bare patches are formed by the degradation of a widely distributed native species, Suaeda salsa (L.) Pall., in the protected areas, which has caught the attention of the government and public. To promote the design of a cost-effective restoration project, the driving mechanisms and the restrictions underlying persistent bare patches must be identified. Therefore, we investigated bare patches in the YRD and explored the underlying restrictions and their relationships with artificial modifications. We also explored the multiple effects of artificial modifications (mainly dike construction) on the natural regeneration process, including seed dispersal and establishment in the surrounding areas. The main hypothesis is that key factors and plant life cycle stages are artificially modified, which hinders the natural regeneration process and reduces self-recovery capacity.
The YRD (37.64°–38.15°N, 118.62°–119.37°E) is located in Shandong Province, eastern China (Figure 2A), which is the youngest and most dynamic coastal wetland in the warm temperate zone. It experiences an irregular semidiurnal tide with an average spring tidal range of 1.06–1.78 m and an average neap tidal range of 0.46–0.78 m, (Zhao and Song, 1995). The average annual temperature is 12.8°C and average annual precipitation is 537.3 mm (Xie et al., 2019). Large-scale reclamation activities, such as oil fields, aquaculture ponds, salt fields, farmland, and port construction, have occupied most of the natural coastlines, resulting in relatively complete, natural coastal wetlands being restricted to both sides of the present Yellow River estuary in the Yellow River Estuary Nature Reserve and the old estuary in Yiqian’er Nature Reserve (Figure 2A).
Figure 2 Location of the study area. (A) Boundaries of the nature reserve. (B) Three types bare patches investigated in this study. BP1, BP2, and BP3 are complete-enclosed bare patch, semi-enclosed bare patch, and open bare patch, respectively.
Vegetation zonation is obvious along the elevation gradients in the estuary (He et al., 2011). The annual S. salsa has the widest distribution range, it covers the low tide zone to the supratidal zone (He, 2012), and is almost the only species in the low and middle tidal zones. It is a succulent euhalophytic herb (Figure 3A) that is highly saline tolerant (He, 2012), and its seeds are characterized by having a low mass and a waxy seed coat (Li et al., 2005). These characteristics enable the plant to be easily dispersed throughout the salt marshes by the tide. After seed maturation between October and December, they fall to the ground and are picked up by the tide, which then disperses them across the mudflats and saltmarshes. From the rear of the mid-tide zone to the supratidal zone, S. salsa coexists with Tamarix chinensis and Phragmites australis and its dominance gradually becomes weaker.
The bare patches are mainly located in the middle to high marshes in the northern part of the Yellow River Estuary Natural Reserve, which contains a number of dikes. They are areas with a vegetation coverage of less than 5%, they have existed more than 5 years, and have an area exceeding 100 m2 (Figures 3B–D). We explored the causes of bare patches in the north bank saltmarshes of the Yellow River Estuary. They can be divided into three types according to the barrier effect of dikes and dams (see, e.g., Xie et al., 2017). 1) The complete-enclosed bare patch is where the patch is completely surrounded by dikes and dams and its tidal activity is blocked. General tides do not supply the area. It is only supplied by extreme high-water tidal events, such as astronomical tides and storm surges. 2) The semi-enclosed bare patch is surrounded by dams with gaps, which means that tidal waters can move through the gaps to the bare patches. 3) The open bare patch, which is not blocked by dams.
We selected three bare patches of each type to conduct the study. These were named BP1, BP2, and BP3 (Figure 2B). BP1 was a complete-enclosed bare patch behind the main dike and BP2 was a semi-enclosed bare patch. There was a gap in the surrounding dikes which allowed storm surge water to break through. BP3 was an open bare patch ahead of the main dike. It was once occupied by aquaculture ponds, but was destroyed by storm surges. All the patches were located in the middle to high marsh with elevations ranging from 0.91 to 1.28 m (field investigation data from a GPS device).
Tidal inundation is an important driver of plant establishment and its pattern (Wang et al., 2019). In order to understand the tidal inundation process on the saltmarsh and to identify its impacts on bare patches, an Odyssey Water Level Logger (Z412, Christchurch, New Zealand) was used to monitor tidal inundation on the saltmarsh for one year to reveal the inundation characteristics. We placed a water level logger in the lower part of the marsh near the tidal creeks in an open bare patch at an elevation of 0.75 m. It was set to record at an interval of 10 minutes and the data was retrieved every two months. The retrieved signal was converted into water depth using a calibration curve (the linear relationship between the signal and the water depth). Thus, the water level was obtained by adding the elevation.
Besides environmental stressors, seed dispersal is another essential natural regeneration process. A seed capture experiment was conducted to verify the effect of the dikes on seed dispersal in bare patches. Eighteen seed capture devices were randomly set up in each type of bare patch before seed dispersal in October, 2017 (Figure 2B). A 20 cm long plastic bottle with an inner diameter of 3 cm was selected as the seed trap and a drainage hole (with an aperture of 1 mm) was set in the lower half of the bottle to prevent water accumulation in the trap. The seed trap was buried in the soil with its top flush with the surface. The next April, seeds or seedlings from the seed traps were collected and brought back to the laboratory to determine the number of captured seeds. The germinated seeds (seedlings) were counted directly while the seeds buried in the sediment were counted using a germination method (TerHeerdt et al., 1996). The total number is the sum of the two.
Seed emergence and establishment follows seed dispersal during the natural regeneration process. Therefore, we measured surface soil hardness, water content, bulk density, and salinity to test whether artificially influenced soil conditions inhibited seed establishment, Soil hardness and bulk density are used to measure soil compactness, which affects seed anchorage (Johnson and Fryer, 1992; Brooks et al., 2015). Soil water content and salinity are the main factors affecting the emergence and growth of plants (Long and Mason, 1983; Adam, 1990). The soil conditions in a natural saltmarsh area were used as the control. A total of 30 sites were randomly selected from each of the three bare patch types and natural saltmarsh (N = 120) to measure soil surface hardness with a sclerometer (TYD-1, Zhejiang, China). Soil samples were taken to the laboratory to measure soil salinity, water content, and bulk density.
To verify the effect of soil compaction on seed establishment, two types of surface soil treatment experiments were set up in the three types of bare patches in April (seed emergence stage) (Figure 2B). 1) Six 50×50 cm2 quadrats at a depth of 5 cm were excavated and the surface soil removed. Then, 100 S. salsa seeds were evenly sown inside (three replicates, N = 18). 2) Six 50×50 cm2 quadrats were set up after the surface soil had been plowed and 100 S. salsa seeds were evenly sown (three replicates, N = 18). It is often difficult to retain the S. salsa seeds on the soil surface so we set up two control treatments for seed retention. The first was a caged treatment consisting of six 50×50 cm2 bare surface quadrats containing 100 evenly sown S. salsa seeds (three replicates, N = 18). Then they were caged by a thin wire mesh and covered with a layer of yarn mesh with a pore size of less than 1 mm to prevent seed loss. The second was a blank treatment. Six 50×50 cm2 bare surface quadrats were set, which contained 100 seeds of S. salsa evenly sown inside (three replicates, N = 18). The first control treatment ensured the retention of seeds in the quadrat, while the second did not. The soil hardness of the two surface soil treatments and the control was measured with a sclerometer. Soil surface hardness in an untreated bare area was used as the control. One month later (in May), the S. salsa seedling numbers in the different quadrats were counted.
Fortran 6.5 (Compaq, Houston, USA) was used to statistically evaluate the water level data to analyze the tidal inundation process. We computed the daily highest, monthly mean highest, daily lowest, and monthly mean lowest water levels. Daily inundation duration and monthly mean inundation duration were also calculated. We also analyzed the relationship between the inundation duration and the highest water level of each tidal event using a linear fitting and counted the frequency of tidal events with different water levels.
To identify the effects of artificial modifications on the regeneration process, a one-way ANOVA and Tukey’s HSD comparisons were used to compare the differences among 1) the number of captured seeds in the three types of bare patch; 2) the soil characteristics (hardness, bulk density, salinity and water content) of the three types of bare patches and natural saltmarsh; 3) the soil hardness of the surface soil removal plots, the plowed plots and the control treatment; and 4) seed emergence rates after surface soil removal, plowing and the control treatments in each type of bare patch. Before ANOVA, data were assessed by the Kolmogorov–Smirnov test (α = 0.05). Data sets were natural-log transformed when necessary to meet assumptions of normality and homogeneity of variance. SPSS 22 software package (IBM, New York, USA) was used for the statistical analyses.
According to the tidal flooding process recorded by the water level logger (Figure 4A), several storm surges occurred during October to November, resulting in high water levels and a prolonged inundation period, with the highest water level exceeding 2 m. Tidal inundation occurred more frequently from June to October, but from December to the next May, both the frequency and daily inundation duration decreased (Figures 4A, B). The monthly mean highest water level decreased from November, reached a minimum in December, January, and April, and then gradually increased from May. The monthly mean lowest water level showed a similar trend.
Figure 4 Tidal inundation characteristics analysis. (A, B) Water level and daily inundation duration, respectively. (C) Relationship between the highest water level and inundation duration during a tidal event. (D) Frequency of tidal events with different highest water levels.
The analysis of each tidal event (Figure 4C) showed that the higher water levels always coincided with the longest inundation duration. However, low-water tidal events tended to have a short inundation duration on the marsh. The frequency analysis (Figure 4D) shows that the frequency of high-water tidal events was very low, but tidal events with water levels below 0.9 m were frequent at up to 66.30%. The frequency of tidal events with water levels between 0.9 m and 1.2 m was 27.35% and the frequency of tidal events with water levels higher than 1.2 m was just 5.35%.
The seed dispersal monitoring results showed that seed traps in the complete-enclosed bare patch area (BP1) did not contain any seeds, while the seed traps in the semi-enclosed bare patch areas (BP2) and open bare patches (BP3) contained 6 ± 2 (N = 18) and 25 ± 11 (N = 18) S. salsa seeds, respectively (Figure 5). The number of seeds in the semi-enclosure bare patch seed traps was significantly lower than that in the open bare patch seed traps (F = 35.504, P = 0.000). Therefore, the dikes do prevent the tides from carrying seeds and the semi-enclosed bare patch was partially affected by the dike, which reduced the number of seeds entering the patch.
Figure 5 Number of trapped seeds in the three types of bare patch and natural saltmarsh. BP1, BP2, and BP3 represent complete-enclosed, semi-enclosed and open bare patches, respectively (N = 54). Data are means ± SE. Significant differences are shown by different letters above the bars.
The soil investigation showed that the surface soil hardness of the bare patches was significantly higher than that of the natural saltmarsh (Figure 6A). The surface soil hardness in the bare patches was above 23 mm, while hardness in the natural saltmarsh was mostly below 20 mm. The soil bulk density of BP1 was significantly higher than that of the BP2 and BP3 bare patches, but the latter two were not significantly different from that of natural saltmarsh (Figure 6B). The dike barrier meant that soil salinity in BP1 was significantly lower than that of the other bare patches and the natural saltmarsh (Figure 6C). In addition, the salinity of the natural saltmarsh was highest, but there was no significant difference between it and BP3. Soil water content was lowest in BP1 and significantly different from those of BP2 and BP3 (Figure 6D). Soil water content in the natural saltmarsh was significantly higher than all the bare patches (Figure 6D).
Figure 6 (A) Soil hardness, (B) bulk density, (C) salinity, and (D) water content of the three types of bare patch and natural saltmarsh. BP1, BP2, and BP3 represent complete-enclosed, semi-enclosed and open bare patches, respectively (N = 120). Data are means ± SE. Significant differences are shown by different letters above the bars.
Surface soil removal and plowing can significantly reduce compaction (Figure 7A) and the patches that had received these treatments approached the level of natural saltmarsh. The sowing experiment showed that excluding hard, smooth, and flat soil surfaces enhanced S. salsa seed duration and emergence (Figures 7B–D). In contrast, there was no seed emergence in the control patches without surface soil treatment (Figures 7B, E, F). The emergence rate with surface soil removal treatment was higher than that of the plowed treatment, and the results for the removal treatment were significantly higher than those for the plowed treatment in BP2 and BP3, whereas there was no significant difference between the two surface soil treatments in BP1.
Figure 7 Soil hardness and the seed emergence rates after surface soil removal and plowing. (A) Soil hardness after the two treatments. Each group is a combination of the treatments for each of the three types bare patch (N = 54 with 18 controls from three bare patches). (B) Results of seed addition when the two treatments were applied (N = 72 with 32 controls). (C, D) Photographs of emerged S. salsa seedlings after the removal and plowing treatments, respectively. (E, F) Photographs of the caged and blank control treatments showing no emerged seedlings, respectively. BP1, BP2, and BP3 represent complete-enclosed, semi-enclosed, and open bare patches, respectively. Data are means ± SE and significant differences are shown by different letters above the bars.
The global loss of coastal wetlands along with climate change mean that there is an increased risk of coastal flooding from more severe storm surges and expected sea level rises (Kirwan and Megonigal, 2013; Enwright et al., 2016; Kirwan and Gedan, 2019; Theuerkauf and Braun, 2021). Therefore, restoring saltmarshes will significantly contribute to nature-based mitigation of coastal flood risks because they can attenuate storm surges, reduce the impact of waves and shoreline erosion, and accumulate sediments in balance with sea level rises (Lovelock et al., 2015; Sandi et al., 2018). Bare patches are important potential units to be recovered. However, the underlying reasons for bare patches may vary. Therefore, we should pay attention to persistent bare patches that have a low regeneration potential and are mainly caused by artificial modifications. Identifying persistent bare patches and the reasons for their existence will improve our ability to formulate cost-effective restoration and management plans.
Many studies have shown that the occurrence of bare patches in coastal wetlands is determined by elevation and oxidative reducibility (Davy et al., 2011; Mossman et al., 2012). Prolonged flooding results in an anoxic, low redox potential, and reductive ion toxic environment (Crooks, 1998; Crooks et al., 2002; Blackwell et al., 2004), which is stressful to saltmarsh plants, such as S. salsa. These types of stressors, which are caused by flooded environments, have been confirmed by many studies, and flooding stress is mainly caused by high frequency tidal events and poor drainage conditions (Crooks et al., 2002; Davy et al., 2011). This may be an important reason for the death of saltmarsh vegetation.
The periodic or intermittent influence of tides means that saltmarsh vegetation is in a dynamic environment that undergoes dramatic hydrological changes and is significantly affected by the hydrological environment (Adam, 1990). Tidal inundation can affect saltmarsh plant growth by altering soil permeability and its chemical characteristics (Adam, 1990; Colmer and Flowers, 2008). Specifically, the various characteristics of the tidal inundation process, such as tidal frequency, tidal range, and inundation duration, may have different effects on species and are correlated with the distribution and richness of saltmarsh plants (Casanova and Brock, 2000; He, 2012). The effects of the depth and duration of an inundation event depend on the plant height relative to the average sea level (Armstrong et al., 1985; Silvestri et al., 2005; Colmer and Flowers, 2008). During high tides, especially spring tides (with a mean level of 1.27m, see Wang et al., 2018), saltmarsh plants are often partially or completely submerged for several hours or even a few days.
According to our analysis of the effects of tidal inundation characteristics on the saltmarsh surface, high-water tidal events tend to last for a long time, resulting in continuous inundation of the saltmarsh surface, usually for more than one day. When experiencing storm surges, such as the tidal event from October 20 to 26, 2017, the highest water levels can exceed 2 m, the inundated area reaches the supratidal zone, and the whole intertidal zone can be inundated for several consecutive days. Although the duration of a storm surge is not very long, the large-scale and high-water level flooding process leads to a very slow receding process, which may take several days. Furthermore, if the area has poor drainage conditions, such as those caused by dikes, the inundation time will be prolonged. When the saltmarsh within the embankment is flooded by a high tide, the drainage rate is slower than that of natural wetland because the latter has microtopographic heterogeneity and natural tidal creek systems. Thus, the hypoxia stress caused by inundation will more serious in poorly drained areas, especially those that have been blocked by dikes.
Long-duration and high-water inundations may mean that the tolerance limits of plants such as S. salsa are exceeded. This leads to the oxygen consumed in saltmarsh soil not being replenished, resulting in soil hypoxia (Adam, 1990; Dat et al., 2006). In addition to the anoxic soil environment, long-duration flooding also leads to the enrichment of other compounds and ions in the soil that affect plant growth, including carbon dioxide, ethylene and S2– (Brinkman and Van Diepen, 1990; Keddy, 2001; Colmer and Flowers, 2008; He, 2012). Tidal inundation also prevents photosynthesis in the above-ground parts of plants because it decreases carbon dioxide and light levels, which further reduces the content and dispersal of oxygen in plants (Colmer and Flowers, 2008). Although these high-water and long-term inundation events are infrequent, their effect on saltmarsh plants cannot be ignored. Highly frequent low-water tidal events tend to have small inundation depths and shorter durations, resulting in smaller inundated areas and a reduced influence on plant growth.
Tidal flows are the main medium for seed dispersal on saltmarshes (2008; Chang et al., 2007; Friess et al., 2012). Therefore, hydrological connectivity conditions (i.e., whether impeded by dikes) have important effects on seed dispersal (Xie et al., 2019) and can have decisive effects on vegetation patterns. Where seeds or propagules finally stay and establish is a result of the multiple and complex interactions between tidal flows and microtopography or vegetation (Chang et al., 2007; Peterson and Bell, 2012). However, dikes block the movement of tidal flows, including carried seeds or propagules. Our monitoring results showed that there was no input of S. salsa seeds from the surrounding saltmarshes to the complete-enclosed bare patches. The input of S. salsa seeds from the surrounding saltmarshes was also limited in the semi-enclosed bare patches.
The initial death of saltmarsh plants within an enclosed patch causes a shortage of local seeds produced by local plants. Saltmarshes are particularly dependent on the dispersed seeds or propagules from surrounding saltmarshes carried by tidal waters. Thus, the dikes will contribute both to prolonged inundation conditions, which are fatal to local plants, and a decrease in the external seeds/propagules needed to replenish the enclosed area. They constrain the seed inputs needed for the regeneration of enclosed bare patches.
Previous studies have shown that defensive facilities (i.e., dikes and dams) have ecologically negative effects on the life cycles of halophytes, including seed/propagule dispersal, and seedling emergence and survival (Friess et al., 2012). Reclamation activities have led to the shrinkage and compaction of surrounding saltmarsh soils (Crooks et al., 2002; Pethick, 2002) and have changed soil conditions, including salinity, water content, and sediment thickness (Bouma et al., 2009; Xie et al., 2017). In addition, these construction activities also change the surface microtopography. Compared to natural saltmarshes, the soil surfaces of these disturbed and compacted saltmarshes lack topographic heterogeneity, which may have a negative impact on colonization by saltmarsh plants (Brooks et al., 2015; Wang et al., 2018). Besides the direct influence of construction activities, soil shrinkage and compaction may also occur under natural conditions, especially in high saltmarshes (Cahoon et al., 2011; Brooks et al., 2015). High saltmarshes or reconstructed areas have a higher starting shear stresses, and lower soil moisture and organic matter contents (Brooks et al., 2015). Therefore, water availability in the soil is low, which makes it difficult for the vegetation to anchor (Brooks et al., 2015). The high starting shear stress of soil may be caused by the anti-flocculation of clayey minerals when soil is exposed to air under low salt conditions, which damages soil structures (Crooks, 1998; Crooks et al., 2002). This in turn leads to soil compaction and it is a common phenomenon in many saltmarshes when the soil is submerged by tidal waters (Crooks, 1998; Crooks et al., 2002). These compacted soil surfaces inhibit the infiltration of new sediments, lead to the underlying soil becoming hypoxic (Crooks et al., 2002), and affect some ecological processes above the ground (Evans et al., 1998). Once saltmarshes are inundated by tidal waters, the large-scale treatment of compacted soil to make it suitable for saltmarsh plant colonization is very challenging (Brooks et al., 2015).
Compared to natural saltmarsh soils, the bare patches (both open and enclosed) were compacted and their hardness was significantly higher than that of natural saltmarsh soil. The soil bulk densities in BP2 and BP3 were close to the natural saltmarsh, which indicates that the soil physical structure of bare patches connected to the natural saltmarsh (the semi-enclosed and open ones) was better than that of the completely blocked bare patches. The dikes, to some extent, block the tidal flows, which leads to decreases in salinity and water content. A lower salinity is beneficial to the emergence and growth of S. salsa, which has an optimum growth and emergence salinity of 12.71 ppt and below 10 ppt, respectively (He, 2012; Xie et al., 2017). In contrast, a lower water content is not beneficial to S. salsa. This compromise between soil salinity and water content means that a constrained saltmarsh is not as effective as natural saltmarsh, but it does not reduce the emergence and growth of S. salsa.
The compacted soil means that it is hard for the seeds to anchor themselves and the lack of topographic heterogeneity means that seeds often do not remain in the patch (Clark et al., 2007; Nilsson et al., 2010; Wang et al., 2018). This was demonstrated by the soil surface and seed retention treatments. The control treatment without a cage showed that S. salsa seeds did not remain on the surface of the bare patch because they are easily carried away by wind or tides on a smooth flat hard surface. However, in the same situation and under the influence of wind or tides, the surface soil removal or plowed treatments allowed the S. salsa seeds to stay and anchor (Figures 6C, D). These treatments decreased soil compaction, but increased roughness and topographic heterogeneity on the surface. Smooth surfaces without topographic heterogeneity easily form after compaction, which means that seed retention is poor (Brooks et al., 2015). Another treatment was to set up a cage to prevent S. salsa seeds from escaping and retain the seeds in the quadrat, but there were still no seedlings. This suggests that even the seeds that were present in these bare patches did not have suitable conditions for emergence. They could not obtain moisture and nutrients from the soil to sustain their emergence (Evans et al., 1998). However, on the surface of natural saltmarshes, topographic heterogeneity and plant structures provide sufficient opportunities for seeds to stay. In addition, due to the presence of plants in natural saltmarshes, feedback between plants and soil, such as shading, reduced evaporation, and improved water retention, provides more favorable establishment conditions for retained seeds (Brooks et al., 2015). Therefore, hardened surfaces hinder the establishment of plants, seed retention, and the ability of seeds to anchor themselves.
Although both the soil removal and plowing treatments removed the effects of hard soil surfaces on seed establishment, their effects varied. This difference did not occur in the BP1 patch, but occurred in the BP2 and BP3 patches where the interaction with tidal waters was not cut off. The results showed that stability in the plowed BP3 patch was poor, that is, it was not easy to maintain the surface treatment in the tidal environment. Tidal flows meant that these surface treatments do not always last very long, meaning that over time they tend to disappear and become less effective. The stability of these soil surface treatments is related to the saltmarsh environment. In areas that are more susceptible to tides, soil surface treatment is less easy to maintain and less stable. In the BP1 patch, the reduced tidal influence meant that both the surface soil removal and surface soil plowed treatments could be maintained, providing enough time for the remaining seeds to emerge. Semi-enclosed and open bare patches are more susceptible to tidal effects, leading to the development of smooth surfaces. The results for the two types of soil treatment showed that the topsoil removal treatment was more resistant to tidal flows and maintained a rough surface better than the plowed treatment. Under the influence of tides, the stability of the topsoil plowing treatment was not good, which shortened the time that suitable emergence conditions existed and reduced overall emergence. However, the topsoil removal treatment produced surfaces that were more stable and offered greater chances for seed emergence. Therefore, when the bare patch surface soil becomes hard, removal of the soil layer can improve the replanting of S. salsa. However, the effect of tides on surface soil treatments should be further considered because treatments that produce higher soil stabilities are more conducive to seed emergence.
The original contributions presented in the study are included in the article/supplementary material. Further inquiries can be directed to the corresponding author.
QW, BC and AW designed the study and contributed to the writing of the manuscript. DS, ZR, ZN, and FG were involved in the field work and data collection. QW and DS analyzed the data. All authors contributed to the article and approved the submitted version.
The study was financially supported by the National Key R&D Program of China (2019YFE0121500), the Youth Program of the Natural Science Foundation of China (42107057), and the Key Project of the National Natural Science Foundation of China (U2243208, U1901212).
We thank Meng Luo, Wei Shi, and Chengjie Xie for their help with the field work and for the help given by the Yellow River Delta National Nature Reserve Administration Bureau. We thank International Science Editing (http://www.internationalscienceediting.com) for editing this manuscript.
The authors declare that the research was conducted in the absence of any commercial or financial relationships that could be construed as a potential conflict of interest.
All claims expressed in this article are solely those of the authors and do not necessarily represent those of their affiliated organizations, or those of the publisher, the editors and the reviewers. Any product that may be evaluated in this article, or claim that may be made by its manufacturer, is not guaranteed or endorsed by the publisher.
Armstrong W., Wright E. J., Lythe S., Gaynard T. J. (1985). Plant zonation and the effects of the springneap tidal cycle on soil aeration in a Humber salt marsh. J. Ecol. 73 (1), 323–339. doi: 10.2307/2259786
Auerbach L. W., Goodbred S. L. Jr., Mondal D. R., Wilson C., Ahmed K. R., Roy K., et al. (2015). Flood risk of natural and embanked landscapes on the Ganges–Brahmaputra tidal delta plain. Nat. Clim. Change 5, 153–157. doi: 10.1038/nclimate2472
Barbier E. B., Hacker S. D., Kennedy C., Koch E. W., Stier A. C., Silliman B. R., et al. (2011). The value of estuarine and coastal ecosystem services. Ecol. Monogr. 81, 169–193. doi: 10.1890/10-1510.1
Blackwell M. S. A., Hogan D. V., Maltby E. (2004). The short-term impact of managed realignment on soil environmental variables and hydrology. Estuar. Coast. Shelf Sci. 59, 687–701. doi: 10.1016/j.ecss.2003.11.012
Bouma T., Friedrichs M., Van Wesenbeeck B. K., Temmerman S., Graf G., Herman P. M.J., et al. (2009). Density-dependent linkage of scale-dependent feedbacks: A flume study on the intertidal macrophyte spartina anglica. Oikos 1182, 260–268. doi: 10.1111/j.1600-0706.2008.16892.x
Brinkman R., Van Diepen C. A. (1990). “Mineral soils,” in Wetlands and shallow continental water bodies. vol. 1. natural and human relationships. Ed. Patten B. C. (Hague, The Netherlands: SPB Academic Publishing), 37–59.
Brooks K. L., Mossman H. L., Chitty J. L., Grant A. (2015). Limited vegetation development on a created salt marsh associated with over-consolidated sediments and lack of topographic heterogeneity. Estuar. Coast. 38, 325–336. doi: 10.1007/s12237-014-9824-3
Cahoon D. R., Perez B. C., Segura B. D., Lynch J. C. (2011). Elevation trends and shrink-swell response of wetland soils to flooding and drying estuarine. Coast. Shelf Sci. 91, 463–474. doi: 10.1016/j.ecss.2010.03.022
Casanova M. T., Brock M. A. (2000). How do depth, duration and frequency of flooding influence the establishment of wetland plant communities? Plant Ecol. 147 (2), 237–250. doi: 10.1023/A:1009875226637
Chang E. R., Veeneklaas R. M., Bakker J. P. (2007). Seed dynamics linked to variability in movement of tidal water. J. Veg. Sci. 18, 253–262. doi: 10.1111/j.1654-1103.2007.tb02536.x
Chang E. R., Veeneklaas R. M., Buitenwerf R., Bakker J. P., Bouma T. J. (2008). To move or not to move: determinants of seed retention in a tidal marsh. Funct. Ecol. 22, 720–727. doi: 10.1111/j.1365-2435.2008.01434.x
Clark C. J., Poulsen J. R., Levey D. J., Osenberg C. W. (2007). Are plant populations seed limited? a critique and meta-analysis of seed addition experiments. Am. Nat. 170, 128–142. doi: 10.1086/518565
Colmer T. D., Flowers T. J. (2008). Flooding tolerance in halophytes. New Phytol. 179 (4), 964–974. doi: 10.1111/j.1469-8137.2008.02483.x
Crooks S. (1998). “A mechanism for the formation of overconsolidated horizons within estuarine floodplain alluvium: implications for the interpretation of Holocene sea-level curves,” in Floodplains: interdisciplinary approaches, special publication- geological society of London. Eds. Marriott S., Alexander J., Hey R.(Norwich, Bath, UK: Geological Society London Special Publications), 197–216.
Crooks S., Schutten J., Sheern G. D., Pye K., Davy A. J. (2002). Drainage and elevation as factors in the restoration of salt marsh in Britain. Restor. Ecol. 10, 591–602. doi: 10.1046/j.1526-100X.2002.t01-1-02036.x
Dat J., Folzer H., Parent C., Badot P. M., Capelli N. (2006). “Hypoxia stress. current understanding and perspectives,” in Floriculture, ornamental and plant biotechnology: Advances and topical issues (Middlesex: UK: Global Science Books, Ltd.), vol. 3. , 664–674.
Dausse A., Bonis A., Bouzillé J. B., Lefeuvre J. C. (2008). Seed dispersal in a polder after partial tidal restoration: Implications for salt-marsh restoration. Appl. Veg. Sci. 11 (1), 3–12. doi: 10.1111/j.1654-109X.2008.tb00199.x
Davy A. J., Brown M. J. H., Mossman H. L., Grant A. (2011). Colonization of a newly developing salt marsh: disentangling independent effects of elevation and redox potential on halophytes. J. Ecol. 99, 1350–1357. doi: 10.1111/j.1365-2745.2011.01870.x
Enwright N. M., Griffith K. T., Osland M. J. (2016). Barriers to and opportunities for landward migration of coastal wetlands with sea-level rise. Front. Ecol. Environ. 14 (6), 307–316. doi: 10.1002/fee.1282
Evans P. R., Ward R. M., Bone M., Leakey M. (1998). Creation of temperate-climate intertidal mudflats: factors affecting colonization and use by benthic invertebrates and their bird predators. Mar. pollut. Bull. 37, 535–545. doi: 10.1016/S0025-326X(98)00140-4
Fagherazzi S., Kirwan M. L., Mudd S. M., Guntenspergen G. R., Temmerman S., D'Alpaos A., et al. (2012). Numerical models of salt marsh evolution: Ecological, geomorphic, and climatic factors. Rev. Geophys. 50 (1), RG1002. doi: 10.1029/2011RG000359
FitzGerald D. M., Hughes Z. (2019). Marsh processes and their response to climate change and Sea-level rise. Annu. Rev. Earth Pl Sc 47, 481–517. doi: 10.1146/annurev-earth-082517-010255
Friess D. A., Krauss K. W., Horstman E. M., Balke T., Bouma T. J., Galli D., et al. (2012). Are all intertidal wetlands naturally created equal? bottlenecks, thresholds and knowledge gaps to mangrove and saltmarsh ecosystems. Biol. Rev. 87, 346–366. doi: 10.1111/j.1469-185X.2011.00198.x
Gedan K. B., Silliman B. R., Bertness M. D. (2009). Centuries of human-driven change in salt marsh ecosystems. Annu. Rev. Mar. Sci. 1, 117–141. doi: 10.1146/annurev.marine.010908.163930
Gu J., Luo M., Zhang X., Christakos G., Agusti S., Duarte C. M., et al. (2018). Losses of salt marsh in China: Trends, threats and management. Estuar. Coast. Shelf Sci. 214, 98–109. doi: 10.1016/j.ecss.2018.09.015
He Q. (2012). Bottom-up, interspecific and top-down determinants of plant communities in salt marshes in the yellow river estuary (Shanghai, China: Shanghai Jiao Tong University).
He Q., Cui B. S., An Y. (2011). The importance of facilitation in the zonation of shrubs along a coastal salinity gradient. J. Veg. Sci. 22, 828–836. doi: 10.1111/j.1654-1103.2011.01300.x
He Q., Silliman B. R. (2019). Climate change, human impacts, and coastal ecosystems in the anthropocene. Curr. Biol. 29, R1021–R1035. doi: 10.1016/j.cub.2019.08.042
Ivajnšič D., Kaligarič M. (2014). How to preserve coastal wetlands, threatened by climate change-driven rises in sea level. Environ. Manage. 54 (4), 671–684. doi: 10.1007/s00267-014-0244-8
Johnson E. A., Fryer G. I. (1992). Physical characterization of seed microsites- movement on the ground. J. Ecol. 80, 823–836. doi: 10.2307/2260869
Jones M. C., Bernhardt C. E., Krauss K. W., Noe G. B. (2017). The impact of late Holocene land use change, climate variability, and sea level rise on carbon storage in tidal freshwater wetlands on the southeastern united states coastal plain. J. Geophys. Res. Biogeosci. 122, 3126–3141. doi: 10.1002/2017JG004015
Keddy P. A. (2001). Wetland Ecology: Principles and Conservation. Cambridge, UK: Cambridge University Press.
Kirwan M. L., Gedan K. B. (2019). Sea-Level driven land conversion and the formation of ghost forests. Nat. Climate Change 9, 450–457. doi: 10.1038/s41558-019-0488-7
Kirwan M., Megonigal J. (2013). Tidal wetland stability in the face of human impacts and sea-level rise. Nature 504, 53–60. doi: 10.1038/nature12856
Krauss K. W., Demopoulos A. W. J., Cormier N., From A. S., McClain-Counts J. P., Lewis R. R. III (2018). Ghost forests of Marco island: Mangrove mortality driven by belowground soil structural shifts during tidal hydrologic alteration. Estuar. Coast. Shelf Sci. 212, 51–62. doi: 10.1016/j.ecss.2018.06.026
Lagomasino D., Fatoyinbo T., Castañeda-Moya. E., Cook B. D., Montesano P. M., Neigh C. S. R., et al. (2021). Storm surge and ponding explain mangrove dieback in southwest Florida following hurricane Irma. Nat. Commun. 12, 4003. doi: 10.1038/s41467-021-24253-y
Leonardi N., Ganju N. K., Fagherazzi S. (2016). A linear relationship between wave power and erosion determines salt-marsh resilience to violent storms and hurricanes. Proc. Natl. Acad. Sci. 113 (1), 64–68. doi: 10.1073/pnas.1510095112
Li W. Q., Liu X. J., Khan M. A., Yamaguchi S. (2005). The effect of plant growth regulators, nitric oxide, nitrite and light on the germination of dimorphic seeds of suaeda salsa under saline conditions. J. Plant Res. 118, 207–214. doi: 10.1007/s10265-005-0212-8
Lovelock C. E., Cahoon D. R., Friess D. A., Guntenspergen G. R., Krauss K. W., Reef R., et al. (2015). The vulnerability of indo-pacific mangrove forests to sea-level rise. Nature 526 (7574), 559–563. doi: 10.1038/nature15538
Mcleod E., Chmura G. L., Bouillon S., Salm R., Björk M., Duarte C. M., et al. (2011). A blueprint for blue carbon: toward an improved understanding of the role of vegetated coastal habitats in sequestering CO2. Front. Ecol. Environ. 9 (10), 552–560. doi: 10.1890/110004
Mossman H. L., Davy A. J., Grant A., Elphick C.. (2012). Does managed coastal realignment create saltmarshes with ‘equivalent biological characteristics’ to natural reference sites? J. Appl. Ecol. 49, 1449–1446. doi: 10.1111/j.1365-2664.2012.02198.x
Murray N. J., Worthington T. A., Bunting P., Duce S., Hagger V., Lovelock C. E., et al. (2022). High-resolution mapping of losses and gains of earth’s tidal wetlands. Science 376 (6594), 744–749. doi: 10.1126/science.abm9583
Newton A., Icely J., Cristina S., Perillo G. M. E., Turner R. E., Ashan D. W., et al. (2020). Anthropogenic, direct pressures on coastal wetlands. Front. Ecol. Evol. 8, 144. doi: 10.3389/fevo.2020.00144
Nilsson C., Brown R. L., Jansson P., Merritt D. M. (2010). The role of hydrochory in structuring riparian and wetland vegetation. Biol. Rev. 85, 837–858. doi: 10.1111/j.1469-185X.2010.00129.x
Perillo G., Wolanski E., Cahoon D. R., Hopkinson C. S. (Eds.) (2018). Coastal wetlands: an integrated ecosystem approach ( New York, USA: Elsevier).
Peterson J. M., Bell S. S. (2012). Tidal events and salt marsh structure influence black mangrove avicennia germinans recruitment across an ecotone. Ecology 937, 1648–1658. doi: 10.1890/11-1430.1
Pethick J. (2002). Estuarine and tidal wetland restoration in the united kingdom: policy versus practice. Restor. Ecol. 10, 431–437. doi: 10.1046/j.1526-100X.2002.01033.x
Ramus A. P., Silliman B. R., Thomsen M. S., Long Z. T. (2017). An invasive foundation species enhances multifunctionality in a coastal ecosystem. Proc. Natl. Acad. Sci. 114 (32), 8580–8585. doi: 10.1073/pnas.1700353114
Sandi S. G., Rodriguez J. F., Saintilan N., Riccardi G., Saco P. M. (2018). Rising tides, rising gates: The complex ecogeomorphic response of coastal wetlands to sea-level rise and human interventions. Adv. Water Resour. 114, 135–148. doi: 10.1016/j.advwatres.2018.02.006
Schuerch M., Spencer T., Temmerman S., Kirwan M. L., Wolff C., Lincke D., et al. (2018). Future response of global coastal wetlands to sea-level rise. Nature 561, 231–234. doi: 10.1038/s41586-018-0476-5
Silvestri S., Defina A., Marani M. (2005). Tidal regime, salinity and salt marsh plant zonation. Estuar. Coast. Shelf Sci. 62 (1-2), 119–130. doi: 10.1016/j.ecss.2004.08.010
Syvitski J. P. M., Kettner A. J., Overeem I., Hutton E. W. H., Hannon M. T., Brakenridge G. R., et al. (2009). Sinking deltas due to human activities. Nat. Geosci. 2, 681–686. doi: 10.1038/ngeo629
Taylor M. S., Jonathan M. W., Mark W. H. (2015). Hydrologic and edaphic constraints on schoenoplectus acutus, schoenoplectus californicus, and typha latifolia in tidal marsh restoration. Restor. Ecol. 23, 430–438. doi: 10.1111/rec.12212
TerHeerdt G. N. J., Verweij G. L., Bekker R. M., Bakker J. P. (1996). An improved method for seed-bank analysis: Seedling emergence after removing the soil by sieving. Funct. Ecol. 10, 144–151. doi: 10.2307/2390273
Tessler Z. D., Vörösmarty C. J., Grossberg M., Gladkova I., Aizenman H., Syvitski J. P., et al. (2015). Profiling risk and sustainability in coastal deltas of the world. Science 349 (6248), 638–643. doi: 10.1126/science.aab3574
Theuerkauf E. J., Braun K. N. (2021). Rapid water level rise drives unprecedented coastal habitat loss along the great lakes of north America. J. Great Lakes Res. 47 (4), 945–954. doi: 10.1016/j.jglr.2021.05.004
Van Coppenolle R., Temmerman S. (2020). Identifying global hotspots where coastal wetland conservation can contribute to nature-based mitigation of coastal flood risks. Global Planet. Change 187, 103125. doi: 10.1016/j.gloplacha.2020.103125
Wang Q., Cui B. S., Luo M. (2018). Effectiveness of microtopographic structure in species recovery in degraded salt marshes. Mar. pollut. Bull. 133, 173–181. doi: 10.1016/j.marpolbul.2018.05.037
Wang Q., Cui B. S., Luo M., Qiu D. D., Shi W., Xie C. J. (2019). Microtopographic structures facilitate plant recruitment across a salt marsh tidal gradient. Aquat. conserv.: Mar. Freshw. ecosyst. 29 (8), 1336–1346. doi: 10.1002/aqc.3120
Wolters M., Garbutt A., Bakker J. P. (2005). Plant colonization after managed realignment: the relative importance of diaspore dispersal. J. Appl. Ecol. 42, 770–777. doi: 10.1111/j.1365-2664.2005.01051.x
Worm B., Barbier E. B., Beaumont N., Duffy J. E., Folke C., Halpern B. S., et al. (2006). Impacts of biodiversity loss on ocean ecosystem services. science 314 (5800), 787–790. doi: 10.1126/science.1132294
Xie T., Cui B., Li S. (2017). Analysing how plants in coastal wetlands respond to varying tidal regimes throughout their life cycles. Mar. pollut. Bull. 123, 113–121. doi: 10.1016/j.marpolbul.2017.09.017
Xie T., Li S., Cui B., Bai J., Wang Q., Shi W. (2019). Rainfall variation shifts habitat suitability for seedling establishment associated with tidal inundation in salt marshes. Ecol. Indic. 98, 694–703. doi: 10.1016/j.ecolind.2018.11.056
Keywords: bare patch, artificial modification, plant regeneration, seed establishment, tidal event, saltmarsh, Yellow River Delta
Citation: Wang Q, Shao D, Cui B, Ren Z, Ning Z, Gao F and Wang A (2022) Artificial modifications lead to the formation of persistent bare patches in saltmarshes. Front. Mar. Sci. 9:1026736. doi: 10.3389/fmars.2022.1026736
Received: 24 August 2022; Accepted: 30 September 2022;
Published: 17 October 2022.
Edited by:
Laibin Huang, University of California, Davis, United StatesCopyright © 2022 Wang, Shao, Cui, Ren, Ning, Gao and Wang. This is an open-access article distributed under the terms of the Creative Commons Attribution License (CC BY). The use, distribution or reproduction in other forums is permitted, provided the original author(s) and the copyright owner(s) are credited and that the original publication in this journal is cited, in accordance with accepted academic practice. No use, distribution or reproduction is permitted which does not comply with these terms.
*Correspondence: Andong Wang, Ymhxa3l6QDEyNi5jb20=
Disclaimer: All claims expressed in this article are solely those of the authors and do not necessarily represent those of their affiliated organizations, or those of the publisher, the editors and the reviewers. Any product that may be evaluated in this article or claim that may be made by its manufacturer is not guaranteed or endorsed by the publisher.
Research integrity at Frontiers
Learn more about the work of our research integrity team to safeguard the quality of each article we publish.