- 1University of Duisburg-Essen, Department of Chemistry, Environmental Microbiology and Biotechnology (EMB) - Aquatic Microbiology, Essen, Germany
- 2Centre for Ecology and Evolution in Microbial Model Systems (EEMiS), Department of Biology and Environmental Science, Linnaeus University, Kalmar, Sweden
Natural and oil surface slicks are widespread phenomena at the air-water interface and represent visible surface films with distinct physicochemical and biological properties compared to non-slick surface films and underlying marine water. Both types of slicks have major functions in nourishing and dispersing surface-dwelling micro- and macroorganisms, contribute to particle generation and carbon cycling, and are known to accumulate pollutants. Despite these functions and the high frequency of slicks in the marine environment, slicks are little understood considering their microbial inhabitants and contributions of these organisms for natural and oil slick establishment, function, and ecology. Our mini review summarizes the current knowledge of microbial life in natural slicks, natural and anthropogenic oil slicks, including the major knowledge gaps and perspectives for future research. Overall, we aim for an increased awareness about the existence of natural slicks, the differentiation between natural and anthropogenic oil slicks and an improved reporting of slick events during sampling of surface film and seawater from the epipelagic zone.
Natural slicks in the marine environment
Most people are aware of oil surface films, so-called oil slicks, as anthropogenic pollution in the marine environment. However, less focus has been granted to natural non-oil surface slicks that establish as “smooth glassy streaks or patches” at the air-sea interface (Dietz and Lafond, 1950). When exploring marine surface slicks, the concept of slicks is not fully separable from investigations of the sea-surface microlayer, which represents a submillimeter film covering the ocean’s surface (reviewed by Cunliffe et al. (2013)). Slicks represent visible films (organic monolayers) (Garrett, 1965), that are much thinner (2.4 - 2.7 nm) than typical microlayer sampling resolution (Hühnerfuss, 2006). Slick formation is often accompanied by different texture and coloring of the affected surface area (Figure 1), which results from increased light reflection and makes these phenomena observable by eye from ships and shore. Natural slicks are generally confined to coastal waters where they can cover the ocean surface to ~80% under calm weather conditions, occur more frequently during mornings and are rarely seen at wind speeds > 6-7 m s-1 (Romano and Marquet, 1991; Romano, 1996). Slicks appear with less frequency in the open sea compared to coastal waters (11 vs. 30%, Romano, 1996) and in proximity to the coast and inshore waters, slicks often contain high organic matter content (Garabetian et al., 1993). In contrast, the microlayer can form at typical oceanic wind speeds > 6 m s-1 (Wurl et al., 2009) and thus exists inside and outside surface slicks. Wurl et al. (2016) proposed the terms slick and non-slick to distinguish between the two types of microlayers. Hunter and Liss (1981) claimed that the presence of slicks is an indicator for a coherent surface film at the sea surface and hence slicks often accumulate along shear currents, like eddies or fronts or in association with Langmuir circulation (Green and Houk, 1979; Marmorino et al., 2002; Gade et al., 2013). Capone et al. (1998) described that natural slicks can cover a surface area of 1300 m2. Slicks can be a warmer, less saline, and a more viscous environment compared to surrounding waters, and they often carry sea weeds like Sargassum sp., particulates including plastics, and foams (Dietz and Lafond, 1950; Carlson, 1987; Gower et al., 2006; Wurl et al., 2016; Wurl et al., 2018; Gallardo et al., 2021). The lifespan of natural slicks typically lasts for several hours (Marmorino et al., 2008), but reformation of slicks after periods of strong winds is possible within an hour (Hardy, 1991). Thus, slick persistence exceeds the life cycle duration of many microbes, which is minutes to hours (Wotton and Preston, 2005).
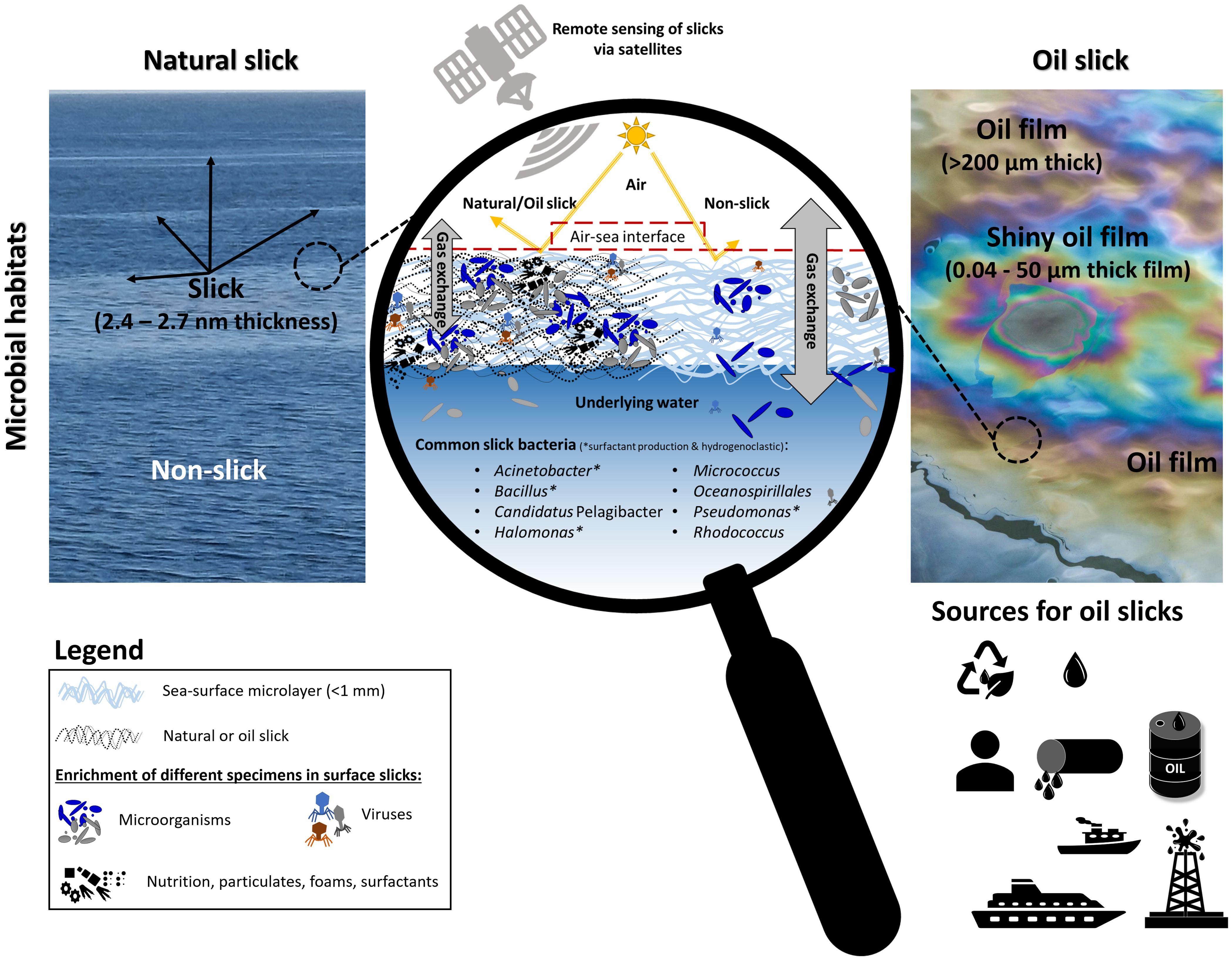
Figure 1 Concept of natural surface slicks (left) and oil slicks (right) and associated features. Both slick types interact with important air-sea interface processes compared to adjacent waters, schematized in the magnifying glass (middle). Changes result in increased organic matter content, temperature, viscosity, light reflection, and suppressed gas exchange (natural slick only). Particulates, foams, surfactants, and nutrients accumulate in slicks. Sources of oil slicks can have natural (from leakage in sea ground or from onshore leakage: two top symbols) or anthropogenic origin (pipelines, oil platforms, from oil prospects or production, from sea travel or transportation). Remote satellite techniques such as Synthetic Aperture Radar (SAR) imagery can be used to detect slicks from space. The oil slick picture was taken from pixabay.com, license is free for commercial use, no attribution is required. The natural slick photo was taken by one of the authors.
Early work suggested that slicks would be formed due to metabolically lipid-releasing organisms, mainly by diatoms. Mass mortalities of copepods releasing large quantities of wax esters were suspected to produce natural slicks in open ocean settings (Lee and Williams, 1974). Consequently, different organisms were assessed for their potential to induce slick-forming reactions (Dietz and Lafond, 1950). For example blooms of Trichodesmium, mainly Trichodesmium erythraeum, are typically associated with slicks (Sieburth and Conover, 1965; Sieburth, 1971; Dupouy et al., 2011; Rahlff et al., 2021) and the production of free fatty acids (Gosselin et al., 2021). In fact, natural surface slicks consist of complex polymeric material with a minor fraction of simple lipids (Hunter and Liss, 1981; Garabetian et al., 1993). Nowadays, it is more accepted that the accumulation of surfactants, exceeding an unknown threshold, seems responsible for the formation of natural slicks (Wurl et al., 2009; Cunliffe et al., 2013). Surfactants produced by bacteria, micro- and macroalgae (Sturdy and Fischer, 1966; Frew et al., 1990; Satpute et al., 2010) consist of well-oriented hydrophilic and hydrophobic parts and constitute diverse chemical structures like glycolipids, lipopeptides, phospholipids, fatty acid salts, and particulate structures (Ron and Rosenberg, 2001). Surfactants are surface-active compounds that lower surface tension and interfacial tension, e.g., between oil and water phase and have dampening effects on ripple waves, which leads to the typical appearance of a calmed water surface (Garrett and Bultman, 1963; Ermakov et al., 1986; Ermakov et al., 1992).
Organisms inhabiting the upper centimeters of the water column including slicks and the microlayer are collectively referred to as neuston (Naumann, 1917), which was estimated to contain 2 x 1023 prokaryotic cells globally (Flemming and Wuertz, 2019). Bacterioneuston community composition sometimes differs from the underlying bacterioplankton community, in particular among the particle-attached bacterial fraction and under slick or slick-like conditions (Stolle et al., 2010; Rahlff et al., 2019), while other studies described more similar community structures of neuston and plankton (Stolle et al., 2011; Zäncker et al., 2018). Overall, surface slicks are an important biological habitat, accumulating different marine organisms (Gallardo et al., 2021). Slicks aid distribution of algae, meroplanktonic larvae, and small fish (Shanks, 1983; Kingsford and Choat, 1986; Weidberg et al., 2014), act as nurseries for neustonic larval fishes and zooplankton (Whitney et al., 2021), and provide shelter and prey to different animals (Kropach, 1971; Whitney et al., 2021).
Prokaryotes in natural slicks
While supporting the higher trophic levels, slicks have been rarely investigated regarding their microbial inhabitants, although bacteria are long known for their abundance in surface slicks (Crow et al., 1975; Sewell et al., 1981). Little work has been done to reveal specific prokaryotic taxa and their metabolic and ecological functions in natural slicks (Table 1). The cyanobacterium Trichodesmium is probably the best and longest studied bacterial slick genus (Sieburth and Conover, 1965). Trichodesmium cells are active in slicks based on assessment of primary productivity and nitrogenase activity (Capone et al., 1998), although the microlayer habitat counts as a net heterotrophic system (Obernosterer et al., 2005; Rahlff et al., 2017) with many phototrophic organisms being substantially inhibited by high light intensities (Hardy and Apts, 1989). Trichodesmium even contributed to warming and inhibition of salinization of the slick surface water (Wurl et al., 2018). Apart from that, Wurl et al. (2016) showed that the bacterial community composition of microlayer collected in a slick was remarkably different from non-slick microlayer communities, which was particularly true for particle colonizers. In addition, community respiration is highly variable in slicks (Obernosterer et al., 2005). Kurata et al. (2016) applied 454 sequencing of the 16S rRNA gene and reported surfactant producers such as Acinetobacter spp. and Bacillus spp. with highest abundance in the subsurface below the slick, while within the slick, surfactant producers were mostly absent. Later, Howe et al. (2018) also detected Bacillus spp. in the water underlying a natural slick that formed at a convergent zone in the Gulf of Mexico. The authors of both studies concluded that surfactants could be produced in the water column and subsequently transported into the slick by means of advection, convection, and bubble scavenging processes. Due to ephemerality of slicks, presence of opportunistic Gammaproteobacteria, that typically inhabit non-slick microlayer, microlayer particles, and sea foams associated with slicks (Cunliffe et al., 2011; Rahlff et al., 2021) is likely. Recently, Rahlff et al. (2022) reported several bacterial taxa from metagenomes of surface microlayers sampled in slicky waters of the Swedish Skagerrak but no direct comparisons with non-slick microlayer from the same location has been carried out. Most abundant in these samples were Oceanospirillales, Flavobacterium psychrophilum, Marine Group I Thaumarchaeota, Hylemonella gracilis, Candidatus Pelagibacter, and Candidatus Ruthia magnifica among others (Table 1). Due to the overall scarcity of studies, it follows that much more work needs to be done to elucidate the prokaryotic community composition of natural slicks including the microbial role in surfactant production and involvement in direct gas metabolism. And doubtlessly, dominant taxa and functions are expected to vary with geographic location.
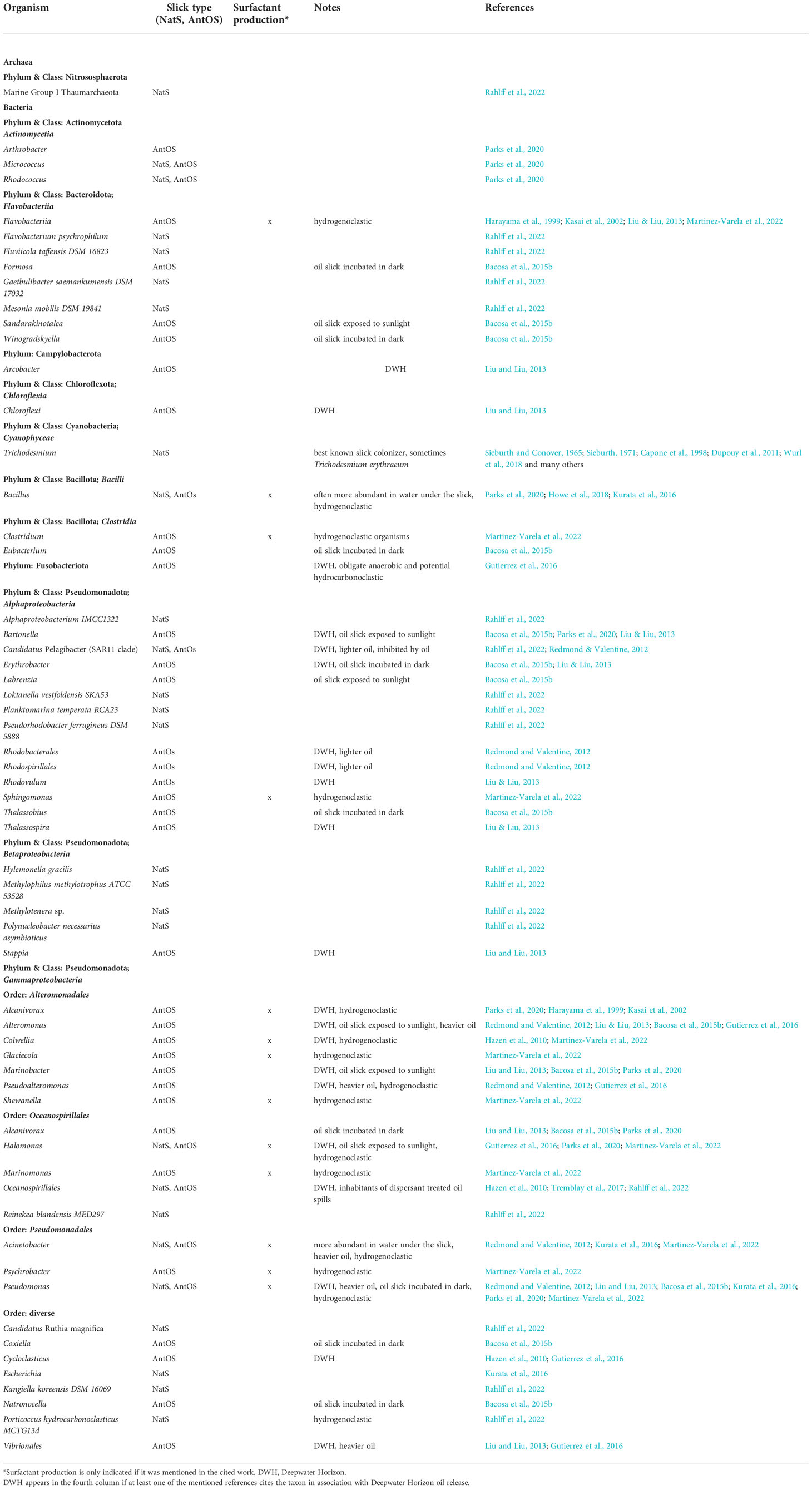
Table 1 Bacteria and archaea typically associated with natural slicks (NatS) and anthropogenic oil slicks (AntOS).
A general introduction into oil slicks
In contrast to natural slicks, oil slicks are less studied from the point of a natural microbial habitat, instead, the focus is often on biology of oil-polluted waters, responses to oil spills, and remediation. Oil slicks can show diverse appearance, i.e., very thin silvery/grey/rainbow shiny films on the water surface (Figure 1), often observable in harbor basins after minor oil releases. They can also occur as thick continuous or discontinuous brown/black oil films on the water surface after contaminations with larger oil volumes. Oil slick thickness ranges between 0.04 µm and > 200 µm (Peperzak et al., 2010), and patches up to 10 km length were reported (MacDonald et al., 2002). A ton of spilled oil can build oil slicks that cover an area of 5 x 106 m2 (Xue et al., 2015). An oil slick’s lifetime strongly depends on its thickness, oil composition, wind speeds and related mixing conditions, but slick dimensions do not affect oil slick lifetime (Zeinstra‐Helfrich et al., 2017). Thick oil slicks inhibit light penetration resulting in lower light intensities reaching the underlying water, affecting the growth of phototrophic organisms (Gao et al., 2021; Quigg et al., 2021). Oils slicks are hydrophobic and chemically complex mixtures containing several thousands of different compounds; hence, many compounds remain chemically uncharacterized through oil studies (Brussaard et al., 2016; Shi and Wu, 2021). Aliphatic, unsaturated, and aromatic hydrocarbons, asphaltenes and resins are the dominating compound classes in oils (Wilkes and Schwarzbauer, 2010). Depending on the chemical composition and the physical properties, oils are classified roughly into light oil and heavy oil. Oil slicks mainly consist of lighter oils, because the denser heavy oil components tend to sink to the sea floor (Stevens et al., 2015). Environmental conditions, such as temperature, sunlight, wind, and rain significantly affect the oil slicks' physicochemical properties and oil quality in terms of weathering, evaporation, dissolution, photo- and biodegradation (Golyshin et al., 2010; Aeppli et al., 2012; Bacosa et al., 2015a; Payne et al., 2016; Cederwall et al., 2020). A detailed physicochemical characterization of marine oil slicks is necessary for making microbiological results comparable across different studies. Sea surface water temperatures (epipelagic zone = top 200 m of the ocean) can range from -1.7°C to 29°C (Ikeda et al., 2001), demonstrating the need for reporting water temperatures to account for the oil slicks’ physical properties in addition to chemical composition. Additionally, oil slick surface area, volume, and thickness must be considered for microbial studies, as they can affect light availability and nutrient transport within the slick. Marine oil slicks can result from either natural leakages from fractures in the seafloor or from on-shore natural seepages spilling into the ocean (Kvenvolden and Cooper, 2003). Alternatively, they can result from anthropogenic sources, such as oil prospects or production, leakage of oil pipelines, oil spills from ships (Figure 1) and sea travel (Jernelöv, 2010a; Jernelöv, 2010b; Chen et al., 2019). When spilled at the water surface, oil components were found to dissolve quickly into sea water and were still detected and biologically available after a few hours down to eight meters below the formed slick (Brussaard et al., 2016). This demonstrates that not necessarily all spilled oil components are resulting in the formation of surface oil films i.e., slicks. Most recent and well-known oil spills that led to enormous oil slick formations at the sea surface are the Deepwater Horizon (DWH) offshore drilling rig explosion in 2010 and the Exxon Valdez oil tanker spill in 1989, located in the Gulf of Mexico and in the Gulf of Alaska, respectively (Zhang et al., 2019). In some anthropogenic oil spills, oils have lost their natural composition, because drilling fluids, different waters, or lighter oils have been added to facilitate oil production or ensure oil dilution for an easier transport through pipelines (Pannekens et al., 2019; Ortmann et al., 2020; Cobanli et al., 2022). Such additives often provide an extra nitrogen or carbon source and thus influence the microbial community composition of marine oil slicks by changing the initial nutrient supply (see below). In 2003, scientists estimated that 47% of crude oil seepages into marine environments originate from natural seeps and 53% from anthropogenic sources (Kvenvolden and Cooper, 2003). The estimated amount of naturally released oil was determined at 600,000 tons year-1 (Kvenvolden and Cooper, 2003; Kimes et al., 2014), which highlights the importance of studying the microbiology of natural oil slicks. In contrast, a recent published study identified most oil slicks in proximity to coast lines and determined that the origin of marine oil pollution is mainly caused by anthropogenic oil slicks (94%) rather than by natural oil slicks (6%) (Dong et al., 2022). Probably because of the lower frequency of natural oil slicks, much more research articles are available on the microbiology of anthropogenic oil slicks including those discussing effects of dispersants (Table 1). Detailed investigations of microbial community compositions of natural oil slicks were lacking (Burns et al., 2010; Ziervogel et al., 2014; Brussaard et al., 2016), which emphasizes a large knowledge gap on these phenomena.
Prokaryotes in oil slicks derived from anthropogenic oil spills
Microorganisms capable of hydrocarbon degradation (hydrocarbonoclasts) are ubiquitously abundant in the marine environment, albeit in low numbers (Golyshin et al., 2010). A metagenomic survey even identified that most metagenome-assembled genomes of non-contaminated samples from the Gulf of Mexico were similar to those from oil contaminated sites (Karthikeyan et al., 2020). This finding demonstrates the broad environmental distribution of hydrocarbonoclastic bacteria and the importance of studying more the overlooked natural oil leakages that are plentiful in the Gulf of Mexico (Ziervogel et al., 2014) and might provide microbial seed banks of hydrocarbonoclastic organisms into the ocean. A rule of thumb is that the heavier the oil, the more photo-oxidized or biologically degraded it is. In oil slicks, microbial degradation has been identified as the dominant degradation mechanism for abundant polyaromatic hydrocarbon compounds (PAHs) (González-Gaya et al., 2019). This contrasts an older study on oil slicks, that identified photo-oxidation as main degradation process of PAHs and bacteria as major source of alkane degradation (Bacosa et al., 2015a). Conflicting results might result from different oil types, different light intensities, or microbial communities with different oil degradation capabilities (reviewed by Bacosa et al., 2022). After an oil spill, the numbers of hydrocarbon-degrading microorganisms change rapidly, and Alpha-, Gammaproteobacteria, or Flavobacteriia were observed to dominate microbial communities with relative abundances > 60% to even 90% (Table 1) (Harayama et al., 1999; Kasai et al., 2002; Liu and Liu, 2013; Kimes et al., 2014). Microbial communities of oil slick and deep-water plume samples investigated from the DWH oil spill differed from each other. Deep water oil but not the slick samples contained DWH related Oceanospirillales (Hazen et al., 2010), while Colwellia, and Cycloclasticus were present in both samples (Redmond and Valentine, 2012). In addition, surface oil slicks were mainly dominated by Cyanobacteria, Alphaproteobacteria, and potential hydrocarbonoclastic organisms such as Alteromonadales and Oceanospirillales made up 15% of the total oil slick community. Within the same study, oil slicks consisting of heavier oil were found to be dominated by other organisms (Redmond and Valentine, 2012). Gutierrez et al. (2016) identified the same organisms and additionally Cycloclasticus and Halomonas as major DHW slick inhabitants (Table 1). Alteromonas and Pseudoalteromonas are known degraders of labile dissolved organic matter, which might be accumulated in oil slicks and thus provide an important carbon source (Sherwood et al., 2015; Goto et al., 2020; Liu et al., 2022). Sunlight, which oxidizes oil, significantly reduced bacterial diversity and evenness in incubations with DHW oil slicks. This is most likely because sunlight can change environmental conditions inside the slick, e.g., nutrient availability and temperature (Bacosa et al., 2015b). Here, microbial communities developed differently in incubations exposed to sunlight or darkness. Candidatus Pelagibacter was found to be inhibited by oil independent of light availability. While the relative abundance of the cyanobacterium Synechococcus was decreased in oil slicks exposed to sunlight, the abundance of Alteromonas, Marinobacter, Labrenzia, Sandarakinotalea, Bartonella, and Halomonas were strongly linked to light incubations. Their abundances increased but the diversity was higher in dark incubations. Oil slick samples incubated in the dark were inhabited by Thalassobius, Winogradskyella, Alcanivorax, Formosa, Pseudomonas, Eubacterium, Erythrobacter, Natronocella, and Coxiella (Table 1) (Bacosa et al., 2015b).
The role of marine oil snow, biosurfactants, and dispersants for oil slick microorganisms
Marine oil snow is degraded oil, which forms tiny particles glued by biogenic exopolymeric substances (Gutierrez et al., 2013a; Gregson et al., 2021; Quigg et al., 2021) sinking to the sea ground (Hua et al., 2018). The formation of marine oil snow at the boundary between oil slick and underlying water indicates that active oil biodegradation is happening (Passow et al., 2012; Passow, 2016), and these particles are hotspots for microbial oil degradation (Ziervogel et al., 2012). Apparently, microbial communities and marine oil snow production vary according to environmental conditions related to seasonal changes (temperature and light dependent) (Ziervogel et al., 2014; Ortmann et al., 2020). In general, microorganisms are capable of utilizing hydrocarbons under oxic, microaerophilic, and anoxic conditions as carbon source (Khot et al., 2021), but in marine oil slicks, microbial oil degradation dominantly occurs aerobically (Kimes et al., 2014; Gutierrez et al., 2016). Surprisingly, obligately anaerobic and potential hydrocarbonoclastic Fusobacteria have been isolated from DWH oil slicks. The authors hypothesized that those organisms got enriched in anaerobic zones related to marine oil snow particles (Gutierrez et al., 2016).
While biosurfactants are essential for the formation of natural slicks, in oil slicks they primarily increase the rate of oil biodegradation. Many Pseudomonadota are known biosurfactant producers and hydrogenoclastic organisms in the sea-surface microlayer of coastal Antarctica (Martinez-Varela et al. (2022), Table 1). A study focusing on microorganisms in microlayer associated with an oil slick identified amplicon sequence variants (ASVs) related to oil-degrading and surfactant-producing genera: Alcanivorax, Halomonas, Marinobacter, and Bacillus (Parks et al., 2020). Especially, Halomonas was dominant in water underlying the oil slick compared to non-slick underlying water, indicating surfactant production under the slick. In addition, the authors proposed that the genera Alcanivorax, Halomonas, Marinobacter might be indicative for the presence of oil in the slick (Parks et al., 2020). The presence of surfactants can even enhance hydrocarbon/oil removal by exerting emulsifying activity (Karanth et al., 1999). Microbial cells with high cell surface hydrophobicity, for example certain cyanobacteria (Fattom and Shilo, 1984) and most oil degraders, can function as the surfactant themselves and thus have high affinity towards air-water or oil-water interfaces (Karanth et al., 1999).
Marine oil slicks from anthropogenic sources often contain artificial dispersants, either added during oil production or for oil transport applied to remove the slick from the sea surface. Many studies have investigated the effect of diverse dispersants on microbial communities, and consistent was that dispersed oils are easier available to biodegradation resulting in higher prokaryotic abundances (Passow et al., 2012; Ziervogel et al., 2014; Duran and Cravo-Laureau, 2016; Quigg et al., 2021), higher oil degradation rates and expression of oil-degrading genes (Passow et al., 2012; Ziervogel et al., 2014; Tremblay et al., 2017). Organisms of the Oceanospirillales group have been proposed as vital inhabitants of dispersant treated oil spills (Tremblay et al., 2017). Dispersants were determined in microcosm experiments to have stronger selective effects on dominant species than crude oil itself (Zhou et al., 2022). Higher trophic levels like phytoplankton are often inhibited by high dispersant doses resulting in lower total carbon cycling rates (Ortmann et al., 2012), because higher doses cause higher solution of hydrocarbons and toxic compounds (Echeveste et al., 2010; Jung et al., 2010). The obvious differences between dispersed/modified and natural oil slicks point out that natural oil slicks provide distinct habitats worthwhile to study in more detail to understand the natural marine carbon-fluxes and biodegradation processes and rates.
Comparison of natural and oil slick features
Most obvious differences are that in comparison to oil slicks, natural slicks are quite unknown and harder to detect on the sea surface, have shorter lifetimes, are much thinner (nm vs. µm), contain other major nutrients (natural marine vs. hydrocarbons) and are not or less harmful and toxic to living organisms. Nevertheless, the two habitats cannot be strictly separated from each other, because some factors, such as slick detection, inhabitants, role of surfactants, varying physicochemical characteristics, and mechanisms of spreading organisms with slicks, play important roles in both. Methods for detection and monitoring natural and oil slicks range from simple visual and photographic observations (Romano and Marquet, 1991; Romano, 1996) to high-resolution remote sensing methods via satellites such as Synthetic Aperture Radar (SAR) (Migliaccio et al., 2015) (Figure 1). Considering key colonizing microorganisms of slicks, eight organisms were reported for both slick types, namely, Acinetobacter, Bacillus, Candidatus Pelagibacter, Halomonas, Micrococcus, Oceanospirillales, Pseudomonas, Rhodococcus, and half of these bacteria were associated with surfactant production (Figure 1 and Table 1). Surfactants play an important role in formation and stability of natural and oil slicks. In addition, by increasing solubility and the surface area of oil, surfactants can help bacteria to grow in slicks or access hydrocarbons (Shreve et al., 1995; Amani et al., 2010). Surface slicks and surfactants in sufficient quantities can suppress gas exchange across the air-sea boundary (Salter et al., 2011), e.g., natural slicks suppressed carbon dioxide flux by 62% (Mustaffa et al., 2020). Oil slicks were determined to reduce water evaporation on the sea surface by 15-33%, which is caused by diffusion and energy resistance at the film-water interface (Anikiev et al., 1988). However, at least according to older studies, gas exchange between water and atmosphere is not affected by oil slicks (Kinsey, 1973). Lowered salinity as caused by reduced evaporation might play an important role for microbial communities in oil slicks because microbial communities for instance in oil reservoirs have been observed to respond threshold-dependent to salinity, resulting in abrupt changes to less diverse and uneven communities (Shelton et al., 2016; Voskuhl et al., 2021).
One question refers to how bacteria spread within slicks and with moving and disintegrating slicks into the sea. So far, this has only been rudimentary investigated for natural slicks, but represents an interesting research question for oil slicks. Stolle et al. (2010) followed the formation and disintegration of a natural surface slick in the southern Baltic Sea and described strong enrichment of bacteria in the slick as well as changes in bacterioneuston composition during slick formation based on 16S rRNA and 16S rRNA gene analysis. During slick disintegration, the community composition of non-attached bacteria underwent stronger changes compared to particle-associated fractions. Passive transport of bacteria with particles from the underlying water has been discussed as a likely cause for the high abundance of bacteria in the slick (Stolle et al., 2010). Hale and Mitchell (1997) investigated bacterial spreading dynamics in artificially induced slicks inoculated with Vibrio natriegens. The bacterium showed localized high concentrations at the point source and at the leading edge of the slick, which suggests that it did not spread evenly within advancing slicks. The authors suggested that bacteria are concentrated and transported at the slick’s leading edge (Hale and Mitchell, 1997, Harper, 2006). Bacteria from the underlying water were incorporated evenly across the slick rather than displaced with potential to change the community composition of the bacterioneuston in the slick. Wotton and Preston (2005), who studied protists in pond slicks, suggested that passive drift with slicks would allow microbes to traverse greater distances with less energetic costs, and slicks would offer “an excellent locomotory substratum for gliding and crawling microorganisms”. In addition, natural slicks and oil slicks provide viscous habitats (Carlson, 1987; Cobanli et al., 2022) and recent work found that bacterial motility could be even enhanced in such complex fluids (Kamdar et al., 2022).
Microbial interactions and understudied groups in natural and oil slicks
Zooplankton and microorganisms comprise complex relationships in the marine environment, e.g., interactions related to predation or to algal cells providing a habitat for bacteria (Ramanan et al., 2015; Seymour et al., 2017). Zooplankton groups, e.g., heterotrophic nanoflagellates, tintinnids, ciliates and copepod larvae, were mostly reported being sensitive to oil spill exposure, probably due to the oil’s toxicity, stickiness and oil-mediated reduced light intensities (Ozhan et al., 2014; Brussaard et al., 2016; Quigg et al., 2021). Bacteria-bacteria and bacteria–phytoplankton interactions can change oil degradation processes and rates in the marine environment, and hence have different effects compared to single organism observations (McGenity et al., 2012), which has however not been investigated in oil slicks. For instance, hydrocarbon degradation was enhanced by photosynthesis in algal-bacterial associations (Borde et al., 2003; Warshawsky et al., 2007). Phytoplankton cells, which naturally produce hydrocarbons, represent a typical habitat for hydrocarbon-degrading bacteria even outside oil contaminations (Gunnison and Alexander, 1975; Binark et al., 2000; Mishamandani et al., 2016). Therefore, phytoplankton communities, despite being sometimes photo-inhibited at the air-sea boundary, could serve as natural seed bank of hydrocarbonoclastic bacteria into newly formed oil slicks (Mishamandani et al., 2016) and even enhance a quick colonization of oil slicks. Microbial community composition related to the diatom Skeletonema costatum changed after being exposed to oil in an artificial slick setting. Oil-degrading taxa such as Arenibacter, Marinobacter, Parvibaculum, Roseobacter clade, and Polycyclovorans algicola became more abundant over Piscirickettsiaceae/Methylophaga, while other common phytoplankton-associated taxa such as Flavobacteriaceae and Alphaproteobacteria became less numerous (Gutierrez et al., 2013b; Mishamandani et al., 2016).
To the best of our knowledge, no study has systematically compared the natural community composition of eukaryotic microbes from natural marine slicks with non-slick microlayer, while much more data exist on marine oil slicks (see above), however mainly on the non-natural ones. Certain dinoflagellates like Prorocentrum micans or Noctiluca scintillans can accumulate in natural slicks, enhance microbial production by releasing exudates, and, as the case for N. scintillans, produce ichthyotoxins (Wandschneider, 1979; Tilstone et al., 2010; Hallegraeff et al., 2019). Investigations on microbes in foams associated with natural slicks showed higher numbers of prokaryotes and small autotrophic eukaryotes as measured from flow cytometry compared to foams from non-slicks (Rahlff et al., 2021). In addition, reports about the abundance and ecological role of archaea in natural slicks are missing and only rarely reported in context of oil slicks, mostly identified via archaeal lipid biomarkers (Naehr et al., 2009; Burns et al., 2010). Euryarchaeota and Thaumarchaeota marine group I were found in the microlayer (Cunliffe et al., 2008; Wong et al., 2018; Rahlff et al., 2022) and in the context of oil microbiology but not specifically in oil slicks (Head et al., 2006; McGenity et al., 2012) although archaea can fulfil important functions, e.g., in the carbon or nitrogen cycle. Fungal populations (yeasts and molds), like bacteria, enrich in surface film within slicks (Crow et al., 1975). Different studies provide proof that fungi from marine environments are capable of hydrocarbon degradation (Sanyal et al., 2016; Dell’Anno et al., 2021), but we could not find literature on fungi investigated directly in oil slicks or detailed studies about fungal communities in natural slicks.
Another open research area is the ecological role of viruses and bacteriophages residing in natural and oil slicks. Viruses are often enriched in the microlayer (reviewed by Rahlff (2019)) and albeit bacteriophage isolates from slicks exist (Rahlff, 2022), the structure of slick viral communities especially on floating particles and their ecological functions are unknown. We assume viral lysis of host cells could substantially influence the local surfactant pool and thus enhance slick formation and associated dissolved organic matter release (the “viral shunt”, Wilhelm and Suttle (1999)) could further enhance the prevailing heterotrophy within surface films. Likewise, lysis-mediated release of complex polymeric particles from host cells, could induce formation of marine snow and oil snow and induce particle export to the deep ocean (concept of the “viral shuttle”, reviewed by Zimmerman et al. (2020)). It is long known that transparent exopolymer particles (TEPs) are enriched in surface slicks and that viruses adhere to them (Brussaard et al., 2005; Wurl et al., 2016). In addition, viruses could supply natural and oil slick bacteria with auxiliary metabolic genes, e.g., related to photosynthesis (Lindell et al., 2004), surfactant production or hydrocarbon degradation (both not known so far), thereby influencing carbon cycling and gas uptake from the atmosphere. Surfactant production may affect phage survival (Chattopadhyay et al., 2002) while on the other hand, bacterial film and foam formation can be prevented by phage exposure (Petrovski et al., 2011) allowing us to conclude that virus-surfactant interactions in marine slicks are complex and require further research efforts.
Discussion and future perspectives
With this mini review we intend to raise awareness for the existence of natural surface and oil slicks and stress the importance of investigating slick microbiology. Despite slicks being widespread and interfering with the ocean’s function as a sink for carbon dioxide by inhibiting its uptake, the importance of the neuston from air-sea interface habitats and the presence of slicks and natural oil slicks in protocols for large-scale neuston samplings have barely been emphasized (Gorsky et al., 2019; Tara Ocean et al., 2022). While wind speed as the most important parameter for sampling surface films/microlayer is often reported, the presence or absence of slicks mostly remains unstated. Reasons might be missing awareness of slick existence or difficulties of identifying slicks when being the middle of one on a boat. Due to their important role in the process of gas exchange suppression and their distinct microbial community composition with possible impacts for hydrocarbon degradation, surfactant production, and carbon export to deeper waters, recording slicks during sampling of surface films for microbial or chemical analyses would allow for a better understanding of their ecological function. Oceanographic surveys typically conduct water sampling along transects with the lowest depth being 3-5 m using a CTD Niskin rosette water sampler (Larkin et al., 2021), thereby neglecting the immediate air-sea interface. We encourage to report on the presence/absence of slicks and any natural or anthropogenic oil appearance in all cases where water sampling is conducted in the epipelagic/photic zone. Microbes and viruses at the shallowest sampling depth could influence slick formation at the air-sea boundary and, vice versa, slicks affect the microbiome beneath the air-water interface, e.g., by introduction of nutritional gradients or increasing light reflection, i.e., less light will penetrate to deeper depth. Moreover, we hope to inspire interdisciplinary collaborations between microbiologists, modelers, gas physicists, geologists, and oceanographers to study the functions of microbes and viruses in natural and oil slicks on directly regulating gas exchange by metabolic processes and indirectly by releasing surfactants. Finally, we assume that an improved understanding on the spreading dynamics of slicks, studied by state-of-the-art remote sensing methods and under consideration of a slick’s life span could aid to assess horizontal transport of multicellular organisms, microbes, and viruses with slicks. This would entail a range of implications, e.g., for investigating spread of viral infections into sea surface blooms, or the potential for oil degradation in the slick destination.
Author contributions
All authors listed have made a substantial, direct, and intellectual contribution to the work and approved it for publication.
Funding
JR received funding from the German Research Foundation (grant number DFG RA3432/1-1) for the project “Exploring the virioneuston: Viral-bacterial interactions between ocean and atmosphere (VIBOCAT)”. We acknowledge support by the Open Access Publication Fund of the University of Duisburg-Essen.
Acknowledgments
We thank the editor and reviewer for their valuable feedback that helped to improve the manuscript.
Conflict of interest
The authors declare that the research was conducted in the absence of any commercial or financial relationships that could be construed as a potential conflict of interest.
Publisher’s note
All claims expressed in this article are solely those of the authors and do not necessarily represent those of their affiliated organizations, or those of the publisher, the editors and the reviewers. Any product that may be evaluated in this article, or claim that may be made by its manufacturer, is not guaranteed or endorsed by the publisher.
References
Aeppli C., Carmichael C. A., Nelson R. K., Lemkau K. L., Graham W. M., Redmond M. C., et al. (2012). Oil weathering after the Deepwater Horizon disaster led to the formation of oxygenated residues. Environ. Sci. Technol. 46, 8799–8807. doi: 10.1021/es3015138
Amani H., Sarrafzadeh M. H., Haghighi M., Mehrnia M. R. (2010). Comparative study of biosurfactant producing bacteria in MEOR applications. J. Pet. Eng. 75, 209–214. doi: 10.1016/j.petrol.2010.11.008
Anikiev V., Mishukov V., Moiseevsky G., Tkalin A. (1988). The effect of oil films on water evaporation and oxygen content in sea water. GeoJournal 19–24. doi: 10.1007/BF02626367
Bacosa H. P., Erdner D. L., Liu Z. (2015a). Differentiating the roles of photooxidation and biodegradation in the weathering of Light Louisiana Sweet crude oil in surface water from the Deepwater Horizon site. Mar. Pollut. Bull. 95, 265–272. doi: 10.1016/j.marpolbul.2015.04.005
Bacosa H. P., Liu Z., Erdner D. L. (2015b). Natural sunlight shapes crude oil-degrading bacterial communities in northern Gulf of Mexico surface waters. Front. Microbiol. 6. doi: 10.3389/fmicb.2015.01325
Bacosa H. P., Ancla S. M. B., Arcadio C. G. L. A., Dalogdog J. R. A., Ellos D. M. C., Hayag H. D. A, et al (2022). From surface water to the deep sea: A review on factors affecting the biodegradation of spilled oil in marine environment. J. Mar. Sci. Eng. 10, 426. doi: 10.3390/jmse10030426
Binark N., Güven K. C., Gezgin T., Ünlü S. (2000). Oil pollution of marine algae. Bull. Environ. Contam. Toxicol. 64, 866–872. doi: 10.1007/s0012800083
Borde X., Guieysse B. T., Delgado O., Muñoz R., Hatti-Kaul R., Nugier-Chauvin C., et al. (2003). Synergistic relationships in algal–bacterial microcosms for the treatment of aromatic pollutants. Bioresour. Technol. 86, 293–300. doi: 10.1016/S0960-8524(02)00074-3
Brussaard C. P. D., Mari X., Van Bleijswijk J. D. L., Veldhuis M. J. W. (2005). A mesocosm study of Phaeocystis globosa (Prymnesiophyceae) population dynamics - II. Significance for the microbial community. Harmful Algae. 4, 875–893. doi: 10.1016/j.hal.2004.12.012
Brussaard C. P., Peperzak L., Beggah S., Wick L. Y., Wuerz B., Weber J., et al. (2016). Immediate ecotoxicological effects of short-lived oil spills on marine biota. Nat. Commun. 7, 11206. doi: 10.1038/ncomms11206
Burns K. A., Brinkman D. L., Brunskill G. J., Logan G. A., Volk H., Wasmund K., et al. (2010). Fluxes and fate of petroleum hydrocarbons in the Timor Sea ecosystem with special reference to active natural hydrocarbon seepage. Mar. Chem. 118, 140–155. doi: 10.1016/j.marchem.2009.11.010
Capone D. G., Subramaniam A., Montoya J. P., Voss M., Humborg C., Johansen A. M., et al. (1998). An extensive bloom of the N2-fixing cyanobacterium Trichodesmium erythraeum in the central Arabian Sea. Mar. Ecol. Prog. Ser. 172, 281–292. doi: 10.3354/meps172281
Cederwall J., Black T. A., Blais J. M., Hanson M. L., Hollebone B. P., Palace V. P., et al. (2020). Life under an oil slick: Response of a freshwater food web to simulated spills of diluted bitumen in field mesocosms. Can. J. Fish. Aquat. Sci. 77, 779–788. doi: 10.1139/cjfas-2019-0224
Chattopadhyay D., Chattopadhyay S., Lyon W. G., Wilson J. T. (2002). Effect of surfactants on the survival and sorption of viruses. Environ. Sci. Technol. 36, 4017–4024. doi: 10.1021/es0114097
Chen J., Zhang W., Wan Z., Li S., Huang T., Fei Y. (2019). Oil spills from global tankers: Status review and future governance. J. Clean. Prod. 227, 20–32. doi: 10.1016/j.jclepro.2019.04.020
Cobanli S. E., Wohlgeschaffen G., Ryther C., Macdonald J., Gladwell A., Watts T., et al. (2022). Microbial community response to simulated diluted bitumen spills in coastal seawater and implications for oil spill response. FEMS Microbiol. Ecol. 98, 1–13. doi: 10.1093/femsec/fiac033
Crow S. A., Ahearn D. G., Cook W. L., Bourquin A. W. (1975). Densities of bacteria and fungi in coastal surface films as determined by a membrane-adsorption procedure. Limnol. Oceanogr. 20, 644–646. doi: 10.4319/lo.1975.20.4.0644
Cunliffe M., Engel A., Frka S., Gašparović B., Guitart C., Murrell J. C., et al. (2013). Sea surface microlayers: A unified physicochemical and biological perspective of the air–ocean interface. Progr. Oceanogr. 109, 104–116. doi: 10.1016/j.pocean.2012.08.004
Cunliffe M., Schafer H., Harrison E., Cleave S., Upstill-Goddard R., Murrell J. C. (2008). Phylogenetic and functional gene analysis of the bacterial and archaeal communities associated with the surface microlayer of an estuary. ISME. J. 2, 776–789. doi: 10.1038/ismej.2008.28
Cunliffe M., Upstill-Goddard R. C., Murrell J. C. (2011). Microbiology of aquatic surface microlayers. FEMS Microbiol. Rev. 35, 233–246. doi: 10.1111/j.1574-6976.2010.00246.x
Dell’anno F., Rastelli E., Sansone C., Brunet C., Ianora A., Dell’anno A. (2021). Bacteria, fungi and microalgae for the bioremediation of marine sediments contaminated by petroleum hydrocarbons in the omics era. Microorganisms 9 (8), 1–22. doi: 10.3390/microorganisms9081695
Dong Y., Liu Y., Hu C., MacDonald I. R., Lu Y. (2022). Chronic oiling in global oceans. Science 17(6599), 1300–1304. doi: 10.1126/science.abm5940
Dupouy C., Benielli-Gary D., Neveux J., Dandonneau Y., Westberry T. (2011). An algorithm for detecting Trichodesmium surface blooms in the South Western Tropical Pacific. Biogeosciences 8, 3631–3647. doi: 10.5194/bg-8-3631-2011
Duran R., Cravo-Laureau C. (2016). Role of environmental factors and microorganisms in determining the fate of polycyclic aromatic hydrocarbons in the marine environment. FEMS Microbiol. Rev. 40, 814–830. doi: 10.1093/femsre/fuw031
Echeveste P., Agusti S., Dachs J. (2010). Cell size dependent toxicity thresholds of polycyclic aromatic hydrocarbons to natural and cultured phytoplankton populations. Environ. Pollut. 158, 299–307. doi: 10.1016/j.envpol.2009.07.006
Ermakov S. A., Salashin S. G., Panchenko A. R. (1992). Film slicks on the sea surface and some mechanisms of their formation. Dyn. Atmos. Oceans. 16, 279–304. doi: 10.1016/0377-0265(92)90010-Q
Ermakov S. A., Zujkova A. M., Panchenko A. R., Salashin S. G., Talipova T. G., Titov V. I. (1986). Surface film effect on short wind waves. Dyn. Atmos. Oceans. 10, 31–50. doi: 10.1016/0377-0265(86)90008-4
Fattom A., Shilo M. (1984). Hydrophobicity as an adhesion mechanism of benthic cyanobacteria. Appl. Environ. Microbiol. 47, 135–143. doi: 10.1128/aem.47.1.135-143.1984
Flemming H. C., Wuertz S. (2019). Bacteria and archaea on Earth and their abundance in biofilms. Nat. Rev. Microbiol. 17, 247–260. doi: 10.1038/s41579-019-0158-9
Frew N. M., Goldman J. C., Dennett M. R., Johnson A. S. (1990). Impact of phytoplankton-generated surfactants on air-sea gas exchange. J. Geophys. Res. 95, 3337–3352. doi: 10.1029/JC095iC03p03337
Gade M., Byfield V., Ermakov S., Lavrova O., Mitnik L. (2013). Slicks as indicators for marine processes. Oceanography 26, 138–149. doi: 10.5670/oceanog.2013.39
Gallardo C., Ory N. C., Gallardo M. D., Ramos M., Bravo L., Thiel M. (2021). Sea-surface slicks and their effect on the concentration of plastics and zooplankton in the coastal waters of Rapa Nui (Easter Island). Front. Mar. Sci. 8, 688224. doi: 10.3389/fmars.2021.688224
Gao J., Ming J., Xu M., Fu X., Duan L.-F., Xu C.-C., et al. (2021). Isolation and characterization of a high-efficiency marine diesel oil-degrading bacterium. Pet. Sci. 18, 641–653. doi: 10.1007/s12182-020-00540-z
Garabetian F., Romano J.-C., Paul R., Sigoillot J.-C. (1993). Organic matter composition and pollutant enrichment of sea surface microlayer inside and outside slicks. Mar. Environ. Res. 35, 323–339. doi: 10.1016/0141-1136(93)90100-E
Garrett W. D. (1965). Collection of slick-forming materials from the sea surface. Limnol. Oceanogr. 10, 602–605. doi: 10.4319/lo.1965.10.4.0602
Garrett W., Bultman J. D. (1963). The damping of water waves by insoluble organic monolayers (Washington DC: Naval Research Lab).
Golyshin P. N., Ferrer M., Chernikova T. N., Golyshina O. V., Yakimov M. M. (2010). “Oleispira,” in Handbook of hydrocarbon and lipid microbiology (Berlin, Heidelberg: Springer), 1755–1763.
González-Gaya B., Martínez-Varela A., Vila-Costa M., Casal P., Cerro-Gálvez E., Berrojalbiz N., et al. (2019). Biodegradation as an important sink of aromatic hydrocarbons in the oceans. Nat. Geosci. 12, 119–125. doi: 10.1038/s41561-018-0285-3
Gorsky G., Bourdin G., Lombard F., Pedrotti M. L., Audrain S., Bin N., et al. (2019). Expanding Tara Oceans protocols for underway, ecosystemic sampling of the ocean-atmosphere interface during Tara Pacific expedition. Front. Mar. Sci. 6, 750. doi: 10.3389/fmars.2019.00750
Gosselin K. M., Nelson R. K., Spivak A. C., Sylva S. P., Mooy V., Benjamin. A. S., et al. (2021). Production of two highly abundant 2-methyl-branched fatty acids by blooms of the globally significant marine cyanobacteria Trichodesmium erythraeum. ACS Omega. 6, 22803–22810. doi: 10.1021/acsomega.1c03196
Goto S., Tada Y., Suzuki K., Yamashita Y. (2020). Evaluation of the production of dissolved organic matter by three marine bacterial strains. Front. Microbiol. 11, 584419. doi: 10.3389/fmicb.2020.584419
Gower J., Hu C., Borstad G., King S. (2006). Ocean color satellites show extensive lines of floating Sargassum in the Gulf of Mexico. IEEE Trans. Geosci. Remote Sens. 44, 3619–3625. doi: 10.1109/TGRS.2006.882258
Green T., Houk D. (1979). The removal of organic surface films by rain. Limnol. Oceanogr. 24, 966–970. doi: 10.4319/lo.1979.24.5.0966
Gregson B. H., McKew B. A., Holland R. D., Nedwed T. J., Prince R. C., McGenity T. J. (2021). Marine oil snow, a microbial perspective. Front. Mar. Sci. 8, 619484. doi: 10.3389/fmars.2021.619484
Gunnison D., Alexander M. (1975). Basis for the resistance of several algae to microbial decomposition. Appl. Microbiol. 29, 729–738. doi: 10.1128/am.29.6.729-738.1975
Gutierrez T., Berry D., Teske A., Aitken M. D. (2016). Enrichment of Fusobacteria in sea surface oil slicks from the Deepwater Horizon oil spill. Microorganisms 4, 1–10. doi: 10.3390/microorganisms4030024
Gutierrez T., Berry D., Yang T., Mishamandani S., McKay L., Teske A., et al. (2013a). Role of bacterial exopolysaccharides (EPS) in the fate of the oil released during the Deepwater Horizon oil spill. PloS One 8, e67717. doi: 10.1371/journal.pone.0067717
Gutierrez T., Green D. H., Nichols P. D., Whitman W. B., Semple K. T., Aitken M. D. (2013b). Polycyclovorans algicola gen. nov., sp. nov., an aromatic-hydrocarbon-degrading marine bacterium found associated with laboratory cultures of marine phytoplankton. Appl. Environ. Microbiol. 79, 205–214. doi: 10.1128/AEM.02833-12
Hale M. S., Mitchell J. G. (1997). Sea surface microlayer and bacterioneuston spreading dynamics. Mar. Ecol. Progr. Ser. 147, 269–276. doi: 10.3354/meps147269
Hallegraeff G. M., Albinsson M. E., Dowdney J., Holmes A. K., Mansour M. P., Seger A. (2019). Prey preference, environmental tolerances and ichthyotoxicity by the red-tide dinoflagellate Noctiluca scintillans cultured from Tasmanian waters. J. Plankton. Res. 41, 407–418. doi: 10.1093/plankt/fbz037
Harayama S., Kishira H., Kasai Y., Shutsubo K. (1999). Petroleum biodegradation in marine environments. J. Mol. Microbiol. Biotechnol. 1, 63–70.
Hardy J. T., Apts C. W. (1989). Photosynthetic carbon-reduction - high-rates in the sea-surface microlayer. Mar. Biol. 101, 411–417. doi: 10.1007/BF00428138
Harper J. F. (2006). The leading edge of an oil slick, soap film, or bubble stagnant cap in stokes flow. J. Fluid. Mech. 237, 23–32. doi: 10.1017/S0022112092003331
Hazen T. C., Dubinsky E. A., Desantis T. Z., Andersen G. L., Piceno Y. M., Singh N., et al. (2010). Deep-sea oil plume enriches indigenous oil-degrading bacteria. Science 330, 204–208. doi: 10.1126/science.1195979
Head I. M., Jones D. M., Roling W. F. (2006). Marine microorganisms make a meal of oil. Nat. Rev. Microbiol. 4, 173–182. doi: 10.1038/nrmicro1348
Howe K. L., Dean C. W., Kluge J., Soloviev A. V., Tartar A., Shivji M., et al. (2018). Relative abundance of Bacillus spp., surfactant-associated bacterium present in a natural sea slick observed by satellite SAR imagery over the Gulf of Mexico. Elem. Sci. Anth. 6, 1–8. doi: 10.1525/elementa.268
Hua Y., Mirnaghi F. S., Yang Z., Hollebone B. P., Brown C. E. (2018). Effect of evaporative weathering and oil-sediment interactions on the fate and behavior of diluted bitumen in marine environments. Part 1. Spill-related properties, oil buoyancy, and oil-particulate aggregates characterization. Chemosphere 191, 1038–1047. doi: 10.1016/j.chemosphere.2017.10.156
Hühnerfuss H. (2006). “Basic physicochemical principles of monomolecular sea slicks and crude oil spills,” in Marine surface films (Berlin, Heidelberg: Springer), vol. 21-35.
Hunter K., Liss P. (1981). “Organic sea surface films,” in Elsevier oceanography series (New York: Elsevier), 259–298.
Ikeda T., Kanno Y., Ozaki K., Shinada A. (2001). Metabolic rates of epipelagic marine copepods as a function of body mass and temperature. Mar. Biol. 139, 587–596. doi: 10.1007/s002270100608
Jernelöv A. (2010a). How to defend against future oil spills. Nature 466, 182–183. doi: 10.1038/466182a
Jernelöv A. (2010b). The threats from oil spills: Now, then, and in the future. Ambio 39, 353–366. doi: 10.1007/s13280-010-0085-5
Jung S. W., Park J. S., Kown O. Y., Kang J. H., Shim W. J., Kim Y. O. (2010). Effects of crude oil on marine microbial communities in short term outdoor microcosms. J. Microbiol. 48, 594–600. doi: 10.1007/s12275-010-0199-2
Kamdar S., Shin S., Leishangthem P., Francis L. F., Xu X., Cheng X. (2022). The colloidal nature of complex fluids enhances bacterial motility. Nature 603, 819–823. doi: 10.1038/s41586-022-04509-3
Karanth N. G. K., Deo P. G., Veenanadig N. K. (1999). Microbial production of biosurfactants and their importance. Curr. Sci. 77, 116–126.
Karthikeyan S., Rodriguez R. L., Heritier-Robbins P., Hatt J. K., Huettel M., Kostka J. E., et al. (2020). Genome repository of oil systems: An interactive and searchable database that expands the catalogued diversity of crude oil-associated microbes. Environ. Microbiol. 22, 2094–2106. doi: 10.1111/1462-2920.14966
Kasai Y., Kishira H., Sasaki T., Syutsubo K., Watanabe K., Harayama S. (2002). Predominant growth of Alcanivorax strains in oil-contaminated and nutrient-supplemented sea water. Environ. Microbiol. 4, 141–147. doi: 10.1046/j.1462-2920.2002.00275.x
Khot V., Zorz J., Gittins D. A., Chakraborty A., Bell E., Bautista M. A., et al. (2021). CANT-HYD: A curated database of phylogeny-derived hidden Markov models for annotation of marker genes involved in hydrocarbon degradation. Front. Microbiol. 12, 764058. doi: 10.3389/fmicb.2021.764058
Kimes N. E., Callaghan A. V., Suflita J. M., Morris P. J. (2014). Microbial transformation of the Deepwater Horizon oil spill-past, present, and future perspectives. Front. Microbiol. 5, 603. doi: 10.3389/fmicb.2014.00603
Kingsford M. J., Choat J. H. (1986). Influence of surface slicks on the distribution and onshore movements of small fish. Mar. Biol. 91, 161–171. doi: 10.1007/BF00569432
Kinsey D. (1973). Small-scale experiments to determine the effects of crude oil films on gas exchange over the coral back-reef at Heron Island. Environ. Pollut. 4, 167–182. doi: 10.1016/0013-9327(73)90037-2
Kropach C. (1971). Sea Snake (Pelamis platurus) aggregations on slicks in Panama. Herpetologica 27 (2), 131–135.
Kurata N., Vella K., Hamilton B., Shivji M., Soloviev A., Matt S., et al. (2016). Surfactant-associated bacteria in the near-surface layer of the ocean. Sci. Rep. 6, 19123. doi: 10.1038/srep19123
Kvenvolden K. A., Cooper C. K. (2003). Natural seepage of crude oil into the marine environment. Geo-Mar. Lett. 23, 140–146. doi: 10.1007/s00367-003-0135-0
Larkin A. A., Garcia C. A., Garcia N., Brock M. L., Lee J. A., Ustick L. J., et al. (2021). High spatial resolution global ocean metagenomes from bio-GO-SHIP repeat hydrography transects. Sci. Data 8, 107. doi: 10.1038/s41597-021-00889-9
Lee R. F., Williams P. M. (1974). Copepod slick in the Northwest Pacific Ocean. Die Naturwissenschaften. 61, 505–506. doi: 10.1007/BF00622974
Lindell D., Sullivan M. B., Johnson Z. I., Tolonen A. C., Rohwer F., Chisholm S. W. (2004). Transfer of photosynthesis genes to and from Prochlorococcus viruses. Proc. Natl. Acad. Sci. U.S.A. 101, 11013–11018. doi: 10.1073/pnas.0401526101
Liu Z., Liu J. (2013). Evaluating bacterial community structures in oil collected from the sea surface and sediment in the northern Gulf of Mexico after the Deepwater Horizon oil spill. Microbiologyopen 2, 492–504. doi: 10.1002/mbo3.89
Liu S. T., Longnecker K., Kujawinski E. B., Vergin K., Bolanos L. M., Giovannoni S. J., et al. (2022). Linkages among dissolved organic matter export, dissolved metabolites, and associated microbial community structure response in the northwestern Sargasso Sea on a seasonal scale. Front. Microbiol. 13, 407. doi: 10.3389/fmicb.2022.833252
Macdonald I. R., Leifer I., Sassen R., Stine P., Mitchell R., Guinasso N. (2002). Transfer of hydrocarbons from natural seeps to the water column and atmosphere. Geofluids 2, 95–107. doi: 10.1046/j.1468-8123.2002.00023.x
Marmorino G., Askari F., Mied R. (2002). Observations of the creation and evolution of small-scale oceanic frontal cusps and slicks. J. Mar. Syst. 37, 17–29. doi: 10.1016/S0924-7963(02)00193-8
Marmorino G. O., Smith G. B., Toporkov J. V., Sletten M. A., Perkovic D., Frasier S. J. (2008). Evolution of ocean slicks under a rising wind. J. Geophys. Res.-Oceans. 113, 1–13. doi: 10.1029/2007JC004538
Martinez-Varela A., Casas G., Berrojalbiz N., Piña B., Dachs J., Vila-Costa M. (2022). Polycyclic aromatic hydrocarbon degradation in the sea-surface microlayer at coastal Antarctica. Front. Microbiol. 13. doi: 10.3389/fmicb.2022.907265
McGenity T. J., Folwell B. D., McKew B. A., Sanni G. O. (2012). Marine crude-oil biodegradation: a central role for interspecies interactions. Aquat. Biosyst. 8, 10. doi: 10.1186/2046-9063-8-10
Migliaccio M., Nunziata F., Buono A. (2015). SAR polarimetry for sea oil slick observation. Int. J. Remote Sens. 36, 3243–3273. doi: 10.1080/01431161.2015.1057301
Mishamandani S., Gutierrez T., Berry D., Aitken M. D. (2016). Response of the bacterial community associated with a cosmopolitan marine diatom to crude oil shows a preference for the biodegradation of aromatic hydrocarbons. Environ. Microbiol. 18, 1817–1833. doi: 10.1111/1462-2920.12988
Mustaffa N. I. H., Ribas-Ribas M., Banko-Kubis H. M., Wurl O. (2020). Global reduction of in situ CO2 transfer velocity by natural surfactants in the sea-surface microlayer. Proc. Math. Phys. Eng. Sci. 476, 20190763. 10.1098/rspa.2019.0763
Naehr T. H., Birgel D., Bohrmann G., Macdonald I. R., Kasten S. (2009). Biogeochemical controls on authigenic carbonate formation at the Chapopote “asphalt volcano”, Bay of Campeche. Chem. Geology. 266, 390–402. doi: 10.1016/j.chemgeo.2009.07.002
Naumann E. (1917). Beiträge zur Kenntnis des Teichnannoplanktons, II. Über das Neuston des Süsswassers. Biol. Centralblatt. 37, 98–106.
Obernosterer I., Catala P., Reinthaler T., Herndl G. J., Lebaron P. (2005). Enhanced heterotrophic activity in the surface microlayer of the Mediterranean Sea. Aquat. Microb. Ecol. 39, 293–302. doi: 10.3354/ame039293
Ortmann A. C., Anders J., Shelton N., Gong L., Moss A. G., Condon R. H. (2012). Dispersed oil disrupts microbial pathways in pelagic food webs. PloS One 7, e42548. doi: 10.1371/journal.pone.0042548
Ortmann A. C., Cobanli S. E., Wohlgeschaffen G., Macdonald J., Gladwell A., Davis A., et al. (2020). Measuring the fate of different diluted bitumen products in coastal surface waters. Mar. Pollut. Bull. 153, 111003. doi: 10.1016/j.marpolbul.2020.111003
Ozhan K., Parsons M. L., Bargu S. (2014). How were phytoplankton affected by the Deepwater Horizon oil spill? BioScience 64, 829–836. doi: 10.1093/biosci/biu117
Pannekens M., Kroll L., Muller H., Mbow F. T., Meckenstock R. U. (2019). Oil reservoirs, an exceptional habitat for microorganisms. N. Biotechnol. 49, 1–9. doi: 10.1016/j.nbt.2018.11.006
Parks G., Dean C. W., Kluge J. A., Soloviev A. V., Shivji M., Tartar A., et al. (2020). Analysis of surfactant-associated bacteria in the sea surface microlayer using deoxyribonucleic acid sequencing and synthetic aperture radar. Int. J. Remote Sens. 41, 3886–3901. doi: 10.1080/01431161.2019.1708508
Passow U. (2016). Formation of rapidly-sinking, oil-associated marine snow. Deep-Sea. Res. Pt. II. 129, 232–240. doi: 10.1016/j.dsr2.2014.10.001
Passow U., Ziervogel K., Asper V., Diercks A. (2012). Marine snow formation in the aftermath of the Deepwater Horizon oil spill in the Gulf of Mexico. Environ. Res. Lett. 7, 1–11. doi: 10.1088/1748-9326/7/3/035301
Payne J. R., Mcnabb G. D. Jr., Clayton. J. R. Jr (2016). Oil-weathering behavior in Arctic environments. Polar. Res. 10, 631–662. doi: 10.3402/polar.v10i2.6774
Peperzak L., Kienhuis P., Brussaard C. P. D., Huisman J. (2010). “Accidental and deliberate oil spills in Europe: Detection, sampling and subsequent analyses,” in Handbook of hydrocarbon and lipid microbiology (Berlin, Heidelberg: Springer),3471–3489.
Petrovski S., Seviour R. J., Tillett D. (2011). Prevention of Gordonia and Nocardia stabilized foam formation by using bacteriophage GTE7. Appl. Environ. Microb. 77, 7864–7867. doi: 10.1128/AEM.05692-11
Quigg A., Parsons M., Bargu S., Ozhan K., Daly K. L., Chakraborty S., et al. (2021). Marine phytoplankton responses to oil and dispersant exposures: Knowledge gained since the Deepwater Horizon oil spill. Mar. Pollut. Bull. 164, 112074. doi: 10.1016/j.marpolbul.2021.112074
Rahlff J. (2019). The virioneuston: A review on viral–bacterial associations at air–water interfaces. Viruses 11, 191. doi: 10.3390/v11020191
Rahlff J. (2022). Havets hud-och dess små invånare. Havsutsikt 1, 8–10. Available at: https://www.havet.nu/havsutsikt/artikel/havets-hud---och-dess-invanare.
Rahlff J., Esser S. P., Plewka J., Heinrichs M. E., Soares A., Scarchilli C., et al. (2022). Heads in the clouds: Marine viruses disperse bidirectionally along the natural water cycle. bioRxiv, 1–35. doi: 10.1101/2022.06.21.497027
Rahlff J., Stolle C., Giebel H. A., Mustaffa N. I. H., Wurl O., Herlemann D. P. R. (2021). Sea foams are ephemeral hotspots for distinctive bacterial communities contrasting sea-surface microlayer and underlying surface water. FEMS Microbiol. Ecol. 97, 1–14. doi: 10.1093/femsec/fiab035
Rahlff J., Stolle C., Giebel H. A., Ribas-Ribas M., Damgaard L. R., Wurl O. (2019). Oxygen profiles across the sea-surface microlayer-effects of diffusion and biological activity. Front. Mar. Sci. 6, 11. doi: 10.3389/fmars.2019.00011
Rahlff J., Stolle C., Wurl O. (2017). SISI: A new device for in situ incubations at the ocean surface. J. Mar. Sci. Eng. 5, 46. doi: 10.3390/jmse5040046
Ramanan R., Kang Z., Kim B.-H., Cho D.-H., Jin L., Oh H.-M., et al. (2015). Phycosphere bacterial diversity in green algae reveals an apparent similarity across habitats. Algal. Res. 8, 140–144. doi: 10.1016/j.algal.2015.02.003
Redmond M. C., Valentine D. L. (2012). Natural gas and temperature structured a microbial community response to the Deepwater Horizon oil spill. Proc. Natl. Acad. Sci. U.S.A. 109, 20292–20297. doi: 10.1073/pnas.1108756108
Romano J. C. (1996). Sea-surface slick occurrence in the open sea (Mediterranean, Red Sea, Indian Ocean) in relation to wind speed. Deep. Sea. Res. Part I. Oceanogr. Res. Pap. 43, 411–423. doi: 10.1016/0967-0637(96)00024-6
Romano J.-C., Marquet R. (1991). Occurrence frequencies of sea-surface slicks at long and short time-scales in relation to wind speed. Estuar. Coast. Shelf. Sci. 33, 445–458. doi: 10.1016/0272-7714(91)90083-N
Ron E. Z., Rosenberg E. (2001). Natural roles of biosurfactants. Environ. Microbiol. 3, 229–236. doi: 10.1046/j.1462-2920.2001.00190.x
Salter M. E., Upstill-Goddard R. C., Nightingale P. D., Archer S. D., Blomquist B., Ho D. T., et al. (2011). Impact of an artificial surfactant release on air-sea gas fluxes during deep ocean gas exchange experiment II. J. Geophys. Res. Oceans. 116, 1–9. doi: 10.1029/2011JC007023
Sanyal O., Shinde V. L., Meena R. M., Damare S., Shenoy B. D. (2016). The ITS-based phylogeny of fungi associated with tarballs. Mar. Pollut. Bull. 113, 277–281. doi: 10.1016/j.marpolbul.2016.09.052
Satpute S. K., Banat I. M., Dhakephalkar P. K., Banpurkar A. G., Chopade B. A. (2010). Biosurfactants, bioemulsifiers and exopolysaccharides from marine microorganisms. Biotechnol. Adv. 28, 436–450. doi: 10.1016/j.biotechadv.2010.02.006
Sewell L. M., Bitton G., Bays J. S. (1981). Evaluation of membrane adsorption-epifluorescence microscopy for the enumeration of bacteria in coastal surface films. Microb. Ecol. 7, 365–369. doi: 10.1007/BF02341431
Seymour J. R., Amin S. A., Raina J. B., Stocker R. (2017). Zooming in on the phycosphere: The ecological interface for phytoplankton-bacteria relationships. Nat. Microbiol. 2, 17065. doi: 10.1038/nmicrobiol.2017.65
Shanks A. L. (1983). Surface slicks associated with tidally forced internal waves may transport pelagic larvae of benthic invertebrates and fishes shoreward. Mar. Ecol. Prog. Ser. 13, 311–315. doi: 10.3354/meps013311
Shelton J. L., Akob D. M., Mcintosh J. C., Fierer N., Spear J. R., Warwick P. D., et al. (2016). Environmental drivers of differences in microbial community structure in crude oil reservoirs across a methanogenic gradient. Front. Microbiol. 7, 1535. doi: 10.3389/fmicb.2016.01535
Sherwood B. P., Shaffer E. A., Reyes K., Longnecker K., Aluwihare L. I., Azam F. (2015). Metabolic characterization of a model heterotrophic bacterium capable of significant chemical alteration of marine dissolved organic matter. Mar. Chem. 177, 357–365. doi: 10.1016/j.marchem.2015.06.027
Shi Q., Wu J. (2021). Review on sulfur compounds in petroleum and its products: State-of-the-art and perspectives. Energy Fuels. 35, 14445–14461. doi: 10.1021/acs.energyfuels.1c02229
Shreve G. S., Inguva S., Gunnam S. (1995). Rhamnolipid biosurfactant enhancement of hexadecane biodegradation by Pseudomonas aeruginosa. Mol. Mar. Biol. Biotechnol. 4, 331–337.
Sieburth J. (1971). An instance of bacterial inhibition in oceanic surface water. Mar. Biol. 11, 98–100. doi: 10.1007/BF00348026
Sieburth J. M., Conover J. T. (1965). Slicks associated with Trichodesmium blooms in the Sargasso Sea. Nature 205, 830–831. doi: 10.1038/205830b0
Stevens C. C., Thibodeaux L. J., Overton E. B., Valsaraj K. T., Nandakumar K., Rao A., et al. (2015). Sea surface oil slick light component vaporization and heavy residue sinking: Binary mixture theory and experimental proof of concept. Environ. Eng. Sci. 32, 694–702. doi: 10.1089/ees.2015.0022
Stolle C., Labrenz M., Meeske C., Jürgens K. (2011). Bacterioneuston community structure in the southern Baltic Sea and its dependence on meteorological conditions. Appl. Environ. Microbiol. 77, 3726–3733. doi: 10.1128/AEM.00042-11
Stolle C., Nagel K., Labrenz M., Jürgens K. (2010). Succession of the sea-surface microlayer in the coastal Baltic Sea under natural and experimentally induced low-wind conditions. Biogeosciences 7, 2975–2988. doi: 10.5194/bg-7-2975-2010
Sturdy G., Fischer W. H. (1966). Surface tension of slick patches near kelp beds. Nature 211, 951–952. doi: 10.1038/211951b0
Tara Ocean F., Tara O., , European Molecular Biology, L., and European Marine Biological Resource Centre - European Research Infrastructure, C (2022). Priorities for ocean microbiome research. Nat. Microbiol. 7, 937–947. doi: 10.1038/s41564-022-01145-5
Tilstone G. H., Airs R. L., Vicente V. M., Widdicombe C., Llewellyn C. (2010). High concentrations of mycosporine-like amino acids and colored dissolved organic matter in the sea surface microlayer off the Iberian Peninsula. Limnol. Oceanogr. 55, 1835–1850. doi: 10.4319/lo.2010.55.5.1835
Tremblay J., Yergeau E., Fortin N., Cobanli S., Elias M., King T. L., et al. (2017). Chemical dispersants enhance the activity of oil- and gas condensate-degrading marine bacteria. ISME. J. 11, 2793–2808. doi: 10.1038/ismej.2017.129
Voskuhl L., Akbari A., Muller H., Pannekens M., Brusilova D., Dyksma S., et al. (2021). Indigenous microbial communities in heavy oil show a threshold response to salinity. FEMS Microbiol. Ecol. 97, fiab157. doi: 10.1093/femsec/fiab157
Wandschneider K. (1979). Vertical distribution of phytoplankton during investigations of a natural surface-film. Mar. Biol. 52, 105–111. doi: 10.1007/BF00390417
Warshawsky D., Ladow K., Schneider J. (2007). Enhanced degradation of benzo[α]pyrene by Mycobacterium sp. in conjunction with green alga. Chemosphere 69, 500–506. doi: 10.1016/j.chemosphere.2007.03.031
Weidberg N., Lobon C., Lopez E., Florez L. G., Rueda M. D. F., Largier J. L., et al. (2014). Effect of nearshore surface slicks on meroplankton distribution: Role of larval behaviour. Mar. Ecol. Prog. Ser. 506, 15–30. doi: 10.3354/meps10777
Whitney J. L., Gove J. M., McManus M. A., Smith K. A., Lecky J., Neubauer P., et al. (2021). Surface slicks are pelagic nurseries for diverse ocean fauna. Sci. Rep. 11, 1–18. doi: 10.1038/s41598-021-81407-0
Wilhelm S. W., Suttle C. A. (1999). Viruses and nutrient cycles in the sea: Viruses play critical roles in the structure and function of aquatic food webs. Bioscience 49, 781–788. doi: 10.2307/1313569
Wilkes H., Schwarzbauer J. (2010). “Hydrocarbons: An introduction to structure, physico-chemical properties and natural occurrence,” in Handbook of hydrocarbon and lipid microbiology. Ed. Timmis K. N. (Berlin, Heidelberg: Springer Berlin Heidelberg), 1–48.
Wong S. K., Ijichi M., Kaneko R., Kogure K., Hamasaki K. (2018). Ammonia oxidizers in the sea-surface microlayer of a coastal marine inlet. PloS One 13, e0202636. doi: 10.1371/journal.pone.0202636
Wotton R. S., Preston T. M. (2005). Surface films: Areas of water bodies that are often overlooked. Bioscience 55, 137–145. doi: 10.1641/0006-3568(2005)055[0137:SFAOWB]2.0.CO;2
Wurl O., Bird K., Cunliffe M., Landing W. M., Miller U., Mustaffa N. I. H., et al. (2018). Warming and inhibition of salinization at the ocean’s surface by cyanobacteria. Geophys. Res. Lett. 45, 4230–4237. doi: 10.1029/2018GL077946
Wurl O., Miller L., Röttgers R., Vagle S. (2009). The distribution and fate of surface-active substances in the sea-surface microlayer and water column. Mar. Chem. 115, 1–9. doi: 10.1016/j.marchem.2009.04.007
Wurl O., Stolle C., Van Thuoc C., The Thu P., Mari X. (2016). Biofilm-like properties of the sea surface and predicted effects on air–sea CO2 exchange. Progr. Oceanogr. 144, 15–24. doi: 10.1016/j.pocean.2016.03.002
Xue J., Yu Y., Bai Y., Wang L., Wu Y. (2015). Marine oil-degrading microorganisms and biodegradation process of petroleum hydrocarbon in marine environments: A review. Curr. Microbiol. 71, 220–228. doi: 10.1007/s00284-015-0825-7
Zäncker B., Cunliffe M., Engel A. (2018). Bacterial community composition in the sea surface microlayer off the Peruvian coast. Front. Microbiol. 9. doi: 10.3389/fmicb.2018.02699
Zeinstra-Helfrich M., Koops W., Murk A. J. (2017). Predicting the consequence of natural and chemical dispersion for oil slick size over time. J. Geophys. Res. 122, 7312–7324. doi: 10.1002/2017JC012789
Zhang B., Matchinski E. J., Chen B., Ye X., Jing L., Lee K. (2019). “Marine oil spills–oil pollution, sources and effects,” in World Seas: An environmental evaluation (Amsterdam, The Netherlands: Elsevier Ltd.), 391–406.
Zhou Y., Kong Q., Zhao X., Lin Z., Zhang H. (2022). Dynamic changes in the microbial community in the surface seawater of Jiaozhou Bay after crude oil spills: An in situ microcosm study. Environ. Pollut. 307, 119496. doi: 10.1016/j.envpol.2022.119496
Ziervogel K., D’souza N., Sweet J., Yan B., Passow U. (2014). Natural oil slicks fuel surface water microbial activities in the northern Gulf of Mexico. Front. Microbiol. 5, 188. doi: 10.3389/fmicb.2014.00188
Ziervogel K., McKay L., Rhodes B., Osburn C. L., Dickson-Brown J., Arnosti C., et al. (2012). Microbial activities and dissolved organic matter dynamics in oil-contaminated surface seawater from the Deepwater Horizon oil spill site. PloS One 7, e34816. doi: 10.1371/journal.pone.0034816
Keywords: neuston, surface film, bacteria, surfactants, sea-surface microlayer, spill, hydrocarbon, biodegradation
Citation: Voskuhl L and Rahlff J (2022) Natural and oil surface slicks as microbial habitats in marine systems: A mini review. Front. Mar. Sci. 9:1020843. doi: 10.3389/fmars.2022.1020843
Received: 16 August 2022; Accepted: 02 November 2022;
Published: 25 November 2022.
Edited by:
Maria Montserrat Sala, Institute of Marine Sciences (CSIC), SpainReviewed by:
Michael Cunliffe, Marine Biological Association of the United Kingdom, United KingdomCopyright © 2022 Voskuhl and Rahlff. This is an open-access article distributed under the terms of the Creative Commons Attribution License (CC BY). The use, distribution or reproduction in other forums is permitted, provided the original author(s) and the copyright owner(s) are credited and that the original publication in this journal is cited, in accordance with accepted academic practice. No use, distribution or reproduction is permitted which does not comply with these terms.
*Correspondence: Lisa Voskuhl, lisa.voskuhl@uni-due.de; Janina Rahlff, janina.rahlff@uol.de