- 1State Key Laboratory of Marine Environmental Science, College of Ocean and Earth Sciences, Xiamen University, Xiamen, China
- 2Fujian Key Laboratory of Genetics and Breeding of Marine Organisms, Xiamen University, Xiamen, China
The circadian rhythm is one of the most crucial and universal biological rhythms in living organisms. As a typical nocturnal creature, the Pacific abalone (Haliotis discus hannai) exhibits rhythmic behaviors in terms of passively selecting whether to avoid predators or to forage, and active adaptation to light cycle changes is regulated by the biological clock. However, no basic data are available to help us to understand these rhythmic behaviors in the abalone species. In the present study, quantification of behavioral data for the abalone and its predator swimming crab Portunus trituberculatus in short-term (24 h) and long-term (40 days) polyculture scenarios suggests that the distance and duration of movement, percentage of feeding individuals, and cumulative duration of feeding of the abalone individuals were significantly lower under the short-term predation risk than the long-term predation risk. The concentrations of 5-hydroxytryptamine (5-HT), cyclic adenosine monophosphate (cAMP), protein kinase A (PKA), and hexokinase (HK) in hemolymph, and expression levels of 5-HT1A receptor and 5-HT2 receptor in cerebral ganglion were significantly higher under the long-term predation risk than the short-term predation risk. The concentration of lactate dehydrogenase (LDH) and glycogen content in adductor muscle of the abalone was significantly higher under the short-term predation risk than the long-term predation risk, thereby implying their role in anaerobic metabolism and aerobic metabolism as primary energy sources under the short-term and long-term predation risk, respectively. The concentrations of 5-HT and cAMP, and the expression levels of Bmal1 and 5-HT2 receptor exhibited no significant signs of cosine rhythmicity under the short-term predation risk, but changes in the movement and feeding behaviors of the abalone still occurred at the night only. Correlation analysis shows that the expression levels of Bmal1 and Clock had significantly positive correlations with the circadian changes in the movement parameters of the abalone, thereby suggesting a dominant role in the rhythmic expression of endogenous circadian clock genes regulating the rhythmic behavior of the abalone. These findings provide new insights into the origin and evolution of biological rhythms in nocturnal marine animals and a reference for developing rational stock enhancement plans, and would improve protection for marine benthic biodiversity.
1 Introduction
All organisms are exposed to predation risk and prey animals must frequently trade off fitness-related behaviors such as foraging against the risk of predation. Predators may directly affect animals by killing prey, or indirectly affect prey through chemical or visual cues (non-consumption effects), thereby resulting in behavioral, physiological, or morphological changes in the prey (Clinchy et al., 2013; Sheriff et al., 2020). In the presence of predators, feeding activity of prey is often accompanied by a high risk of predation, so it is necessary to assess the feeding cost under predation risk and any consequent changes in feeding activities due to hiding or avoiding detection by predators (Brown and Kotler, 2004). For example, prey may adjust the foraging time, reduce or change the feeding time (Macleod et al., 2005; Strobbe et al., 2011), or choose a safer feeding mode to reduce the level of predation risk, e.g., by feeding in a place closer to shelter or adopting additional cautious behaviors while feeding (Biesinger et al., 2011). However, anti-predation behaviors often lead to lower energy acquisition. In addition, prey may exhibit various physiological responses to enhance the defense level to support escape and remain highly alert, thereby boosting the likelihood of survival (Briceno et al., 2018; Tigreros et al., 2018). According to the general stress paradigm, it is accepted that the presence of a predator would lead to physiological changes in prey. The physiological stress responses of prey include higher respiration and metabolic rates, as well as the catabolism of energy storage molecules such as glycogen and triglycerides. These physiological responses incur additional energy costs which affect the growth and normal physiology of prey (Hawlena and Schmitz, 2010). Decreased energy intake and increased energy expenditure lead to an energy deficit, thereby turn stimulating a greater desire for foraging and increasing the possibility of mortality due to the future predation risk (Hernandez et al., 2019). Thus, a trade-off must be made by prey between the effect of starvation and the predation risk.
The anti-predation behaviors of animals are connected with the temporal pattern of predation risk. The risk allocation hypothesis predicts that the anti-predation behavior of an animal is considered to follow the threat sensitivity rule, which means that the anti-predation behavior and feeding behavior are allocated according to the level of risk present in the environment (Helfman, 1989). The feeding activity level diminishes under high risk, whereas additional time would be allocated to feeding activity under low risk condition. However, the anti-predation behavior of prey would change with the duration under predation risk. In particular, if predation risk is present for a long period and the safe period is shorter, prey would reduce the defense cost while increase its feeding activity to the greatest extent possible to maintain the energy required for moving (Lima and Bednekoff, 1999). Many models and experiments have confirmed the risk allocation hypothesis but some findings do not fully support this theory. For example, the foraging activity of the dogwhelk (Nucella lapillus) declines during periods of danger. A longer period under predation risk results in a shorter safe period and a gradual increase in feeding activity, but this only occurs when N. lapillus is starved (Matassa and Trussell, 2014). In addition, prey may experience chronic stress under long-term risk, and a delay in the stress response may have adverse consequences, such as retarded growth, the consumption of stored energy, and accumulation of toxic compounds. As a result, prey may readjust its physiological functions and utilize physiological compensation mechanisms (e.g., improving assimilation efficiency) to avoid the losses due to a long-term predation stress (Thaler et al., 2012; Jermacz et al., 2020). For example, the energy cost may increase if a high metabolic rate is maintained. To decrease the costs, an individual prey may reduce its metabolic levels under long-term predation risk (Handelsman et al., 2013). Compared with short-term predation risk, the growth rate of the common blue damselfly (Enallagma cyathigerum) did not decrease under long-term predation risk but decreased in the glycogen content, indicating the redistribution of energy during growth (Van Dievel et al., 2016).
Most animals follow a circadian rhythm with a cycle of about 24 h. In general, the circadian rhythm of physiology and behavior is a strategy for adapting to periodic change in environmental conditions, but this does not mean that the activities of animals should follow a rhythm precisely. Avoiding predators is considered one of the reasons for the origin and change in an animal’s circadian rhythm (Vaze and Sharma, 2013). For example, the feeding rhythm of a marine zooplankter, Acartia hudsonica, involves consuming food at the night as a strategy for avoiding diurnal foraging predators (Bollens and Stearns, 1992). After a few months of culture without exposure to predation risk, the diurnal feeding rhythm of a marine protozoan, Strombidium arenicola, is lost, but reintroducing predators leads to the reappearance of this circadian rhythm (Arias et al., 2021). The Pacific abalone, Haliotis discus hannai, is an economically important marine shellfish in China with an output of 203,500 tons in 2020, which was about 90% of the world’s gross output of abalones. Despite increases in outputs from artificial culture each year, the wild resources had declined drastically. To enhance the natural populations, stock enhancement is expected to rapidly restore declining populations and maintain the sustainability of fishery activities (Aspe et al., 2019; Chauvaud et al., 2021). Increasing the success of stock enhancement requires assessments of how well the hatchery-bred individuals adapt to wild conditions. In particular, abalones are a group of typical nocturnal animals that stay away from light and hide in dark areas in the daytime, with a feeding peak at the night (Lyu et al., 2021). Olfaction cues are widely used by aquatic gastropods to detect and avoid predators, as well as to search for food (Manriquez et al., 2021). It is not clear whether the circadian behavior of abalones is a passive response to avoid predators or an active adaptation to light cycle change regulated by the circadian clock. In the present study, we employed the swimming crab, Portunus trituberculatus (a common species found in shallow seas), as a predator, and observed the circadian changes in the behavior and physiology of the Pacific abalone under short-term and long-term predation pressure to obtain new insights into the mechanism associated with the rhythmic behavioral patterns of the abalone. Based on the risk allocation hypothesis, we predicted that: (1) under the short-term predation risk, the abalone would reduce its feeding activity and exhibit stress responses, thereby increase its metabolic rate to boost levels of caution and responsiveness, and thus incur increased energy consumption; (2) to compensate for decreased energy intake and the resultant risk of starvation, the abalone would intensify its level of feeding activity under the long-term predation risk, or exhibit compensatory physiological responses with an enhanced aerobic metabolic capacity to utilize energy more effectively, and thus offset the adverse effects of energy consumption under long-term stress; and (3) the presence of predation risk would change the feeding rhythm of the abalone, with a shift in the peak feeding period.
2 Materials and methods
2.1 Collection and acclimation of abalones
The abalone of a shell length of 4.93 ± 0.18 cm and weight of 16.59 ± 1.96 g used in the experiments were purchased from Fuda Abalone Co., Ltd. They had not been co-cultured with any other organism prior to the experiment. The abalone were evenly assigned to the following three groups to investigate the effects of short-term and long-term predation risk on their rhythmic behavior: zero predation risk group (control) where 90 abalones were acclimated in three aquaria; short-term predation risk group (90 abalones were acclimated in three aquaria) where the abalone were only co-cultured with the crab for 24 h after the start of the experiment; and a long-term predation risk group where one cage was hung in each of the three aquaria and one P. trituberculatus (body weight: 192.15 ± 22.06 g) was introduced into each cage as a predator, and 90 abalones were acclimated for 40 days in three aquaria. During the course of acclimation, the ambient conditions were maintained at a water temperature of 19.1 ± 0.5°C, salinity of 30 ± 1, pH of 8.2, dissolved oxygen concentration > 6 mg L−1, with the natural light cycle, and entire seawater was exchanged once a day. Fresh Gracilaria lemaneiformis were provided as food daily at 16:00 pm at a feeding rate of approximately 3% of the wet weight of an abalone to ensure that each abalone was fully fed. Fresh abalones were collected as a food source for crabs and the feeding frequency was once each day.
2.2 Experimental design
Movement behavior of the abalone was continuously observed in a purpose-built rectangular tank (105 cm × 35 cm × 30 cm). The rectangular tank was divided into three zones by two perforated (hole diameter: 1 cm) partition boards, thereby allowing the abalone and crabs to be placed separately in different parts, but water could still flow throughout between the different parts (Figure 1). An infrared camera placed above the tank was connected to a hard disk recorder to record behavior of the abalone. To observe behavior of the abalone under zero predation risk, six abalones were introduced from the zero predation risk group into the middle part of the unit at 16: 00 every day. Food was provided and the abalone were observed continuously for 24 h. All of the seawater was exchanged on day 2 and six abalones were introduced from the zero predation risk group again. Six replicates were observed. In the short-term predation risk experiment (in which the The abalone had no previous contact with the crab prior to the experiment), six abalones from the short-term predation risk group were introduced into the middle part of the tank at 16:00 every day and a crab was then randomly placed in either left or right part of the tank to eliminate a bias effect of the position where the predator was present. Observations were conducted continuously for 24 h, with six replicates. In the long-term predation risk assessment, six abalones from the acclimated long-term predation risk group were introduced into the middle part of the tank at 16: 00 every day and a crab was randomly placed in either left or right part of the tank. Observation procedure was the same as mentioned above. No abalone or crab was reused in the experiment.
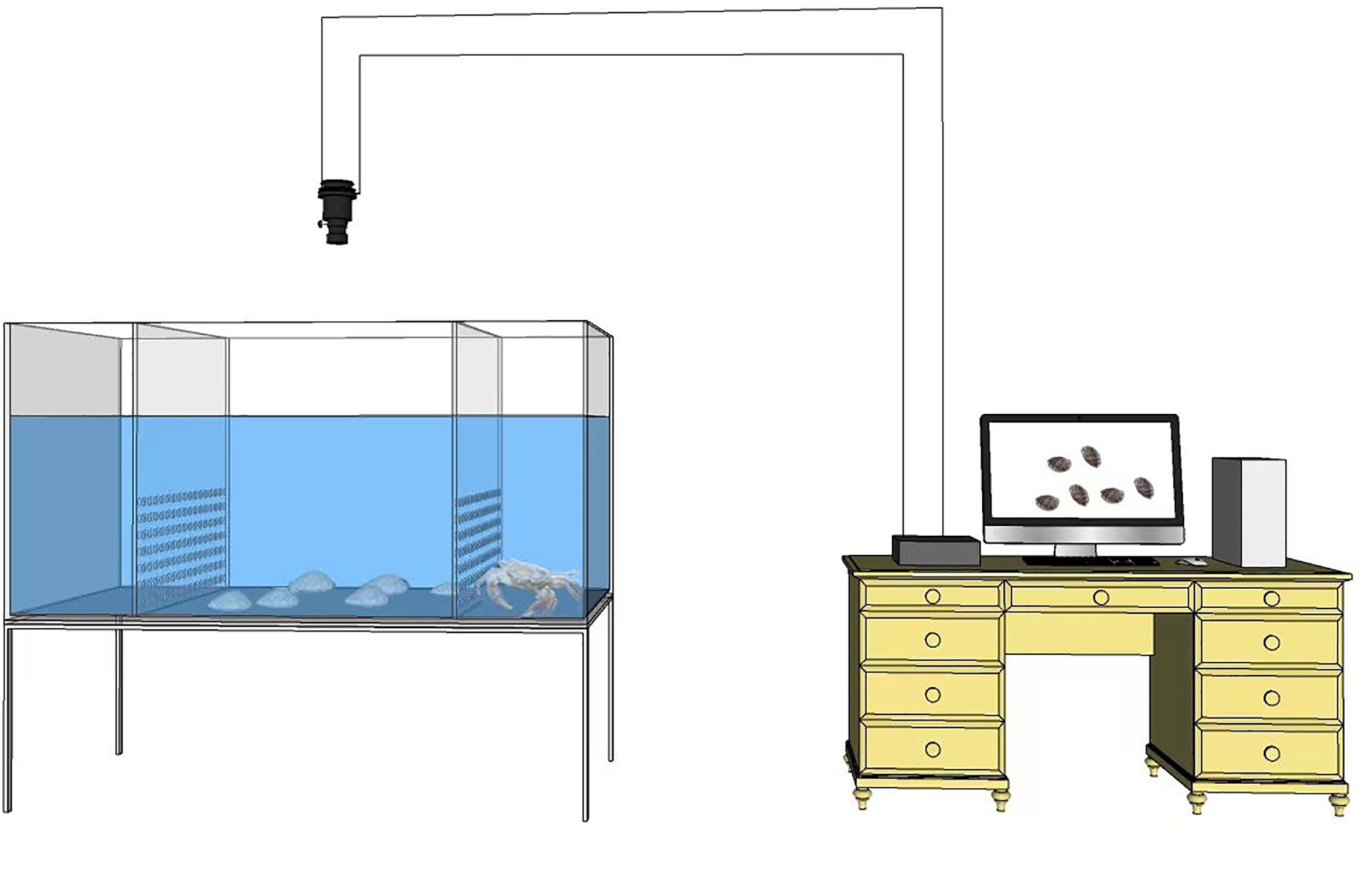
Figure 1 Moving behavior determined by real-time monitoring for Pacific abalone and crab under short-term (24 h) and long-term (40 days) polyculture in a rectangular water tank.
To determine the effect of predation risk on physiology of the abalone, after acclimation for 40 days six abalones were collected randomly from the three groups (for the short-term predation risk group, the cage loaded with the crab was not placed into the tank until the experiment commenced) and dissected at 16: 00 (ZT0), 20: 00 (ZT4), 00: 00 (ZT8), 04: 00 (ZT12), 08: 00 At 00 (ZT16), 12: 00 (ZT20), and 16: 00 (ZT24). The hemolymph, adductor muscle, and cerebral ganglion were placed into 2 mL centrifuge tubes, before immediately storing in liquid ammonia and transferring to a freezer at –80°C for subsequent determinations of the hormone contents, enzyme activities, and expression levels of related genes.
2.3 Behavioral observations
Behavioral parameters were analyzed with EthoVision XT 9.0 Behavioral Analysis Software (Noldus Information Technology, Wageningen, Netherlands). The distance moved (DM), cumulative duration of movement (CDM), and mean velocity of moving (MVM) of the abalone were recorded continuously for 24 h. We observed whether the abalone consumed food at specific location in the tank and the reduction of the food amount to derive the cumulative duration of feeding (CDF), and calculated the ratio of feeding individual relative to all individuals (PTSF) in different periods. Clustering was represented as the mean individual-to-individual distance (IID), where the coordinate points per frame for each abalone were acquired from the recordings to calculate the IID. The video settings comprised a total recording time of 24 h, time interval of 5 s, AVI video format, video resolution of 680 × 480 pixels, and frame speed of 10 fps. IID was calculated as:
where. xt ., yt denote the horizontal and vertical coordinates of an abalone at time t, respectively.
2.4 Sample analyses
2.4.1 Determination of 5-hydroxytryptamine, cyclic adenosine monophosphate, and protein kinase A concentrations
Fluid on the surfaces of the abalone was absorbed with blotting paper and the surfaces of their feet were sterilized with alcohol, before removing the blood sinusoids using a sterilized scalpel and collecting the hemolymph with a pipette. Each sample was transferred to a centrifuge tube and kept at room temperature for 20 min, before centrifuging at 3000 × g for 10 min and collecting the supernatant. The samples were analyzed using an ELISA kit (Shanghai Chuntest Biotechnology Co. Ltd, China). The concentrations of 5-HT (Code CS-00E96322), cAMP (CS-00E985211), and PKA (CS-00E985210) were determined using the double antibody sandwich method. Purified 5-HT, cAMP, and PKA antibodies were used to coat microtiter plate wells to prepare solid-phase antibodies. Next, 5-HT, cAMP, and PKA were added to the microwells in order to bind to the detection antibody labeled with horseradish peroxidase until the antibody–antigen-enzyme labeled antibody complex formed. To separate the antibody–antigen-enzyme labeled antibody complex fixed to the bottom of the microwells from the rest of the liquid, the supernatant was discarded and the microwells was rinsed five times with the washing fluid. Then adding 3,3′,5,5′-tetramethylbenzidine for color development, which changed to blue under catalysis by horseradish peroxidase and finally to yellow under the action of sulfuric acid solution. The color strength was positively correlated with the 5-HT, cAMP, and PKA concentrations in the samples. The absorbance (optical density) was measured with a microplate reader at 450 nm to build standard curves, the 5-HT, cAMP, and PKA concentrations in the samples were determined from the standard curves.
2.4.3 Enzyme activity and glycogen content analyses
Approximately 0.2–0.4 g of hepatopancreas was removed and ground with 1.8 mL of 0.86% normal saline in an ice-water bath, before centrifuged at 3,500 × g for 10 min to prepare 10% tissue homogenate for metabolic enzyme activity assays. Hexokinase (HK), lactate dehydrogenase (LDH), and glycogen were determined using a kit (Beijing Solarbio Science & Technology Co., Ltd) following the method described by Gao et al. (2016). The HK (Code BC0740) activity was determined based on the coupling reaction with glucose-6-phosphate dehydrogenase at 37°C and pH 7.6, where the amount of tissue protein per 1 g that generated 1 mmol NADPH every 1 min (i.e., 1 enzyme activity unit) was calculated. One LDH (BC0740) activity unit was determined as that required to produce 1 μmol pyruvic acid from the entire chemical reaction system (4 mol/L NaOH, 2,4-dinitrophenylhydrazine, 2 mmol/L sodium pyruvate, and coenzyme I) with 1 mg of tissue protein. The glycogen content was determined using the anthrone method. In particular, glycogen was extracted with strong alkaline extraction solution and measured with anthrone chromogenic reagent under strong acidic conditions. The protein content of the homogenate was determined using Coomassie blue staining, as described by Bradford (1976), with bovine serum albumin as the protein marker.
2.4.4 Assays of expression levels of 5-HT1A receptor, 5-HT2 receptor, Clock, and Bmal1 in the day and night
The cerebral ganglion samples were stored in liquid nitrogen, ground, and then rapidly mixed with 1mL TRIzol (Invitrogen, USA) to extract the total RNA. The primer sequences (Table 1) were designed for fluorescent quantification of 5-HT1A receptor, 5-HT2 receptor, Clock, and Bmal1 using primer3 (v0.4.0; http://bioinfo.ut.ee/primer3-0.4.0/primer3/) by referring to the genome sequencing data that we obtained for H. discus hannai (unpublished) and published sequence information for the 5-HT receptor (Kim et al., 2019). Each sample was evenly mixed in a PCR tube and then placed in a PCR plate (Roche Diagnostics, Indianapolis, IN, USA). PCR amplification was performed after transient centrifugation. The PCR reaction conditions comprised initial denaturation at 94°C for 30 s, followed by cycling at 94°C for 5 s and 60°C for 30 s, with 40 cycles in total. Melting curve analysis was conducted at the end of the experiment. For each RNA sample and gene, three replicates were analyzed by PCR. The mRNA levels of the target genes were calibrated using the real-time PCR Ct (2–ΔΔCt) relative quantitative method, with the 18S gene reference as the quantitative standard.
2.5 Data analysis
Results were expressed as the mean ± standard deviation. A two-factor analysis of variance (ANOVA) was performed using IBM SPSS Statistics 26 to determine the statistical significance of differences in the main effects of time and predation risk. Before analyzing the data, their conformance to a normal distribution and the homogeneity of variance assumption were validated using the Kolmogorov–Smirnov test and Levene’s test, respectively. Data that did not conform to the homogeneity of variance assumption were analyzed using Dunnett’s T3 test. If two-way ANOVA results are significant, Tukey test was applied to within group comparisons. Because time point in the present study is not a real effective variable, the interaction between risk and time point was ignored.
Pearson’s correlation coefficients were calculated between the behavioral parameters, hormone enzyme activities, and gene expression levels using IBM SPSS Statistics 26. The intrinsic relationships were identified between behavioral characteristics and the circadian expression of hormones and circadian clock genes to elucidate the mechanisms associated with the rhythmic behavior of the abalone.
Cosine fitting was performed based on the data using the Cosinor program package in Matlab. M (MESO) represents the median value of the fitted cosine curve, A, and Φ represents the amplitude and peak value of the cosine fit (Nelson et al., 1979). The F-test was used in the zero-amplitude test to analyze the significance of the circadian rhythm. P-values lower than 0.05 were considered significant.
3 Results
3.1 Comparative analysis of behavioral parameters
ANOVA results indicate that the presence of predation risk significantly (Table 2, P< 0.05) affected all measured behavioral parameters which are significantly (Table 2, P< 0.05) differed at different time points. In contrast to the control group, the presence of the long-term and short-term predation risks had significant effect on the duration and velocity of movement, and distance of moving by the abalone (Table 2, P< 0.05). Under the long-term predation risk, the duration and distance of movement by the abalone were significantly higher than those under the short-term predation risk (Figures 2A, B, P< 0.05). At the ZT0, ZT4, ZT8, ZT12, and ZT24 time points, the durations of moving were significantly shorter under the short-term predation risk compared with the control group. Except at ZT4, the distances of moving at all other time points were significantly less than those in the control group (P< 0.05). The mean velocities of moving at ZT0 and ZT24 were significantly higher in the short-term predation risk group than the control group. Except at ZT4, the mean velocities of moving at all time points were significantly higher in the long-term predation risk group than the control group (Figure 2C, P< 0.05). Observations of the clustering behavior of the abalone show that the predation risk had a significant effect on IID (Figure 2D, P< 0.05). Compared with the control group, exposure to predation risk significantly decreased the IID values at ZT0, ZT4, ZT8, and ZT24. The IID values in the long-term predation risk group were significantly higher at ZT12, ZT16, and ZT20 compared with those in the control and short-term predation risk groups (P< 0.05). Cosine rhythm analysis shows that the distance of moving had no cosine rhythm in the control group, whereas significant cosine rhythms were found in the other groups (Table 3). The peak for the duration of moving in the control group occurred at ZT04:49, which was almost 2 h after the peaks in the long-term and short-term predation risk groups at ZT02:28 and ZT02:30, respectively. The maximum mean velocities of moving in the control group and long-term predation risk group occurred at ZT05:40 and ZT05:30, respectively, but at ZT03:42 in the short-term predation risk group.
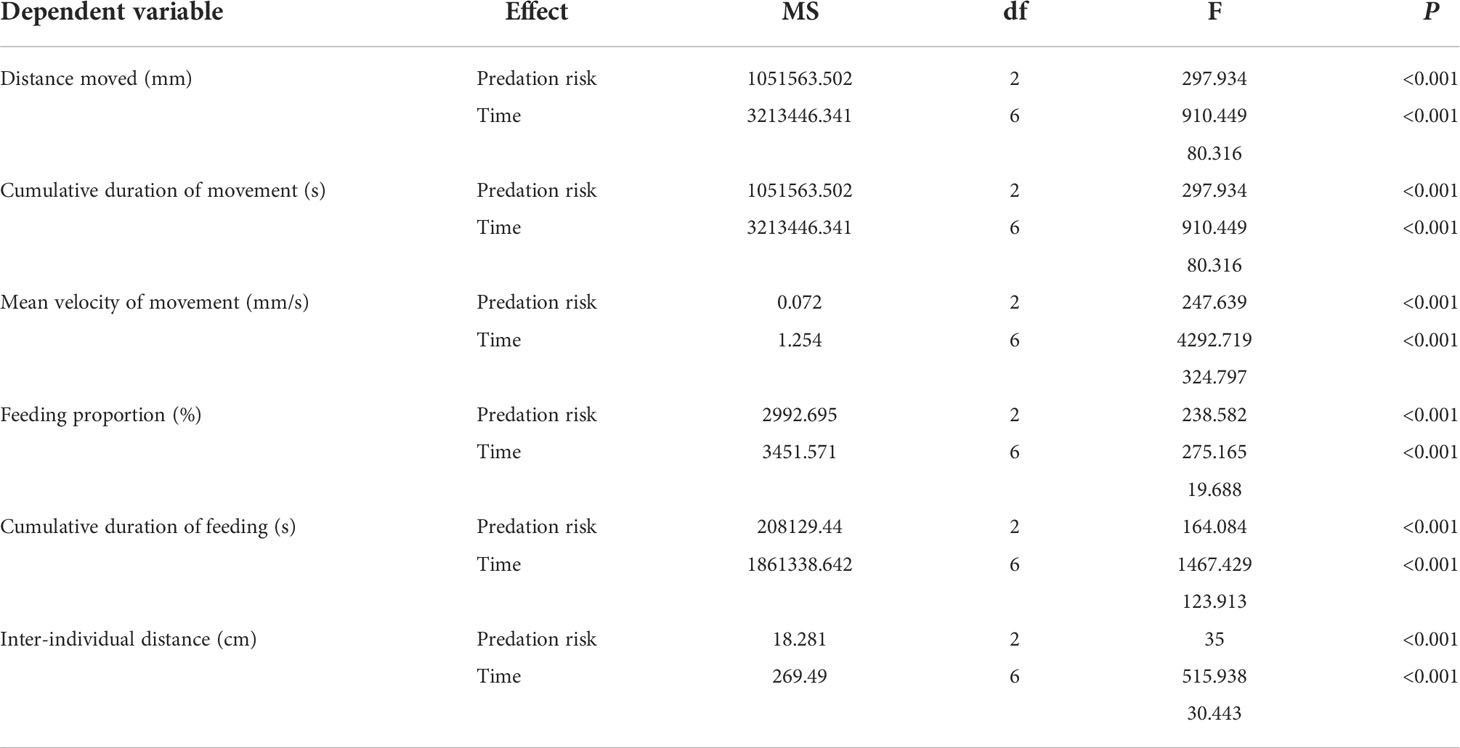
Table 2 Results of the two-way ANOVA conducted to test the effect of different predation risks and times on the behavioral parameters.
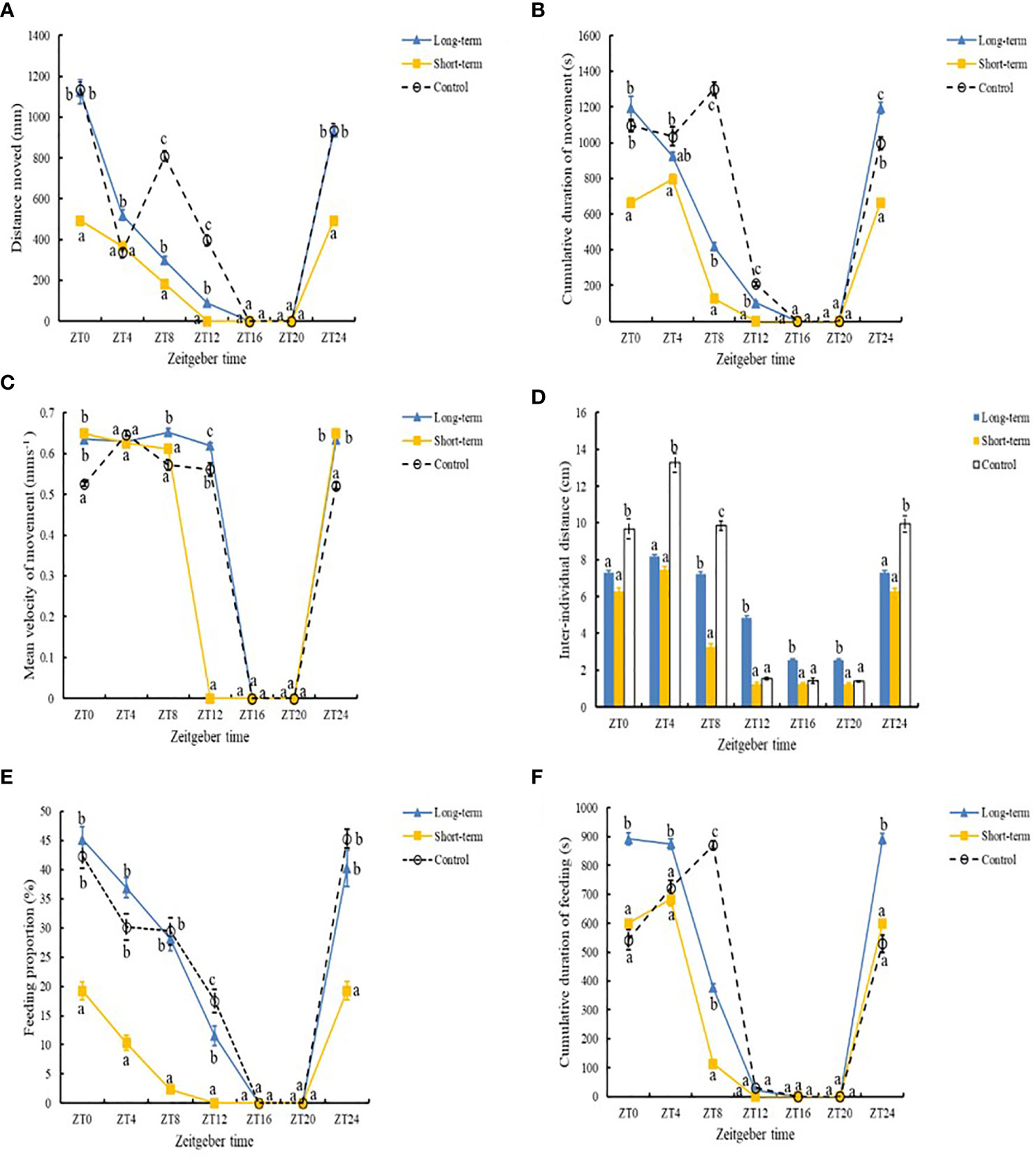
Figure 2 Distance moved (A), cumulative duration of movement (B), mean velocity of movement (C), proportion of time spent feeding (D), cumulative duration of feeding (E), and inter-individual distance (F) under different predation risks: Long-term (long-term predation risk), Short-term (short-term predation risk), and Control (no predation risk). Values are expressed as mean ± SD (n = 6). Different lowercase letters indicate significant differences in the behavioral parameters of abalones among different groups at the same sampling time (P< 0.05).
Predation risk also had significant effects on the percentage of feeding individuals and the cumulative duration of feeding (Table 2, P< 0.05). The percentages of feeding individuals in the long-term predation risk group and control group were significantly higher than that in the short-term predation risk group (Figure 2E, P< 0.05). The cumulative duration of feeding was significantly longer in the long-term predation risk group than the control group (Figure 2F, P< 0.05). At ZT0, ZT4, ZT8, ZT12, and ZT24, the percentages of feeding individuals were significantly lower in the short-term predation risk group than the control group. Except at ZT12, no significant differences were found in the percentages of feeding individuals in the long-term predation risk group and control group at all time points. Cosine rhythm analysis showed that the maximum percentages of feeding individuals and cumulative durations of feeding occurred at similar time points in the control group and long-term predation risk group, but the peak values for these two parameters occurred almost 2 h earlier in the short-term predation risk group at ZT01:27 and ZT02:26, respectively (Table 3).
3.2 Differences in blood biochemical indexes and cosine rhythm analysis
The presence of predation risk did not significantly affect the 5-HT concentration (Table 4, P > 0.05)but significantly (Table 4, P< 0.05) effect cAMP, PKA and glycogen contents, as well as HK and LDH activities, however, all measured blood biochemical indexes differed significantly at different time points (Table 4, P< 0.05). At ZT0 and ZT24, the 5-HT concentration was significantly higher in the control group than the short-term predation risk group (Figure 3A). At ZT20, the 5-HT concentration was significantly higher in the short-term predation risk group than the long-term predation risk group (P< 0.05). According to cosine rhythm analysis, cosine rhythms were found in terms of the variations in the 5-HT concentration in the control group and long-term predation risk group, but not in the short-term predation risk group (Table 5). The cAMP and PKA contents were significantly higher in the long-term predation risk group than the short-term predation risk group (Figures 3B, C, P< 0.05). At ZT4 and ZT8, the cAMP contents were significantly higher in the long-term predation risk group than the short-term predation risk group, but no significant differences were identified at any other time points. Except at ZT0, ZT20, and ZT24, the PKA concentrations were significantly lower in the short-term predation risk group than the long-term predation risk group at all time points. Under long-term predation risk, significant cosine rhythms were identified in terms of the variations in the cAMP and PKA contents (Table 5, P< 0.05).
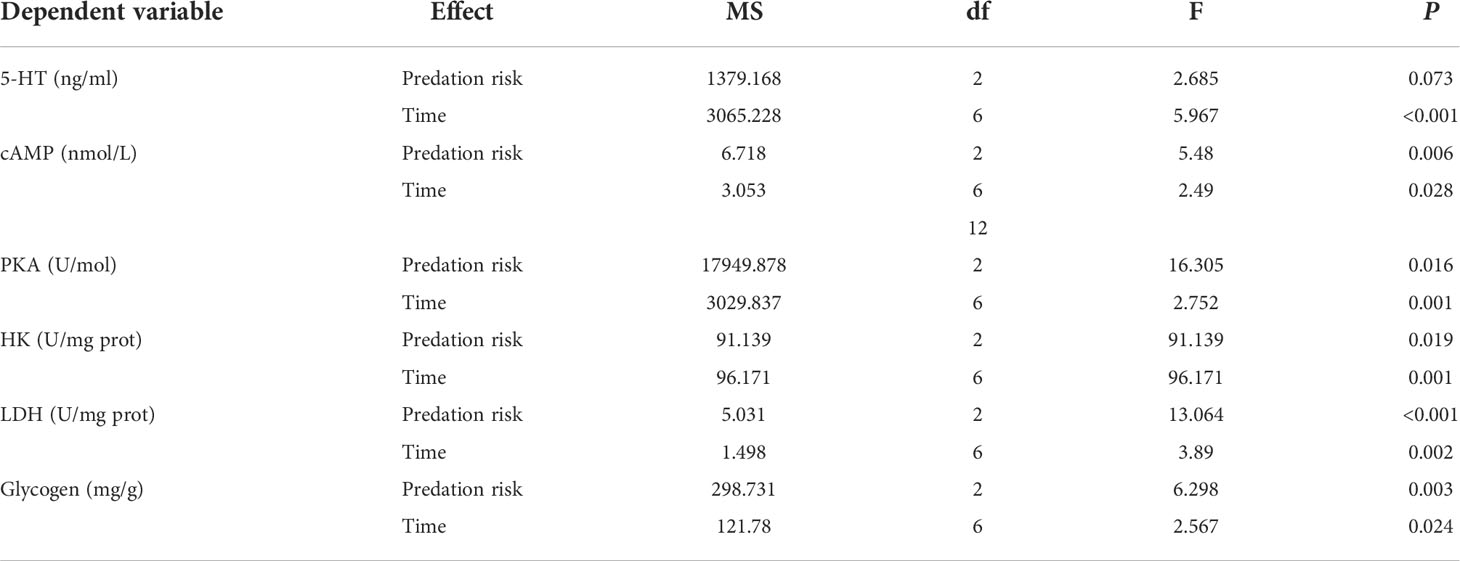
Table 4 Results of the two-way ANOVA conducted to test the effect of different predation risks and times on the contents of hormones and glycogen, and enzyme activities.
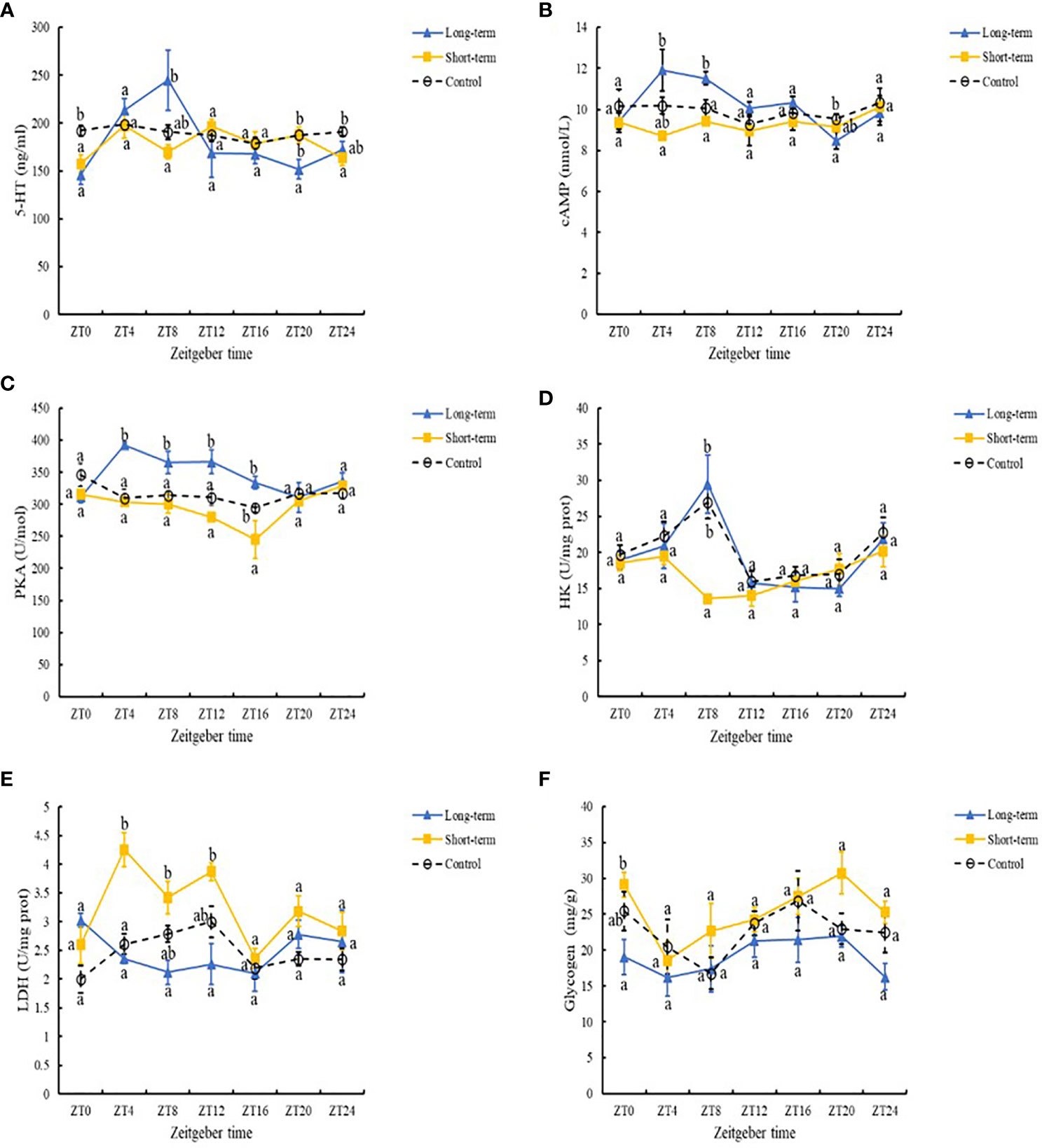
BAFigure 3 5-HT (A), cAMP (B), PKA (C), and glycogen (D) contents, as well as HK (E) and LDH (F) enzyme activities under different predation risks: Long-term (long-term predation risk), Short-term (short-term predation risk), and Control (no predation risk). Values are expressed as mean ± SD (n = 6). Different lowercase letters indicate significant differences among different groups at the same sampling time (P< 0.05).
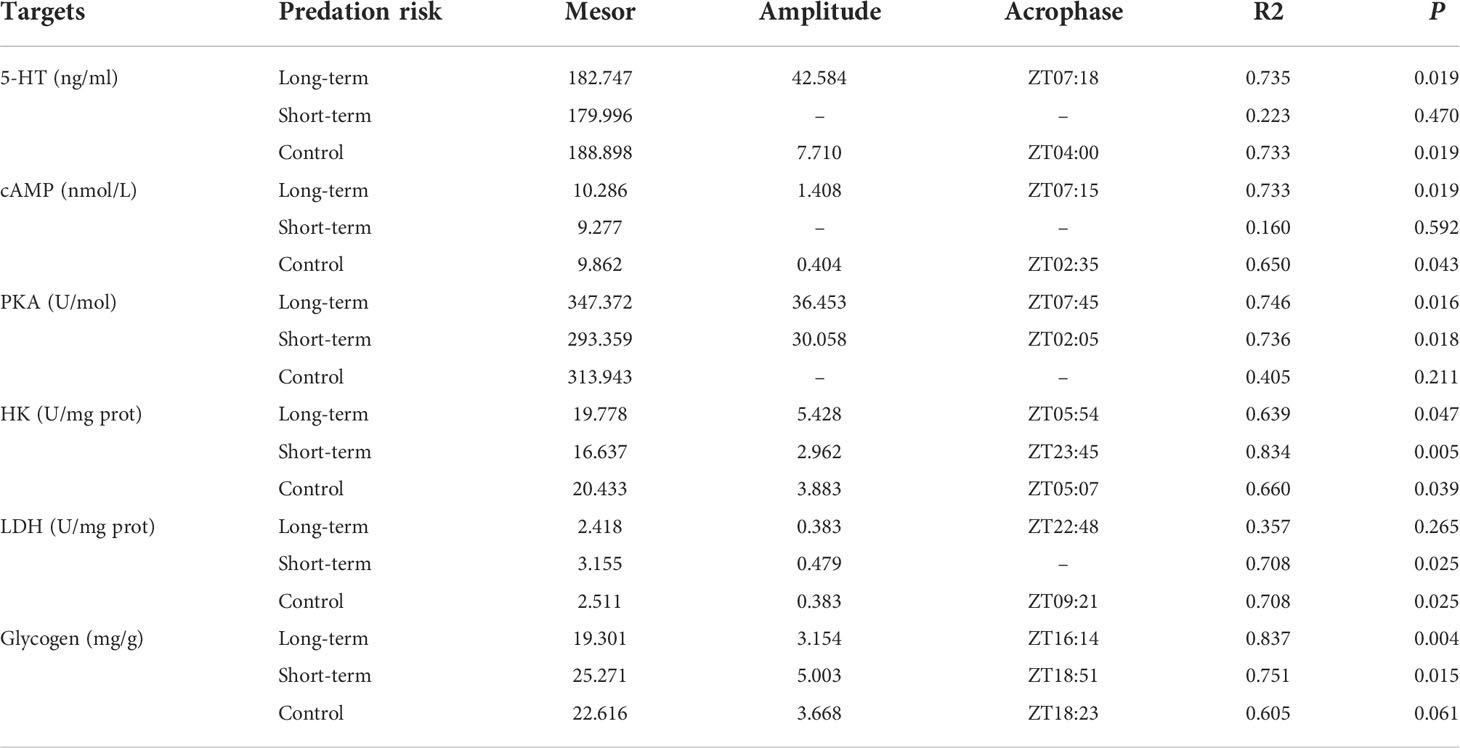
Table 5 Cosinor analysis results obtained based on the concentrations of hormones and glycogen, and enzyme activities.
The HK activity was significantly lower in the short-term predation risk group than the control group, but not significantly different between the long-term predation risk group and control group (Figure 3D). The LDH activity was significantly higher in the short-term predation risk group than those in the control group and long-term predation risk group (Figure 3E, P< 0.05). At ZT4, ZT8, and ZT12, the LDH activities were significantly higher in the short-term predation risk group than the long-term predation risk group (P< 0.05), but no significant differences were found at other time points. Significant cosine rhythms were identified in terms of the variations in the LDH activities in the control group and long-term predation risk group, but not in the short-term predation risk group. No significant differences in the glycogen contents were found between the short-term predation risk group and control group, but the glycogen content was significantly higher in the short-term predation risk group than the long-term predation risk group (Figure 3F). Significant cosine rhythms were identified in terms of the variations in the glycogen contents in the long-term predation risk and short-term predation risk groups (P< 0.05).
3.3 Expression of rhythm-related genes
The presence of predation risk had significant effects on the expression levels of Clock, Bmal1, 5-HT1A receptor and 5-HT2 receptor (Table 6, P< 0.05) which are significantly (Table 6, P< 0.05) differed at different time points. The expression levels of Clock were significantly lower in the control group than the long-term predation risk group, but significantly higher in the control group than the short-term predation risk group (Figure 4A). Compared with the control group, the presence of predation risk significantly reduced the expression levels of Bmal1 (Figure 4B). No significant difference in the expression level of Bmal1 was found in the long-term predation risk group and the control group. Except at ZT4 and ZT16, the expression levels of Clock and Bmal1 were significantly lower in the short-term predation risk group compared with the control group, but no significant differences were found at any other time points between these two treatment groups. At ZT0, ZT4, and ZT8, the expression levels of Clock were significantly higher in the long-term predation risk group than the control group. At ZT16 and ZT20, the expression levels of Clock were significantly lower in the long-term predation risk group than the control group. At ZT0, ZT4, and ZT8, the expression levels of Bmal1 were higher in the long-term predation risk group than the control group, but the difference was only significant at ZT4 (P< 0.05). At ZT16 and ZT20, the expression level of Bmal1 was lower in the long-term predation risk group than the control group. The presence of predation risk did not change the rhythmic variations in terms of the expression levels of Clock. The cosine rhythm in terms of the expression levels of Bmal1 was not detected under the short-term predation risk. However, the expression levels of Bmal1 had a significant cosine rhythm under the long-term predation risk (Table 7).
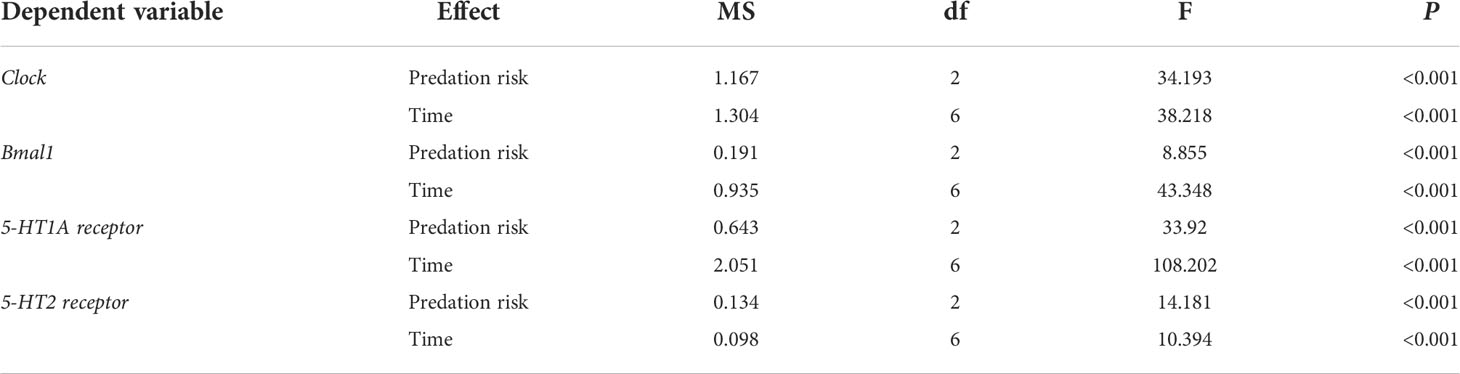
Table 6 Results of the two-way ANOVA conducted to test the effect of different predation risks and times on the expression levels of rhythm-related genes.
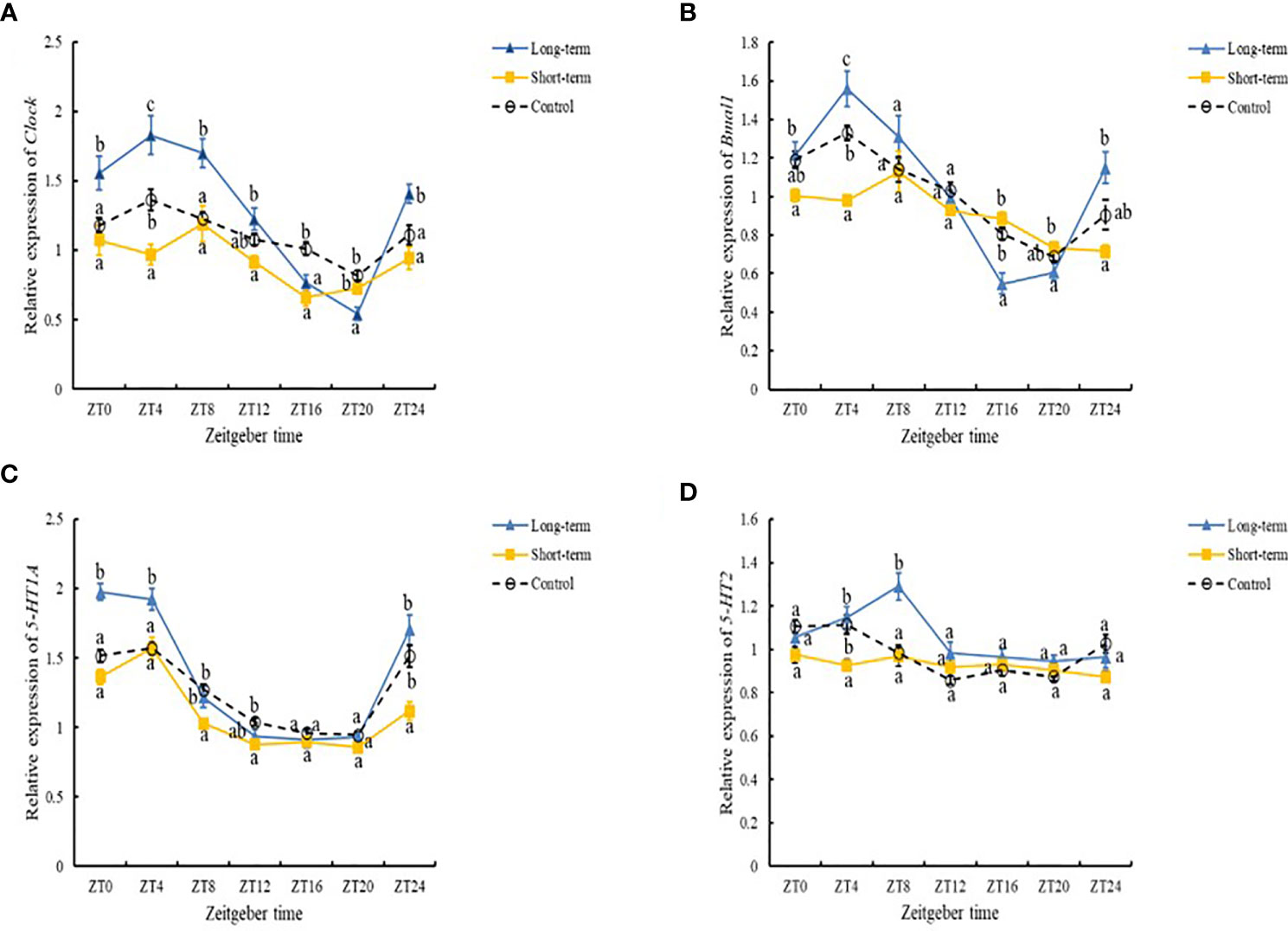
Figure 4 Expression levels of Clock (A), Bmal1 (B), 5-HT1A receptor (C), 5-HT2 receptor (D) in the cerebral ganglion of abalones under different predation risks: Long-term (long-term predation risk), Short-term (short-term predation risk), and Control (no predation risk).
The expression levels of 5-HT1A receptor and 5-HT2 receptor were significantly higher in the control group than the short-term predation risk group, but the expression levels in the control group were significantly lower than those in the long-term predation risk group (Figures 4C, D, P< 0.05). At ZT8, ZT12, and ZT24, the expression levels of 5-HT1A receptor were significantly lower in the short-term predation risk group than the control group, but not significantly different at other time points. At ZT4, the expression level of 5-HT1A was significantly higher in the long-term predation risk group than the control group. Under the long-term predation risk, the peak (ZT02:39) expression levels of 5-HT1A occurred nearly 1 h before those in the control group (ZT03:21) and short-term risk group (ZT03:25). At ZT4, the expression level of 5-HT2 receptor was significantly lower in the short-term predation risk group than the control group, but no significant differences were found at other time points. At ZT8, the expression level of 5-HT2 receptor was significantly higher in the long-term predation risk group than the control group. At ZT4 and ZT8, the expression levels of 5-HT2 were significantly higher in the long-term predation risk group than the short-term predation risk group. The expression levels of 5-HT2 receptor had no significant rhythm under the short-term predation risk (Table 7). Compared with the control group, the peak expression level of 5-HT2 receptor was delayed by 3.5 h in the long-term predation risk group and it occurred at ZT06:37.
3.4 Correlation analysis
In the control group, the cumulative duration of moving had no significant correlation with the 5-HT concentration but it had significant correlations with the variations in the cAMP concentration and the expression levels of 5-HT1A receptor, 5-HT2 receptor, Clock, and Bmal1 (Figure 5, P< 0.05). In the short-term and long-term predation risk groups, significant correlations were not found between the variations in the cumulative duration of moving and cAMP concentration with the expression levels of 5-HT2 receptor, Clock, and Bmal1. In the control group, the mean velocity of moving had significant positive correlations with the variations in the 5-HT concentration and expression levels of 5-HT1A receptor, Clock, and Bmal1 (P< 0.05), In the short-term predation risk group, no correlation was found between the mean velocity of moving and the expression level of Bmal1. In the long-term predation risk group, a significant positive correlation was found between the mean velocity of moving and the expression level of Bmal1 (P< 0.05).
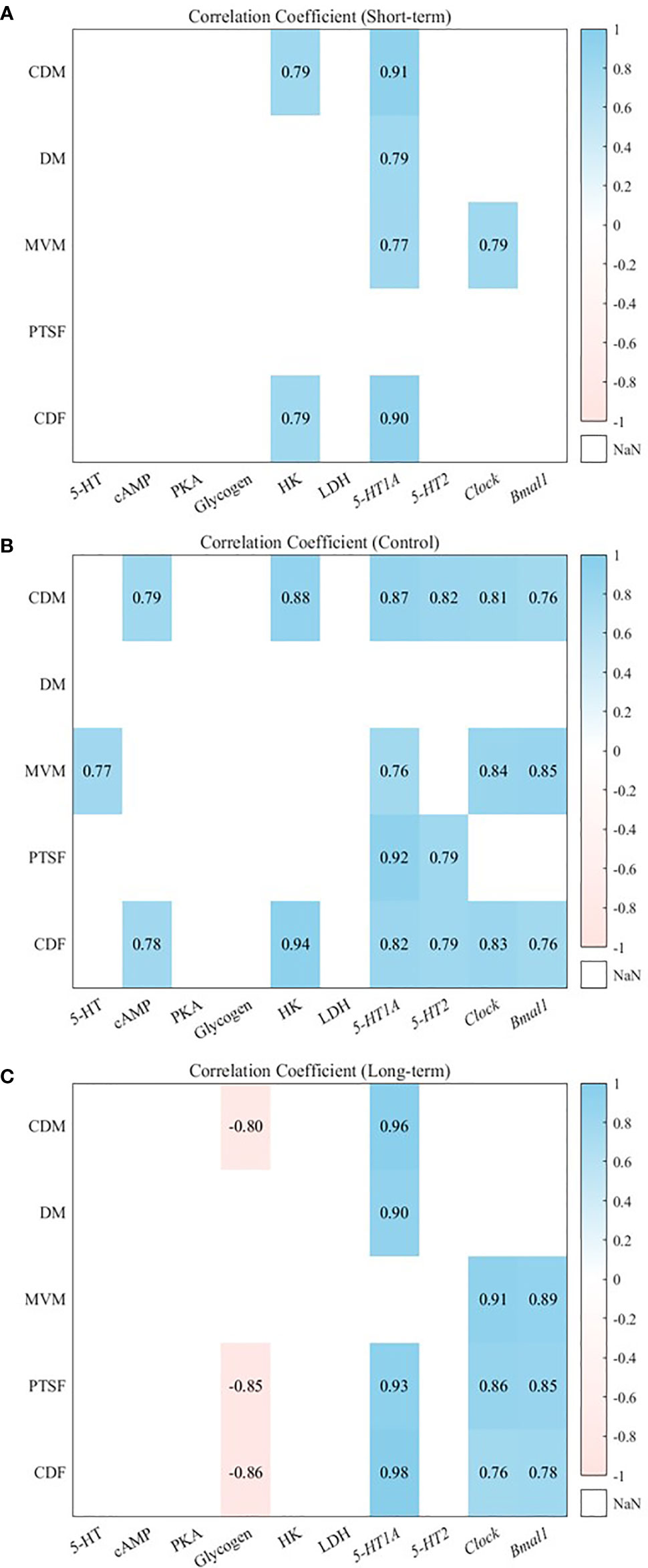
Figure 5 Heat maps showing Pearson correlation between different variables among different groups, long-term predation risk (A), short-term predation risk (B), no predation risk (C). Positive correlations are blue, while negative correlations are pink, P > 0.05 is not listed. CDM: distance of moving; DM: cumulative duration of moving; MVM: mean velocity of moving; PTSF: proportion of time spent feeding; CDF: cumulative duration of feeding.
No significant correlations were found between the percentage of feeding individuals relative to all individuals and the expression levels of Clock and Bmal1 in the control group. However, in the long-term predation risk group, the percentage of feeding individuals relative to all individuals had significant positive correlations with the expression levels of Clock and Bmal1 (Figure 5, P< 0.05). In the control group, the cumulative duration of feeding had significant positive correlations with the variations in the cAMP concentration, HK activity, and expression levels of 5-HT1A receptor and 5-HT2 receptor (P< 0.05). In the short-term predation risk group, no correlations were found between the cumulative duration of feeding and the expression levels of 5-HT2 receptor, Clock, and Bmal1. By contrast, in the long-term predation risk group, the cumulative duration of feeding had significant positive correlations with the variations in the expression levels of Clock and Bmal1 (P< 0.05).
4 Discussion
Predation risk leads to anti-predation strategies in prey. These strategies can then protect prey from predation but they also cause the prey to consume more energy, which adversely impacts their growth and productivity. If the predator persists, the prey must make a trade-off between the advantages and disadvantages of anti-predation behaviors. In the present study, we found that the Pacific abalone displayed responses to the predator in a scenario with adequate food. Under the short-term predation stress, the cumulative duration of feeding and IID decreased significantly in the abalone. A shorter duration of feeding may reduce the likelihood of encountering predators (Thaler et al., 2012; Norin et al., 2021). Under the long-term predation risk, the diel duration of feeding was obviously longer in the abalone, which conforms to the risk allocation hypothesis. Under exposure to predation risk, prey must balance the risk of starvation against the risk of predation due to decreased food intake. Under long-term predation risk, the energy deficit due to decreased food intake will reduce the anti-predation behavior by prey and increase the food intake in order to protect against the risk of starvation (Lima and Bednekoff, 1999). Norin et al. (2021) found that juvenile corkwing wrasse (Symphodus melops) tended to be more active under long-term stress due to predator exposure than short-term predation exposure. We found that the duration of moving and cumulative duration of feeding were significantly higher in the abalone under the long-term predation risk than the short-term predation risk, possibly due to an increased risk of starvation because of reduced energy storage, and thus the behavior of the abalone was “bolder” under the risk of predation. Living in groups (reduced IID) is a response to the predation risk by prey because the per capita predation risk may be inversely related to the size of the group due to the decreased chance of predation (dilution effect), increased vigilance, or predator confusion (Peacor, 2003). Under the risk of predation, changes in the movement and feeding behaviors of the abalone only occurred at night, and little movement occurred during the day, which was probably due to the type of predation risk. Another study showed that after the octopus (Octopus vulgaris) introduced chemical stimuli into an aquarium, the shore crab (Gaetice depressus)moved less in the principal active period (night) but no reduction occurred in the inactive period (daytime). When chemical stimuli were introduced from crushed G. depressus, the activity of the crabs increased in the inactive period whereas the stimuli decreased the activity in the active period (Sakamoto et al., 2006). As a typical nocturnal animal, abalone rarely moves in the daytime and remaining still may be an anti-predation strategy. Clearly, it is also possible to determine whether the abalone exhibit other anti-predation behaviors by changing the type of predator or introducing other chemical signals (such as warning signals), thereby showing whether the presence of a predator would affect anti-predation behavior in the daytime (in the inactive period for abalone).
Predation risk may also lead to changes in the physiological state of prey. We found that the glycogen content and LDH activity were significantly higher under the short-term predation risk than the long-term predation risk. The HK activity was higher under the long-term predation risk than the short-term predation risk, thereby indicating that the abalone exposed to the short-term predation risk mainly depended on anaerobic metabolism to supply energy to the body. The LDH activity is related to the anaerobic metabolism of glucose. Anaerobic glycolysis is a relatively inefficient way of utilizing energy (Lumb, 2017). In the presence of a predator, prey must enhance its aerobic capacity and fully utilize energy in order to improve the usage of energy and enhance the capacity to escape or remain alert (Jermacz et al., 2022). After adapting to the persistent presence of a predator, aerobic metabolism became the principal energy source for the abalone instead of anaerobic metabolism. A previous study also showed that prey can use energy more efficiently by enhancing the aerobic capacity under the long-term predation risk. The duration of movement shortened dramatically in the abalone following the introduction of the predator, thereby indicating that the abalone employed an “energy-saving” strategy in order to reduce energy consumption in response to a high risk situation (e.g., active attack by the predator). In spiny lobster Jasus edwardsii, metabolic rate declined following exposure to predation risk, which may have been due to the adoption of a “stand still” anti-predation strategy against the predator (Briceno et al., 2018). In addition to decreased movement, prey may exhibit a physiological stress response, which increases the respiratory metabolism level and consumes energy in order to remain highly alert (Hawlena and Schmitz, 2010). However, this type of stress response is connected to the “vulnerability” of prey in the presence of predators. Based on comparisons with the body types of prey that are vulnerable to predation, a predator may determine a preferred prey body type for successful feeding, but other prey may then reduce its respiratory oxygen consumption in the presence of the predator because of a decreased predation risk, and thus the predator may favor smaller prey to enhance the success of predation (Karythis et al., 2020). The selection of an anti-predation strategy may depend on the type of predation risk sensed by abalone. In the presence of slow-moving predators such as starfish Coscinasterias calama, abalone may choose to escape, whereas in the presence of predators such as the velvet crab Necora puber, abalone may prefer to stay put and retract the epipodial tentacles beneath the broad shell in order to avoid this type of predation risk (Chauvaud et al., 2021). In the present study, we also found that the abalone preferred to retract beneath their shells in the presence of the predator, thereby giving the crab little chance of success.
5-HT can affect a range of behaviors and physiological responses in fishes, including attacking, spontaneous swimming, feeding, and stress responses. The release and conversion of 5-HT can be induced by various stressors. 5-HT is involved in the regulation of stress, endocrine, and emotional responses in animals by mediating specific receptors (Backstrom and Winberg, 2017). 5-HT signaling leads to changes in the concentrations of intracellular secondary messengers by activating downstream G protein-coupled receptors such as cAMP. The cAMP/PKA signaling pathway is one of the key pathways for glycogen degradation and synthesis, where cAMP can regulate the metabolism of glycogen via the action of protein kinase. Increasing the cAMP concentration will boost the decomposition of glycogen, which then increases the glucose content of muscle cells to the greatest extent possible in order to meet the ongoing energy needs of the body (Yang and Yang, 2016). Under the long-term predation risk, we found that the variations in the 5-HT, cAMP, and PKA concentrations, and the expression levels of 5-HT1A receptor and 5-HT2 receptor had significant cosine rhythms. However, under the short-term predation risk, we found no cosine rhythms in terms of the 5-HT and cAMP contents, and the expression levels of 5-HT2 receptor, thereby indicating that the presence of short-term predation stress led to changes in the physiological state of the abalone, as well as directly affecting the rhythm of their movement behavior (which was confirmed by correlation analysis). Thus, as an exogenous factor, the presence of the predator in the short term triggered a change in the movement peak for the abalone. By contrast, the physiological and behavioral rhythms detected in the long-term predation stress group and control group can be attributed to changes in the light period and regulation by endogenous circadian clock genes resulting in a stable “reciprocating” mechanism.
Biological rhythms comprise intrinsic rhythms in the body and synchronize with the environment, and thus they are affected by changes in the natural environment. The circadian rhythm refers to regular oscillations driven cyclically by the Clock gene and Clock-controlled genes with a cycle of about 24 h, which are evident in physiological, biochemical, behavioral, and other activities. To adapt to cyclical changes in the circadian environment, various animals have evolved biological clocks, which can signal different tissues and organs modulating their adaption to changes in the circadian rhythm (Karatsoreos et al., 2011). Light is a key factor that affects the circadian rhythm, including the neuroendocrine system and neurobehavior, and it has critical effects on the health and well-being of all animals (Tan et al., 2014). In particular, a change in the light period will have an immediate effect on abalone because it is a nocturnal marine animal. The cumulative distance moved and cumulative duration of movement were significantly higher in abalones with a 0 h light:24 h dark photoperiod than 12 h light:12 h dark and 24 h light:0 h dark photoperiods (Gao et al., 2020). CLOCK is a core regulatory protein with specific effects on the circadian rhythm that is expressed in various organs and tissues, where it can catalyze the acetylation of brain and muscle aryl hydrocarbon receptor nuclear translocator-like protein 1 (BMAL1). BMAL1 is a key transcription factor that regulates biological rhythms and it plays a pivotal part in the clock system, where it can bind to the CLOCK protein to form the BMAL1/CLOCK heterodimer, thereby activating the transcription of downstream CLOCK-controlled genes (Trott and Menet, 2018; Zhuang et al., 2019). We found that the expression levels of Clock and Bmal1 were significantly higher under the long-term predation risk than the short-term predation risk. The expression levels of Clock had a cosine rhythm under the long-term or the short-term predation risk, but the expression level of Bmal1 had no cosine rhythm under the short-term predation risk. Correlation analysis also confirmed that the expression levels of Clock were only significantly positively correlated with the mean velocity of moving under the short-term predation risk, and not in the long-term predation risk group and control group. However, the expression levels of Bmal1 were not significantly correlated with any behavioral parameters, thereby suggesting that short-term predation stress reshaped the rhythmic behavior of the abalone, and thus the movement behavior of the abalone exhibited plasticity following abrupt changes in external environmental factors. The predation risk is often used to explain why minnow Rhinichthys cataractae is active at a given time of a day (Culp, 1989). Thus, to maximize the possibility of survival, animals must change their daily activities and rest duration in the presence of predators, where these changes can maintain the energy balance and also reduce the risk of predation (Van Der Vinne et al., 2019). The perch Perca fluviatilis can perceive the circadian rhythms of different predators and regulate its activity depending on the activity levels of predators (Ylonen et al., 2007). The presence of predators also delays the onset time of feeding activity in juvenile plaice Pleuronectes platessa (Burrows et al., 1994). In addition, we found that the variations in the expression levels of Clock and Bmal1 were significantly negatively correlated with change in the glycogen content under the long-term predation risk, but no correlation was detected in the control and short-term predation risk groups. Thus, the abalone changed its mode of energy utilization after adapting to the long-term presence of the predator to make a strategic choice between predation and starvation stress. A previous study also showed that the Clock and Bmal1 genes can participate in the metabolism of nutrients including glycolipids by regulating certain genes, such as glucose transporter and fatty acid synthase, which play pivotal roles in maintaining the normal physiological function of the body (Jha et al., 2015).
In conclusion, prey can respond to the risk of predation by adopting a range of anti-predation behaviors and undergoing physiological changes. Under the short-term predation risk, the abalone reduced their distance that they moved, shortened the duration of movement, intensified the degree of clustering, and enhanced their glycolytic capacity through anaerobic metabolism in order to release additional energy to meet their energy needs. Despite the loss of circadian rhythm in terms of variation in 5-HT and cAMP concentrations, and the expression levels of the 5-HT2 receptor and Bmal1, the movement behavior of the abalone mainly changed at night and their behaviors still had significant cosine rhythms, except that the peaks for the behavioral parameters shifted forward. These findings suggest that short-term predation stress reshaped the characteristic movement behaviors of the abalone. However, the significant positive correlations between the expression levels of endogenous circadian clock genes and movement behavior parameters in the long-term predation risk group and the control group indicate that the rhythmic expression of circadian genes was mainly affected by changes in the light period, which determined the homeostasis of the abalone behavior and circadian rhythm. Therefore, identifying the effects of predation risk on the circadian behavior of abalones will help to understand the origin and evolution of biological rhythm behaviors in marine nocturnal animals, and also provide a reference for developing enhancement and release plans to protect coastal marine ecosystems and benthic biodiversity.
Data availability statement
The datasets presented in this study can be found in online repositories. The names of the repository/repositories and accession number(s) can be found below: https://www.ncbi.nlm.nih.gov/genbank/, MN454859.1.
Author contributions
SL: Data curation, Formal analysis, Investigation, Methodology, Roles/Writing - original draft. XG: Formal analysis, Methodology, Roles/Writing - original draft. MZ: Investigation, Methodology. ML: Investigation, Methodology. CK: Funding acquisition, Project administration, Supervision, Validation. All authors contributed to the article and approved the submitted version.
Funding
This study was supported by grants from the National Natural Science Foundation of China (No. 32273105), National Key R&D Program of China (2018YFD0901400), Key S & T Program of Fujian Province (No. 2020NZ08003), Special Fund for Ocean and Fisheries of Xiamen (No. 21CZY018HJ01), China Agriculture Research System of MOF and MARA, China Postdoctoral Science Foundation Grant [2019M650153, 2021T140393], and the Outstanding Postdoctoral Scholarship from the State Key Laboratory of Marine Environmental Science at Xiamen University. We are also grateful for the support given by the Germplasm Resource-sharing Platform of Aquatic Species in Fujian Province and the XMU-MRB Abalone Research Center.
Acknowledgments
We are also grateful for the support given by the Germplasm Resource-sharing Platform of Aquatic Species in Fujian Province and the XMU-MRB Abalone Research Center.
Conflict of interest
The authors declare that the research was conducted in the absence of any commercial or financial relationships that could be construed as a potential conflict of interest.
Publisher’s note
All claims expressed in this article are solely those of the authors and do not necessarily represent those of their affiliated organizations, or those of the publisher, the editors and the reviewers. Any product that may be evaluated in this article, or claim that may be made by its manufacturer, is not guaranteed or endorsed by the publisher.
References
Arias A., Selander E., Saiz E., Calbet A. (2021). Predator chemical cue effects on the diel feeding behaviour of marine protists. Microbial. Ecol. 82, 356–364. doi: 10.1007/s00248-020-01665-9
Aspe N. M., Cabales R. G., Sajorne R. E., Creencia L. A. (2019). Survey on the predators of abalone haliotis asinina from the perspective of the local fisherfolks in selected sites of palawan, the philippines. J. Shellfish. Res. 38, 463–473. doi: 10.2983/035.038.0230
Backstrom T., Winberg S. (2017). Serotonin coordinates responses to social stress-what we can learn from fish. Front. Neurosci. 11. doi: 10.3389/fnins.2017.00595
Biesinger Z., Bolker B. M., Lindberg W. J. (2011). Predicting local population distributions around a central shelter based on a predation risk-growth trade-off. Ecol. Model. 222, 1448–1455. doi: 10.1016/j.ecolmodel.2011.02.009
Bollens S. M., Stearns D. E. (1992). Predator-induced changes in the diel feeding cycle of a planktonic copepod. J. Exp. Mar. Biol. Ecol. 156, 179–186. doi: 10.1016/0022-0981(92)90244-5
Bradford M. M. (1976). A rapid and sensitive method for the quantitation of microgram quantities of protein utilizing the principle of protein-dye binding. Analytical. Biochem. 72, 248–254. doi: 10.1016/0003-2697(76)90527-3
Briceno F. A., Polymeropoulos E. T., Fitzgibbon Q. P., Dambacher J. M., Peel G. T. (2018). Changes in metabolic rate of spiny lobster under predation risk. Mar. Ecol. Prog. Ser. 598, 71–84. doi: 10.3354/meps12644
Brown J. S., Kotler B. P. (2004). Hazardous duty pay and the foraging cost of predation. Ecol. Lett. 7, 999–1014. doi: 10.1111/j.1461-0248.2004.00661.x
Burrows M. T., Gibson R. N., Maclean A. (1994). Effects of endogenous rhythms and light conditions on foraging and predator-avoidance in juvenile plaice. J. Fish. Biol. 45, 171–180. doi: 10.1006/jfbi.1994.1221
Chauvaud P., Day R., Roussel S. (2021). No evident effect of domestication on the anti-predator behaviour of European abalone (Haliotis tuberculata): Implications for stock enhancement programs. Appl. Anim. Behav. Sci. 244, 105470. doi: 10.1016/j.applanim.2021.105470
Clinchy M., Sheriff M. J., Zanette L. Y. (2013). Predator-induced stress and the ecology of fear. Funct. Ecol. 27, 56–65. doi: 10.1111/1365-2435.12007
Culp J. M. (1989). Nocturnally constrained foraging of a lotic minnow (rhinichthys-cataractae). Can. J. Zoology-Revue. Can. Zoologie. 67, 2008–2012. doi: 10.1139/z89-285
Gao X., Luo X., You W., Ke C. (2020). Circadian movement behaviours and metabolism differences of the pacific abalone Haliotis discus hannai. J. Photochem. Photobiol. B-Biology. 211, 111994. doi: 10.1016/j.jphotobiol.2020.111994
Gao X., Zhang M., Li X., Shi C., Song C., Liu Y. (2016). Effects of LED light quality on the growth, metabolism, and energy budgets of Haliotis discus discus. Aquaculture 453, 31–39. doi: 10.1016/j.aquaculture.2015.11.033
Handelsman C. A., Broder E. D., Dalton C. M., Ruell E. W., Myrick C. A., Reznick D. N., et al. (2013). Predator-induced phenotypic plasticity in metabolism and rate of growth: rapid adaptation to a novel environment. Integr. Comp. Biol. 53, 975–988. doi: 10.1093/icb/ict057
Hawlena D., Schmitz O. J. (2010). Physiological stress as a fundamental mechanism linking predation to ecosystem functioning. Am. Nat. 176, 537–556. doi: 10.1086/656495
Helfman G. S. (1989). Threat-sensitive predator avoidance in damselfish-trumpetfish interactions. Behav. Ecol. Sociobiology. 24, 47–58. doi: 10.1007/bf00300117
Hernandez M. C., Navarro-Castilla A., Wilsterman K., Bentley G. E., Barja I. (2019). When food access is challenging: evidence of wood mice ability to balance energy budget under predation risk and physiological stress reactions. Behav. Ecol. Sociobiology. 73, 145. doi: 10.1007/s00265-019-2756-y
Jermacz L., Kletkiewicz H., Nowakowska A., Dzierzynska-Bialonczyk A., Klimiuk M., Kobak J. (2020). Continuity of chronic predation risk determines changes in prey physiology. Sci. Rep. 10, 8484. doi: 10.1038/s41598-020-64000-9
Jermacz L., Kletkiewicz H., Poznanska-Kakareko M., Klimiuk M., Kobak J. (2022). Chronic predation risk affects prey escape abilities through behavioral and physiological changes. Behav. Ecol. 33, 298–306. doi: 10.1093/beheco/arab142
Jha P. K., Challet E., Kalsbeek A. (2015). Circadian rhythms in glucose and lipid metabolism in nocturnal and diurnal mammals. Mol. Cell. Endocrinol. 418, 74–88. doi: 10.1016/j.mce.2015.01.024
Karatsoreos I. N., Bhagat S., Bloss E. B., Morrison J. H., Mcewen B. S. (2011). Disruption of circadian clocks has ramifications for metabolism, brain, and behavior. Proc. Natl. Acad. Sci. United. States America 108, 1657–1662. doi: 10.1073/pnas.1018375108
Karythis S., Cornwell T. O., Noya L. G., Mccarthy I. D., Whiteley N. M., Jenkins S. R. (2020). Prey vulnerability and predation pressure shape predator-induced changes in O-2 consumption and antipredator behaviour. Anim. Behav. 167, 13–22. doi: 10.1016/j.anbehav.2020.07.009
Kim K. S., Kim M. A., Sohn Y. C. (2019). Molecular characterization, expression analysis, and functional properties of multiple 5-hydroxytryptamine receptors in pacific abalone (Haliotis discushannai). Gen. Comp. Endocrinol. 276, 52–59. doi: 10.1016/j.ygcen.2019.03.001
Lima S. L., Bednekoff P. A. (1999). Temporal variation in danger drives antipredator behavior: The predation risk allocation hypothesis. Am. Nat. 153, 649–659. doi: 10.1086/303202
Lumb A. B. (2017). Nunn's applied respiratory physiology. ed. Lumb A. B. (Italy, Elsevier Ltd) Hypoxia, 327–334.e321. doi: 10.1016B978-0-7020-6294-0.00022-8
Lyu M., Gao X., Zhang M., Lin S., Luo X., You W., et al. (2021). Comparative study of the feeding characteristics and digestion physiology of Haliotis discus hannai and Haliotis gigantea. Front. Mar. Sci. 8. doi: 10.3389/fmars.2021.751401
Macleod R., Gosler A. G., Cresswell W. (2005). Diurnal mass gain strategies and perceived predation risk in the great tit parus major. J. Anim. Ecol. 74, 956–964. doi: 10.1111/j.1365-2656.2005.00993.x
Manriquez P. H., Jara M. E., Gonzalez C. P., Seguel M., Quijon P. A., Widdicombe S., et al. (2021). Effects of artificial light at night and predator cues on foraging and predator avoidance in the keystone inshore mollusc Concholepas concholepas. Environ. pollut. 280, 116895. doi: 10.1016/j.envpol.2021.116895
Matassa C. M., Trussell G. C. (2014). Prey state shapes the effects of temporal variation in predation risk. Proc. R. Soc. B-Biological. Sci. 281, 20141952. doi: 10.1098/rspb.2014.1952
Nelson W., Tong Y. L., Lee J. K., Halberg F. (1979). Methods for cosinor-rhythmometry. Chronobiologia 6, 305–323.
Norin T., Sundin J., Morgan R., Andreassen A. H., Amcoff M., Speers-Roesch B., et al. (2021). Predator presence affects activity patterns but not food consumption or growth of juvenile corkwing wrasse (Symphodus melops). Behav. Ecol. Sociobiology. 75, 14. doi: 10.1007/s00265-020-02947-5
Peacor S. D. (2003). Phenotypic modifications to conspecific density arising from predation risk assessment. Oikos 100, 409–415. doi: 10.1034/j.1600-0706.2003.12043.x
Sakamoto R., Ito A., Wada S. (2006). Combined effect of risk type and activity rhythm on anti-predator response of the shore crab Gaetice depressus (Crustacea : Grapsidae). J. Mar. Biol. Assoc. United. Kingdom. 86, 1401–1405. doi: 10.1017/s0025315406014433
Sheriff M. J., Peacor S. D., Hawlena D., Thaker M. (2020). Non-consumptive predator effects on prey population size: A dearth of evidence. J. Anim. Ecol. 89, 1302–1316. doi: 10.1111/1365-2656.13213
Strobbe F., Mcpeek M. A., De Block M., Stoks R. (2011). Fish predation selects for reduced foraging activity. Behav. Ecol. Sociobiology. 65, 241–247. doi: 10.1007/s00265-010-1032-y
Tan D.-X., Zheng X., Kong J., Manchester L. C., Hardeland R., Kim S. J., et al. (2014). Fundamental issues related to the origin of melatonin and melatonin isomers during evolution: relation to their biological functions. Int. J. Mol. Sci. 15, 15858–15890. doi: 10.3390/ijms150915858
Thaler J. S., Mcart S. H., Kaplan I. (2012). Compensatory mechanisms for ameliorating the fundamental trade-off between predator avoidance and foraging. Proc. Natl. Acad. Sci. United. States America 109, 12075–12080. doi: 10.1073/pnas.1208070109
Tigreros N., Wang E. H., Thaler J. S. (2018). Prey nutritional state drives divergent behavioural and physiological responses to predation risk. Funct. Ecol. 32, 982–989. doi: 10.1111/1365-2435.13046
Trott A. J., Menet J. S. (2018). Regulation of circadian clock transcriptional output by CLOCK: BMAL1. PLoS Genet. 14, e1007156. doi: 10.1371/journal.pgen.1007156
Van Der Vinne V., Tachinardi P., Riede S. J., Akkerman J., Scheepe J., Daan S., et al. (2019). Maximising survival by shifting the daily timing of activity. Ecol. Lett. 22, 2097–2102. doi: 10.1111/ele.13404
Van Dievel M., Janssens L., Stoks R. (2016). Short- and long-term behavioural, physiological and stoichiometric responses to predation risk indicate chronic stress and compensatory mechanisms. Oecologia 181, 347–357. doi: 10.1007/s00442-015-3440-1
Vaze K. M., Sharma V. K. (2013). On the adaptive significance of circadian clocks for their owners. Chronobiology. Int. 30, 413–433. doi: 10.3109/07420528.2012.754457
Yang H., Yang L. (2016). Targeting cAMP/PKA pathway for glycemic control and type 2 diabetes therapy. J. Mol. Endocrinol. 57, R93–R108. doi: 10.1530/jme-15-0316
Ylonen H., Kortet R., Myntti J., Vainikka A. (2007). Predator odor recognition and antipredatory response in fish: does the prey know the predator diel rhythm? Acta Oecologica-International. J. Ecol. 31, 1–7. doi: 10.1016/j.actao.2005.05.007
Keywords: circadian rhythm, feeding behavior, haliotis discus hannai, predation risk, energy metabolism
Citation: Lin S, Gao X, Zhang M, Lyu M and Ke C (2022) Feeding strategy trade-off and selection of marine nocturnal gastropods under predation risk. Front. Mar. Sci. 9:1015076. doi: 10.3389/fmars.2022.1015076
Received: 09 August 2022; Accepted: 20 September 2022;
Published: 05 October 2022.
Edited by:
Menghong Hu, Shanghai Ocean University, ChinaReviewed by:
Harry Gorfine, The University of Melbourne, AustraliaGeng Qin, South China Sea Institute of Oceanology (CAS), China
Dong Zhang, East China Sea Fisheries Research Institute (CAFS), China
Copyright © 2022 Lin, Gao, Zhang, Lyu and Ke. This is an open-access article distributed under the terms of the Creative Commons Attribution License (CC BY). The use, distribution or reproduction in other forums is permitted, provided the original author(s) and the copyright owner(s) are credited and that the original publication in this journal is cited, in accordance with accepted academic practice. No use, distribution or reproduction is permitted which does not comply with these terms.
*Correspondence: Xiaolong Gao, eGxnYW9AeG11LmVkdS5jbg==; Caihuan Ke, Y2hrZUB4bXUuZWR1LmNu