- 1Chinese Academy of Science (CAS) Key Laboratory of Marine Ecology and Environmental Sciences, Institute of Oceanology, Chinese Academy of Sciences, Qingdao, China
- 2Laboratory of Marine Ecology and Environmental Science, Qingdao National Laboratory for Marine Science and Technology, Qingdao, China
- 3University of Chinese Academy of Sciences, Beijing, China
- 4Center for Ocean Mega-Science, Chinese Academy of Sciences, Qingdao, China
Red tide is a kind of marine disaster caused by the accumulation or proliferation of microalgae and other organisms in a short period of time, and utilizing modified clay to control and inhibit red tide is the preferred method. Among them, the application potential of organic-modified clay is high; unlike inorganic and microbial modifications, it has a broad-spectrum removal capacity on red tide algae at extremely low dosages. However, it has some disadvantages such as severe toxicity and high residual turbidity, leading to several limitations in its practical application. Therefore, it has become urgent to select organic-modified reagents with higher efficiency, weaker toxicity and lower residual turbidity. In this study, the typical red tide alga——Prorocentrum donghaiense was selected to detect the removal capacity of Polydimethyl diallyl ammonium chloride (PDMDAAC) modified clay (MP) by comparing with the Hexadecyl trimethyl ammonium bromide (HDTMA) modified clay (MH). Not only the physiological stress and flocculation effects of two modified clays on microalgae had been discussed, but also the properties of the modified clays had been characterized in this study. The results showed that the low degree of oxidative stress and less damage to the cell membrane make MP more environmentally friendly, PDMDAAC can remove microalgae at a low dose (2 mg/L) and quickly clarify the water by significantly enhancing the flocculation capacity of clay. In addition to discussing the removal mechanism of two modified clays on microalgae, schematic diagrams of the pathways were drafted. This study will provide support for the development of organic-modified clay.
1 Introduction
Red tide is mainly caused by the proliferation of microalgae and other organisms within a short period of time, which can inhibit marine organisms, endanger ecosystems and compromise human security (Anderson, 2009; Lee et al., 2013; NOAA, 2016). Therefore, the effective prevention and emergency control of red tide has become a hotspot. Currently, utilizing clay minerals to control and inhibit red tide is a common measure. Multinational scholars from China (Cao et al., 2006; Liu et al., 2016; Yu et al., 2017; Wu et al., 2020), Japan (Shirota, 1989), South Korea (Na et al., 1996; Seger et al., 2017), the United States (Sengco and Anderson, 2004; Sengco et al., 2005), Saudi Arabia (Alshahri et al., 2021) and other countries have promoted the development of this method. However, due to the disadvantages of poor sol properties and low removal efficiency of clay minerals, in practical applications, due to the large amount of clay, the amount of sludge is high (Na et al., 1996). Therefore, scholars have developed inorganic or organic reagents, such as polyaluminum chloride (PAC), Aluminum sulfate, hexadecyl trimethyl ammonium bromide (HDTMA), and ferrate, to modifyclay, which greatly improved the removal efficiency of microalgae (Sengco et al., 2005; Cao et al., 2006; Liu et al., 2016; Yu et al., 2017; Alshahri et al., 2021).
Through the above studies, we found that inorganic modification can significantly improve the flocculation capacity of clay, while organically modified reagents tend to sterilize algae (Cao et al., 2006; Yu et al., 2017). Organically modified reagents are widely used in the control of red tides, and they always have a broad-spectrum removal capacity on microalgae at extremely low dosages (Cao et al., 2006; Zhang, 2016; Wu et al., 2020). Generally, due to its severe sterilization, organic-modified reagents always have a high toxicity, and the 48 h-LC50 of HDTMA on Penaeus japonicus was 1.482 mg/L (Cao et al., 2006), so there are several limitations in practical applications. Scholars have paid increasing attention to the sterilization effect of organic reagents, but have ignored the flocculation efficiency. Therefore, most organic-modified clays have defects such as slow floc sedimentation and high turbidity. Therefore, it has become an urgent demand to select organic-modified reagents with environmental friendliness and well promoted flocculation capacity. Polydimethyl diallyl ammonium chloride (PDMDAAC) is a cationic surfactant, that is widely used in water treatment (Li et al., 2018; Xin et al., 2015; Zhang et al., 2019). In the previous experiments of this study, it was found that PDMDAAC has a relatively high synergistic effect on clay, which can effectively remove Prorocentrum donghaiense in modified clay with a low dose (≤2 mg/L), and can rapidly flocculate and sediment microalgae, which can clarify the solution in 3 h. Different from that of the organic-modified reagents utilized in previous researches, the 96h-LC50 of PDMDAAC on juveniles of Penaeus vannamei is as high as 364 mg/L, which means PDMDAAC is an environmentally friendly algaecide.
This study speculates that the mechanism by which PDMDAAC inhibits algae is different from that of algaecides in previous researches. Therefore, this study utilized PDMDAAC to modify kaolin, explored the removal performance and mechanism of PDMDAAC modified clay, by observing the physiological indicators of microalgae, flocculation parameters and the property characterization of modified clay. This study will provide support for the development of organic-modified clay.
2 Materials and methods
2.1 Preparation of experimental reagents and modified clay
The clay used in the experiment was kaolin, produced in Indonesia. PDMDAAC was obtained from Aladdin, and the relative molecular mass was 100, 000, in a 35% aqueous solution. HDTMA was obtained from Aladdin, and was analytically pure. In the experiment, the PDMDAAC-modified clay was recorded as MP, HDTMA-modified clay was recorded as MH. Two kinds of modified clays were manufactured according to the mass ratio of clay: PDMDAAC or HDTMA of 200: 1, 2 or 5, recorded as MP1 or MH1, MP2 or MH2, and MP5 or MH5, respectively. The dose of modified clay was set to 0.2 g/L and the samples were prepared as aqueous solutions before use.
2.2 Modified material safety assessment
In this study, P. vannamei (approximately 11.91 mm in length and 26.28 mg in weight) was chosen as the test organism, and a 96-h acute toxicity test was conducted to evaluate the safety of PDMDAAC. PDMDAAC concentration gradients were set to 0, 20, 40, 80, 120, 200, 400, 800 mg/L. After domestication and cultivation, 20 P. vannamei were randomly selected and placed in a 5 L beaker containing sterilized seawater. PDMDAAC was added according to the dose above. Three parallels experimental groups were conducted, and dead individuals were counted and removed every 24 h, continuous experiment for 96h. After plotting the viability table, 96h-LC50 was calculated by linear interpolation.
2.3 Microalgal culture and treatment system
P. donghaiense was isolated from the Changjiang Estuary, which was kindly provided by Key Laboratory of Marine Ecology and Environmental Sciences, Institute of Oceanology, Chinese Academy of Sciences. The natural seawater used in this experiment was taken from the coastal waters of Qingdao, filtered through a 0.45 μm cellulose acetate membrane (Shanghai, Xinya) before the experiment, and used after sterilization. The salinity and pH of seawater were 31 ± 1 and 8.00 ± 0.05 respectively. Inoculated an appropriate amount of P. donghaiense into a 5 L conical flask containing L1 cultural medium (Guillard and Hargraves, 1993), the initial density was 1×104 cells/mL, the culture temperature was 20 ± 1°C, the light intensity was 65 μmol photons m−2 s−1, and the light-dark cycle was 12 h: 12 h. Experiments were performed when cultured to the later stage of exponential growth.
1) The microalgae removal experiment was carried out in 50 mL colorimetric tubes. After adding the modifier or modified clay according to the corresponding amount, the tubes were inverted and mixed three times, until sampling after 3 h. Three parallel experimental groups were conducted, and a blank control group was used. A chlorophyll in vivo fluorescence detector (Trilogy, Turner Designs, US) was used to measure viable microalgae density after treatment and calculate the removal rate of microalgae according to the following formula:
2) The determination of the reactive oxygen species (ROS) was carried out in a 1 L beaker. The modified clay was added to the algae solution according to the corresponding amount, mixed well and sampled after 3 h. Three parallels experimental groups were conducted, and a blank control group was set. The microalgae in supernatant were collected by filtration through a GF/D membrane. Then, a cell disruptor was used to crush the cells (5.5 m/s, 30 s, 2 times) in an ice-bath environment, and the supernatant was extracted by centrifugation (5000 rpm, 10 min), which was the crude enzyme solution. Then use the crude enzyme solution obtained by the above method to measure the indicators including the protein content (BCA method), superoxide dismutase (SOD, WST-1 method) activity, catalase (CAT, ammonium molybdate method) activity, peroxidase (POD, colorimetry method) activity and malondialdehyde (MDA) content, which were determined with the assay kits purchase from Jiancheng (Nanjing) by a microplate reader (EnSight, Perkinelmer, US). Three parallel experimental groups were conducted, and a blank control group was used.
3) Trypan blue (C34H24N6Na4O14S4) is a kind of nucleic acid stain, that can be applied to detect the integrity of cell membranes. In this part of experiment, the proportion of modified clay reduced to clay: modified reagent of 200: 0.2, 0.5, 0.8 or 1 (mass ratio), the naming method of each group was similar as section 2.1, due to the low density of residual microalgae. The membrane stability test of microalgae was carried out in a 50mL colorimetric tube. After adding the modified clay according to the corresponding amount, the tube was inverted and mixed for three times. Three parallel experimental groups were conducted, and a blank control group was used. The supernatant was sampled at 3 h and stained and unstained cells were counted under an inverted microscope. The ratio of unstained cells was defined as cell activity in this study.
2.4 Modified clay flocculation experiment
2.4.1 Static flocculation experiment
The flocculation process of P. donghaiense by the modified clay under static conditions was observed by Particle Image Velocimetry (PIV, Flow Master, LaVision, Germany) The laser was used to illuminate a particular layer of flocs in the quartz tank, and a high-resolution image was obtained with a high-speed charge-coupled device (CCD) camera. The image was analyzed by using Davis 8.40 software (LaVision, Germany) to obtain the particle size and number of flocs. Experiments were carried out in 50ml quartz tanks, modified clay were added into algae according to the dose of 0.2 g/mL and mixed well. The samples were photographed with a specific time gradient until 180 min. The main indicators were floc particle size (D50) and the particle density. The surface morphology of the flocs was carefully observed by scanning electron microscopy.
2.4.2 Coagulated flocculation experiments
The flocculation process of P. donghaiense with the modified clays and the self- flocculation process of the modified clays under coagulation conditions were observed by PIV. The measurement protocol is described in Section 2.4.1. Experiments were carried out in 100 ml quartz dishes; modified clay were added into algae according to the dose and mixed well. By adjusting the stirring speed, divide the experimental process into the growth phase (50 rpm, 15 min) - breakage phase (150 rpm, 10 min) - regrowth phase (50 rpm, 20 min). The main indicators (Wu et al., 2020): ①the floc particle size (D50); ②particle density; ③fractal dimension; ④ flocculation speed (sg): sg = dt1/tg; ⑤ floc recovery factor (Rf): Rf = 100% × (dt3 – dt2) / (dt2 – dt1); ⑤ floc strength (M): M = 100 × dt2 / dt1. The M indicates the ability of flocs to resist rupture by a certain velocity gradient and the capacity of flocs to regrow is evaluated by the Rf (Jarvis et al., 2005; Zhao et al., 2011).
2.5 Characterization of modified clay
The zeta potentials of the samples were measured by a Malvern nanoparticle potentiometer (Zetasizer, Malvern, UK). The apparatus used for FTIR spectroscopy was a Nicolet FTIR-740 spectrometer. The mineral sample ground to −5 μm was put into a beaker with a certain amount of polymer agents according to the experimental conditions and the suspension was stirred for 20 min. After washing and filtration, the filter cake was dried at 50°C in a vacuum drying oven. Finally, the infrared spectra of the mineral samples and these reagents were determined by this spectrometer. Scanning electron microscopy (SEM) images were taken by using a Japan Hitachi S-3400N. The powdered samples were first affixed onto adhesive tapes supported on metallic disks and then covered with a thin, electrically conductive gold film. Images were recorded at different magnifications (200–7000×) at an operating voltage of 5.0 kV. ①The flocs for SEM was collected in the bottom of the vessel in microalgae removal experiment, and freeze-dried for 48 h, the samples were obtained; ② The modified clay samples were dried at 60°C for 12 h, and ground into powder prior to SEM analysis.
2.6 Data statistics and analysis
Origin 2018 (OriginPro 2018C, Origin Lab, US) and Microsoft Excel (Microsoft® Excel® 2019MSO, Microsoft, US) were used to generate graphs, and data are expressed as the mean ± standard deviation (mean ± SD); Statistical analysis of the data was performed using one-way ANOVA and the independent samples T test in SPSS 19.0 (IBM SPSS Statistics 19, IBM, US), P<0.05 indicated that the difference was significant, and P<0.01 indicated that the difference was extremely significant.
3 Results
3.1 Modified material safety assessment
A 96h-acute toxicity experiment of PDMDAAC on juveniles of P. vannamei was carried out to evaluate its ecological safety (Table 1). The 96 h-LC50 can be calculated by the linear interpolation method to be approximately 364.96 mg/L. The safe concentration of PDMDAAC was determined to be 36.45 mg/L and subsequent experimental concentrations were based on the above results.
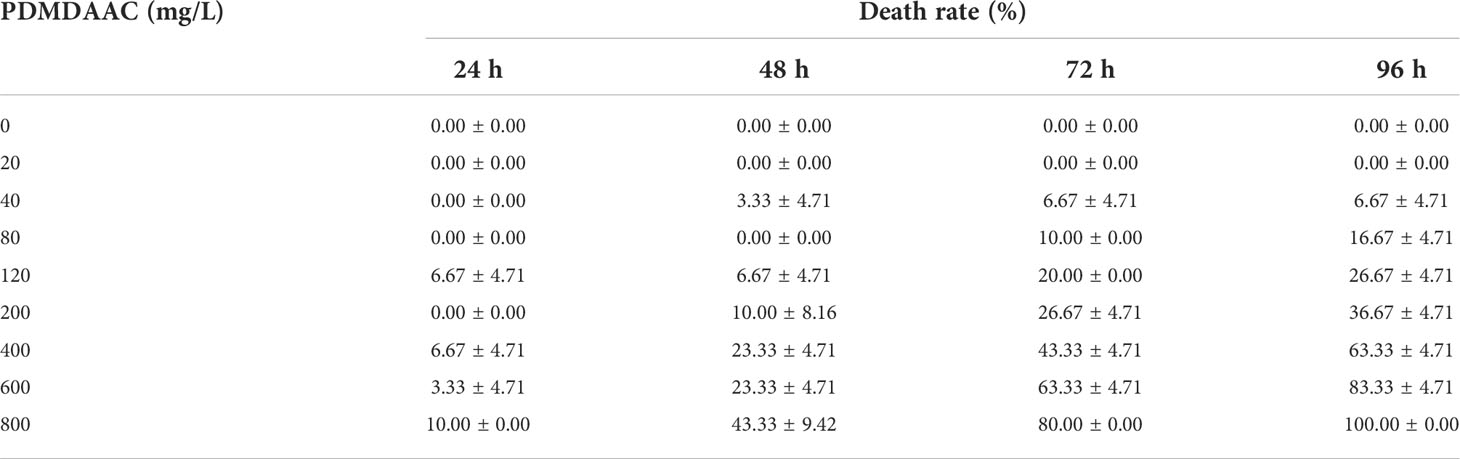
Table 1 96h acute toxicity test results of Poly dimethyl diallyl ammonium chloride (PDMDAAC) on juveniles of P. vannamei.
3.2 Removal performance of modified clay on microalgae
The removal ability of P. donghaiense by kaolin was weak, and the removal rate was only approximately 20% when the dose was 0.2 g/L (Figure 1). PDMDAA had a significant synergistic effect on kaolin (P<0.05). MP can significantly reduce the algal density rapidly (3 h), even the removal rate of the lowest concentration group (MP1) reached approximately 50%, and gradually surpassing 95% removal, the removal effect can be maintained up to 24 h. The treatments of microalgae by HDTMA modified clay had a similar trend to the MP group, and the removal rate of each group was slightly improved at 24 h.
3.3 Physiological stress of microalgae
After adding the two modified clays, the activities of the two antioxidant enzymes (SOD and CAT) in microalgae were significantly promoted (P<0.05), indicating that these two modified clays could inhibit the microalgae by producing ROS stress (Figure 2A). The activities of the three antioxidant enzymes in the MH group were higher than those in the MP group, and the enzyme activities showed a more significant gradient growth. PDMDAAC had a weak induction of POD, and only the highest concentration group (MP5) significantly increased (P<0.05). The MDA content had a positive correlation with the degree of lipid peroxidation, and both modified clays can increase the MDA content of the microalgae (Figure 2B). However, microalgae in the MH group experienced higher lipid peroxidation than those in the control group, and the MDA content in MP5 was only 68.8% of that in MH2 (Figure 2B). In conclusion, MH led to severe peroxidative stress of P. donghaiense and induce a higher degree of lipid peroxidation than MP.
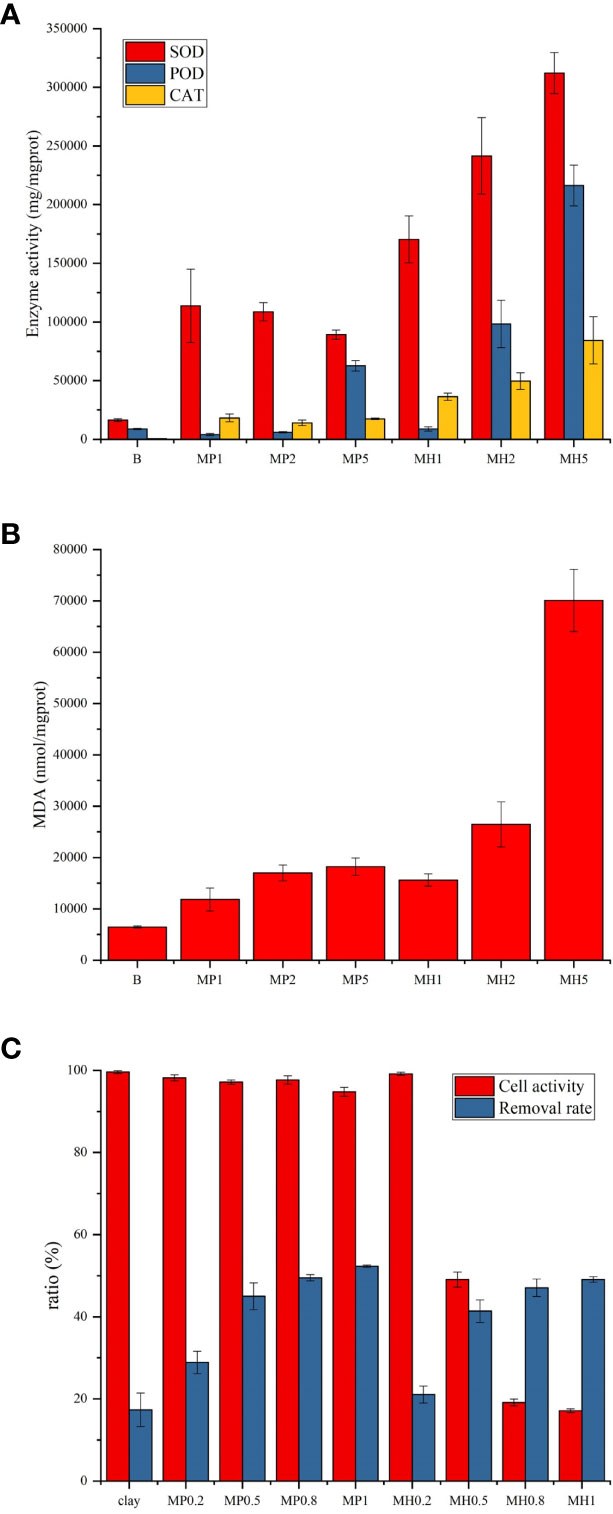
Figure 2 Effects of two modified clays on antioxidant enzyme activity, MDA and cell activity in P. donghaiense (A–C).
The removal ability of P. donghaiense by kaolin was slightly increased after modification with a lower dose of PDMDAAC and HDTMA (Figure 2C). The microalgae of the MP group were almost unstained, and 94% of the MP5 cells were healthy. The cell membranes of the MH group lacked integrity, and the cell activity gradually decreased from 99% to 17%. The above results indicated that MH caused a more severe damage to the microalgal membrane, which was consistent with the change in MDA content.
3.4 Discrepancies in the flocculation of modified clays
3.4.1 Flocculation performance under static conditions
The addition of modified clay resulted in a blurred view, and evenly distributed microalgae could still be observed. It could be observed that the microalgae in the MP group began to aggregate at 15 min, and flocs were continuously generated in the following 10 min; the D50 and particle density began to decrease after 25 min (Figure 3), which indicated that the flocs could sediment quickly. Until 90 min, the visual field was dominated by small flocs. There was no significant change of D50 in the MH group among the whole experimental process (Figure 3), and the particles were evenly distributed in the view. The tendency of the particle density in the MH group was similar to that in the MP group, but caused a higher residual turbidity because of the slower sedimentation speed of flocs. In conclusion, MP had a higher flocculation capacity on P. donghaiense, and the flocs hada higher D50 and faster sedimentation speed.
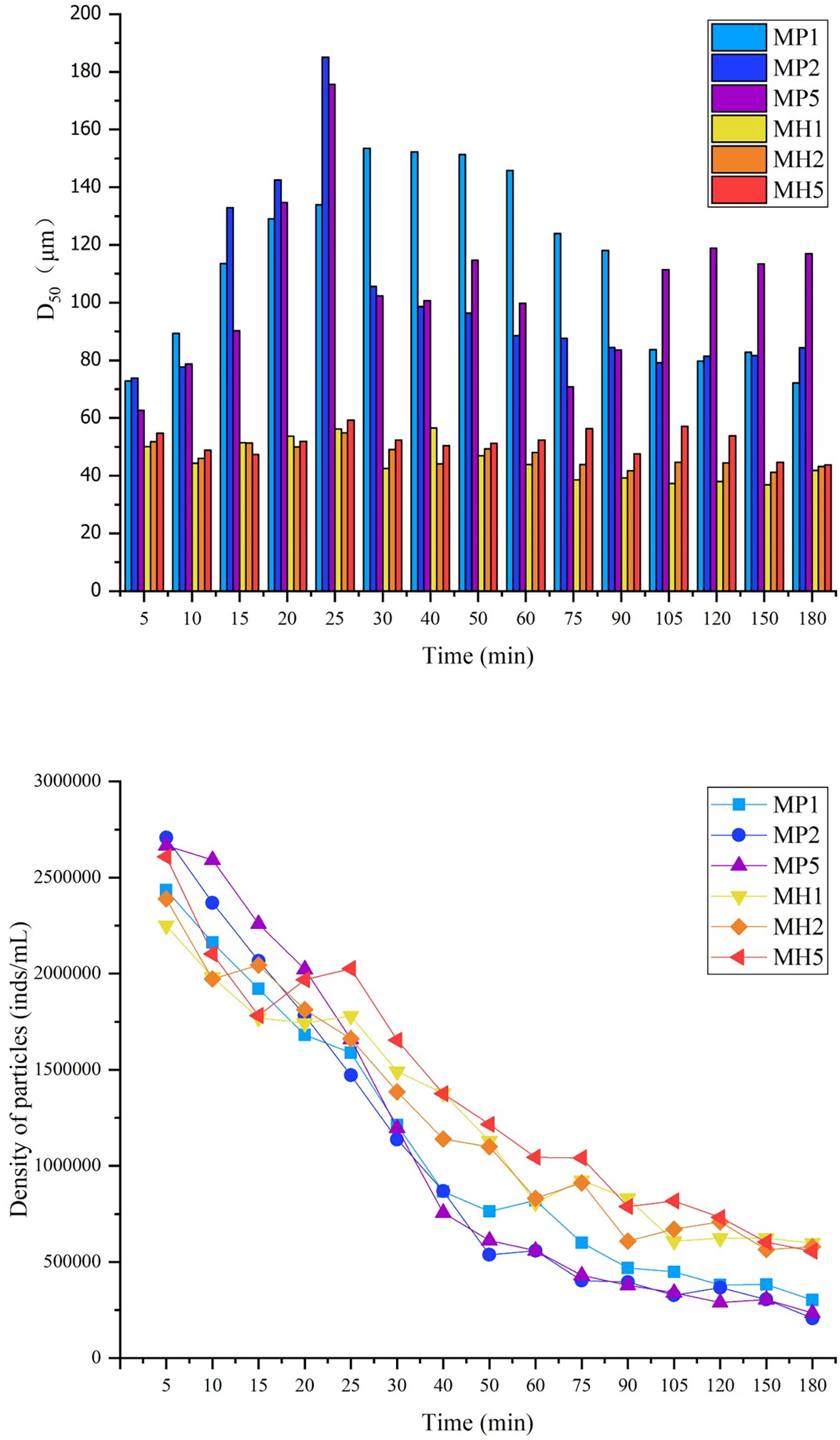
Figure 3 Changes in D50 and particle density during the process of flocculating microalgae with modified clay under static conditions.
3.4.2 The surface morphology of the floc surface
To further explore the differences in flocculation, we photographed the surface images of flocs in section 3.4.1. The kaolin group was dominated by small flocs, and there were many clustered structures attached to the surface of flocs (Figures 4A, B). MP could flocculate microalgae well, even though the lowest concentration group (MP1) mainly consisted of medium-sized flocs (≥200 μm) (Figure 4C). The surface of the flocs was dominated by square-shaped protrusions with rounded edges (Figures 4D–F). These protrusions were arranged in an irregular superimposed manner, causing the flocs to present a relatively three-dimensional spatial structure. With the increase in PDMDAAC addition, the density of such protrusions gradually increased, and microalgae embedded in flocs were observed in each group. The flocculation capacity of MH was weaker, and MH5 was dominated by small flocs with particle sizes between 100-200 μm (Figure 4G). After adjusted the magnification to 7000×, the morphology of the flocs was coated with clustered structures similar in the kaolin group and a small amount of square protrusions; only in the two relatively high concentration groups (MH2&MH5) could embedded microalgae be observed (Figures 4H–J).
3.4.3 Flocculation process under coagulation conditions
Similar to the static flocculation experiment, the vision clarity gradually recovered after the addition of the modified clay, and this process was significantly accelerated under coagulated conditions. This phenomenon caused the particle density of each group to first increase and then decrease from 0 to 15 min (Figure 5). Both modified clays showed a strong flocculation effect, and the MP group clarified faster and had a higher sg than the MH group (Table 2). The higher stirring rate (150 rpm) in the breakage phase greatly improved the growth of flocs, leading to a nearly double increase in D50. Afterwards, due to the rapid sedimentation of large flocs from 25 to 27 min, the particle density and D50 of each group were maintained at a low degree (Figure 5). The M and Rf of the two modified clays were high (Table 2), which indicated that the coagulation system could significantly improve the flocculation capability.
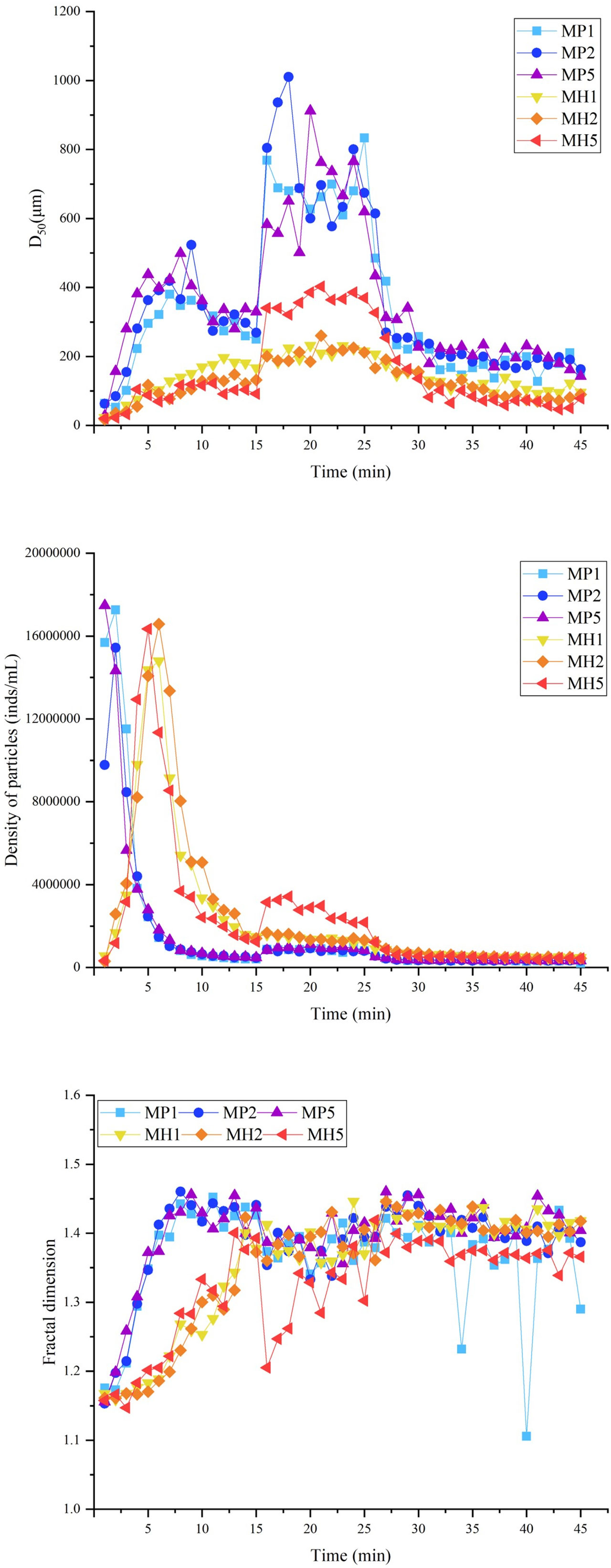
Figure 5 Changes in the D50, particle density and fractal dimension during flocculation of microalgae with modified clay under coagulation conditions.

Table 2 Changes in parameters during flocculation of microalgae with modified clay under coagulation conditions.
The self-flocculation experiment showed that, the D50 and fractal dimension of the MH group were higher than those of the MP group (Figure 6), which indicated that HDTMA could combine with clay to generate large and compact flocs. The flocs produced by self-flocculation had a low strength (Table 3), which was reflected in the significant changes in the floc size and particle density when the stirring speed increased to 150 rpm. After 35 min, the D50 and fractal dimension of the MH group decreased significantly, which might be caused by floc sedimentation. The self-flocculation capability of MP was slightly weaker, and MP was greatly affected by high-speed stirring, causing the wild fluctuations of the three parameters in Figure 6. However, the flocs formed by MP had higher recovery rates between 100-181 (Table 3), which helped the floc size to improve quickly.
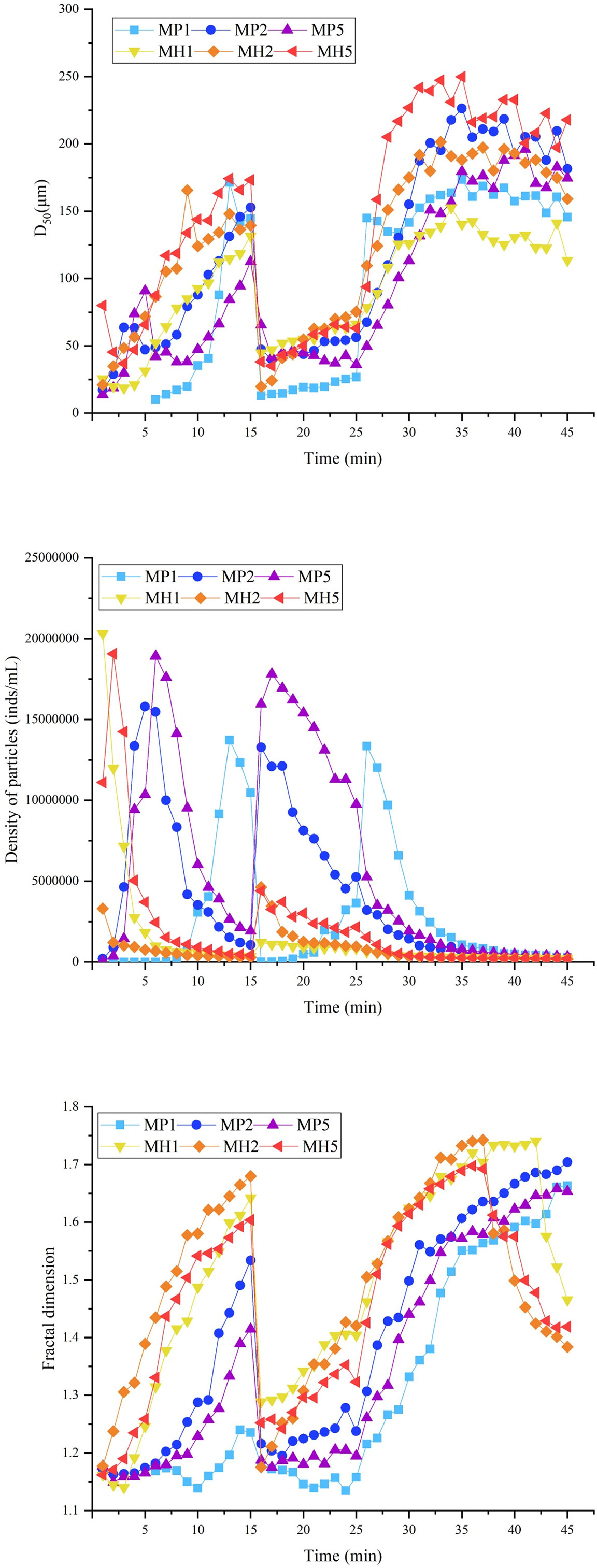
Figure 6 Changes in the D50, particle density and fractal dimension during self-flocculation of modified clay under coagulation conditions.

Table 3 Changes in parameters during self-flocculation of modified clay under coagulation conditions.
3.5 Characterization of modified materials
3.5.1 Zeta potential of modified clay in different media
The results showed that Indonesian kaolin exhibited strong electronegativity (-10 mV) in pure water (Figure 7). Both modification methods significantly increased the zeta potential of kaolin, MP was more positive than MH, and the zeta potential of the three concentration groups reached 26.30, 37.00, and 44.80 mV (Figure 7). The zeta potential elevating effect of MH was weak, and the zeta potential of each concentration was lower than that of the former samples.
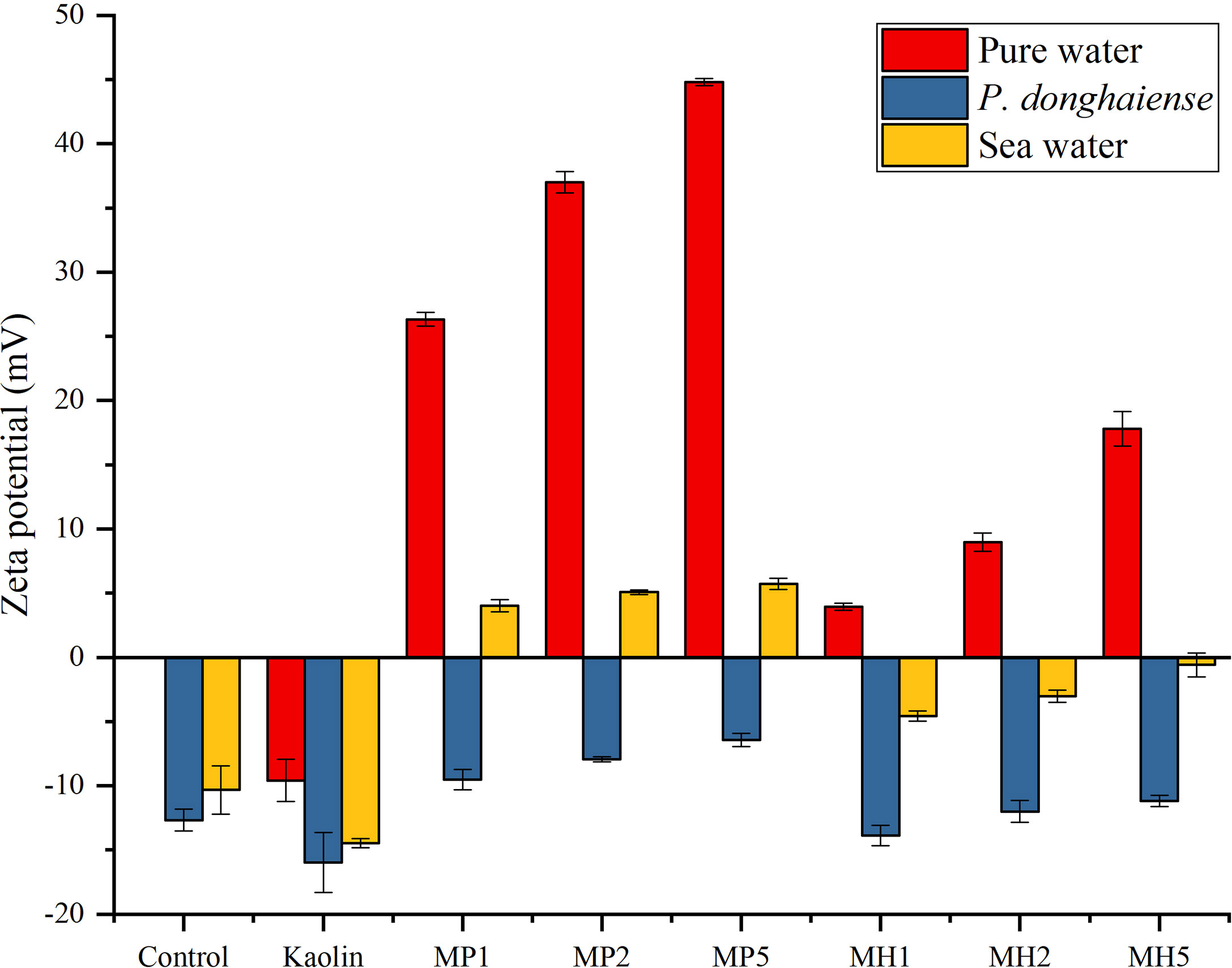
Figure 7 Effects of two modified clays on the zeta potential of pure water, P. donghaiense and natural seawater.
Zeta potential can be applied to evaluate the physical stability of the particle dispersion system. The lower the absolute value of the zeta potential, the weaker the electrostatic repulsion is between particles and the easier the aggregation or agglomeration. The addition of kaolin to the microalgae would reduce the potential to approximately -16.00 mV (Figure 7). The repulsion between particles was strong under these conditions, which proved the weak flocculation ability of kaolin to microalgae. The PDMDAAC modified clay had a strong positive charge, which could significantly increase the zeta potential. The improvements in zeta potential of the MH group were weak, and MH5 had no significant difference from P. donghaiense, which could be one of the reasons for the observed weaker flocculation of MH than of MP outlined in Section 3.4.1. Aiming at self-flocculation, this study investigated the zeta potential of two modified clays in seawater, and kaolin could further reduce the zeta potential of seawater to -14.50 mV (Figure 7). The potential of the two modified clays increased in steps, and MP could turn the entire solution positively charged. While HDTMA failed to change the electronegativity, however, the absolute value of its zeta potential was low. which could promote particle agglomeration.
3.5.2 The surface morphology of clay
As shown in the SEM images, a dense laminated structure could be observed in Figure 8A, and the surface of the layered structure was mostly covered with a large number of clustered structures (Figure 8B).
Solid PDMDAAC was white and translucent crystalline particles. We observed several holes and fragments attached to the compound surface (Figure 8C). The protuberances were distributed on the surface of the particles in a scale-like pattern, presenting a three-dimensional spatial network structure, and there were rounded protrusions attached to the surface (Figure 8D). PDMDAAC could significantly reduce the clustered structures on the clay surface, and replace them with rounded protrusions. Similar to PDMDAAC, the surface of MP became compact (Figures 8E, F). The above phenomenon showed that PDMDAAC had been successfully loaded on the clay, and the density of such a flaky distribution gradually increased, indicating that the loading of the modified reagents increased gradually.
The surface of HDTMA particles was free of voids and depressions, and the structure was entirely compact and smooth (Figure 8G). It could be observed (Figure 8H) that a large number of sheet-like structures with smooth edges were distributed on the surface of material. HDTMA modification has a certain ability to modify the surface structure of clay. Two high concentration groups (MH2&MH5) were accompanied by a small number of sheet-like protrusions and a higher proportion of clustered structures (Figures 8I, J).
3.5.3 Characterization of the surface functional groups of clay and modified reagents
The surface functional groups of clay and modified reagents were observed by FT-IR spectroscopy (Figure 9). As an inorganic mineral, the characteristic peaks of kaolin are mainly distributed in the far-infrared region (400-33 cm-1). Two weak absorption peaks could be observed above 3000 cm-1, O-H at 3688.62 cm-1 and =C-H at 3619.09 cm-1. The absorption peaks below were all located in the fingerprint region (1300-400 cm-1), 1050 ± 50 cm-1 was generally the stretching vibration peak of -C-O, 910.54 cm-1 was the bending vibration of -OH, and 660 ± 20 cm-1 was the rocking vibration peak of -OH.
The infrared spectrum of PDMDAAC was investigated, and we observed a strong N-H absorption peak at 3361.88 cm-1 and a weaker stretching vibration absorption peak of unsaturated C(sp2)-H at 3010.40 cm-1. The asymmetric stretching vibration peak of -CH2 appeared at 2934.25 cm-1. Corresponding to the two absorption peaks above 3000 cm-1, the bending vibration peak of N-H appeared at 1630.20 cm-1and the out-of-plane twist vibration peak of =CH2 appeared at 988.84 cm-1.
The infrared spectrum of HDTMA is shown in Figure 9. We found that 3016.90 cm-1 is the weak stretching vibration peak of C-H, 2915.52 cm-1 is the asymmetric stretching vibration peak of -CH2, and 2848.09 cm-1 is the symmetric stretching vibration peak of -CH2. The absorption peaks in the interval of 1500-1400 cm-1 were mostly the bending vibration region of the group, such as the scissor vibration peak of -CH2 at 1462.05 cm-1, the bending vibration peak of C-N at 1430.74 cm-1, and the symmetric bending vibration peak of CH3 at 1382.50 cm-1. In the fingerprint region, 1000-650 cm-1 was the out-of-plane bending vibration region of unsaturated C-H, 910.97 and 829.75 cm-1 represented the C-N absorption peaks, and the peak at 720 ± 10 cm-1 should be the rocking vibration of -CH2.
4 Discussion
Through microalgae removal experiments, this study found that PDMDAAC has a significantly synergistic effect on kaolin and can quickly remove P. donghaiense at a relatively low dose. Considering the practical application, this study conducted toxicological experiments and found that PDMDAAC is environmentally friendly, which is contrary to the high toxicity of the organic-modified reagents used in previous researches. Therefore, this study hypothesized and explored the removal mechanism of PDMDAAC, with the physiological indicators, flocculation parameters and modified clay characterization.
4.1 The flocculation mechanism of modified clay on microalgae
The sterilization ability of Indonesian kaolin clay against P. donghaiense was weak, and the removal rate was approximately 20% when the dosage was 0.2 g/L, and no microalgae were observed on flocs. The addition of clay reduced the zeta potential of the microalgal solution from -12.7 mV to approximately -16.0 mV. The repulsion between particles was intensive under this condition, indicating that particles do not easily to agglomerate. This should be one of the reasons why it is difficult for kaolin to flocculate microalgae. The synergistic ability of PDMDAAC to clay was remarkable, and the removal rate of microalgae in the MP2 group reached 90% with a lower residual turbidity after treatment. The flocculation experiment could intuitively explain the above phenomenon. The MP could significantly reduce the absolute value of the zeta potential in solution; that is, adding MP to the algae liquid could provide a suitable environment for particle aggregation and agglomeration, and promote flocculation. The electron microscopic images showed that PDMDAAC can be loaded on the surface of kaolin. The flocs of the MP group also retained more sheet-like protrusions similar to MP, which enabled the flocs to electrostatically tract the microalgae, and the spatial net structure helped the flocs to further sweep and capture microalgae. This prompted the microalgae to aggregate and form flocs quickly, which manifested as a rapid decrease in particle density and an increase in D50 in the experimental parameters. The higher agglomeration and sedimentation capacity of flocs jointly accelerated the clarification of the solution.
Among the MH group, the average distribution of microalgae and small flocs could be observed during the whole experiment. Due to the low sedimentation speed, the solution turbidity was still high until 180 min. This phenomenon could be due to the inappropriate zeta potential of the solution, and the intense repulsion between particles obstructs MH flocculation of microalgae. Through SEM images, we found that HDTMA did not modify the clay surface as much as PDMDAAC, which weakened the electrostatic traction effect of modified clay. The floc particle size of MH5 was approximately 50 μm, which was consistent with the PIV observation (180 min-D50 = 43.8 μm). The surface of the floc had no kaolin, and no attached modified structure, which may be one of the reasons for its weak floc growth, and only a small number of microalgae can be observed in the two high concentration groups.
Red tides often break out in coastal areas, estuaries, etc. Wind, ocean currents and thermal cycles maintain the environmental dynamics, and these disturbances will inevitably affect the flocculation. Appropriate physical disturbance can increase the collision frequency of particles in the dispersion system, thereby improving the flocculation efficiency, making it obvious to identify differences in flocculation characteristics. Therefore, the flocculation ability of modified clay under coagulation conditions was observed in this study. The flocculation efficiency of MP on microalgae increased with increasing stirring speed, and D50 increased significantly at 150 rpm. The coagulation conditions improved the flocculation effect of MH on microalgae, and the flocs could also resist the shear force caused by a high stirring speed, but the flocculation parameters such as D50 and particle density reduction rate were lower than those of MP. The binding force between MP and microalgae was tough, and this force should be related to the PDMDAAC loaded on the modified clay.
In addition to the flocculation of microalgae, there is also an interaction between modified clay particles, called self-flocculation. Studies have shown that powerful self-flocculation impacts the algae removal efficiency of modified clays (Jiang et al., 2021). In seawater under coagulation conditions, the D50 and fractal dimension of the MH group were both higher, which indicated that there was a powerful binding force between HDTMA and clay. Additionally, this study found that the zeta potential of kaolin in seawater was -14.5 mV, and MH had an appropriate prompt effect on the zeta potential, which could significantly reduce the repulsion between particles in the solution. The strong self-flocculation ability of MH should be due to the moderate stirring rate and the zeta potential, the former can significantly improve the collision efficiency of particles, and the latter may reduce the repulsion between particles.
4.2 Environmental friendliness mechanism of PDMDAAC modified clay
Quaternary ammonium salts (QACs) are a kind of sterilant, that are widely used in water disinfection, biofilm inhibition and other fields (Andrzej et al., 2017; Pang et al., 2020; Zhang et al., 2021), Some kinds of QACs can notably promote the disinfectant properties of clay (Cao et al., 2006; Zhang, 2016; Wu et al., 2020). It is well known that the sterilization mechanisms of QACs include the following: ①The positively charged nitrogen atom in the QAC molecule has a severe electrostatic interaction with the negatively charged cell membrane, and the long-chain alkyl group binds to the cell membrane, destroying the hydrophobic surface, resulting in irreversible damage, which will force the cell contents to flow out, killing cells by lysis (Huang and Kim, 2013; Wessels and Ingmer, 2013; Gerba, 2015; Seo et al., 2016); ②inducing the production of excess reactive oxygen species, including single oxygen (1O2-), hydroxyl radicals (OH·), superoxide anion radicals (O2·) and hydrogen peroxides (H2O2) could cause oxidative stress and lead to cell injury (Mittler et al., 2011; Fu et al., 2014); ③positively charged nitrogen atoms in QAC molecules tend to replace Ca2+ on the cell membrane, which can reduce the stability of the cell membrane and interfere with the ion exchange between cells and outside (Chen and Cooper, 2002; Jellali et al., 2013; Das et al., 2014); and ④QACs can reduce the chlorophyll content, weaken photochemical quenching, break the chloroplast membrane structure, and inhibit the photoreaction process (Cao et al., 2006; Pérez et al., 2009; Sukenik et al., 2017). Based on the above mechanisms, this study explored the stress effects of MP and MH on the antioxidant defenced system and cell membrane of microalgae. The results showed that both modified clays could induce microalgae cells to produce excess reactive oxygen species, resulting in peroxidative stress. MDA is an essential indicator of peroxidative damage in addition to the antioxidant enzyme system, and its content is positively correlated with the degree of lipid peroxidation. The MDA content of the microalgae in the MH group was higher than that in the MP group, which may indicate that the cell membrane of the microalgae in the MH group was more damaged. The results of trypan blue staining showed that the microalgae in the MP group were almost unstained, while the microalgae in the MH group suffered strong damage to the cell membrane, and the cell viability gradually decreased with increasing modifier concentration, which was similar to lipid peroxidation.
Through the above process, this study found that the ability of QACs to sterilize microalgae mainly originates from there quaternary amino (C-N) functional groups. By exploring the molecular configurations of the two organic agents, this study found that the content of quaternary amino groups of PDMDAAC should be 2.25 times that of HTDMA of the same quality. According to the mechanism of action of QAC, compounds with more C-N should be more toxic. However, PDMDAAC is significantly safer than HDTMA, which is contrary to the observed results. Through FT-IR, this study found that a strong N-H absorption peak can be observed on PDMDAAC, which might be due to the high level of polymerization of this molecule. The stacking of long carbon chains exposed the conjugated electrons on the quaternary ammonium group and bound to H. The N-H bond might weaken the sterilization effect of PDMDAAC by inhibiting the activity of the quaternary ammonium group, thereby making PDMDAAC environmentally friendly.
4.3 Overview of modified clay removal pathways
In summary, the removal pathway of MP to microalgae was shown in a schematic diagram (Figure 10): PDMDAAC can exert an electric neutralization effect to increase the zeta potential of the solution, reduce the repulsion between particles, and promote agglomeration. The PDMDAAC attached to the surface of MP and flocs can assist the clay in capturing and sweeping the microalgae through electrostatic traction and bridging, thereby promoting floc aggregation and sedimentation, to achieve rapid water clarification. MP can also reduce microalgal activity by inducing peroxidative stress, making it easier to remove.
As shown in Figure 10, the inferior flocculation capacity of MH on microalgae should be consistent with the following points: Weak electric neutralization of MH made it difficult for the particles in the solution to agglomerate. HDTMA had a weak modification effect on the surface of clay, which undermined the binding force between MH and microalgae, and the strong self-flocculation ability jointly obstructed the flocculation of microalgae. However, MH could induce microalgae to produce excess reactive oxygen species, resulting in significant peroxidative stress, forcing high degree of lipid peroxidation and destroying the structure of microalgal membranes. The intense cytotoxicity of HDTMA compensated for the lack of flocculation, thereby sterilizing microalgae effectively.
5 Conclusions
1. The extremely low dose of PDMDAAC had a significantly synergistic effect on kaolin, and the MP could remove P. donghaiense effectively.
2. The flocculation effect of MH on microalgae was negligible, which should be caused by its strong self-flocculation ability, weak positive charge, and short carbon chain. MH could increase the degree of peroxidative stress and lipid peroxidation, and destroy the structure of the cell membrane, thereby sterilizing microalgae.
3. The low degree of oxidative stress and less damage to the cell membrane made MP more environmentally friendly, and the microalgae removal effect was mainly due to flocculation. PDMDAAC could exert an electrical neutralization effect to increase the zeta potential of algal liquid and reduce the repulsion between particles in the solution, and the PDMDAAC attached to the surface of modified clay and flocs assisted clay in capturing and sweeping microalgal cells through electrostatic traction and bridging.
Data availability statement
The original contributions presented in the study are included in the article/Supplementary Material. Further inquiries can be directed to the corresponding author.
Author contributions
ZL, conceptualization, writing – original draft, investigation, resources, and methodology. ZY, conceptualization, formal analysis, writing – review and editing, project administration, and funding acquisition. XC, conceptualization, writing – review and editing, funding acquisition. WJ, resources and investigation. YY, writing – review and editing, investigation. XS, writing – review and editing, funding acquisition. All authors contributed to the article and approved the submitted version.
Funding
This research was financially supported by the Marine S&T Fund of Shandong Province for Pilot National Laboratory for Marine Science and Technology (Qingdao) (No. 2021QNLM040001), National Natural Science Foundation of China (41976145), and the Taishan Scholars Climbing Program of Shandong Province of 2019.
Conflict of interest
The authors declare that the research was conducted in the absence of any commercial or financial relationships that could be construed as a potential conflict of interest.
Publisher’s note
All claims expressed in this article are solely those of the authors and do not necessarily represent those of their affiliated organizations, or those of the publisher, the editors and the reviewers. Any product that may be evaluated in this article, or claim that may be made by its manufacturer, is not guaranteed or endorsed by the publisher.
Supplementary material
The Supplementary Material for this article can be foundonline at: https://www.frontiersin.org/articles/10.3389/fmars.2022.1013471/full#supplementary-material
References
Alshahri A. H., Fortunato L., Ghaffour N., Leiknes T. O. (2021). Controlling harmful algal blooms (HABs) by coagulation-Flocculation-Sedimentation using liquid ferrate and clay. Chemosphere 274 (68), 129676. doi: 10.1016/j.chemosphere.2021.129676
Anderson D. M. (2009). Approaches to monitoring, control and management of harmful aalgal blooms (habs). Ocean Coast. Manage. 52 (7), 342. doi: 10.1016/j.ocecoaman.2009.04.006
Andrzej B., Pawe K., Grzegorz C., Jolanta C., Tomasz C., Anna M. F., et al. (2017). Interaction of quaternary ammonium ionic liquids with bacterial membranes – studies with escherichia coli R1–R4-type lipopolysaccharides - ScienceDirect. J. Mol. Liquids 246, 282–289. doi: 10.1016/j.molliq.2017.09.074
Cao X., Song X., Yu Z., Wang K. (2006). Study on the mechanism of removing red tide organisms by organically modified clay. Environ. Sci. 27 (8), 1522–1530. doi: 10.3321/j.issn:0250-3301.2006.08.009
Chen C. Z., Cooper S. L. (2002). Interactions between dendrimer biocides and bacterial membranes. Biomaterials 23 (16), 3359–3368. doi: 10.1016/S0142-9612(02)00036-4
Das T., Sehar S., Koop L., Wong Y. K., Ahmed S., Siddiqui K. S., et al. (2014). Influence of calcium in extracellular DNA mediated bacterial aggregation and biofilm formation. PloS One 9 (3), e91935. doi: 10.1371/journal.pone.0091935
Fu P., Xia Q., Hwang H., Ray P. C., Yu H. (2014). Mechanisms of nanotoxicity: Generation of reactive oxygen species. J. Food Drug Anal. 22 (1), 64–75. doi: 10.1016/j.jfda.2014.01.005
Gerba C. P. (2015). Quaternary ammonium biocides: Efficacy in application. Appl. Environ. Microbiol. 81 (2), 464–469. doi: 10.1128/AEM.02633-14
Guillard R., Hargraves P. E. (1993). Stichochrysis immobilis is a diatom, not a chrysophyte. Phycologia 32 (3), 234–236. doi: 10.2216/i0031-8884-32-3-234.1
Huang W. C., Kim J. D. (2013). Cationic surfactant-based method for simultaneous harvesting and cell disruption of a microalgal biomass. Bioresource Technol. 149 (Complete), 579–581. doi: 10.1016/j.biortech.2013.09.095
Jarvis P., Jefferson B., Gregory J., Parsons S. A. (2005). A review of floc strength and breakage. Water Res. 39 (14), 3121–3137. doi: 10.1016/j.watres.2005.05.022
Jellali R., Kromkamp J. C., Campistron I., Laguerre A., Lefebvre S., Perkins R. G., et al. (2013). Antifouling action of polyisoprene-based coatings by inhibition of photosynthesis in microalgae. Environ. Sci. Technol. 47 (12), 6573–6581. doi: 10.1021/es400161t
Jiang W., Yu Z., Cao X., Jiang K., Yuan Y., Anderson D. M., et al. (2021). Effects of soluble organics on the settling rate of modified clay and development of improved clay formulations for harmful algal bloom control. Environ. pollut. (2021) 289, 117964. doi: 10.1016/j.envpol.2021.117964
Lee C. K., Park T. G., Park Y. T., Lim W. A. (2013). Monitoring and trends in harmful algal blooms and red tides in Korean coastal waters, with emphasis on Cochlodinium polykrikoides. Harmful Algae 30 (dec.suppl.1), S3–S14. doi: 10.1016/j.hal.2013.10.002
Liu Y., Cao X., Yu Z., Song X., Qiu L. (2016). Controlling harmful algae blooms using aluminum-modified clay. Mar. Pollut. Bull. 103 (1/2), 211–219. doi: 10.1016/j.marpolbul.2015.12.017
Li N., Yue Q., Gao B., Xu X., Kan Y., Zhao P. (2018). Magnetic graphene oxide functionalized by polydimethyldiallylammonium chloride for efficient removal of cr(vi). J. Taiwan Inst Chem. Engineers 91, S187610701830302X. doi: 10.1016/j.jtice.2018.05.028
Mittler R., Vanderauwera S., Suzuki N., Miller G., Tognetti V. B., Vandepoele K., et al. (2011). Ros signaling: the new wave? Trends Plant Sci. 16 (6), 300–309. doi: 10.1016/j.tplants.2011.03.007
Na G. H., Choi W. J., Chun Y. Y. (1996). A study on red tide control with loess suspension. J. Aquacult 9.3, 239–245.
NOAA (2016). Available at: http://www.noaa.gov/what-is-harmful-algal-bloom.
Pang X., Chen L., Yuk H. G. (2020). Stress response and survival of salmonella enteritidis in single and dual species biofilms with pseudomonas fluorescens following repeated exposure to quaternary ammonium compounds - sciencedirect. Int. J. Food Microbiol. 325, 108643. doi: 10.1016/j.ijfoodmicro.2020.108643
Perez P., Fernandez E., Beiras R. (2009). Toxicity of Benzalkonium Chloride on Monoalgal Cultures and Natural Assemblages of Marine Phytoplankton. Water Air & Soil Pollution 201 (1–4), 319–330. doi: 10.1007/s11270-008-9947-x
Seger A., Park T. G., Hallegraeff G. (2017). Assessment of the efficacy of clay flocculation in Korean fish farm waters: Cochlodinium cell removal and mitigation of ichthyotoxicity. Harmful Algae 61, 46–55. doi: 10.1016/j.hal.2016.11.014
Sengco M. R., Anderson D. M. (2004). Controlling harmful algal blooms through clay Flocculation1. J. Eukaryotic Microbiol. 51 (2), 169–172. doi: 10.1111/j.1550-7408.2004.tb00541.x
Sengco M. R., Hagstroem J. A., E Granéli Anderson D. M. (2005). Removal of prymnesium parvum (haptophyceae) and its toxins using clay minerals. Harmful Algae 4 (2), 261–274. doi: 10.1016/j.hal.2004.05.001
Seo J. Y., Praveenkumar R., Kim B., Seo J. C., Oh Y. K. (2016). Downstream integration of microalgae harvesting and cell disruption by means of cationic surfactant-decorated Fe3O4 nanoparticles. Green Chem. 18 (14), 3981–3989. doi: 10.1039/C6GC00904B
Sukenik A., Viner-Mozzini Y., Tavassi M., Nir S. (2005). Removal of cyanobacteria and cyanotoxins from lake water by composites of bentonite with micelles of the cation octadecyltrimethyl ammonium (ODTMA). Water Research 120 (Sept. 1), 165. doi: 10.1016/j.watres.2017.04.075
Wessels S., Ingmer H. (2013). Modes of action of three disinfectant active substances: a review. Regul. Toxicol. Pharmacol. 67 (3), 456–467. doi: 10.1016/j.yrtph.2013.09.006
Wu T., Cao X., Yu Z., Song X., Jiang W., Yuan Y., et al. (2020). Study on the flocculation behavior of DPQAC-PAC-MC composite modified clay in different media. Oceanol Limnol Sin. 52 (1), 106–113. doi: 10.11693/hyhz20200400118
Xin H., Gao B., Rong H., Yue Q., Zhang Y., Teng P. (2015). Effect of using poly dimethyl diallyl ammonium chloride as coagulation aid on polytitanium salt coagulation performance, floc properties and sludge reuse. Separation Purification Technol. 143, 64–71. doi: 10.1016/j.seppur.2015.01.024
Yu Z., Song X., Cao X., Liu Y. (2017). Mitigation of harmful algal blooms using modified clays: Theory, mechanisms, and applications. Harmful Algae 69 (nov.), 48–64. doi: 10.1016/j.hal.2017.09.004
Yu Z., Zou J., Ma X., Li Q. (1993). Chemical methods for red tide control. Oceanol Limnol Sin. 24 (3), 314–318. doi: CNKI:SUN:HYFZ.0.1993-03-012
Zhang Z. (2016). Preparation of quaternized organoclay minerals and their algae-removing properties (Engineering University of Xian), Degree thesis. (in Chinese).
Zhang J., Tan W., Li Q., Liu X., Guo Z. (2021). Preparation of cross-linked chitosan quaternary ammonium salt hydrogel films loading drug of gentamicin sulfate for antibacterial wound Dressing. Mar. Drugs 19 (9), 479. doi: 10.3390/md19090479
Zhang S., Zheng H., Tang X., Zhao C., Gao B. (2019). Sterilization by flocculants in drinking water treatment. Chem. Eng. J. 382, 122961. doi: 10.1016/j.cej.2019.122961
Zhao Y. X., Gao B. Y., Rong H. Y., Shon H. K., Kim J. H., Yue Q. Y., et al. (2011). The impacts of coagulant aid-polydimethyldiallylammonium chloride on coagulation performances and floc characteristics in humic acid–kaolin synthetic water treatment with titanium tetrachloride. Chem. Eng. J. 173 (2), 376–384. doi: 10.1016/j.cej.2011.07.071
Keywords: poly dimethyl diallyl ammonium chloride, modified clay, flocculation performance, physiological stress, removal mechanism
Citation: Liu Z, Yu Z, Cao X, Jiang W, Yuan Y and Song X (2022) An environmentally friendly material for red tide algae removal: Performance and mechanism. Front. Mar. Sci. 9:1013471. doi: 10.3389/fmars.2022.1013471
Received: 07 August 2022; Accepted: 01 September 2022;
Published: 20 September 2022.
Edited by:
Zhangxi Hu, Guangdong Ocean University, ChinaReviewed by:
Linjian Ou, Jinan University, ChinaXudong Ye, Memorial University of Newfoundland, Canada
Copyright © 2022 Liu, Yu, Cao, Jiang, Yuan and Song. This is an open-access article distributed under the terms of the Creative Commons Attribution License (CC BY). The use, distribution or reproduction in other forums is permitted, provided the original author(s) and the copyright owner(s) are credited and that the original publication in this journal is cited, in accordance with accepted academic practice. No use, distribution or reproduction is permitted which does not comply with these terms.
*Correspondence: Zhiming Yu, enl1QHFkaW8uYWMuY24=