- 1College of Earth, Ocean and Atmospheric Sciences, Oregon State University, Corvallis, OR, United States
- 2College of Earth, Ocean, and Environment, University of Delaware, Newark, DE, United States
- 3Alaska Fisheries Science Center, National Marine Fisheries Service, National Oceanic and Atmospheric Administration, Hatfield Marine Science Center, Newport, OR, United States
- 4College of Science, Oregon State University, Corvallis, OR, United States
The overwinter survival mechanisms of Antarctic krill, Euphausia superba, are poorly characterized, especially for juveniles. It has been suggested that juveniles adopt a mix of strategies characteristic of both larvae and adults. Like larvae, they may feed opportunistically throughout winter when food is available, and like adults they may be able to suppress their metabolism when food is scarce. In this study we look at the overwinter strategies of juvenile krill and how their reproductive development changes when energy input exceeds what is necessary for survival. We take a closer look at how the sexual maturation of juvenile krill progresses in response to different environmental conditions throughout the fall and winter. We exposed juvenile Antarctic krill to four different “food environment scenarios”, supplementing them with various diets from May to September 2019 that were representative of environmental conditions that they may encounter in different regions of the Western Antarctic Peninsula during autumn and winter. Each month, we measured the physiology and condition of the krill, and assessed the reproductive development of females. We found that when female juvenile krill have greater energy reserves than what is needed to survive the winter, they will begin to sexually mature. Further, when there are sufficient levels of the fatty acids eicosapentaenoic acid (EPA), docosahexaenoic acid (DHA) and 16:4 (n-1), krill are likely to be in a more reproductive advanced stage. However, when lipids, EPA, DHA and 16:4 (n-1) are depleted throughout the winter, juvenile female krill lose their ability to develop reproductively. We also found that sexual development is an energy intensive process that requires high respiration rates in juvenile krill. Furthermore, when juvenile females expend energy maturing, their physiological condition declines. This trade-off between early reproductive development and condition in juvenile female krill has important implications for individual health and population fecundity. Gaining a better understanding of the mechanisms behind juvenile krill winter survival strategies and their consequences will allow us to predict how future change at the western Antarctic Peninsula may affect krill population dynamics, especially in light of a warming climate.
Introduction
Antarctic krill, Euphausia superba (hereafter “krill”), populations are dependent on individuals surviving the winter: a time characterized by low light levels and food scarcity in the environment (Quetin and Ross, 2003). Larvae continuously feed throughout the autumn and winter in order to survive, while adults can suppress their metabolism and survive on lipid reserves when food is scarce (Ikeda and Dixon, 1982; Meyer and Teschke, 2016). Juvenile krill overwintering strategies, in contrast, have remained unknown, and it has been suggested that juveniles implement a “compromise” strategy (Atkinson et al., 2002), adopting opportunistic feeding behaviors, similar to larvae, while at the same time exhibiting a reduction in their metabolism, similar to adults (Hagen, 1999; Meyer et al., 2009; Meyer et al., 2010).
Unlike larvae, juveniles have the capacity to prioritize lipid storage over growth and can build up lipid reserves leading up to and throughout winter (Hagen et al., 1996; Schaafsma et al., 2017). The allocation of energy towards lipid storage increases with ontogeny, and previous studies have found that adult krill can survive for longer than six months without eating, relying solely on their lipid reserves (Clarke, 1980; Quetin and Ross, 1991). This is primarily due to the highly reduced metabolic state that adults enter into at the onset of winter in an effort to conserve energy (Quetin and Ross, 1991). Unlike adults, however, juvenile krill show a less pronounced decrease in seasonal metabolism (Meyer et al., 2010).
Some studies have found that juvenile krill, like adults, will limit their feeding behavior at the onset and throughout the winter season, even if there is excess food available in the environment (Meyer, 2012). Conversely, Bernard et al. (2019) found that, when exposed to sea ice during the winter, grazing on ice algae contributed ~146% to the winter energy budget of juvenile krill. The fact that juveniles may continue to feed throughout the winter suggests that they may not rely solely on their lipid reserves to survive this period of food paucity in the environment. Furthermore, it suggests that if juveniles are in an environment with high food concentrations throughout the winter, they may be able to accumulate enough energy and allocate it towards reproductive or somatic growth, in addition to survival. In this study, we aimed to determine whether juvenile krill can initiate early reproductive development if supplied with ample energy inputs. Additionally, we take a closer look at the energetic costs of reproductive development.
Reproductive development in krill varies regionally based on diet and other environmental conditions, such as temperature (Quetin and Ross, 2001; Kawaguchi, 2016). Sexual maturation can be delayed up to a year if the nutritional content of a krill’s diet is insufficient to meet the energetic demands for reproduction (Quetin and Ross, 2001). The exact amount of food needed to initiate reproductive development varies based on the body size and lipid content of an individual, and it is believed that krill will not initiate maturation if they do not possess adequate levels of energy (Cuzin-Roudy and Amsler, 1991; Cuzin-Roudy and Labat, 1992). In addition to the quantity of food, the quality of food has been observed to affect maturation in krill. Previous studies have found that feeding on diatoms enhances the reproductive development and growth rates of krill, compared to other dietary sources including copepods, dinoflagellates and cryptophytes (Pond et al., 2005; Atkinson et al., 2006; Yoshida, 2009; Schmidt et al., 2014). Further, Cuzin-Roudy and Labat (1992) observed female krill ovarian oocytes developing in response to feeding on phytoplankton blooms at the ice-edge. Environmental conditions during the reproductive development period are especially important for juvenile krill that are first time spawners as their maturation rate has found to more heavily rely on food availability than older spawners (Kawaguchi et al., 2007).
The food available for krill to feed on throughout the winter changes in response to oceanographic conditions at the Western Antarctic Peninsula (WAP). Intensive warming at the northern WAP has been especially pronounced throughout the autumn and winter, resulting in a later sea ice advance, a shorter sea ice season duration and an earlier sea ice retreat (Vaughan et al., 2003; Stammerjohn et al., 2008). These changes in sea ice dynamics have led to lower abundances of sea ice algae concentrations, which are primarily made up of diatoms (Garrison et al., 2005; Fritsen et al., 2011). When phytoplankton are not abundant during the winter, post-larval krill will often feed on other zooplankton, predominantly copepods, if they are available (Schmidt et al., 2014). In contrast to the decline in sea ice algae, copepod abundances have increased at the wAP in response to an earlier than average sea ice retreat (Gleiber, 2014). Krill are opportunistic foragers and have been found near the seabed during the winter, and with varying levels of detritus and remnants of benthic species in their stomachs (Ligowski, 2000; Nicol, 2007; Schmidt et al., 2011) In this study, we used controlled laboratory experiments to test how four different food environment scenarios (FES) impacted the reproductive development, respiration, condition and lipid content of juvenile female krill throughout the winter.
Materials and methods
Study site and krill collection
Juvenile krill (> 24 and < 40 mm, mean = 29 mm) were collected at several sites in the Gerlache Strait and Wilhelmina Bay (Figure 1) in April 2019 from aboard the ARSV Laurence M. Gould using an Isaacs-Kidd Midwater Trawl (IKMT) towed obliquely between the surface and an average of 50 m, targeting krill aggregations observed acoustically. These regions along the northern Western Antarctic Peninsula (WAP) were chosen for sampling based on their historically high densities of krill. We used an acoustic echosounder to track krill aggregations and to pick our locations for targeted net tows. Krill were collected at night and were held in two flow-through tanks aboard the ship until arrival at Palmer Station. We collected ~5,000 juvenile krill to be used for our overwinter experiments that we conducted at Palmer Station, Antarctica from May to September 2019.
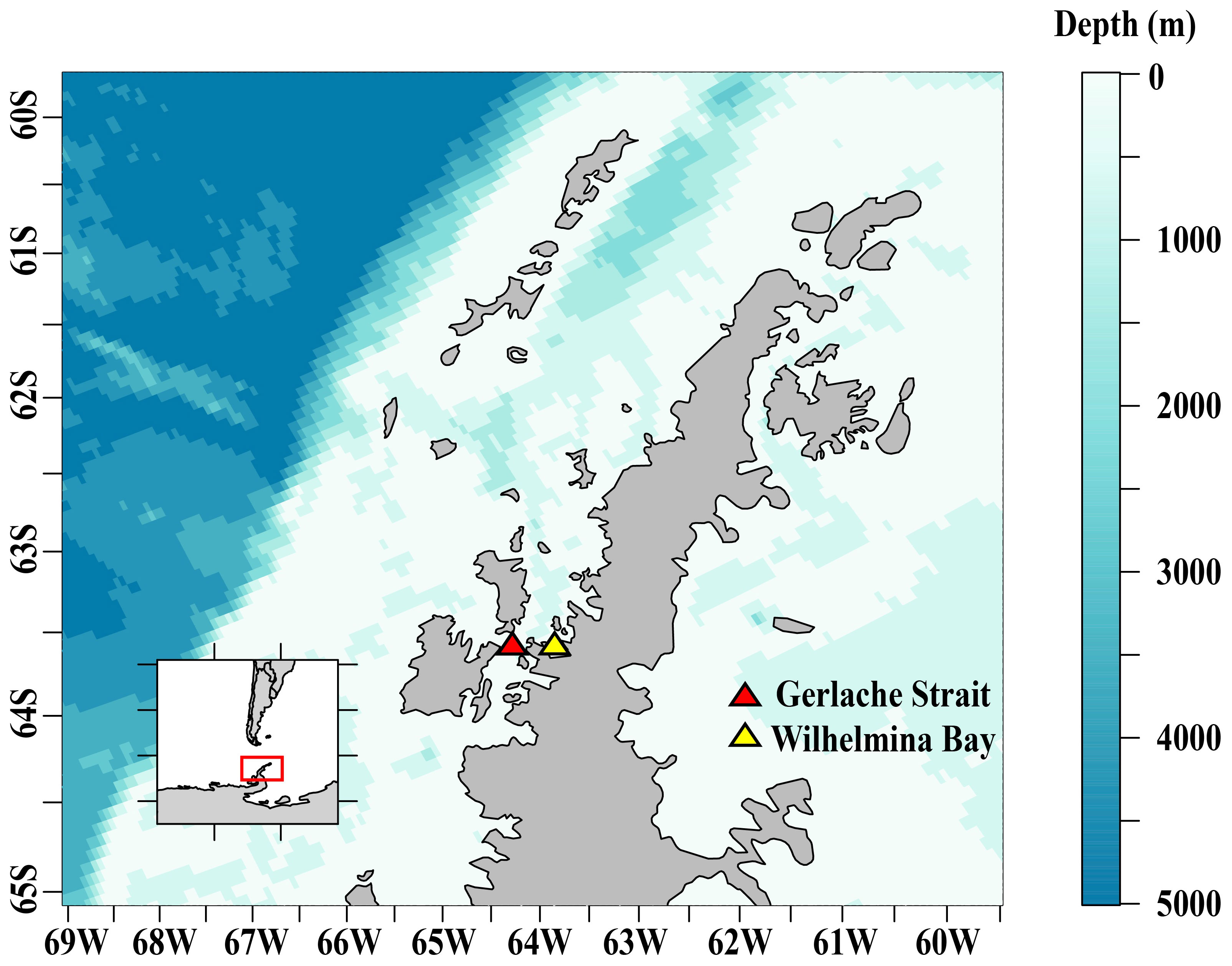
Figure 1 The study area along the Western Antarctic Peninsula where juvenile Antarctic krill were collected for overwinter experiments at Palmer Station, Antarctica.
Experimental setup
Details of the experimental setup are provided in our companion paper (Bernard et al., 2022). Briefly, juvenile Antarctic krill were placed into four large (1,330 L) circular flow-through tanks at Palmer Station, Antarctica, and were supplemented with different prey items representing four unique food environment scenarios (FES). The FES in the tanks (T1- 4) were as follows: (T1) the natural seawater assemblage (NAT) was supplemented with a freeze-dried and ground local mixed zooplankton assemblage (MZA) for the first half of the field season only, simulating autumn feeding and winter starvation, we refer to this here as MZA-NAT; (T2) the NAT was supplemented with MZA for the entire duration of the season, simulating continuous feeding throughout the developmental period, we refer to this here as MZA-MZA; (T3) the NAT was supplemented with a local diatom culture (DIA) for the first half of the season only, we refer to this as DIA-NAT; and (T4) the NAT was supplemented with DIA the entire season, which we refer to here as DIA-DIA. Without stopping the inflow of water into the tanks, krill were supplemented with food every 3-5 days. The concentrations of the dried zooplankton and diatoms within the tanks were ~0.12 mg C L-1 and ~0.05 mg CL-1, respectively. Concentrations of the MZA diet were in the higher end of the range of reported values for under-ice zooplankton biomass in the Antarctic (0.001 - 0.88 mg CL-1). Similarly, the DIA diet concentrations were in the higher end of the range of reported values for phytoplankton in the fall and sea ice algae in the winter (0.004 - 0.40 mg CL-1) (Bernard et al., 2022 and references therein).The krill were kept in constant darkness and at ambient temperatures with flow rates of ~ 11 liters per minute resulting in the turnover of seawater within each tank every ~ 2 hours. Krill were sampled every four weeks, beginning in May and ending in September. In May, prior to placing krill into their respective FES tanks, 160 krill were randomly selected, measured, weighed and preserved in formalin solution for reproductive analysis. From May to September, 40 krill from each FES were randomly selected, measured (Standard Length 1; Mauchline, 1981), weighed for wet weight (mg), sexed, and staged. Female krill were then preserved in a 10% formalin solution for subsequent reproductive analysis. Oxygen consumption rates were measured for individual krill during each sampling time point (µl O2 (mg DW)-1 hr-1) and were then converted to mass of carbon respired (mg C ind-1 day-1) following methods outlined by Ikeda et al. (2000). In this paper, we refer to the loss of carbon through respiration (mg C ind-1 day-1) as the respiration rate. For a more thorough description of the respiration rate experiments and conversions used, we refer the reader to Bernard et al. (2022). We calculated krill condition factor in the same manner that is outlined in Steinke et al. (2021), using an empirical formula to identify the relationship between wet weight and total length of krill.
Reproductive staging
Female krill reach sexual maturity within their third year of growth, as age-class 2+ krill. At this age, krill are typically > 26 mm in length by the time spawning occurs (Siegel and Loeb, 1994). There are ten sexual development stages (SDS) for gonadal maturation in female krill (Cuzin-Roudy and Amsler, 1991; Cuzin-Roudy, 2000). During the first stage of gonadal development (SDS 1), also known as gametogenesis, primary oogonia (og1) proliferate into secondary oogonia (og2) in the germinal zone of ovaries. In SDS 1, females have not yet developed their external sexual organs (i.e., thelycum). The thelycum begins to develop prior to SDS 2, which is the stage of oogenesis. During oogenesis the ovary expands and og2 transition into primary oocytes (yoc). Once yoc have been established in the ovary, they begin to accumulate glycoproteic yolk and grow into type 1 oocytes (oc1) during SDS 3-4, which is the stage of previtellogenesis.
Once females begin to accumulate lipidic yolk, they transition to the stage of vitellogenesis (SDS 5-7) and oc1 mature into type 2 oocytes (oc2). During vitellogenesis, oc2 grow into type 3 and eventually transition to type 4 oocytes (oc3 and oc4, respectively). Once females have reached SDS 7, oc4 are mature and krill are ready to spawn. After a spawning event, the ovary enters the oviposition stage (SDS 8). Once all oocytes produced during previtellogenesis have been used, or the female has insufficient energy levels to carry out another spawning event, the ovary enters the reproductive rest phase (SDS 9-10) in which ovarian reorganization occurs. Krill carry out cyclic spawning (SDS 5-8) in the summer when resources are prevalent and enter the reproductive rest period (SDS 9-10) in fall and early winter when food availability starts to dwindle. Krill remain in their immature stages throughout winter (SDS 1-2) and will start developing reproductively (SDS 3-4) when exposed to higher food concentrations (Kawaguchi et al., 2007).
First, we identified the sex of each krill using external morphological characteristics (Makarov and Denys, 1984). Then, we identified the SDS of each female krill using a histological squash method (Cuzin-Roudy and Amsler, 1991). Through dissection, we removed a single lobe of the female ovary and covered it with a drop of ethanol prior to placing it on a microscope slide. Cuzin-Roudy and Amsler (1991) found that ethanol used in this way enhanced the details of the cellular structure. After placing the lobe onto the slide, we covered it with a glass coverslip and gently squashed the tissue onto the microscope slide by lightly pressing down on the coverslip and separating the germ cells into a single layer, careful not to add any horizontal movement that would break the cells apart. We used a Nikon E800 compound microscope (200x magnification) to examine the cellular structures. In order to quantify the cellular development of female krill ovaries using image analysis techniques, we photographed the cells on each slide using a digital camera attached to the microscope.
Segmentation and quantification of ovarian cells
To measure the ovarian cells, we used the machine learning software programs ilastik and CellProfiler. We used the machine learning software ilastik to segment the images into two categories: cells and background (Berg et al., 2019). We annotated cell and background pixels in each image and ilastik used a Random Forest classifier to segment the images based on our annotations. Ilastik was trained on a subset of the images (i.e., training set), until it could accurately predict the cell objects in the images that were not trained (i.e., validation set). For a more detailed explanation of segmentation methods, we refer the reader to the Supplementary Materials.
We used CellProfiler, a software that extracts data from biological images, to classify and quantify the objects within the images (McQuin et al., 2018). We developed a pipeline in CellProfiler that (i) removed the background from each image, (ii) thresholded the objects within each image, and (iii) quantified the respective areas of the cells found in each image. Batch processing in CellProfiler enabled us to accurately and rapidly quantify the cells from all of the images in the image set. For a step-by-step walkthrough of our pipeline development and quantification steps, we refer the reader to the Supplementary Materials.
The pilot study on Antarctic krill cellular reproductive staging carried out by Cuzin-Roudy and Amsler (1991) used cell diameter (µm), rather than area (µm2), to estimate SDS. Cell area is a more accurate way of measuring the maturity of a cell because you get a 2-dimensional representation of cell size, rather than a one-dimensional representation of cell diameter. In that study, cell diameter was used rather than cell area because the authors were limited to microscope observations and did not have the capacity to measure cell area using computer software programs (M. Amsler, personal communication). In an effort to stage our female krill based on the key developed by Cuzin-Roudy and Amsler (1991) we plotted ovarian cell areas against their respective diameters (Supplementary Figure 1, R2 = 0.98). The empirical relationship between the two was used to develop a new key for staging female Antarctic krill based on cell area, rather than cell diameter (Table 1).
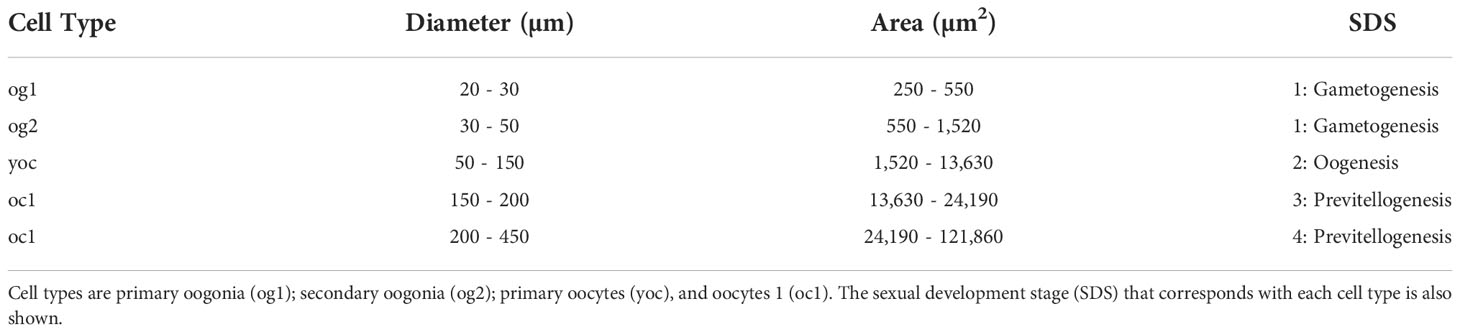
Table 1 Ovarian cell types with corresponding cell diameters (µm) as determined by Cuzin-Roudy and Amsler (1991) and cell areas (µm2) measured in this study.
Estimation of the onset of maturity index
Due to the variability in the number of female krill that were randomly sub-sampled each month (Table 2), we examined the proportion of female krill that were more reproductively mature at each time point, rather than comparing the total number of individuals. Each individual krill ovary consisted of numerous cells and we used the maximum cell area (µm2) in each image to estimate SDS. The maximum cell area represents the most advanced oocytes, and thus the most advanced SDS, of the female ovary. To estimate the proportion of krill that were reproductively mature enough to initiate the first steps in sexual reproduction, SDS was used to calculate an onset of maturity index (OMI):
Where n is the number of females at a particular SDS. The OMI is a measure of the proportion of the female population that are in previtellogenesis. A high OMI suggests that more of the female population are in previtellogenesis than in either gametogenesis, oogenesis (nSDS1-2) or resting (nSDS9-10) stages. Throughout the length of this study, we did not see any female krill in SDS 4 - 10, as these stages are only observed just prior to, during and at the end of the spawning season (spring - summer).
Analysis of total lipids and fatty acids
Due to logistical constraints, total lipids and fatty acids were only measured from July to September. Six krill from each of the four tanks were randomly selected during our discrete monthly sampling time-points for lipid and fatty acid analyses. These krill were measured for length (Standard Length 1, mm) and wet weight (mg) before being freeze-dried and reweighed for dry weight (mg). Freeze-dried krill were placed individually into 20 mL glass scintillation vials and stored at -80 °C until later analysis at the Marine Lipid Ecology Laboratory at the Hatfield Marine Science Center. In the laboratory, freeze-dried krill were homogenized and vortexed in chloroform and methanol and total lipids were extracted in chloroform:methanol:water (8:4:3) according to Parrish (1987) using a modified Folch procedure (Folch et al., 1956). Lipid classes were determined using thin layer chromatography with flame ionization detection (TLC/FID) with a MARK VI Iatroscan (Iatron Laboratories, Tokyo, Japan) as described by Lu et al. (2008) and Copeman et al. (2017). Total lipids were estimated by using the summation of the individual calibrated lipid classes (Parrish, 1987).
Following lipid class analyses, lipid extracts were processed for identification and quantification of fatty acids. An internal standard (23:0 methyl ester) was added at approximately 10% of the total fatty acids to all samples and total lipid extracts were derivatized into their fatty acid methyl esters (FAMEs) using sulphuric acid-catalyzed transesterification (Budge et al., 2006). Resulting FAMEs were analyzed on an HP 7890 GC FID equipped with an autosampler and a DB wax+ GC column (Agilent Technologies, Inc., U.S.A.). The column was 30 m in length, with an internal diameter of 0.25 mm and film thickness of 0.25 µm. The column temperature began at 65°C and held this temperature for 0.5 min. Temperature was increased to 195°C (40°C min-1), held for 15 min then increased again (2°C min-1) to a final temperature of 220°C. Final temperature was held for 1 min. The carrier gas was hydrogen, flowing at a rate of 2 mL min-1. Injector temperature was set at 250°C and the detector temperature was constant at 250°C. Peaks were identified using retention times based upon standards purchased from Supelco (37 component FAME, BAME, PUFA 1, PUFA 3) and in consultation with retention index maps performed under similar chromatographic conditions as our GC-FID (Wasta and Mjøs, 2013). Column function was checked by comparing chromatographic peak areas to empirical response areas using a quantitative FA mixed standard, GLC 487 (NuCheck Prep). Chromatograms were integrated using Chem Station (version A.01.02, Agilent).
Statistical analyses
Third-order polynomial models were used to identify changes in the onset of maturity index (OMI), respiration rate and condition factor (CF) of juvenile krill throughout the winter. A third-order polynomial model was also used to identify the relationship between krill OMI and their respiration rate. We modeled the relationship between krill total length (mm), weight (mg), OMI and respiration rate. Condition factor [CF; WWT (mg) mm-3.44] was the only variable that was significant in any of the models, so length and weight were removed from the model formulations. The relationship between krill respiration rate and condition factor was best explained with a second-order polynomial, or linear, model. The order of the OMI, respiration and CF regression models was chosen based on their Akaike Information Criterion (AIC) score. The model with the lowest AIC is considered the best model based on the model fit and complexity (Bozdogan, 1987), therefore the model with the lowest AIC score was the model we chose (Table 3). Additionally, we constructed a model to identify any differences in the OMI between krill in the various food environment scenarios (FES).
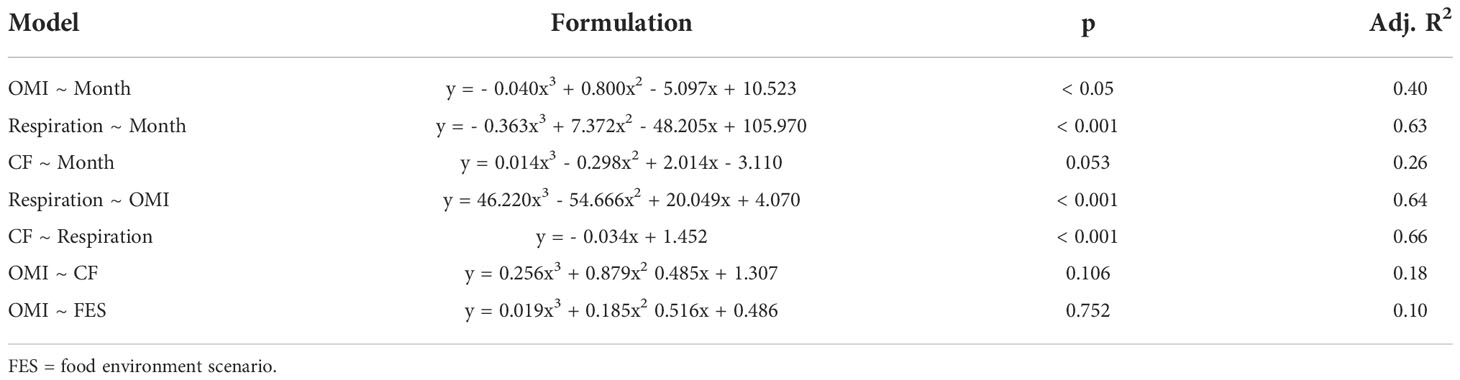
Table 3 Model formulations, p-values and adjusted R-squared values (Adj. R2) for the regressions used to determine the relationships between the onset of maturity index (OMI), respiration rate (mg C ind-1 day-1), and condition factor (CF; WWT (mg) mm-3.44).
After ensuring that the data were normally distributed, had equal variance and no outliers, and remained linear over time, we ran a two-way analysis of covariance (ANCOVA), followed by a post-hoc simple main effects analysis test to identify differences in total lipids and reproductively important fatty acids EPA [20:5 (n-3)], DHA [22:6 (n-3)] and 16:4 (n-1) over time and in each of the four FES tanks. Linear regressions were then used to show the change in total lipids, EPA, DHA and 16:4 (n-1) from July to September. All statistical tests were performed in RStudio 2022.07.01 (RStudio Team, 2022).
Results
The onset of maturity in juvenile krill
Most of the female krill were in oogenesis throughout the length of the experiment, with only a few in gametogenesis each month and previtellogenesis observed from July to September (Table 2). Krill did not reach the onset of maturity until July, when their OMI was 0.23, and the onset of maturity index (OMI) peaked in August at 0.27 (Table 2, Figure 2A). In September, the OMI dropped from 0.27 in August to 0.02 (Table 2). No significant relationship was observed between the OMI and food environment scenario (FES) (Table 3). The respiration rate of krill was low in May and June, averaging 4.00 and 3.42 mg C ind-1 day-1, respectively. Respiration increased in July to an average rate of 5.91 mg C ind-1 day-1 and peaked in August with an average rate of 6.06 mg C ind-1 day-1. In September, the respiration rate of krill dropped to an average of 4.93 mg C ind-1 day-1 (Table 4). The patterns observed in krill respiration rate were similar to those observed for OMI throughout the winter (Figure 2B). Respiration rate was positively correlated with the OMI, and as the OMI reached ~0.2, the respiration rate of krill plateaued (Figure 2D).
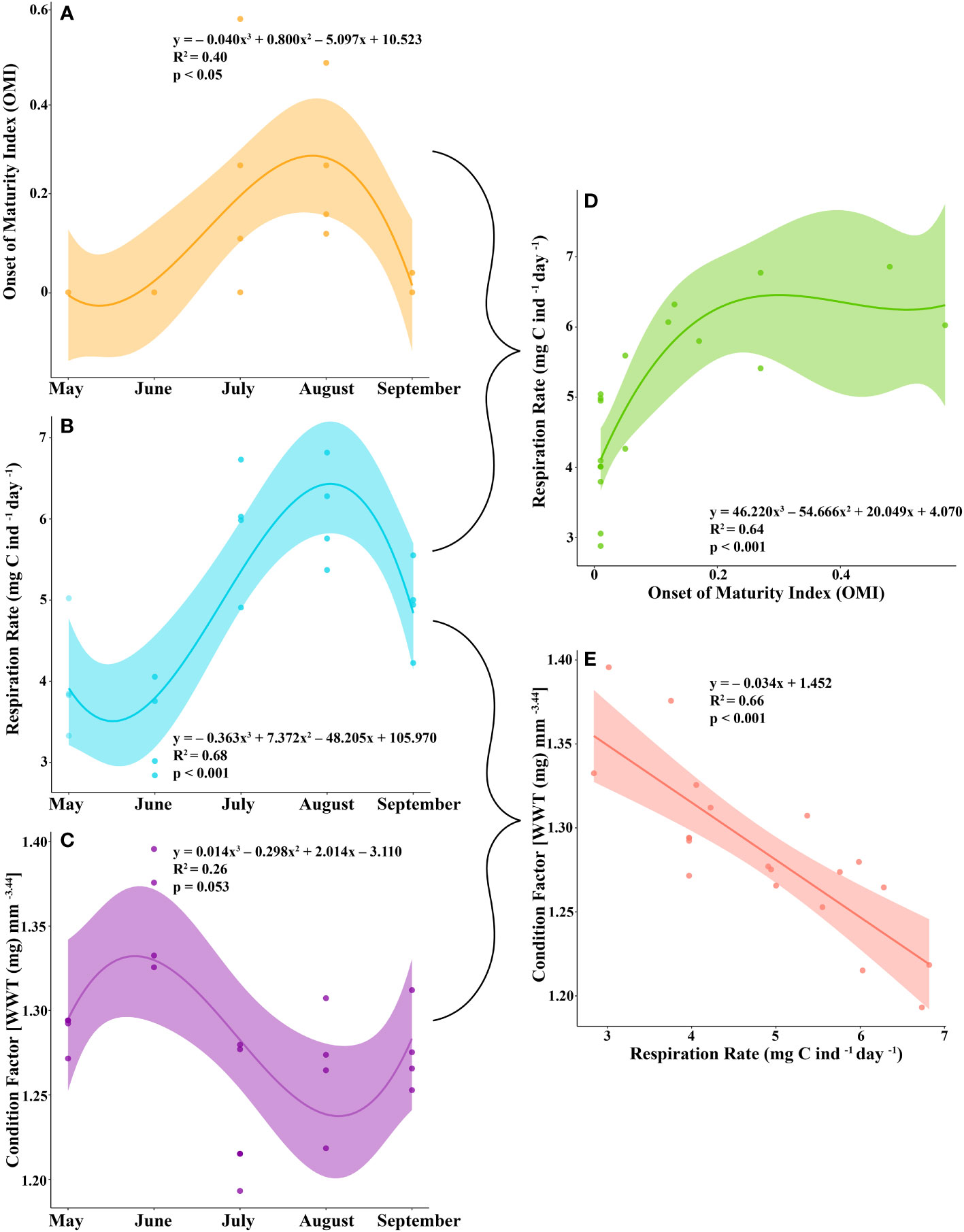
Figure 2 The (A) onset of maturity index (OMI), (B) respiration rate (mg C ind-1 day-1), and (C) condition factor [wet weight (WWT; mg) mm-3.44 of juvenile krill from May to September. The relationship between (D) OMI and respiration rate and (E) respiration rate and condition factor.

Table 4 The mean and standard deviation of krill respiration rate (mg C ind-1 day-1), condition factor (CF; WWT (mg) mm-3.44), length (mm) and wet weight (mg) from May to September.
The energetic cost of early reproductive development in juvenile krill
The average condition factor of juvenile female krill in May was 1.28 mg mm-3.44. In June, their condition factor peaked, averaging 1.36 mg mm-3.44. The condition factor of juvenile female krill was lowest in July, averaging 1.24 mg mm-3.44 and increased slightly in August and September with an average condition of 1.27 mg mm-3.44 and 1.28 mg mm-3.44, respectively (Table 4). The condition factor of juvenile female krill had an opposite trend to what was observed in both OMI and krill respiration rate throughout the winter (Figure 2C). Juvenile female krill condition factor had a negative linear relationship with krill respiration rate (Figure 2E).
Lipids and key fatty acids for reproductive development
Krill in all food environment scenarios (FES), with the exception of krill in the MZA-MZA tank, showed significant depletion of their lipid reserves from July to September (Figure 3A). The rate of lipid utilization over time varied significantly by FES (Table 5; p < 0.001). Krill in the DIA-NAT tank utilized lipids at a greater rate (3.9% per month) than krill in the other FES tanks (p < 0.05). Krill in the MZA-MZA tank did not differ in lipid utilization rate from krill in the MZA-NAT tank (0.95% and 2.1% per month, respectively; p = 0.08). Similarly, krill in the DIA-DIA tank (3.4% per month) showed no significant differences in lipid utilization rate from krill in the MZA-NAT tank (p = 0.51). However, krill in the DIA-DIA tank had a significantly higher lipid utilization rate than krill in the MZA-MZA tank (p < 0.05). In July, total lipids made up ~15% of an individual’s total dry body mass, averaging 154.62 µg mg-1 across the four FES. In August, total lipids made up ~12% of an individual’s total dry body mass, averaging 124.87 µg mg-1 across the four FES. In September, total lipids made up ~10% of an individual’s total dry body mass, averaging 97.95 µg mg-1 across the four FES (Table 6).
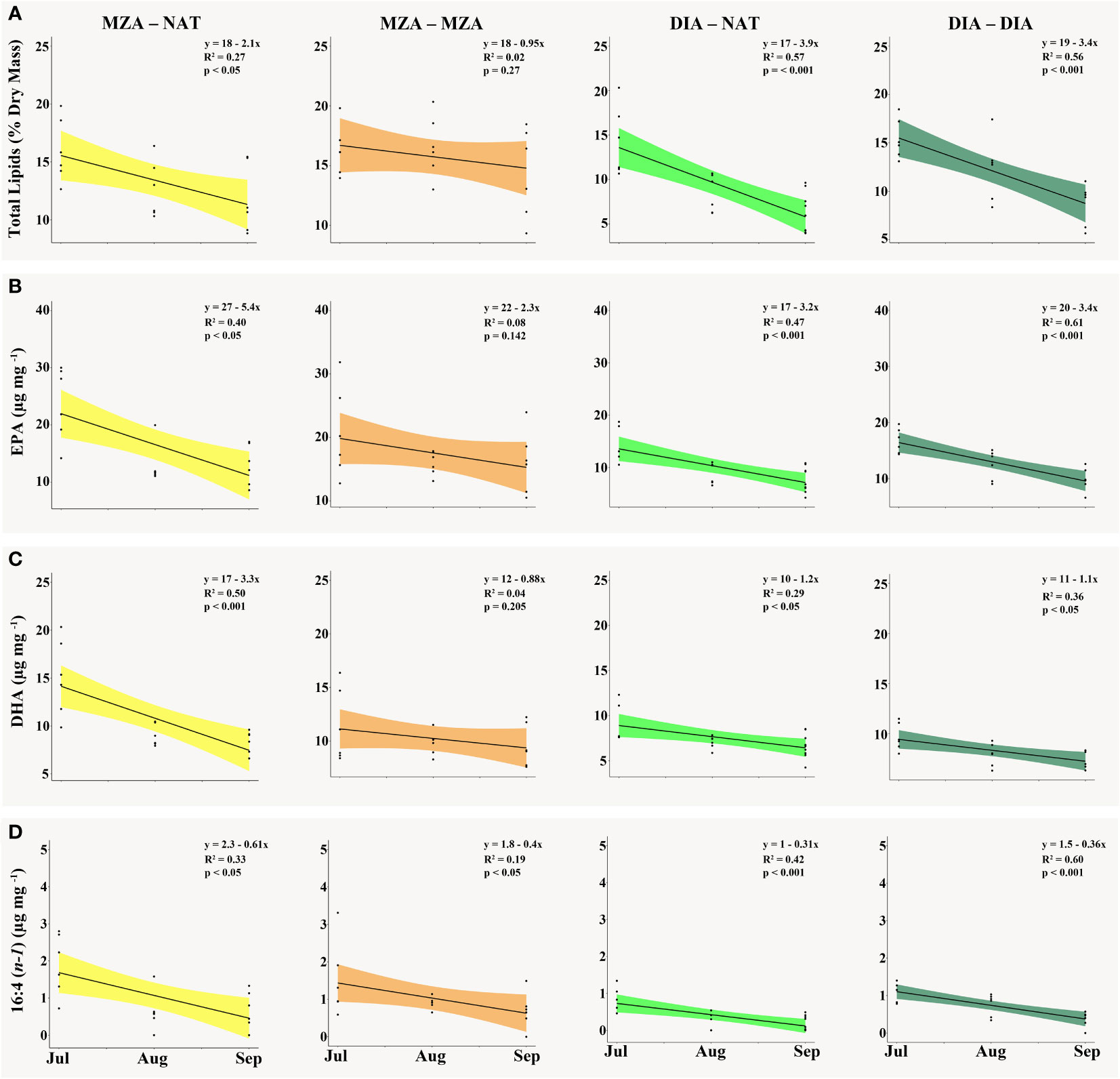
Figure 3 The (A) total lipid content as a percentage of krill dry mass (mg), (B) EPA [20:5(n-3)] content (µg mg-1), (C) DHA [22:6(n-3)] content (µg mg-1) and (D) 16:4(n-1) content (µg mg-1) present in krill tissue in the MZA - NAT (yellow), MZA - MZA (orange), DIA - NAT (light green) and DIA - DIA (dark green) food environment scenarios (FES) from July to September. Abbreviations: MZA - MZA, mixed zooplankton assemblage from May to September; DIA - DIA, diatoms from May to September; MZA - NAT, natural seawater, fed MZA from May to July, and DIA - NAT, natural seawater, fed DIA from May to July.
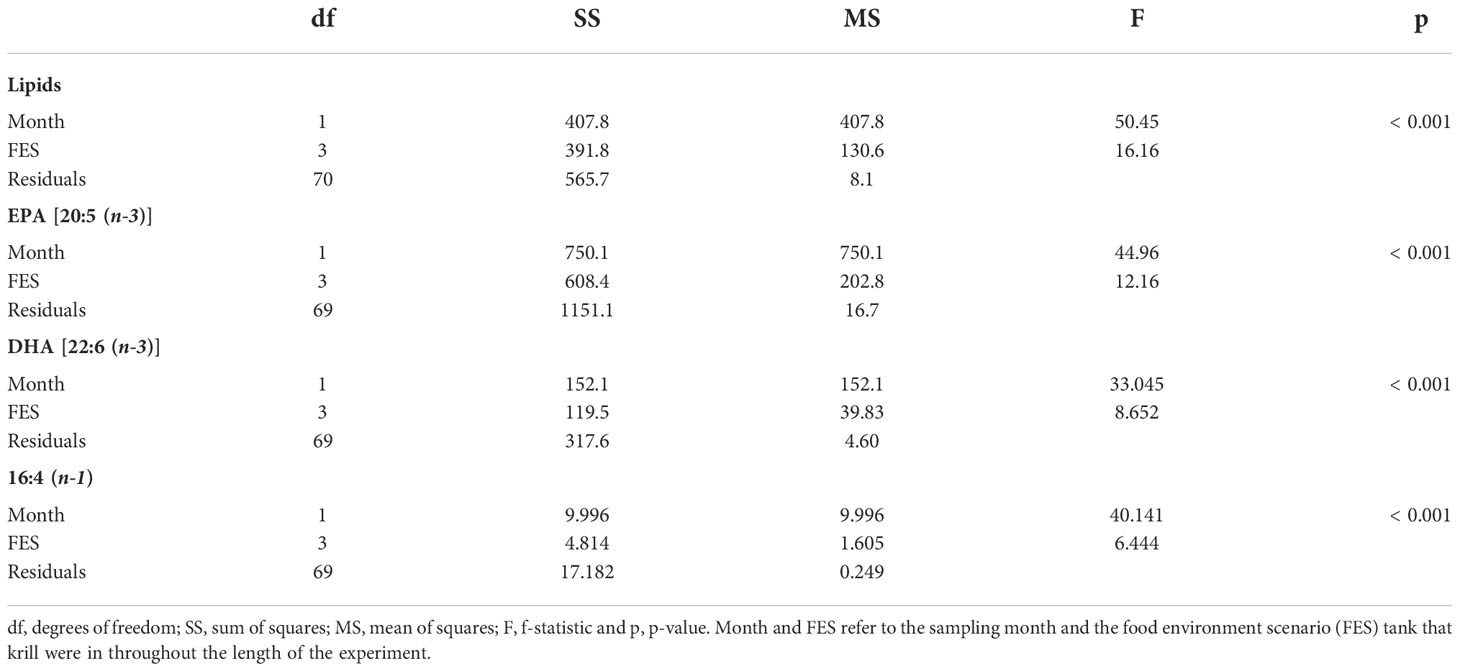
Table 5 Analysis of covariance (ANCOVA) statistics for total lipids (% dry mass), eicosapentaenoic acid (EPA; µg mg -1), docosahexaenoic acid (DHA; µg mg -1) and 16:4 (n-1) (µg mg -1).
Krill in all FES, with the exception of krill in the MZA-MZA tank, showed a significant decrease in EPA [20:5(n-3)] and DHA [22:6(n-3)] between July and September (Figures 3B, C). The rates of depletion of these fatty acids varied significantly between FES (Table 5; p < 0.001). Krill in the MZA-NAT tank (decrease of 5.4 µg mg-1 of EPA per month) did not differ in utilization rate of EPA from krill in the MZA-MZA tank (decrease of 2.3 µg mg-1 of EPA per month; p = 0.88). However, EPA concentrations in krill in the MZA-NAT tank were depleted at a greater rate than those in krill in both the DIA-NAT (decrease of 3.2 µg mg-1 of EPA per month) and DIA-DIA (decrease of 3.4 µg mg-1 of EPA per month) tanks (p < 0.05). Krill in the MZA-MZA tank had a significantly lower EPA utilization rate than krill in both the DIA-NAT and DIA-DIA tanks (p < 0.05). Krill in the DIA-NAT and DIA-DIA tanks did not differ in utilization rate of EPA over time (p = 0.22). Similarly, krill in the MZA-NAT tank (decrease of 3.3 µg mg-1 of DHA per month) did not differ in utilization rate of DHA from krill in the MZA-MZA tank (decrease of 0.88 µg mg-1 of DHA per month; p = 0.87), and krill in the MZA-NAT tank depleted DHA at a greater rate (decrease of 3.3 µg mg-1 of DHA per month) than krill in both the DIA-NAT (decrease of 1.2 µg mg-1 of DHA per month) and DIA-DIA (decrease of 1.1 µg mg-1 of DHA per month) tanks (p < 0.05). Krill in the DIA-NAT and DIA-DIA tanks did not differ in utilization of DHA over time (p = 0.82). Utilization of the fatty acid 16:4(n-1) showed significant depletion from July to September in all FES (Figure 3D). Krill in the DIA-NAT tank (decrease of 0.31 µg mg-1of 16:4(n-1) per month) had the same 16:4(n-1) utilization rate as krill in the DIA-DIA (decrease of 0.36 µg mg-1 of 16:4(n-1) per month) tank (p = 0.28), but a lower 16:4(n-1) utilization rate than krill in both the MZA-NAT (decrease of 0.61 µg mg-1 of 16:4(n-1) per month) and MZA-MZA (decrease of 0.4 µg mg-1 of 16:4(n-1) per month) tanks (p < 0.05). Besides these differences, the rate of depletion of 16:4(n-1) was the same for krill in each FES tank (p > 0.05). EPA averaged 19.10 µg mg-1 in July and dropped in August and September to an average of 12.53 and 11.19 µg mg-1, respectively, for krill across all four FES tanks (Table 6). DHA averaged 11.42 µg mg-1 in July and fell in August and September to an average of 8.44 and 7.86 µg mg-1, respectively, for krill across all four FES tanks. The fatty acid 16:4(n-1) averaged 1.36 µg mg-1 in July and decreased in August and September to 0.63 and 0.45 µg mg-1, respectively, for krill across all four FES tanks (Table 6).
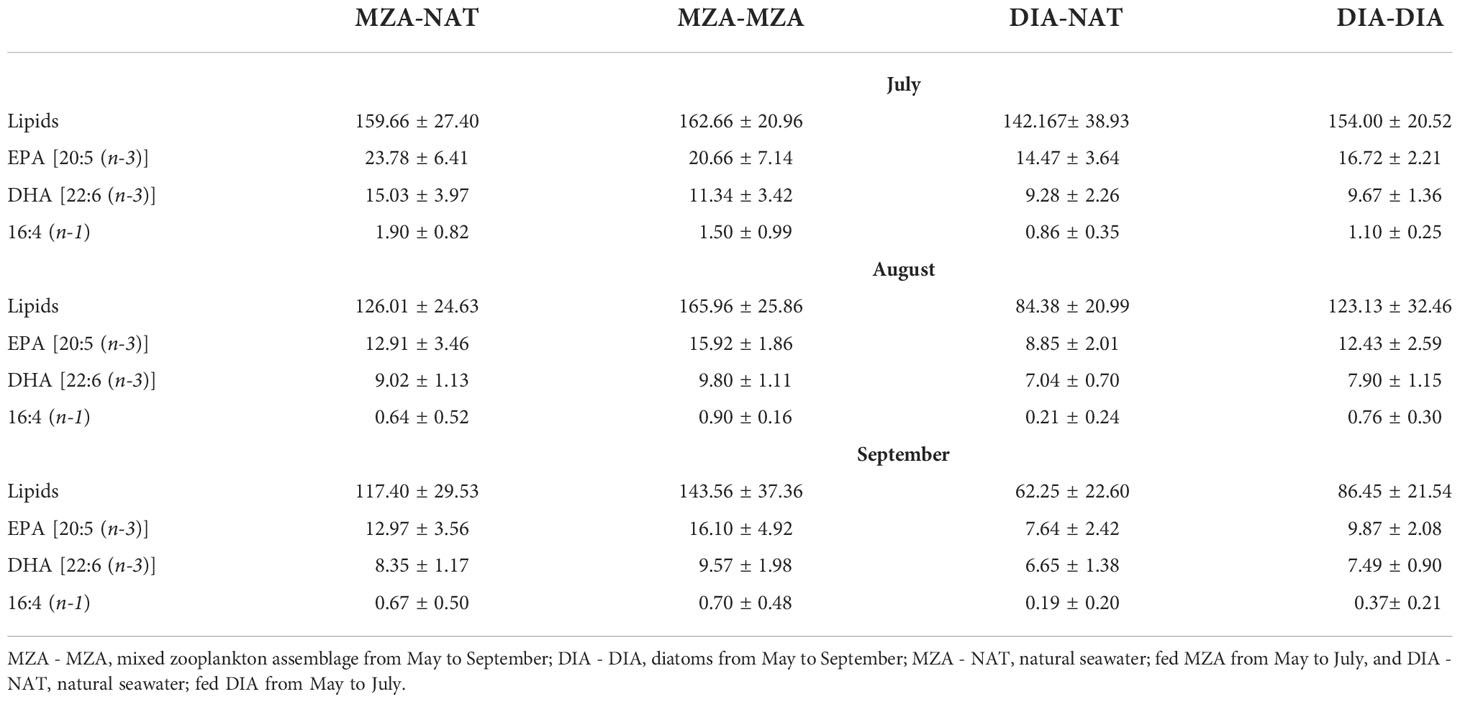
Table 6 The mean and standard deviation of total lipids and the fatty acids EPA, DHA and 16:4 (n-1), (µg mg-1) in in the various food environment scenario (FES) tanks during July, August and September.
Discussion
Reproductive development is a physically demanding process that necessitates an adequate supply of energy in the form of lipids. In this study, we found that the maturation of juvenile female krill occurs during months when they have higher lipid and essential fatty acid content, and that the depletion rate of lipids and fatty acids varies according to a krill’s diet. As krill mature, their respiration rates increase and their physiological condition declines. The trade-off between early reproductive development and condition in juvenile krill can alter the health of an individual, with potential implications on reproductive success at the population-level. In this study we found that as winter progressed, juvenile female krill depleted their lipid and fatty acid reserves, while simultaneously losing their ability to continue sexually maturing. If juveniles are to undergo early reproductive development to completion, they must have sufficient energy to do so. In a concurrent study by Bernard et al. (2022), we show that juvenile krill will feed throughout the winter if food is available to them, while also having the capacity to utilize lipid stores as energy. Juvenile krill with an average dry weight of 20 and 33 mg would experience positive growth or maturation during this time if food concentrations are < 0.05 and 0.10 - 0.15 mg C L-1, respectively (Bernard et al., 2022). In certain regions along the western Antarctic Peninsula (wAP), juvenile krill may experience high enough food concentrations throughout the autumn and winter to carry out early reproductive development (Fritsen et al., 2008; Espinasse et al., 2012; Vernet et al., 2012). Identifying these areas and how they may change in response to a warming climate is critical if we are to fully understand the factors affecting reproductive success in Antarctic krill.
The onset of maturity in juvenile krill
Juvenile krill did not mature past sexual development stage (SDS) 3, early-stage previtellogenesis. This was not surprising, as krill do not typically begin maturing until the spring, when resources are more abundant in the environment (Ross and Quetin, 2000). In this study, we defined this developmental stage as the point at which juveniles reached the onset of maturity. Although some female krill reached SDS 3, most remained in SDS 2, oogenesis. Interestingly, only a handful of female krill were found to be in SDS 1, gametogenesis. Winter is typically a time of low food availability at the wAP, and female krill will revert to immature stages to conserve energy. However, it is more favorable for krill to resist fully reverting to gametogenesis if there is enough food to support a later SDS, because it requires more energy for krill to fully mature from gametogenesis than it would from oogenesis (Kawaguchi, 2016). There may be an adaptive advantage in progressing to a more mature SDS during winter when food is sufficient, so that krill are more prepared for the upcoming spawning season when food becomes abundant again.
The number of juveniles in previtellogenesis was used to estimate the onset of maturity index (OMI), which peaked from July to August. The proportion of krill in previtellogenesis, or the OMI, was positively correlated with their respiration rate. Reproduction in euphausiids is an energy-intensive process and is dependent on the amount of food available prior to and throughout the spawning season (Ross and Quetin, 2000). Some studies have found that Antarctic krill will initiate early maturation and spawning in certain regions in response to early season phytoplankton blooms (Spiridonov, 1995; Siegel, 2012). Krill in our study were feeding throughout the field season, enabling them to obtain a positive growth potential (Bernard et al., 2022) and allocate surplus energy towards reproductive development. When animals exert substantial amounts of energy, their respiration rate increases in response to the work that they are doing (Young and Webster, 1963). In our study, we found that the respiration rate of juvenile krill tracked well with their reproductive development from May to September.
The energetic cost of early reproductive development in juvenile krill
Although juvenile krill can undergo early reproductive development if resources are plentiful, the question remains if it is beneficial to do so. In this study, we found that the condition factor of juvenile krill was lowest when they were more reproductively mature and when their respiration was highest. Although the OMI itself was not significantly correlated with a lowered condition in krill, respiration rate was. Because of this, when juvenile krill put more energy into early sexual maturation, they are more likely to be in worse condition, presumably due to the amount of effort it takes to reach their next SDS.
The decision to initiate reproductive development prior to the end of the winter season can determine whether juvenile females enter spring as more sexually mature krill. Being more mature at the beginning of spring could promote early spawning in juvenile krill, if resources allow (Schmidt et al., 2012). Krill typically undergo several spawning cycles throughout the spring and summer, and an earlier initiation of the spawning season could result in more spawning events in one season (Cuzin-Roudy and Labat, 1992). If this occurs at a population-level, then the physiological response to begin sexually maturing at the end of winter in preparation for spring could lengthen the spawning season and increase the reproductive success of Antarctic krill in the region.
On the other hand, we found that early initiation of reproductive development can negatively impact the condition of juvenile krill. Therefore, it is possible that if krill collectively choose to mature early in response to abundant resources, there could be negative consequences at a population-level. It takes a significant amount of energy to move to a more advanced SDS, which can be physiologically taxing on an individual (Nicol et al., 1995). In general, juvenile krill must maintain higher growth rates than adults so that they can reach a life history stage in which they are able to accumulate more energy reserves in the form of lipids (Atkinson et al., 2006; Kawaguchi et al., 2006). Therefore, funneling all of their energy into sexual maturation, rather than growth, can have consequences on the growth dynamics of a population. Further, juveniles have less robust energy reserves than adults, and are thus more likely to be affected by energy-intensive processes, like reproductive development (Quetin and Ross, 1991). Being in a worse physiological condition can increase the susceptibility of krill to environmental stressors and slow down their growth and reproduction rates (Nicol, 2006).
Lipids and key fatty acids for reproductive development
Total lipids per dry weight found in juvenile krill from July to September were similar to values observed in previous studies (e.g., Hagen et al., 1996). Krill use lipids for growth, reproduction and survival during periods of low food availability (Clarke and Morris, 1983; Quetin et al., 1994; Meyer et al., 2010). Reproductive development is an energy-intensive process and krill were unable to progress past SDS 3 (i.e., early previtellogenesis) in our study. We found that when total lipids were high in krill tissue, there were more krill in SDS 3 than more immature stages (i.e., SDS 1 and 2). Total lipids were higher in July than in August and September, which likely fueled the peak in reproductive development that was observed from July to August. In mid-late winter (July to August) at the WAP food is normally scarce in the environment (Daly, 2004). In preparation for this, krill build up their lipid reserves prior to the onset of winter, when food is more abundant, so that they can harness their lipid reserves for energy when food becomes limiting (Hagen et al., 1996). During our experiment, however, krill were supplemented with food for 2-4 months (depending on their food environment scenario, or FES), buffering the depletion of their lipid reserves. Because of this, juveniles acquired more energy than they needed to carry out their basic metabolic needs and had a positive growth potential (Bernard et al., 2022), evidenced by the development of their oocytes.
The drop in total lipid content over time in all FES tanks, except the MZA-MZA tank, was followed by a decrease in the OMI in September, reflecting the likelihood that juveniles in the MZA-NAT, DIA-NAT and DIA-DIA FES did not have enough energy to sustain reproductive development. When feeding on diatoms, krill acquire high levels of polyunsaturated fatty acids (PUFAs) that they quickly catabolize (Cripps et al., 1999). While PUFAs are useful for growth and reproduction, krill need to accumulate lipids that can be used as energy reserves during periods of low food availability in the environment (e.g., winter). Antarctic krill rely on triacylglycerol lipids (TAGs) to serve as their primary storage lipids, and previous studies have found that Antarctic krill accumulate higher levels of TAGs when feeding carnivorously than they do when feeding exclusively on phytoplankton (Hagen et al., 2007; Schmidt et al., 2014). Krill in the MZA-NAT tank were supplemented with a mixed zooplankton assemblage (MZA) for the first half of the season and switched to feeding on a natural seawater assemblage (NAT) for the second half of the season (July to September). Krill in this FES had significantly reduced their lipid levels from July to September, similar to krill in the DIA-NAT and DIA-DIA tanks. The difference between lipid utilization in the MZA-NAT and MZA-MZA FES suggests that krill must continuously feed on zooplankton in order to keep the lipid storage stable, and that diet throughout autumn and winter is important in shaping the energy allocation of krill during this time period. It takes consistent energy input from the environment for krill to reach previtellogenesis, and previous studies suggest that these energetic levels typically are not observed in situ until the spring bloom occurs (Cuzin-Roudy and Labat, 1992; Quetin and Ross, 2001). The results from our study suggest that feeding on zooplankton throughout the autumn and winter may be an important energy source for juvenile krill upon entering the spawning season.
While krill in the MZA-MZA FES tank did not deplete their lipid levels to the extent that krill in the other FES tanks did, only two krill in the MZA-MZA tank advanced to previtellogenesis in September, indicating that more than just lipid utilization is important for reproductive development. There are certain fatty acids that play a critical role in sexual maturation as well. Three fatty acids in particular: eicosapentaenoic acid (EPA), docosahexaenoic acid (DHA), and 16:4 (n-1) are important in the development of lipidic yolk in krill oocytes (Lee et al., 2006). In addition to serving as components of lipidic yolk, EPA and DHA are vital to the integrity of cell membranes (Virtue et al., 1993). EPA, DHA and 16:4 (n-1) were found in higher amounts in krill tissue in July than later in the season (August and September). Our observations are similar to those found in other studies with higher levels of fatty acids found in krill tissue in the autumn and early winter than in late winter and early spring (Ericson et al., 2018; Walsh et al., 2020). It is plausible that the low levels of these fatty acids in the later months of winter might explain the stunted reproductive development we observed in September.
EPA is typically associated with a diatom-heavy diet (Phleger et al., 2002; Alonzo et al., 2005), however it is notable that our study found EPA content to be relatively similar in krill that were feeding primarily on zooplankton (i.e., MZA-NAT and MZA-MZA) and krill that were feeding primarily on diatoms (i.e., DIA-NAT and DIA-DIA). Further, krill in the MZA-NAT tank were depleting EPA faster than krill in both the DIA-NAT and DIA-DIA tanks. This suggests that krill in the two zooplankton FES tanks were consuming more EPA than krill in the diatom FES tanks. EPA utilization rate was similar for krill in the MZA-MZA tank and MZA-NAT tank, however unlike krill in the MZA-NAT tank, EPA utilization rate for krill in the MZA-MZA tank did not change significantly over time. We believe that this could be because there was more variability in EPA content for krill in the MZA-MZA tank each month than there was for krill in the MZA-NAT tank each month.
DHA is typically associated with a zooplankton or dinoflagellate-heavy diet (Hagen et al., 2007). Although we observed that krill in the two zooplankton FES tanks (i.e., MZA-NAT and MZA-MZA) were depleting DHA at a quicker rate than krill in the two diatom FES tanks (i.e., DIA-NAT and DIA-DIA), we also saw significant decreases in DHA content over time for krill primarily feeding on diatoms. This suggests that krill were consuming DHA in all four FES tanks throughout the duration of the experiment, but were likely consuming more in the zooplankton FES tanks. DHA utilization rate was similar for krill in the MZA-MZA tank and MZA-NAT tank, however unlike krill in the MZA-NAT tank, DHA utilization rate for krill in the MZA-MZA tank did not change significantly over time. Similar to what was observed with EPA content, we believe that this could be because there was more variability in DHA content for krill in the MZA-MZA tank each month than there was for krill in the MZA-NAT tank each month.
The fatty acid 16:4 (n-1) is another fatty acid that is commonly correlated with a diatom-heavy diet (Dalsgaard et al., 2003). However, we found it to be present in similar levels in all krill measured, regardless of the FES they came from. It is possible that the zooplankton in the MZA-NAT and MZA-MZA FES tanks were retaining more of the essential fatty acids (EFAs) from their phytoplanktonic prey prior to being captured for use in this experiment. Kainz et al. (2004) found that larger zooplankton are more likely to retain EFAs, such as EPA, to aid in somatic growth. We assessed the broad taxonomic composition of the MZA food supply and found that it was dominated by large calanoid copepods, such as Calanus propinquus. Krill in all four FES tanks showed significant depletion of 16:4 (n-1) from July to September. Similar to EPA and DHA, krill in the zooplankton FES tanks (i.e., MZA-NAT and MZA-MZA) had a higher 16:4 (n-1) drawdown rate than krill in the diatom FES tanks (i.e., DIA-NAT and DIA-DIA). While previous work has established identifiable differences in prey sources based on their fatty acid signatures (e.g., high EPA is suggestive of a diatom-dominated diet while high DHA is suggestive of a zooplankton-dominated diet), we found that krill feeding primarily on zooplankton throughout the fall and winter had higher drawdown rates of EPA, DHA and 16:4 (n-1) than krill feeding primarily on diatoms. This indicates that juvenile krill may be able to accumulate more EFAs throughout the fall and winter by feeding primarily on large zooplankton than by feeding on diatoms. Ericson et al. (2019) found that krill incorporate more zooplankton into their diet as the seasons progress from summer to winter. There is also the argument that fatty acids that are conserved for somatic growth, such as EPA and DHA, are less useful as dietary biomarkers because they may not be metabolized when krill switch to a new diet (Ericson et al., 2018). Future work on the differences in fatty acid assimilation and utilization by juvenile krill throughout winter should be done to elucidate which food sources are the most energetically rewarding during this time period.
Differences in fatty acid content between FES may have also been influenced by the variable carbon content of the food that juvenile krill were fed throughout the length of the experiment (Supplementary Table 1). Fatty acid percentages (% dry weight of krill) were also measured and can be found in the Supplementary Materials (Supplementary Table 2). Despite differences in lipid and fatty acid drawdown across the four FES tanks, there were no significant differences in the OMI of krill in relation to their FES. This means that krill in all four FES tanks had sufficient levels of fatty acids to initiate reproductive development through August and insufficient levels of fatty acids in September to sustain reproductive development. More studies need to be carried out to decipher the energetic threshold that is needed to begin the process of sexual maturation in Antarctic krill.
Conclusions
Most of the winter research on Antarctic krill at the Western Antarctic Peninsula (WAP) has been on larvae and adults, with little understood about juvenile physiology and condition during this season. This study elucidated that juveniles will allocate their energy towards reproductive development in winter when their lipid levels and reproductively important fatty acids supersede what is necessary for survival. However, sexual development comes at a cost, as juveniles that were more mature were found to be in worse physiological condition. The trade-off between reproductive development and condition in juvenile krill is significant, as early maturation may better prepare krill for the spawning season but may also leave them more susceptible to suboptimal conditions in the environment. Juvenile krill have the capacity to utilize stored lipids throughout the fall and winter that can be used to initiate early reproductive development. While this study focuses on the allocation of energy by juvenile female krill towards sexual maturation in the winter, future work needs to be done to determine the extent to which juvenile krill undergo somatic growth during winter if resources are sufficient to meet their basic metabolic needs. Gaining a better understanding of the mechanisms behind juvenile krill flexibility throughout the fall and winter will allow us to predict how future changes at the wAP may affect krill population dynamics, especially in light of a warming climate.
Data availability statement
The raw data supporting the conclusions of this article will be made available by the authors, without undue reservation.
Author contributions
KS conceived of the research, conducted the field and lab work, analysed data, and wrote the manuscript. KB acquired the funding, designed the experiment, conceived of the research, conducted the field work, analysed data, and contributed to the manuscript. JF conducted the field work, analysed data, and contributed to the manuscript. LC analysed lipid and fatty acid data and contributed to the manuscript. LG analysed data and contributed to the manuscript. All authors contributed to the article and approved the submitted version.
Acknowledgments
This work could not have been accomplished without the logistical support of the US Antarctic Program, the Antarctic Support Contractors at Palmer Station and aboard the ARSV Laurence M. Gould, and the Captain and crew of the ARSV Laurence M. Gould. In addition, we would like to thank G. Morón Correa who provided valuable insight on statistical analyses and model selection used in this study. D. Wildenschild contributed constructive comments regarding techniques carried out in FIJI, ilastik and CellProfiler. We would also like to thank Michelle Stowell, Jessica Andrade, and Kylie Welch for performing lipid extractions and analyses in the Marine Lipid Ecology Laboratory.
Funding
Funding for this study was provided by the United States National Science Foundation (NSF) Office for Polar Programs (OPP) Grant number 1753101.
Conflict of interest
The authors declare that the research was conducted in the absence of any commercial or financial relationships that could be construed as a potential conflict of interest.
The reviewer BM declared a past co-authorship with one of the authors KB to the handling Editor.
Publisher’s note
All claims expressed in this article are solely those of the authors and do not necessarily represent those of their affiliated organizations, or those of the publisher, the editors and the reviewers. Any product that may be evaluated in this article, or claim that may be made by its manufacturer, is not guaranteed or endorsed by the publisher.
Supplementary material
The Supplementary Material for this article can be found online at: https://www.frontiersin.org/articles/10.3389/fmars.2022.1009385/full#supplementary-material
References
Alonzo F., Virtue P., Nicol S., Nichols P. D. (2005). Lipids as trophic markers in Antarctic krill. II. lipid composition of the body and digestive gland of Euphausia superba in controlled conditions. Mar. Ecol. Prog. Ser. 296, 65–79. doi: 10.3354/meps296065
Atkinson A., Meyer B., Stuϋbing D., Hagen W., Schmidt K., Bathmann U. V. (2002). Feeding and energy budgets of Antarctic krill Euphausia superba at the onset of winter-II. juveniles and adults. Limnol. Oceanogr. 47, 953–966. doi: 10.4319/lo.2002.47.4.0953
Atkinson A., Shreeve R. S., Hirst A. G., Rothery P., Tarling G. A., Pond D. W., et al. (2006). Natural growth rates in Antarctic krill (Euphausia superba): II. predictive models based on food, temperature, body length, sex, and maturity stage. Limnol. Oceanogr. 51, 973–987. doi: 10.4319/lo.2006.51.2.0973
Berg S., Kutra D., Kroeger T. (2019). Ilastik: interactive machine learning for (bio)image analysis. Nat. Methods 16, 1226–1232. doi: 10.1038/s41592-019-0582-9
Bernard K. S., Gunther L. A., Mahaffey S. H., Qualls K. M., Sugla M., Saenz B. T., et al. (2019). The contribution of ice algae to the winter energy budget of juvenile Antarctic krill in years with contrasting sea ice conditions. ICES. J. Mar. Sci. 76, 206–216. doi: 10.1093/icesjms/fsy145
Bernard K. S, Steinke K. B, Fontana J. M. (2022). Winter condition, physiology, and growth potential of juvenile Antarctic krill. Front. Mar. Sci. 9, 990853. doi: 10.3389/fmars.2022.990853
Bozdogan H. (1987). Model selection and akaike’s information criterion (AIC): The general theory and its analytical extensions. Psychometrika 52, 345–370. doi: 10.1007/BF02294361
Budge S. M., Iverson S. J., Koopman H. N. (2006). Studying trophic ecology in marine ecosystems using fatty acids: A primer on analysis and interpretation. Mar. Mammal. Sci. 22, 759–801. doi: 10.1111/j.1748-7692.2006.00079.x
Clarke A. (1980). The biochemical composition of krill, Euphausia superba Dana, from south Georgia. J. Exp. Mar. Biol. Ecol. 43, 221–236. doi: 10.1016/0022-0981(80)90049-0
Clarke A., Morris D. J. (1983). Towards an energy budget for krill: The physiology and biochemistry of Euphausia superba Dana | SpringerLink. Polar. Biol. 2, 69–86. doi: 10.1007/BF00303172
Copeman L. A., Laurel B. J., Spencer M., Sremba A. (2017). Temperature impacts on lipid allocation among juvenile gadid species at the pacific Arctic-Boreal interface: an experimental laboratory approach. Mar. Ecol. Prog. Ser. 566, 183–198. doi: 10.3354/meps12040
Cripps G. C., Watkins J., Hill H., Atkinson A. (1999). Fatty acid content of Antarctic krill Euphausia superba at south Georgia related to regional populations and variations in diet. Mar. Ecol. Prog. Ser. 181, 177–188. doi: 10.3354/meps181177
Cuzin-Roudy J. (2000). Seasonal reproduction, multiple spawning, and fecundity in northern krill, Meganyctiphanes norvegica, and Antarctic krill, Euphausia superba. Can. J. Fish. Aquat. Sci. 57, 6–15. doi: 10.1139/f00-165
Cuzin-Roudy J., Amsler M. O. (1991). Ovarian development and sexual maturity staging in Antarctic krill, Euphausia superba Dana (Euphausiacea). J. Crustacean. Biol. 11, 236. doi: 10.2307/1548361
Cuzin-Roudy J., Labat J. P. (1992). Early summer distribution of Antarctic krill sexual development in the Scotia-weddell region: a multivariate approach. Polar Biol. 12, 65–74. doi: 10.1007/978-3-642-77595-6_7
Dalsgaard J., St. John M., Kattner G., Müller-Navarra D., Hagen W. (2003). Fatty acid trophic markers in the pelagic marine environment. Adv. Mar. Biol. 46, 225–340. doi: 10.1016/S0065-2881(03)46005-7
Daly K. L. (2004). Overwintering growth and development of larval Euphausia superba: an interannual comparison under varying environmental conditions west of the Antarctic peninsula. Deep. Sea. Res. Part II: Topical. Stud. Oceanogr. 51, 2139–2168. doi: 10.1016/j.dsr2.2004.07.010
Ericson J. A., Hellessey N., Nichols P. D., Kawaguchi S., Nicol S., Hoem N., et al. (2018). Seasonal and interannual variations in the fatty acid composition of adult Euphausia superba Dana 1850 (Euphausiacea) samples derived from the Scotia Sea krill fishery. J. Crustacean. Biol. 38, 662–672. doi: 10.1093/jcbiol/ruy032
Ericson J. A., Hellessey N., Nichols P. D., Nicol S., Kawaguchi S., Hoem N., et al. (2019). New insights into the seasonal diet of Antarctic krill using triacylglycerol and phospholipid fatty acids, and sterol composition. Polar. Biol. 42, 1985–1996. doi: 10.1007/s00300-019-02573-6
Espinasse B., Zhou M., Zhu Y., Hazen E. L., Friedlaender A. S., Nowacek D. P., et al. (2012). Austral fall–winter transition of mesozooplankton assemblages and krill aggregations in an embayment west of the Antarctic peninsula. Mar. Ecol. Prog. Ser. 452, 63–80. doi: 10.3354/meps09626
Folch J., Less M., Sloane Stanley G. H. (1956). A simple method for the isolation and purification of total lipids from animal tissues. J. Biol. Chem. 22, 497–509.
Fritsen C. H., Memmott J. C., Ross R. M., Quetin L. B., Vernet M., Wirthlin E. D. (2011). The timing of sea ice formation and exposure to photosynthetically active radiation along the Western Antarctic peninsula. Polar. Biol. Heidelberg. 34, 683–692. doi: 10.1007/s00300-010-0924-7
Fritsen C. H., Memmott J., Stewart F. J. (2008). Inter-annual sea-ice dynamics and micro-algal biomass in winter pack ice of Marguerite bay, Antarctica. Deep. Sea. Res. Part II: Topical. Stud. Oceanogr. 55, 2059–2067. doi: 10.1016/j.dsr2.2008.04.034
Garrison D. L., Gibson A., Coale S. L., Gowing M. M., Okolodkov Y. B., Fritsen C. H., et al. (2005). Sea-Ice microbial communities in the Ross Sea: autumn and summer biota. Mar. Ecol. Prog. Ser. 300, 39–52. doi: 10.3354/meps300039
Gleiber M. (2014). Long-term change in copepod community structure in the Western Antarctic peninsula: Linkage to climate and implications for carbon cycling. Master's Thesis, College of William and Mary, Virginia Institute of Marine Science. doi: 10.25773/v5-we5s-aq55
Hagen W. (1999). Reproductive strategies and energetic adaptations of polar zooplankton. Invertebrate. Reprod. Dev. 36, 25–34. doi: 10.1080/07924259.1999.9652674
Hagen W., Van Vleet E. S., Kattner G. (1996). Seasonal lipid storage as overwintering strategy of Antarctic krill. Mar. Ecol. Prog. Ser. 134, 85–89. doi: 10.3354/meps134085
Hagen W., Yoshida T., Virtue P., Kawaguchi S., Swadling K. M., Nicol S., et al. (2007). Effect of a carnivorous diet on the lipids, fatty acids and condition of Antarctic krill, Euphausia superba. Antarctic. Sci. 19, 183–188. doi: 10.1017/S0954102007000259
Ikeda T., Dixon P. (1982). Body shrinkage as a possible over-wintering mechanism of the Antarctic krill, Euphausia superba Dana. J. Exp. Mar. Biol. Ecol. 62, 143–151. doi: 10.1016/0022-0981(82)90088-0
Ikeda T., Torres J. J., Hernández-León S., Geiger S. P. (2000). “Metabolism,” in ICES zooplankton methodology manual (San Diego: Academic Press), 455–532.
Kainz M., Arts M. T., Mazumder A. (2004). Essential fatty acids in the planktonic food web and their ecological role for higher trophic levels. Limnol. Oceanogr. 49, 1784–1793. doi: 10.4319/lo.2004.49.5.1784
Kawaguchi S. (2016). “Reproduction and larval development in Antarctic krill (Euphausia superba),” in Biology and ecology of Antarctic krill advances in polar ecology. Ed. Siegel V. (Cham: Springer International Publishing), 225–246. doi: 10.1007/978-3-319-29279-3_6
Kawaguchi S., Candy S., King R., Naganobu M., Nicol S. (2006). Modelling growth of Antarctic krill. i. growth trends with sex, length, season, and region. Mar. Ecol. Prog. Ser. 306, 1–15. doi: 10.3354/meps306001
Kawaguchi S., Yoshida T., Finley L., Cramp P., Nicol S. (2007). The krill maturity cycle: a conceptual model of the seasonal cycle in Antarctic krill. Polar. Biol. 30, 689–698. doi: 10.1007/s00300-006-0226-2
Lee R., Hagen W., Kattner G. (2006). Lipid storage in marine zooplankton. Mar. Ecol. Prog. Ser. 307, 273–306. doi: 10.3354/meps307273
Ligowski R. (2000). Benthic feeding by krill, Euphausia superba Dana, in coastal waters off West Antarctica and in admiralty bay, south Shetland islands. Polar. Biol. 23, 619–625. doi: 10.1007/s003000000131
Lu Y., Ludsin S. A., Fanslow D. L., Pothoven S. A. (2008). Comparison of three microquantity techniques for measuring total lipids in fish. Can. J. Fish. Aquat. Sci. 65, 2233–2241. doi: 10.1139/F08-135
Makarov R., Denys C. J. (1984). Stages of sexual maturity of Euphausia superba Dana. In: BIOMASS Handbook. Oban, United Kingdom: BIOMASS Handbook 11, 1–11. SCAR/SCOR.IABO/ACMRR
Mauchline J. (1981). Measurement of body-length of Euphausia superba Dana. BIOMASS Handbook. Oban, United Kingdom: BIOMASS Handbook 4, 1–9. SCAR/SCOR.IABO/ACMRR
McQuin C., Goodman A., Chernyshev V., Kamentsky L., Cimini B. A., Karhohs K. W., et al. (2018). CellProfiler 3.0: Next-generation image processing for biology. PloS Biol. 16, 1–17. doi: 10.1371/journal.pbio.2005970
Meyer B. (2012). The overwintering of Antarctic krill, Euphausia superba, from an ecophysiological perspective. Polar. Biol. 35, 15–37. doi: 10.1007/s00300-011-1120-0
Meyer B., Auerswald L., Siegel V., Spahic C., Pape C., Fach B., et al. (2010). Seasonal variation in body composition, metabolic activity, feeding, and growth of adult krill Euphausia superba in the lazarev Sea. Mar. Ecol. Prog. Ser. 398, 1–18. doi: 10.3354/meps08371
Meyer B., Fuentes V., Guerra C., Schmidt K., Atkinson A., Spahic S., et al. (2009). Physiology, growth, and development of larval krill Euphausia superba in autumn and winter in the lazarev Sea, Antarctica. Limnol. Oceanogr. 54, 1595–1614. doi: 10.4319/lo.2009.54.5.1595
Meyer B., Teschke M. (2016). “Physiology of Euphausia superba,” in Biology and ecology of Antarctic krill advances in polar ecology. Ed. Siegel V. (Cham: Springer International Publishing), 145–174. doi: 10.1007/978-3-319-29279-3_4
Nicol S. (2006). Krill, currents, and Sea ice: Euphausia superba and its changing environment. BioScience 56, 111–120. doi: 10.1641/0006-3568(2006)056[0111:KCASIE]2.0.CO;2
Nicol S. (2007). Learning about Antarctic krill from the fishery. Antarct. Sci. 19, 219–230. doi: 10.1017/S0954102007000296
Nicol S., de la Mare W. K., Stolp M. (1995). The energetic cost of egg production in Antarctic krill (Euphausia superba Dana). Antartic. Sci. 7, 25–30. doi: 10.1017/S0954102095000058
Parrish C. C. (1987). Separation of Aquatic Lipid Classes by Chromarod Thin-Layer Chromatography with Measurement by latroscan Flame Ionization Detection. Can. J. Fish. Aquat. Sci. 44, 722–731.
Phleger C. F., Nelson M. M., Mooney B. D., Nichols P. D. (2002). Interannual and between species comparison of the lipids, fatty acids and sterols of Antarctic krill from the US AMLR Elephant Island survey area. Comp. Biochem. Physiol. Part B.: Biochem. Mol. Biol. 131, 733–747. doi: 10.1016/S1096-4959(02)00021-0
Pond D. W., Atkinson A., Shreeve R. S., Tarling G., Ward P. (2005). Diatom fatty acid biomarkers indicate recent growth rates in Antarctic krill. Limnol. Oceanogr. 50, 732–736. doi: 10.4319/lo.2005.50.2.0732
Quetin L. B., Ross R. M. (1991). Behavioral and physiological characteristics of the Antarctic krill, Euphausia superba. Am. Zool. 31, 49–63. doi: 10.1093/icb/31.1.49
Quetin L. B., Ross R. M. (2001). Environmental variability and its impact on the reproductive cycle of Antarctic krill. Am. Zool. 41, 74–89. doi: 10.1093/icb/41.1.74
Quetin L. B., Ross R. M. (2003). Episodic recruitment in Antarctic krill Euphausia superba in the palmer LTER study region. Mar. Ecol. Prog. Ser. 259, 185–200. doi: 10.3354/meps259185
Quetin L. B., Ross R. M., Clarke A. (1994). “Krill energetics: seasonal and environmental aspects of the physiology of Euphausia superba,” in Southern ocean ecology. the BIOMASS perspective. Ed. El-Sayed S. Z. (Cambridge: Cambridge University Press).
Ross R. M., Quetin L. B. (2000). “Reproduction in Euphausiacea,” in Krill. Ed. Everson I. (Oxford, UK: Blackwell Science Ltd), 150–181. doi: 10.1002/9780470999493.ch6
RStudio Team (2022) RStudio: Integrated development environment for r. Available at: http://www.rstudio.com/.
Schaafsma F., Kohlbach D., David C., Lange B., Graeve M., Flores H., et al. (2017). Spatio-temporal variability in the winter diet of larval and juvenile Antarctic krill, Euphausia superba, in ice-covered waters. Mar. Ecol. Prog. Ser. 580, 101–115. doi: 10.3354/meps12309
Schmidt K., Atkinson A., Pond D. W., Ireland L. C. (2014). Feeding and overwintering of Antarctic krill across its major habitats: The role of ice cover, water depth, and phytoplankton abundance. Limnol. Oceanogr. 59, 17–36. doi: 10.4319/lo.2014.59.1.0017
Schmidt K., Atkinson A., Steigenberger S., Fielding S., Lindsay M. C. M., Pond D. W., et al. (2011). Seabed foraging by Antarctic krill: Implications for stock assessment, bentho-pelagic coupling, and the vertical transfer of iron. Limnol. Oceanogr. 56, 1411–1428. doi: 10.4319/lo.2011.56.4.1411
Schmidt K., Atkinson A., Venables H. J., Pond D. W. (2012). Early spawning of Antarctic krill in the Scotia Sea is fuelled by “superfluous” feeding on non-ice associated phytoplankton blooms. Deep. Sea. Res. Part II: Topical. Stud. Oceanogr. 59-60, 159–172. doi: 10.1016/j.dsr2.2011.05.002
Siegel V. (2012). Krill stocks in high latitudes of the Antarctic lazarev Sea: seasonal and interannual variation in distribution, abundance and demography. Polar. Biol. 35, 1151–1177. doi: 10.1007/s00300-012-1162-y
Siegel V., Loeb V. J. (1994). Length and age at maturity of Antarctic krill. Antarctic. Sci. 6, 479–482. doi: 10.1017/S0954102094000726
Spiridonov V. A. (1995). Spatial and temporal variability in reproductive timing of Antarctic krill (Euphausia superba Dana). Polar. Biol. 15, 161–174. doi: 10.1007/BF00239056
Stammerjohn S. E., Martinson D. G., Smith R. C., Iannuzzi R. A. (2008). Sea Ice in the western Antarctic peninsula region: Spatio-temporal variability from ecological and climate change perspectives. Deep. Sea. Res. Part II: Topical. Stud. Oceanogr. 55, 2041–2058. doi: 10.1016/j.dsr2.2008.04.026
Steinke K., Bernard K., Ross R., Quetin L. (2021). Environmental drivers of the physiological condition of mature female Antarctic krill during the spawning season: implications for krill recruitment. Mar. Ecol. Prog. Ser. 669, 65–82. doi: 10.3354/meps13720
Vaughan D. G., Marshall G. J., Connolley W. M., Parkinson C., Mulvaney R., Hodgson D. A., et al. (2003). Recent rapid regional climate warming on the Antarctic peninsula. Clim. Change 60, 243–274. doi: 10.1023/A:1026021217991
Vernet M., Kozlowski W. A., Yarmey L. R., Lowe A. T., Ross R. M., Quetin L. B., et al. (2012). Primary production throughout austral fall, during a time of decreasing daylength in the western Antarctic peninsula. Mar. Ecol. Prog. Ser. 452, 45–61. doi: 10.3354/meps09704
Virtue P., Nichols P. D., Nicol S., McMinn A., Sikes E. L. (1993). The lipid composition of Euphausia superba Dana in relation to the nutritional value of phaeocystis pouchetii (Hariot) lagerheim. Antarctic. Sci. 5, 169–177. doi: 10.1017/S0954102093000239
Walsh J., Reiss C., Watters G. (2020). Flexibility in Antarctic krill Euphausia superba decouples diet and recruitment from overwinter sea-ice conditions in the northern Antarctic peninsula. Mar. Ecol. Prog. Ser. 642, 1–19. doi: 10.3354/meps13325
Wasta Z., Mjøs S. A. (2013). A database of chromatographic properties and mass spectra of fatty acid methyl esters from omega-3 products. J. Chromatogr. A. 1299, 94–102. doi: 10.1016/j.chroma.2013.05.056
Yoshida T. (2009). Environmental influences on reproduction in Antarctic krill, Euphausia superba. PhD Thesis, University of Tasmania.
Keywords: Antarctic krill, reproduction, energy, juvenile, trade-off, body condition
Citation: Steinke KB, Bernard KS, Fontana JM, Copeman LA and Garcia LM (2022) The energetic cost of early reproductive development in juvenile Antarctic krill at the Western Antarctic Peninsula. Front. Mar. Sci. 9:1009385. doi: 10.3389/fmars.2022.1009385
Received: 01 August 2022; Accepted: 22 November 2022;
Published: 09 December 2022.
Edited by:
Vitor H. Paiva, University of Coimbra, PortugalReviewed by:
Nicole G. Hellessey, Georgia Institute of Technology, United StatesBettina Meyer, Alfred Wegener Institute Helmholtz Centre for Polar and Marine Research (AWI), Germany
So Kawaguchi, Australian Antarctic Division, Australia
Copyright © 2022 Steinke, Bernard, Fontana, Copeman and Garcia. This is an open-access article distributed under the terms of the Creative Commons Attribution License (CC BY). The use, distribution or reproduction in other forums is permitted, provided the original author(s) and the copyright owner(s) are credited and that the original publication in this journal is cited, in accordance with accepted academic practice. No use, distribution or reproduction is permitted which does not comply with these terms.
*Correspondence: Kirsten B. Steinke, c3RlaW5ra2lAb3JlZ29uc3RhdGUuZWR1