- 1Conservation Ecology Group, Groningen Institute for Evolutionary Life Sciences, University of Groningen, Groningen, Netherlands
- 2Département Observatoire, Parc National du Banc d’Arguin (PNBA), Chami, Mauritania
- 3Department of Earth Sciences, Faculty of Geosciences, Utrecht University, Utrecht, Netherlands
- 4Department of Estuarine and Delta Systems, Royal Netherlands Institute for Sea Research (NIOZ), Yerseke, Netherlands
- 5Department of Coastal Systems, Royal Netherlands Institute for Sea Research (NIOZ), Texel, Netherlands
- 6Department of Physical Geography, Faculty of Geosciences, Utrecht University, Utrecht, Netherlands
- 7Aix Marseille Uni, CNRS Minist Culture, LAMPEA, Aix-en-Provence, France
- 8School of Environmental Sciences, University of Liverpool, Liverpool, United Kingdom
Coastal systems store enormous carbon quantities in their sediment, which originates from various autochthonous and allochthonous sources. Carbon fluxes in coastal ecosystems have a strong effect on the recipient food-webs and carbon emission offsets. Yet, the relative importance of autochthonous vs. allochthonous C inputs to coastal carbon budget is still challenging to identify. Here, we combine diatoms preserved in the sediment with geochemical analyses to identify the sources of carbon stored in Africa’s largest intertidal seagrass beds at Banc d’Arguin, Mauritania. The area lies between an active ocean upwelling and the ‘Sahara-dust hotspot’ systems. The extensive seagrass beds of the area are thus expected to receive C from these neighboring systems in addition to producing C in-situ. Three sediment cores (50 cm) were collected at three intertidal sites with different hydrodynamic regimes, and analyzed for diatom composition, total organic carbon (TOC), total nitrogen (TN), total phosphorus (TP), and carbon isotopic signatures (δ13C). Diatom taxa are grouped into three guilds: (1) benthic (epiphyte, epipelon, and epipsammon), (2) planktonic, and (3) freshwater. Benthic diatoms are considered to be autochthonous, while typical oceanic and freshwater diatoms are considered to be allochthonous. Benthic diatoms are the most diverse and abundant group, while allochthonous freshwater (i.e., dust imported) and typical upwelling (i.e., tidal imported) taxa ranked last in both abundance and species’ richness. Structure equation modelling shows that variation in the stored carbon is best explained by the total abundance of diatoms and guild composition. We conclude that the C stored in the intertidal seagrass beds of Banc d’Arguin is predominantly autochthonous. Our method provides an effective way to identify historical carbon sources in coastal systems.
Introduction
Coastal systems play a crucial role worldwide as habitats for birds, fish, and other coastal fauna and flora as well as carbon sinks. These coastal systems are supported by organic carbon of different origins (Reef et al., 2017). For instant, iconic intertidal habitats such as seagrass beds, saltmarshes, and mangrove forests are generally characterized by high productivity as well as by high sediment trapping capacity (Phang et al., 2015). Thus, C in these coastal sediments is either locally produced (autochthonous) or comes from elsewhere (allochthonous) by tidal and fluvial transport and atmospheric deposition (Duarte et al., 2013; Hayes et al., 2017). Allochthonous C can contribute significantly to the growth of different trophic levels of the recipient habitats (Polis et al., 1997; Garcia et al., 2019). It is hence not surprising that in a recent review authored by 36 leading scientists in the field of carbon research, the quantification of the sources of carbon sequestration was ranked as one of the ten key fundamental questions in this field that must be urgently addressed (MacReadie et al., 2019).
Vegetated coastal ecosystems such as seagrass, salt marshes, and mangroves are major carbon (C) sinks and play a significant role in buffering global warming. Although these coastal ecosystems occupy less than 2% of the marine biome, they account for 50% of all green carbon burial in marine sediments (Nellemann et al., 2009). The origin of this high carbon sequestration is often from different sources. For instance, the efficiency of seagrass beds at storing carbon is primarily due to their capacity to (1) produce large quantity of C as detritus (leaves, roots, and rhizomes) (Pergent et al., 1994); (2) capture suspended material (sediment and/or carbon) from tidal water with their aboveground biomass (Bouma et al., 2005); (3) stabilize and bury both locally produced and allochthonous captured C with the aid of the usually complex belowground structure and relatively fast rate of sediment accretion (Bos et al., 2007); and (4) preserve buried C within anoxic sediment conditions that reduce its decomposition rate and thus the loss of C (Sun et al., 2020). As a consequence, seagrass beds across the globe store large quantities of both allochthonous and autochthonous C that accumulated in most cases over millennia (Duarte et al., 2010; Fourqurean et al., 2012). However, reliable reconstructions of seagrass presence and their long-term contributions to carbon sequestration remain challenging, as plant material of marine angiosperm generally do not preserve well in the sediment (Tuya et al., 2017), with few exceptions such as the Mediterranean seagrass, Posidonia oceanica, that is known to form mats and preserve for thousands of years (López-Merino et al., 2017). Hence, assessment of seagrass in the geological record has typically involved indirect indicators such as geochemical and sediment indexes, i.e., stable isotopes, elemental, lipid biomarkers, and environmental DNA (Reich et al., 2015; Forsey, 2016). Thus, there is a need for novel alternative ways to identify the origin of historical-accumulated carbon sources in seagrass ecosystems.
Carbon isotopes (δ13C) and total carbon to nitrogen (C/N) ratios are the most commonly used techniques for assessing sources of C in the sediment (Phillips and Gregg, 2003). Carbon isotopes have widely been used to differentiate between C3 and C4 photosynthetic pathways in plants as well as the fractionation of δ13C between atmospheric (terrestrial plants) and dissolved sources of CO2 (plankton and aquatic plants). Most seagrass species belong to C3 pathway group although some species appear to be C3–C4 intermediates (Touchette and Burkholder, 2000). Further, the C/N ratio is also an indicator of the C origin (terrestrial versus marine) in coastal sediments (Lamb et al., 2006). Overall, marine and terrestrial sources have distinct carbon isotopic and elemental signatures (France, 1995). Stable isotopes signatures can be used in Bayesian mixing models to estimate the contributions of different primary producers (Kennedy et al., 2010; Bulmer et al., 2020). The main disadvantage of these methods is the great overlap in isotopic and elemental signatures among species from the same biome, beside that these signatures are known to change with life stages, tissue types, and over seasons (Geraldi et al., 2019). Lipid biomarkers such as fatty acids and alkanes have also been used to infer past and present coastal C sources (Bianchi et al., 2016; Geraldi et al., 2019). For example, the terrestrial-to-aquatic ratio of fatty acid (TARFA) is useful to evaluate the relative contributions of terrigenous and aquatic C stocks (Geraldi et al., 2019). The main inconvenience of biomarkers is that their concentrations in the sediment may be dependent on the degree of sediment oxygenation (Bianchi et al., 2016). Moreover, some biomarkers originate from both allochthonous and autochthonous sources (Waterson and Canuel, 2008). Finally, the environmental DNA (eDNA) technique has been recently used to accurately assign stored C to its sources (Reef et al., 2017; Ortega et al., 2020). The main drawback of this technique is that eDNA reads depend heavily on the selection of primers. Besides that, DNA of marine plankton origin is known to undergo faster degradation than that of terrestrial origin (Boere et al., 2011).
Seagrass associated organisms, such as a variety of epiphytes including diatoms (Vermaat and Verhagen, 1996), are known to often preserve well in sediment (Vos and de Wolf, 1993). In addition, epiphytic and microphytobenthic algae contribute significantly to seagrass meadow productivity, up to 62 and 54% respectively (Borowitzka et al., 2006; Lebreton et al., 2009). Moreover, it is possible to distinguish 3 diatom guilds representing important carbon sources: (1) benthic locally produced (autochthonous), (2) allochthonous planktonic coming from the open seaward area and (3) allochthonous freshwater coming from the landward area. In this study, we aim to combine fossilized diatoms with geochemical analyses in sediments to backtrack the sources of the stored organic carbon in the intertidal seagrass beds of the Parc National du Banc d’Arguin (Mauritania).
The study was conducted at the Parc National du Banc d’Arguin, protecting a 12,000 km2 wetland along the coast of Mauritania (Figure 1). Banc d’Arguin contains the largest intertidal seagrass beds in Africa and is considered among the most pristine in the world (UNESCO, 2020). The dominant intertidal seagrass species in term of biomass and cover, Zostera noltei, has been considered the most important primary producer in the area (Clavier et al., 2014), with microphytobenthos being important on bare mudflats. The park is surrounded by two important systems that can significantly contribute to its carbon budget: (1) the ocean upwelling of Cap Blanc, which is one of the most productive in the world (Wefer and Fischer, 1993) and is characterized by an intense exchange of neritic and pelagic water masses (van Camp et al., 1991; Romero et al., 2020; Romero et al., 2021); (2) the Sahara dust hotspots, which are known to export large quantities of organic carbon and nutrients westwards to regions as far as the Amazon Forest in Brazil (Prospero et al., 1981; Ben-Ami et al., 2010; Barkley et al., 2021). Both the upwelling (Romero et al., 2020) and Saharan dust (Pokras, 1991; Romero et al., 2003; Barkley et al., 2021) are known to transport large quantities of planktonic and freshwater diatoms that are indicators of their origin. The food web of the inner intertidal system of Banc d’Arguin, however, has been suggested to rely predominately on in-situ benthic primary production than on transported C sources (Wolff et al., 1993; Carlier et al., 2015). However, these shallow inner intertidal flats represent relatively recent deposition (ca. 800 cal. yr. BP) (Proske et al., 2008), and seagrass stands were found only in the youngest part of these intertidal flats (Proske et al., 2008). The origin of the longer-term carbon sequestration thus remains unknown.
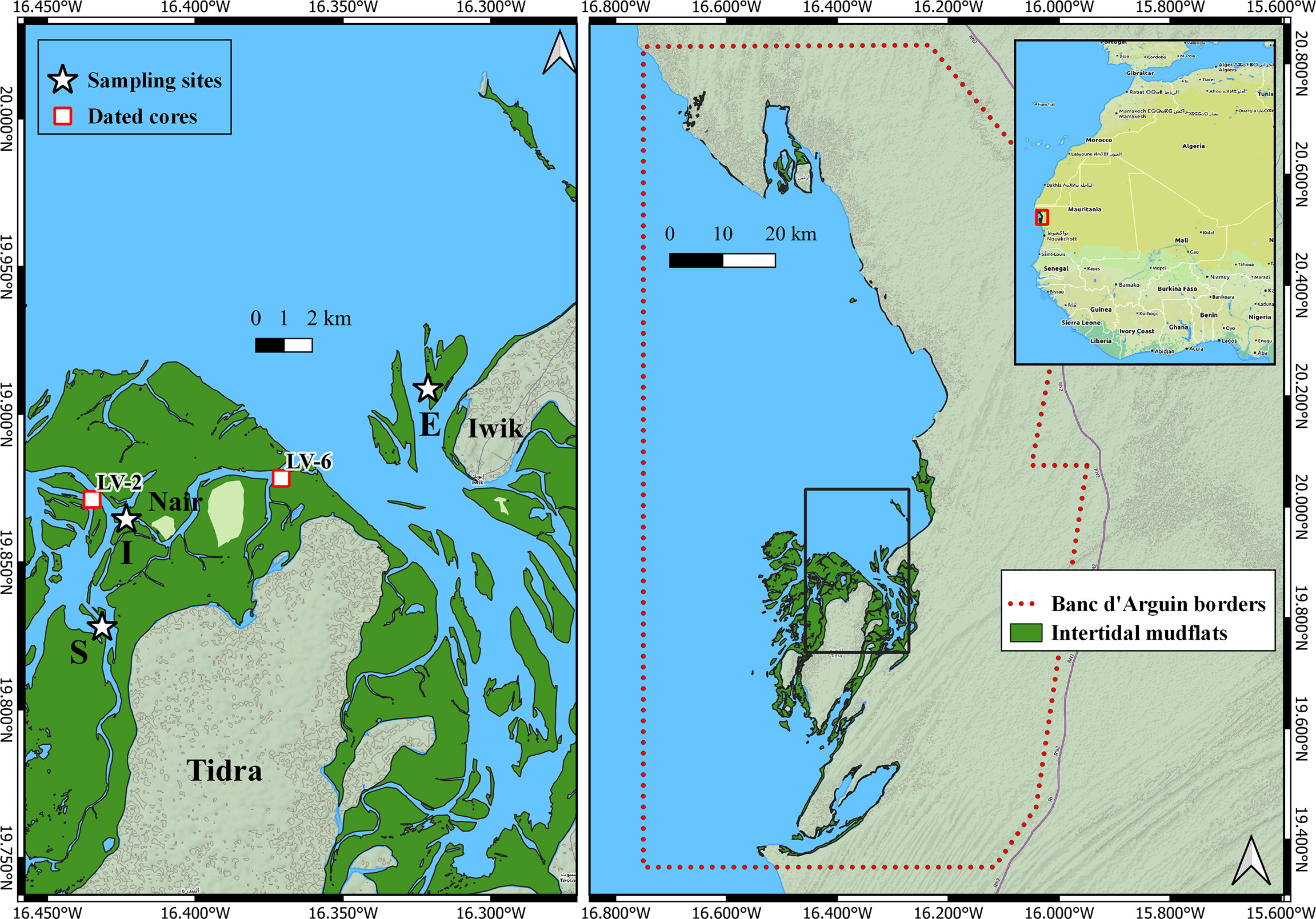
Figure 1 Map of the Parc National du Banc d’Arguin, Mauritania, with sampling locations: black stars indicate where cores were taken for carbon, diatom and geochemical analyses; while red squares represent site where dated cores were previously taken. The sampling sites represent three distinct wave action regimes: E = Exposed (19°54′34″ N, 16°19′10″ W), I = Intermediate (19°51′52″ N, 16°25′26″ W) and S = Sheltered (19°49′39″ N, 16°25′54″ W) (El-Hacen et al., 2019).
Materials and methods
Sample collection
Sampling locations were chosen to represent different hydrodynamic regimes: exposed, intermediate, and sheltered from wave energy (El-Hacen et al., 2019). At each location (Figure 1), a sediment push core was taken in January 2015. The cores had a length of 50 cm and an inner diameter of 2.5 cm. Each core was divided in slices of 2 cm, resulting in 25 depth samples per core. Each depth sample was oven dried, stored in plastic containers and transported to the Netherlands for further analyses.
Age determination of core sediments
210Pb dating of the core was performed but the unsupported levels of 210Pb were too low, and the data could not be used to determine the age of the sediments. Instead, we estimated the age of our samples using the data of two cores taken in close proximity of our sampling stations and which were dated using 14C (Proske et al., 2008). These 14C ages are based on deep shell layers from the sedimentary succession. Using radiocarbon dates at 239 cm depth in core LV-6 and 248 and 159 cm in core LV-2, sedimentation rates of 0.5 cm/yr. and 0.3-1.1 cm/yr. were obtained for LV-6 and LV-2, respectively (Supplementary 1). The age-depth model (cal. yr. BP, ± 2σ) of core LV-6 was extrapolated to the core collected at the wave exposed site due to their proximity and similarity in wave energy index (El-Hacen et al., 2019). Similarly, the age-depth model of core LV-2 was extrapolated to the cores of the intermediate and wave sheltered sites. This extrapolation, however, should be interpreted with caution (transfer from one core to another, sediment compaction, and large confidence interval of the calibrated radiocarbon dates) and will only serve as an indication of the possible time interval represented in our push cores for discussion.
Diatom analyses
Of the 25 depth samples in each push core, fifteen per core were selected for diatom analysis. Subsamples of ± 0.75 g were taken and prepared for siliceous microfossil analysis at the University of Utrecht, The Netherlands. Each subsample was oxidized with 1.5 mL permanganate (KMnO4) to remove organic matter and treated with 3 mL 30% HCL to remove carbonates. Then, the subsamples were rinsed and diluted ten times with distilled water. Diatoms were left to settle on coverslips overnight using Battarbee evaporation trays to optimize random distribution (Battarbee, 1973). The coverslips were then permanently mounted on slides in Naphrax® (RI = 1.7). A Leica DM2500 microscope was used to count diatoms and photos were taken with a Leica DFC320 and an Olympus DP25. At each depth level, the conventional counting of 300 diatom valves following Schrader & Gersonde (1978) was used. These counts were then used to calculate taxon percentages. The objective was set to 63x and the Optovar to 1.5x. Thus, the eyepiece-micrometer reticule had 1.05 μm divisions and the area counted could be calculated. The diatom relative abundance in valves/g can be calculated as:
Taxonomical identification was based on well-known bibliographies (Halse and Syvertsen, 1996; Witkowski et al., 2000) and online databases such as algaebase.org, www.diatombase.org and the World Register of Marine Species (WoRMS). In total, 43 specimen-type were identified to the species level, 61 to the genus level, and other twelve-types could not be identified (Table S1, Supplementary 2). In addition, identified diatom species and genera were grouped (Table S1) into three guilds (benthic, planktonic, and freshwater) based on previous studies on diatoms in the region (Margalef, 1975; Sterrenburg and Sterrenburg, 1990; Vos and de Wolf, 1993; Romero et al., 1999; Romero et al., 2003; Bernárdez et al., 2010; Romero and Fischer, 2017; Romero et al., 2020; Romero et al., 2021). Benthic taxa were further divided into two groups: (1) epiphytes that grow attached to seagrass leaves and (2) non-epiphytic that grow on the sediment surface.
Geochemical analyses
Ground sediment subsamples of each depth levels were analyzed at NIOZ, Yerseke, The Netherlands. Total organic carbon (TOC), total nitrogen (TN), and δ13C were measured simultaneously by a CNS analyzer (Vario MACRO CNS) on 5 mg of sediment per subsample (n = 25/core; every 2 cm).
Statistical analyses
All statistical analyses were performed in R (v. 4.0.2) (R Development Core Team, Vienna, Austria) in RStudio environment (v. 1.3.1073) (RStudio Inc., Boston, USA). Changes in the vertical (n = 25) abundances of the most common diatom guilds were evaluated using change point analysis with the ‘changepoint’ package in R (Killick and Eckley, 2014). Change point analysis identifies statistically significant changes in the mean or variance within series of observations based on likelihood ratios (Killick and Eckley, 2014). Linear regression analysis was used to test if the total diatom abundance as well as the abundances of the different guilds significantly explain the carbon stocks over time. One-way analysis of variance (ANOVA) and post hoc Tukey test were used to analyze the effect of site (n = 3) on both sediment carbon isotopes (δ13C) and C/N ratios (n =25) as an indication for the sources of carbon in the system. Parametric assumptions were checked on the residuals.
Additionally, we performed a multi-level piecewise structural equation modelling (piecewise SEM) to simultaneously assess the relative importance of diatom diversity (richness, abundance, and community structure) and environmental conditions (depth and source of carbon) on carbon stocks (n = 75: 25 per site). SEM was conducted in ‘piecewiseSEM’ R package, which can account for hierarchically structured data by incorporating mixing effect modelling approach (Lefcheck, 2016). A priori meta-model was developed, based upon known knowledge on factors controlling C stocks in intertidal systems (Figure S1, Supplementary 3), which was improved by removing non-significant paths using Akaike’s information criterion (AICc) (Grace et al., 2010; Lefcheck, 2016). The goodness of fit of the final SEM was assessed using directional separation test (d-separations test) Fisher’s C statistic (Shipley, 2013). Homogeneity of variance was checked visually using residuals plots (Figure S2). To characterize diatom community composition, the first two axes of Redundancy analysis (RDA) were used as a proxy. RDA analyses relate trait-environmental relationship at the community level (Lepš and Šmilauer, 2003; Legendre et al., 2011; Legendre et al., 2012). RDA first and second scores describe assemblages in terms of similarities in traits and responses to environmental gradients (Kleyer et al., 2012; Guillaume Blanchet et al., 2014), and have been used before as a proxy for community composition (Widenfalk et al., 2015; Sgro and Reavie, 2018). RDA analysis was performed using the ‘vegan’ package on Hellinger distance transformed data, to reduce the influence of the most abundant taxa. Prior RDA procedure, a detrended correspondence analysis (DCA) on diatom abundance data resulted in very short gradient lengths (< 0.2), suggesting that RDA analysis was more appropriate than non-linear approach such as NMDS (Šmilauer and Lepš, 2014).
Results
In total, 76 different diatom taxa were identified at the exposed site, 48 at the intermediate, and 93 at the sheltered site (Supplementary 2). Across sites, the tychopelagic (grows predominantly benthic and is easily lifted into planktonic form) species, Paralia sulcata, was by far the most common, accounting for 33, 74, and 36% of the total recovered taxa at the exposed, intermediate, and sheltered sites, respectively. At the wave-exposed site, epiphytic (56-59%) and freshwater (10-25%) taxa were the most abundant in the upper layers (0-4 cm), whereas benthic non-epiphytic (growing on sediment) (34-85%) and planktonic (12-50%) taxa dominated down-core (Figure 2, Figure S3). The intermediate site was barren of diatoms in the lower part (34-50 cm) of the core, and benthic non-epiphytic taxa (73-86%) dominated above 34 cm, with two small peaks of freshwater taxa (10 and 6%, respectively) and a very recent peak of epiphyte taxa (21%) (Figure 2, Figure S3). At the wave-sheltered site, epiphyte (21-42%) and freshwater (7-21%) taxa were the most abundant in the upper layers (0-14 cm), while the lower part (14-50 cm) was dominated by benthic non-epiphytic (40-73%) taxa. Moreover, there was almost a complete absence of planktonic taxa throughout this core (Figure 2; Figure S3).
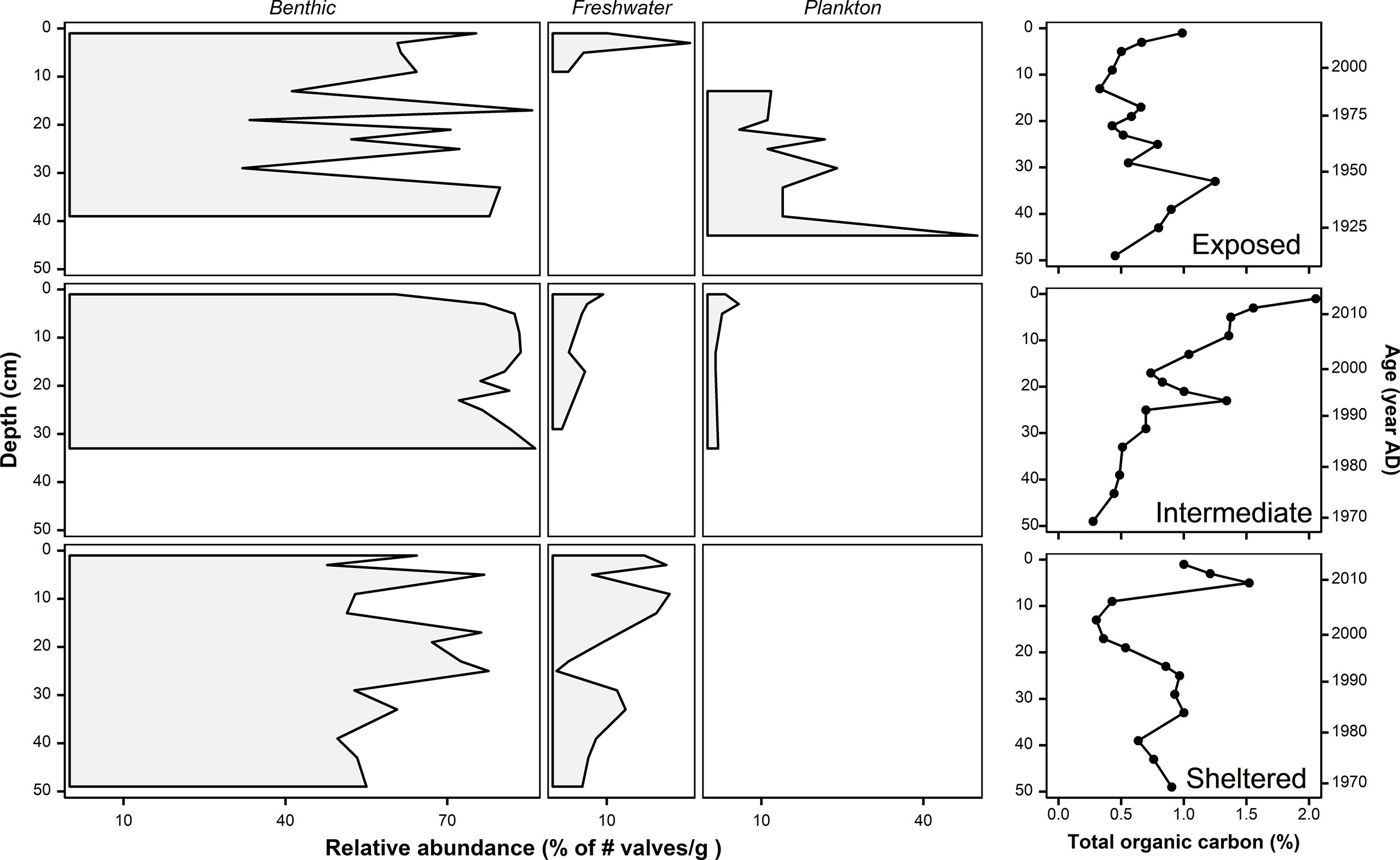
Figure 2 A stratigraphic diagram showing the relative abundance (%) of diatom guilds (benthic, freshwater and plankton) as well total organic carbon (%). Samples were analyzed in three cores, 50 cm-long each, at three sites representing different hydrodynamic regime sites: Exposed (top panel), Intermediate (middle panel) and Sheltered (bottom panel). Age estimates (right y-axis) are based on sediment accumulation rates obtained from 14C ages of shells retrieved from nearby cores (Proske et al., 2008).
Total organic carbon content (%) of the sediment was significantly correlated to total diatom relative abundance (# valves/g) (R2 = 0.19, F1,42 = 10.1, p = 0.002), as well as the relative abundance of benthic non-epiphytic group (R2 = 0.1, F1,42 = 4.8, p = 0.034), but not the other guilds (Figure 2).
Sediment carbon isotopes (δ13C) values significantly differed between the three sites (F2,41 = 48.9, p< 0.001, Figure S4). A post hoc Tukey test showed that the δ13C values of the wave-sheltered site (M = -10.5, SE = 0.15) was significantly higher than in the intermediate (M = -11.8, SE = 0.1) and wave-exposed site (M = -12.38, SE = 0.13), indicating a dominance of benthic sources at the sheltered site and more mixed sources at the exposed and intermediate sites (Figure 3). Sediment C/N ratio also significantly differed between the three sites (F2,41 = 4.7, p = 0.01, Figure S4). A post hoc Tukey test showed that the C/N ratio of the wave-sheltered site (M = 10.3, SE = 0.5) was significantly higher than in the intermediate (M = 8.9, SE = 0.2) but not different from the ratio of the wave-exposed site (M = 9.6, SE = 0.15), indicating a dominance of seagrass sources at the sheltered site, a microalgae sources at the intermediate site and mixed sources at the exposed site (Figure 3).
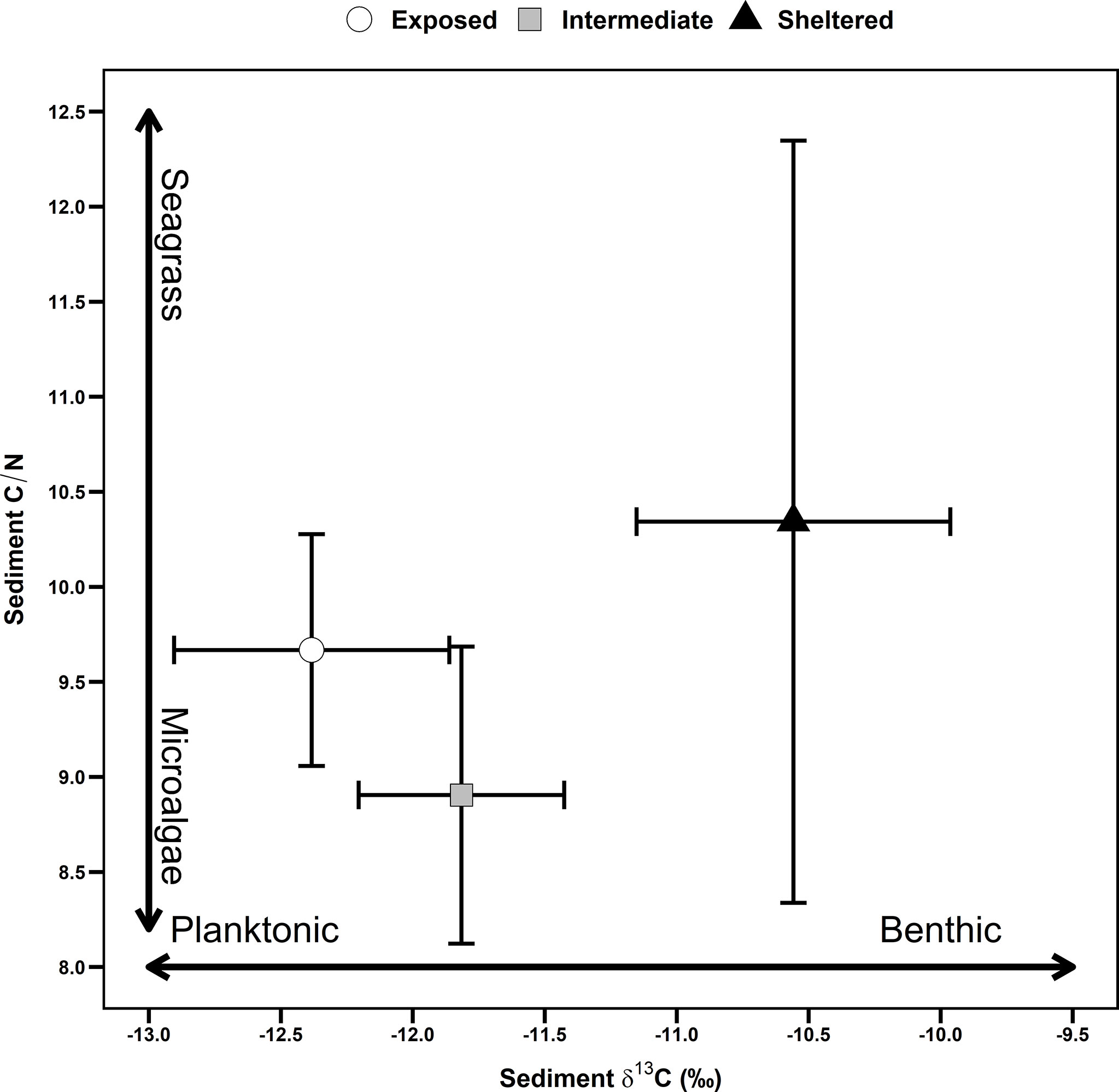
Figure 3 Spatial variation in C:N ratio and carbon stable isotope (δ13C) values of the sediment sampled in sheltered (triangle), intermediate (square) and exposed (circle) intertidal flats in Banc d’Arguin, Mauritania. Ratios are calculated per subsample (n = 25 per site) and the mean ± standard deviation is estimated per core.
The final structural equation model (SEM) yielded a good fit to the data (Fisher’s C statistic = 6.1, p = 0.64, Table S2), explaining 57% of the variance in sediment TOC (Figure 4). Both diatom relative abundance and species composition (RDA1) had significant and positive effect on sediment TOC (Figure 4). The effect of sediment depth on TOC was more complex, including direct and indirect associations: a significant direct negative effect was observed, while we also found a significant indirect negative effect mediated through abundance, plus a significant indirect positive effect mediated through RDA1 (Figure 4). Similarly, δ13C had both positive (via abundance) and negative (via RDA1) indirect significant effects on TOC (Figure 4), and a direct marginally significant positive effect on it. No direct link was identified between diatom species richness, RDA2 and sediment TOC (Figure 4). Overall, the SEM implies that variation in the stored TOC is best explained by the total abundance of diatoms and guild composition.
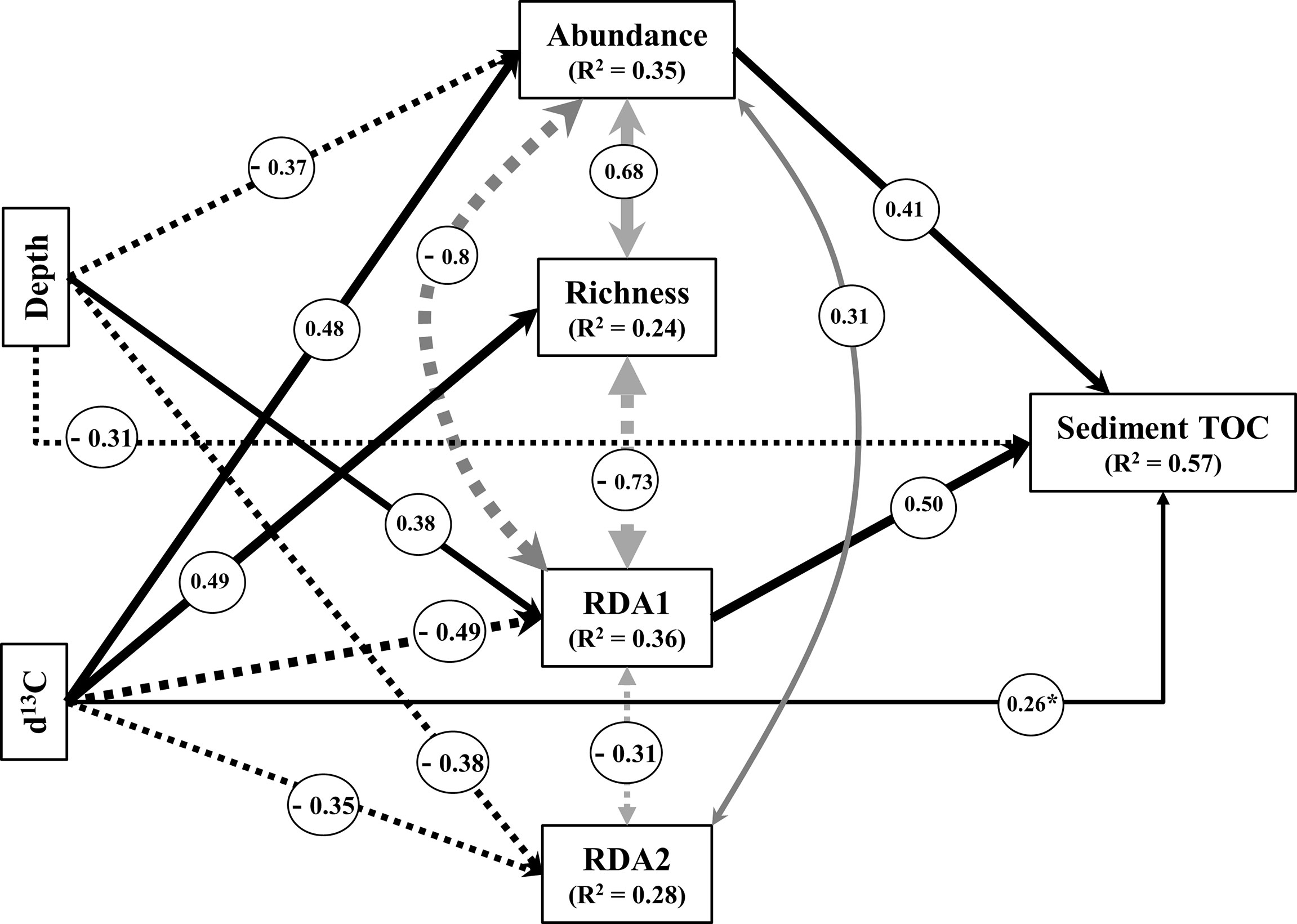
Figure 4 Most parsimonious structural equation model (Fisher’s C statistic = 6.1, p = 0.64), depicting the causal link between sediment organic carbon, depth and diatom community structure and composition. Black arrows represent unidirectional relationships among variables and grey arrows non-causal correlations. Solid and dashed arrows denote positive and negative relationships, respectively. The thickness of the paths is scaled based on the magnitude of path coefficient (β). The R2 value indicates the amount of variance explained by the model. The final model included only significant and almost significant R2 values (0.05 > p< 0.1; indicated by * pathways). RDA = redundancy analysis.
Discussion
Diatom guild and carbon stocks
In this study, we found that the exposed site was dominated by autochthonous benthic diatoms (locally produced) followed by allochthonous planktonic taxa (seaward origin), while the sheltered site was dominated by autochthonous benthic taxa followed by allochthonous freshwater ones (landward origin). The intermediate site was generally poor in abundance and dominated by benthic taxa with compete absence of diatom valves in the lower part of the core. Further, we found that the relative abundance and guild types of preserved diatoms statistically explained a large part of the variation in the amount of carbon in the intertidal sediments at Banc d’Arguin (Figure 4). Diatom valves from different guilds seem to generally preserve well in the sediment, which confirms that fossilized diatoms are informative on the source of stored C (Reich et al., 2015). In addition, the combination of δ13C and C/N geo-markers showed that the C at the wave-sheltered site was dominated by seagrass signatures, while at the exposed and intermediate-sites the planktonic signatures prevail (Figure 3). The average δ13C and C/N values of the exposed and intermediate sites fall within the ranges reported for planktonic/microphytobenthos sources, while the values of the sheltered site fall within seagrass sources (Meyers, 1994; Machás et al., 2006; Kim et al., 2014; Tuntiprapas et al., 2019). Marine algae usually have lower C/N (<10) ratios compared to seagrass (Meyers, 1994; Tuntiprapas et al., 2019). Similarly, the sediment δ13C values of the sheltered site are similar to the ones generally reported for Zostera sp. elsewhere (Machás et al., 2006; Kim et al., 2014). Indeed, the observed δ13C signatures in this site are similar to the signatures of the shoots of Z. noltei in our study area (El-Hacen et al., 2019).
An interesting observation that is hard to explain is the shift of dominance over the last century from planktonic to benthic taxa at the exposed site. This could be the result of an increase in seagrass cover as has been documented over the last three decades, particularly in the northern most exposed area in Banc d’Arguin (El-Hacen et al., 2020). Another unexpected observation is the absence of diatom valves in the lowest part of the intermediate core, which coincides with a strong decrease in stored organic matter (Figure 2). It could be that the lowest part of the core consists of a large sand deposition from the nearby sandy island, Nair (Figure 1). Occasional extreme storm events have been shown to cause strong morphological changes in the intertidal systems of Banc d’Arguin (Trégarot et al., 2021).
The main challenge that we faced in using fossilized diatoms in tracking sources of C was the lack of detailed knowledge on species composition and characteristics of the different sources. In our study, diatoms were identified mostly at the genus level, and thus species that belonged to the same genus were pulled together although they might have originated from completely different sources. This might be true for many species of Nitzschia and Synedra that were considered in our study as marine benthic although many of them are known to be planktonic and dust-freshwater species (Romero et al., 1999; Breuning-Madsen and Awadzi, 2005). A best practice would have been to identify in advance the diatom species of the different sources (benthic, pelagic, and dust) contributing to the C pool of our study site.
Origin of the diatom species
Overall, freshwater species were more abundant at the sheltered site. In both the exposed and intermediate sites, freshwater diatoms appeared only in few occasions after 1976 (Figure 2), which is the peak year of the Sahel great drought that was characterized by intense dust storms (Goudie and Middleton, 1992). It is surprising though that a previous diatom survey conducted in Banc d’Arguin in 1988, including sheltered flats, did not encounter freshwater species (Sterrenburg and Sterrenburg, 1990). Nonetheless, a study on fluxes of airborne freshwater diatoms at Cap Blanc (close proximity to the northern border of Banc d’Arguin), showed also a strong peak in 1988 (Romero et al., 2003). Although, Saharan largest dust source, the Bodélé depression in Chad, is known to consist largely of freshwater diatoms particularly Aulacoseira, Fragilaria and Stephanodiscus species (Gasse, 2002), only Fragilaria were present in our cores. However, the input of diatoms via Saharan dust seems to vary a lot spatially and temporally (Romero et al., 1999). For instant, it has been shown before that the Saharan wind-transported diatoms deposited in the equatorial Atlantic are dominated by Melosira and Stephanodiscus species, accounting for approximately 70 and 17% of the assemblage, respectively (Pokras, 1991). Again, both genera were not present in our samples. This spatio-temporal variation in the distribution of Saharan wind-transported diatoms can be explained by the strong seasonality in storms with a maximum in summer and minimum in winter (Prospero et al., 1981; Prospero et al., 2014) as well as the weight and shape of the diatom species (Mallios et al., 2020; Barkley et al., 2021), which will determine where they deposit. In addition, the fragmentation process that happens to many diatom valves during the wind-transport events is likely to lower their recovery in the geological records (Warren et al., 2007). Hence, fully quantifying the contribution of Saharan dust to Banc d’Arguin C stocks and the functioning of its intertidal system requires conducting additional studies including dust flux measurements and identification of the wind-transported diatom species. This study, however, allowed us to gain insight into the spatial distribution as well as the historical dynamic of dust-imported C into Banc d’Arguin intertidal systems.
The most common species that made a significant contribution to the total abundance of fossilized diatoms in all cores was Paralia sulcata, a species that grows predominantly benthic and is easily lifted into plankton by strong winds and tidal mixing (Roelofs, 1984; Zong, 1997; McQuoid and Nordberg, 2003). This species has been suggested to adopt a dual benthic-planktonic lifestyle: settles benthic in the growing season (spring) and suspends planktonic in autumn due to the increase of storms (Gebühr, 2011). Although, not considered an upwelling species (Romero et al., 2021), P. sulcata has been associated with nutrient rich waters (Gebühr, 2011). The association of P. sulcata to seagrass is, to our knowledge, not known and worth further investigation. Further, the rarity of both upwelling and pelagic planktonic species in our cores supports earlier findings showing low allochthonous planktonic biomass in the shallow part of Banc d’Arguin ecosystem (Sevrin-Reyssac, 1977; Sevrin-Reyssac, 1982; Sevrin-Reyssac, 1984). Indeed, the fact that planktonic species were only present in the core of the wave-exposed site is in agreement with the notion that the upwelling of Cap Blanc has only an effect on the northern most exposed area of Banc d’Arguin (Carlier et al., 2015). Thus, consistent with the suggestion by (Wolff et al., 1993), we conclude that the carbon stored in the intertidal system of Banc d’Arguin is dominated by autochthonous benthic products such as seagrass and microphytobenthos; a conclusion that was made before but lacked empirical evidences (Wolff et al., 1993).
Conclusions
The combined use of preserved diatoms in sediment and geochemical analyses allowed us backtrack the main sources of carbon in Banc d’Arguin intertidal system over time. Diatom taxa were divided into three guilds (benthic, freshwater, and pelagic) representing different sources of C: autochthonous benthic taxa and allochthonous freshwater and pelagic taxa. Autochthonous benthic diatom taxa were the most diverse and abundant group, while typical allochthonous freshwater and upwelling taxa ranked last in both abundance and species richness. Carbon stored in the sediment (% TOC) was significantly correlated to the total diatom abundance as species composition (guilds). Further, the δ13C and C/N ratios, showed a dominance of autochthonous benthic sources at the sheltered site and mixed sources at the wave-exposed site. We conclude that the C supporting the food webs and stored in the inner intertidal flats of Banc d’Arguin are predominantly autochthonous with a significant allochthonous contribution from the planktonic taxa in the wave-exposed and from freshwater taxa in the wave-sheltered zones. Thus, studies of fossilized diatoms along a sediment core will provide a useful estimate of C sources over time.
Data availability statement
The original contributions presented in the study are included in the article/Supplementary Material. Further inquiries can be directed to the corresponding author.
Author contributions
Conceptualization: TB, HO, EE, FS, and TP. Methodology: FS, TB, TM, HO, and EE. Data acquisition and analyses: EE, TM, TB, FS, and SL. Writing (original draft): EE and TM. Supervision: TP, SL, FS, and TB. Funding acquisition: HO, FS, and TP. All authors contributed to the article and approved the submitted version.
Funding
This study was funded by MAVA Foundation, Switzerland, through a PhD scholarship to EE. This work was supported by MAVA Foundation, Switzerland [grant numbers 116, 2013].
Acknowledgments
We thank the managers of the Parc National du Banc d’Arguin for granting us permission to conduct our study and to use the research vessel ‘Théodore Monod’. We extend our sincere thanks to the scientific coordinator Lemhaba Yarba, the boat captain Mohamed Salem El Hadi and the skipper Mohamed Cheddad. We are grateful to the following people for assistance in the laboratory: Peter van Breugel, Natasja Welters, Hans van Aken, Goldenberg Vilar and Keechy Akkerman.
Conflict of interest
The authors declare that the research was conducted in the absence of any commercial or financial relationships that could be construed as a potential conflict of interest.
Publisher’s note
All claims expressed in this article are solely those of the authors and do not necessarily represent those of their affiliated organizations, or those of the publisher, the editors and the reviewers. Any product that may be evaluated in this article, or claim that may be made by its manufacturer, is not guaranteed or endorsed by the publisher.
Supplementary material
The Supplementary Material for this article can be found online at: https://www.frontiersin.org/articles/10.3389/fmars.2022.1006847/full#supplementary-material
References
Barkley A. E., Olson N. E., Prospero J. M., Gatineau A., Panechou K., Maynard N. G., et al. (2021). Atmospheric transport of north African dust-bearing supermicron freshwater diatoms to south America: Implications for iron transport to the equatorial north Atlantic ocean. Geophys. Res. Lett. 48, e90476. doi: 10.1029/2020GL090476
Battarbee R. W. (1973). A new method for the estimation of absolute microfossil numbers, with reference especially to diatoms. Limnol. Oceanogr. 18, 647–653. doi: 10.4319/lo.1973.18.4.0647
Ben-Ami Y., Koren I., Rudich Y., Artaxo P., Martin S. T., Andreae M. O. (2010). Transport of north African dust from the bodélé depression to the Amazon basin: a case study. Atmos. Chem. Phys. 10, 7533–7544. doi: 10.5194/acp-10-7533-2010
Bernárdez P., Varela M., Pazos Y., Prego R., Francés G. (2010). Biocoenosis and thanatocoenosis of diatoms in a western Galician ría. J. Plankton Res. 32, 857–883. doi: 10.1093/plankt/fbq025
Bianchi T. S., Schreiner K. M., Smith R. W., Burdige D. J., Woodard S., Conley D. J. (2016). Redox effects on organic matter storage in coastal sediments during the holocene: A biomarker/proxy perspective. Annu. Rev. Earth Planet. Sci. 44, 295–319. doi: 10.1146/annurev-earth-060614-105417
Boere A. C., Rijpstra W. I. C., De Lange G. J., Sinninghe Damsté J. S., Coolen M. J. L. (2011). Preservation potential of ancient plankton DNA in pleistocene marine sediments. Geobiology 9, 377–393. doi: 10.1111/j.1472-4669.2011.00290.x
Borowitzka M. A., Lavery P. S., Van Keulen M. (2006). “Epiphytes of seagrasses,” in Seagrasses: Biology, ecology and conservation, (Springer, Dordrecht), 441–461. doi: 10.1007/978-1-4020-2983-7_19
Bos A. R., Bouma T. J., de Kort G. L. J., van Katwijk M. M. (2007). Ecosystem engineering by annual intertidal seagrass beds: sediment accretion and modification. Estuar. Coast. Shelf Sci. 74, 344–348. doi: 10.1016/j.ecss.2007.04.006
Bouma T. J., de Vries M. B., Low E., Peralta G., Tánczos I. C., van de Koppel J., et al. (2005). Trade-offs related to ecosystem engineering: a case study on stiffness of emerging macrophytes. Ecology 86, 2187–2199. doi: 10.1890/04-1588
Breuning-Madsen H., Awadzi T. W. (2005). Harmattan dust deposition and particle size in Ghana. Catena 63, 23–38. doi: 10.1016/j.catena.2005.04.001
Bulmer R. H., Stephenson F., Jones H. F. E., Townsend M., Hillman J. R., Schwendenmann L., et al. (2020). Blue carbon stocks and cross-habitat subsidies. Front. Mar. Sci. 7. doi: 10.3389/fmars.2020.00380
Carlier A., Chauvaud L., van der Geest M., Le Loc’h F., Le Duff M., Vernet M., et al. (2015). Trophic connectivity between offshore upwelling and the inshore food web of banc d’Arguin (Mauritania): new insights from isotopic analysis. Estuar. Coast. Shelf Sci. 165, 149–158. doi: 10.1016/j.ecss.2015.05.001
Clavier J., Chauvaud L., Amice E., Lazure P., van der Geest M., Labrosse P., et al. (2014). Benthic metabolism in shallow coastal ecosystems of the banc d’Arguin, Mauritania. Mar. Ecol. Prog. Ser. 501, 11–23. doi: 10.3354/meps10683
Duarte C. M., Losada I. J., Hendriks I. E., Mazarrasa I., Marbà N. (2013). The role of coastal plant communities for climate change mitigation and adaptation. Nat. Clim. Change 3, 961–968. doi: 10.1038/nclimate1970
Duarte C. M., Marbà N., Gacia E., Fourqurean J. W., Beggins J., Barrón C., et al. (2010). Seagrass community metabolism: assessing the carbon sink capacity of seagrass meadows. Global Biogeochem. Cycles 24, GB4032. doi: 10.1029/2010GB003793
El-Hacen E. M., Bouma T. J., Govers L. L., Piersma T., Olff H. (2019). Seagrass sensitivity to collapse along a hydrodynamic gradient: evidence from a pristine subtropical intertidal ecosystem. Ecosystems 22, 1007–1023. doi: 10.1007/s10021-018-0319-0
El-Hacen E. M., Sidi Cheikh M. A., Bouma T. J., Olff H., Piersma T. (2020). Long-term changes in seagrass and benthos at banc d’Arguin, Mauritania, the premier intertidal system along the East Atlantic flyway. Glob. Ecol. Conserv. 24, e01364. doi: 10.1016/j.gecco.2020.e01364
Forsey G. F. (2016). Ostracods as proxies for past seagrass: A review. Palaeogeogr. Palaeoclimatol. Palaeoecol. 447, 22–28. doi: 10.1016/j.palaeo.2016.01.028
Fourqurean J. W., Duarte C. M., Kennedy H., Marbà N., Holmer M., Mateo M. A., et al. (2012). Seagrass ecosystems as a globally significant carbon stock. Nat. Geosci. 5, 505–509. doi: 10.1038/ngeo1477
France R. (1995). Carbon-13 enrichment in benthic compared to planktonic algae: foodweb implications. Mar. Ecol. Prog. Ser. 124, 307–312. doi: 10.3354/meps124307
Garcia A. M., Oliveira M. C. L. M., Odebrecht C., Colling J. L. A., Vieira J. P., Rodrigues F. L., et al. (2019). Allochthonous versus autochthonous organic matter sustaining macroconsumers in a subtropical sandy beach revealed by stable isotopes. Mar. Biol. Res. 15, 241–258. doi: 10.1080/17451000.2019.1627559
Gasse F. (2002). Diatom-inferred salinity and carbonate oxygen isotopes in Holocene waterbodies of the western Sahara and sahel (Africa). Quat. Sci. Rev. 21, 737–767. doi: 10.1016/S0277-3791(01)00125-1
Gebühr C. (2011) Investigations on the ecology of the marine centric diatom paralia sulcata at helgoland roads, north Sea, Germany. Available at: https://epic.awi.de/id/eprint/23721/1/Geb2010f.pdf.
Geraldi N. R., Ortega A., Serrano O., Macreadie P. I., Lovelock C. E., Krause-Jensen D., et al. (2019). Fingerprinting blue carbon: Rationale and tools to determine the source of organic carbon in marine depositional environments. Front. Mar. Sci. 6. doi: 10.3389/fmars.2019.00263
Goudie A. S., Middleton N. J. (1992). The changing frequency of dust storms through time. Clim. Change 20, 197–225. doi: 10.1007/BF00139839
Grace J. B., Anderson T. M., Olff H., Scheiner S. M. (2010). On the specification of structural equation models for ecological systems. Ecol. Monogr. 80, 67–87. doi: 10.2307/27806874
Guillaume Blanchet F., Legendre P., Colin Bergeron J. A., He F. (2014). Consensus RDA across dissimilarity coefficients for canonical ordination of community composition data. Ecol. Monogr. 84, 491–511. doi: 10.1890/13-0648.1
Halse G. R., Syvertsen E. E. (1996). “Marine diatoms,” in Identifying marine diatoms and dinoflagellates (London, Elsevier), 5–385. doi: 10.1016/b978-012693015-3/50005-x
Hayes M. A., Jesse A., Hawke B., Baldock J., Tabet B., Lockington D., et al. (2017). Dynamics of sediment carbon stocks across intertidal wetland habitats of moreton bay, Australia. Glob. Change Biol. 23, 4222–4234. doi: 10.1111/gcb.13722
Kennedy H., Beggins J., Duarte C. M., Fourqurean J. W., Holmer M., Marbà N., et al. (2010). Seagrass sediments as a global carbon sink: Isotopic constraints. Global Biogeochem. Cycles 24, GB4026. doi: 10.1029/2010GB003848
Killick R., Eckley I. A. (2014). Changepoint: An r package for changepoint analysis. J. Stat. Software 58, 1–19. doi: 10.18637/jss.v058.i03
Kim M. S., Lee S. M., Kim H. J., Lee S. Y., Yoon S. H., Shin K. H. (2014). Carbon stable isotope ratios of new leaves of Zostera marina in the mid-latitude region: Implications of seasonal variation in productivity. J. Exp. Mar. Bio. Ecol. 461, 286–296. doi: 10.1016/j.jembe.2014.08.015
Kleyer M., Dray S., Bello F., Lepš J., Pakeman R. J., Strauss B., et al. (2012). Assessing species and community functional responses to environmental gradients: Which multivariate methods? J. Veg. Sci. 23, 805–821. doi: 10.1111/j.1654-1103.2012.01402.x
Lamb A. L., Wilson G. P., Leng M. J. (2006). A review of coastal palaeoclimate and relative sea-level reconstructions using δ13C and C/N ratios in organic material. Earth-Science Rev. 75, 29–57. doi: 10.1016/j.earscirev.2005.10.003
Lebreton B., Richard P., Radenac G., Bordes M., Bréret M., Arnaud C., et al. (2009). Are epiphytes a significant component of intertidal Zostera noltii beds? Aquat. Bot. 91, 82–90. doi: 10.1016/j.aquabot.2009.03.003
Lefcheck J. S. (2016). piecewiseSEM: Piecewise structural equation modelling in r for ecology, evolution, and systematics. Methods Ecol. Evol. 7, 573–579. doi: 10.1111/2041-210X.12512
Legendre P., Oksanen J., ter Braak C. J. F. (2011). Testing the significance of canonical axes in redundancy analysis. Methods Ecol. Evol. 2, 269–277. doi: 10.1111/j.2041-210X.2010.00078.x
Lepš J., Šmilauer P. (2003). Multivariate analysis of ecological data using CANOCO. 1st ed (Cambridge, UK: Cambridge University Press).
López-Merino L., Colás-Ruiz N. R., Adame M. F., Serrano O., Martínez Cortizas A., Mateo M. A. (2017). A six thousand-year record of climate and land-use change from Mediterranean seagrass mats. J. Ecol. 105, 1267–1278. doi: 10.1111/1365-2745.12741
Machás R., Santos R., Peterson B. (2006). Elemental and stable isotope composition of zostera noltii (Horneman) leaves during the early phases of decay in a temperate mesotidal lagoon. Estuar. Coast. Shelf Sci. 66, 21–29. doi: 10.1016/j.ecss.2005.07.018
MacReadie P. I., Anton A., Raven J. A., Beaumont N., Connolly R. M., Friess D. A., et al. (2019). The future of blue carbon science. Nat. Commun. 10, 1–13. doi: 10.1038/s41467-019-11693-w
Mallios S. A., Drakaki E., Amiridis V. (2020). Effects of dust particle sphericity and orientation on their gravitational settling in the earth’s atmosphere. J. Aerosol Sci. 150, 105634. doi: 10.1016/j.jaerosci.2020.105634
Margalef R. (1975). Phytoplankton communities in upwelling areas. the example of NW Africa. Oecologia Aquat. 3, 97–132.
McQuoid M. R., Nordberg K. (2003). The diatom Paralia sulcata as an environmental indicator species in coastal sediments. Estuar. Coast. Shelf Sci. 56, 339–354. doi: 10.1016/S0272-7714(02)00187-7
Meyers P. A. (1994). Preservation of elemental and isotopic source identification of sedimentary organic matter. Chem. Geol. 114, 289–302. doi: 10.1016/0009-2541(94)90059-0
Nellemann C., Corcoran E., Duarte C. M., Valdés L., De Young C., Fonseca L., et al. (2009). Blue carbon: A rapid response assessment (Birkeland, Norway, United Nations Environment Programme, GRID-Arendal). Available at: www.grida.no.
Ortega A., Geraldi N. R., Duarte C. M. (2020). Environmental DNA identifies marine macrophyte contributions to blue carbon sediments. Limnol. Oceanogr. 65, 3139–3149. doi: 10.1002/lno.11579
Pergent G., Romero J., Pergentmartini C., Mateo M. A., Boudouresque C. F. (1994). Primary production, stocks and fluxes in the Mediterranean seagrass Posidonia oceanica. Mar. Ecol. Prog. Ser. 106, 139–146. doi: 10.3354/meps106139
Phang V. X. H., Chou L. M., Friess D. A. (2015). Ecosystem carbon stocks across a tropical intertidal habitat mosaic of mangrove forest, seagrass meadow, mudflat and sandbar. Earth Surf. Process. Landforms 40, 1387–1400. doi: 10.1002/esp.3745
Phillips D. L., Gregg J. W. (2003). Source partitioning using stable isotopes: Coping with too many sources. Oecologia 136, 261–269. doi: 10.1007/s00442-003-1218-3
Pokras E. M. (1991). Source areas and transport mechanisms for freshwater and brackish-water diatoms deposited in pelagic sediments of the equatorial Atlantic. Quat. Res. 35, 144–156. doi: 10.1016/0033-5894(91)90101-A
Polis G. A., Anderson W. B., Holt R. D. (1997). Toward an integration of landscape and food web ecology: the dynamics of spatially subsidized food webs. Annu. Rev. Ecol. Syst. 28, 289–316. doi: 10.1146/annurev.ecolsys.28.1.289
Proske U., Hanebuth T. J. J., Meggers H., Leroy S. A. G. (2008). Tidal flat sedimentation during the last millennium in the northern area of tidra island, banc d’Arguin, Mauritania. J. Afr. Earth Sci. 50, 37–48. doi: 10.1016/j.jafrearsci.2007.09.002
Prospero J. M., Collard F.-X., Molinié J., Jeannot A. (2014). Characterizing the annual cycle of African dust transport to the Caribbean basin and south America and its impact on the environment and air quality. Global Biogeochem. Cycles 28, 757–773. doi: 10.1002/2013GB004802
Prospero J. M., Glaccum R. A., Nees R. T. (1981). Atmospheric transport of soil dust from Africa to south America. Nature 289, 570–572. doi: 10.1038/289570a0
Reef R., Atwood T. B., Samper-Villarreal J., Adame M. F., Sampayo E. M., Lovelock C. E. (2017). Using eDNA to determine the source of organic carbon in seagrass meadows. Limnol. Oceanogr. 62, 1254–1265. doi: 10.1002/lno.10499
Reich S., Di Martino E., Todd J. A., Wesselingh F. P., Renema W. (2015). Indirect paleo-seagrass indicators (IPSIs): A review. Earth-Science Rev. 143, 161–186. doi: 10.1016/j.earscirev.2015.01.009
Roelofs A. K. (1984). Distributional patterns and variation of valve diameter of Paralia sulcata in surface sediments of southern British Columbia inlets. Estuar. Coast. Shelf Sci. 18, 165–176. doi: 10.1016/0272-7714(84)90104-5
Romero O. E., Baumann K. H., Zonneveld K. A. F., Donner B., Hefter J., Hamady B., et al. (2020). Flux variability of phyto- and zooplankton communities in the Mauritanian coastal upwelling between 2003 and 2008. Biogeosciences 17, 187–214. doi: 10.5194/bg-17-187-2020
Romero O. E., Dupont L. M., Wyputta U., Jahns S., Wefer G. (2003). Temporal variability of fluxes of eolian-transported freshwater diatoms, phytoliths, and pollen grains off cape blanc as reflection of land-atmosphere-ocean interactions in northwest Africa. J. Geophys. Res. 108, 1-12. doi: 10.1029/2000jc000375
Romero O. E., Fischer G. (2017). Shift in the species composition of the diatom community in the eutrophic Mauritanian coastal upwelling: Results from a multi-year sediment trap experiment, (2003–2010). Prog. Oceanogr. 159, 31–44. doi: 10.1016/j.pocean.2017.09.010
Romero O. E., Lange C. B., Swap R., Wefer G. (1999). Eolian-transported freshwater diatoms and phytoliths across the equatorial Atlantic: Providing records of temporal changes in Saharan dust transport patterns. J. Geophys. Res. Ocean. 104, 3211–3322. doi: 10.1029/1998jc900070
Romero O. E., Ramondenc S., Fischer G. (2021). A 2-decade, (1988-2009) record of diatom fluxes in the Mauritanian coastal upwelling: Impact of low-frequency forcing and a two-step shift in the species composition. Biogeosciences 18, 1873–1891. doi: 10.5194/bg-18-1873-2021
Schrader H., Gersonde R. (1978). “Diatoms and silicoflagellates,” in An excercise on an eight metres section of the lower pliocene of capo rossello. Eds. Zachariasse W. J., Riedel W. R., Sanfilippo A., Schmidt R. R., Brolsma M. J., Schrader H. J., et al (Utrecht, Netherlands: University of Utrecht), 129–176.
Sevrin-Reyssac J. (1977). Hydrologie, phytoplancton et production primaire de la baie du lévrier et du banc d’Arguin. Bull. L’IFAN 39, 486–554.
Sevrin-Reyssac J. (1982). Caractéristiques du phytoplancton de la région de l’île tidra (Mauritanie) en septembre et octobre 1980. Bull. du CNROP 10, 41–47.
Sevrin-Reyssac J. (1984). Conditions hydrologiques et phytoplancton dans la partie méridionale du parc national du banc d’Arguin (Mauritanie) en mars et avril 1983. Bull. du CNROP 12, 107–113.
Sgro G. V., Reavie E. D. (2018). Fossil diatoms, geochemistry, and the anthropocene paleolimnology of lake Huron. J. Great Lakes Res. 44, 765–778. doi: 10.1016/J.JGLR.2018.05.015
Shipley B. (2013). The AIC model selection method applied to path analytic models compared using a d-separation test. Ecology 94, 560–564. doi: 10.1890/12-0976.1
Šmilauer P., Lepš J. (2014). Multivariate analysis of ecological data using canoco. (Cambridge University Press, Cambridge, U.K) 5, 373. doi: 10.1017/CBO9781139627061
Sterrenburg F. A. S., Sterrenburg F. J. G. (1990). An outline of the marine littoral diatom biocoenosis of the banc d’Arguin, Mauritania, West Africa. Bot. Mar. 33, 459–465. doi: 10.1515/botm.1990.33.5.459
Sun Y., Song Z., Zhang H., Liu P., Hu X. (2020). Seagrass vegetation affects the vertical organization of microbial communities in sediment. Mar. Environ. Res. 162, 105174. doi: 10.1016/j.marenvres.2020.105174
Touchette B. W., Burkholder J. A. M. (2000). Overview of the physiological ecology of carbon metabolism in seagrasses. J. Exp. Mar. Bio. Ecol. 250, 169–205. doi: 10.1016/S0022-0981(00)00196-9
Trégarot E., Catry T., Pottier A., El-Hacen E. M., Sidi Cheikh M. A., Cornet C. C., et al. (2021). Coastal protection assessment: a tradeoff between ecological, social, and economic issues. Ecosphere 12, e03364. doi: 10.1002/ecs2.3364
Tuntiprapas P., Hayashizaki K., Ogawa H., Panyawai J., Tamada S., Stankovic M., et al. (2019). The contributions of allochthonous and autochthonous materials to organic carbon in coastal sediment: A case study from tangkhen bay, phuket, Thailand. Ecol. Res. 34, 718–729. doi: 10.1111/1440-1703.12040
Tuya F., Betancort J. F., Haroun R., Espino F., Lomoschitz A., Meco J. (2017). Seagrass paleo-biogeography: Fossil records reveal the presence of Halodule cf. in the canary islands (eastern Atlantic). Aquat. Bot. 143, 1–7. doi: 10.1016/j.aquabot.2017.08.002
UNESCO (2020). UNESCO Marine world heritage: Custodians of the globe’s blue carbon assets (Paris, France, The United Nations Educational,Scientific and Cultural Organization). Available at: https://unesdoc.unesco.org/ark:/48223/pf0000375565.
van Camp L., Nykjaer L., Mittelstaedt E., Schlittenhardt P. (1991). Upwelling and boundary circulation off Northwest Africa as depicted by infrared and visible satellite observations. Prog. Oceanogr. 26, 357–402. doi: 10.1016/0079-6611(91)90012-B
Vermaat J. E., Verhagen F. C. A. (1996). Seasonal variation in the intertidal seagrass Zostera noltii hornem.: coupling demographic and physiological patterns. Aquat. Bot. 52, 259–281. doi: 10.1016/0304-3770(95)00510-2
Vos P. C., de Wolf H. (1993). Diatoms as a tool for reconstructing sedimentary environments in coastal wetlands; methodological aspects. Hydrobiologia 269, 285–296. doi: 10.1007/BF00028027
Warren A., Chappell A., Todd M. C., Bristow C., Drake N., Engelstaedter S., et al. (2007). Dust-raising in the dustiest place on earth. Geomorphology 92, 25–37. doi: 10.1016/j.geomorph.2007.02.007
Waterson E. J., Canuel E. A. (2008). Sources of sedimentary organic matter in the Mississippi river and adjacent gulf of Mexico as revealed by lipid biomarker and δ13CTOC analyses. Org. Geochem. 39, 422–439. doi: 10.1016/j.orggeochem.2008.01.011
Wefer G., Fischer G. (1993). Seasonal patterns of vertical particle flux in equatorial and coastal upwelling areas of the eastern Atlantic. Deep. Res. Part I 40, 1613–1645. doi: 10.1016/0967-0637(93)90019-Y
Widenfalk L. A., Bengtsson J., Berggren Å., Zwiggelaar K., Spijkman E., Huyer-Brugman F., et al. (2015). Spatially structured environmental filtering of collembolan traits in late successional salt marsh vegetation. Oecologia 179, 537. doi: 10.1007/S00442-015-3345-Z
Witkowski A., Lange-Bertalot H., Metzeltin D. (2000). Diatom flora of marine coasts. Ed. Lange-Bertalot H. (Königstein, Germany, A.R.G. Gantner Verlag).
Wolff W. J., van der Land J., Nienhuis P. H., de Wilde P. A. W. J. (1993). The functioning of the ecosystem of the banc d’Arguin, Mauritania: a review. Hydrobiologia 258, 211–222. doi: 10.1007/BF00006198
Keywords: Banc d’Arguin-Mauritania, carbon sequestration, global warming, carbon flux, fossils, seagrass (Zostera)
Citation: El-Hacen E-HM, Mens T, Bouma TJ, Piersma T, Leroy SAG, Olff H and Sangiorgi F (2022) Fossilized diatoms as indirect indicators of the origin of carbon stored in intertidal flats. Front. Mar. Sci. 9:1006847. doi: 10.3389/fmars.2022.1006847
Received: 29 July 2022; Accepted: 11 October 2022;
Published: 01 November 2022.
Edited by:
Angel Borja, Technology Center Expert in Marine and Food Innovation (AZTI), SpainReviewed by:
Vona Meleder, Université de Nantes, FranceSophie Westacott, Yale University, United States
Copyright © 2022 El-Hacen, Mens, Bouma, Piersma, Leroy, Olff and Sangiorgi. This is an open-access article distributed under the terms of the Creative Commons Attribution License (CC BY). The use, distribution or reproduction in other forums is permitted, provided the original author(s) and the copyright owner(s) are credited and that the original publication in this journal is cited, in accordance with accepted academic practice. No use, distribution or reproduction is permitted which does not comply with these terms.
*Correspondence: El-Hacen M. El-Hacen, ZS5oLm0uZWwuaGFjZW5AcnVnLm5s