- 1Laboratorio de Ecología de Sistemas Pelágicos, Departamento de Ecología y Biodiversidad Acuática, Instituto de Ciencias del Mar y Limnología, Universidad Nacional Autónoma de México, Ciudad de México, Mexico
- 2Laboratorio de Oceanografía Física, Departamento de Procesos Oceánicos y Costeros, Instituto de Ciencias del Mar y Limnología, Universidad Nacional Autónoma de México, Ciudad de México, Mexico
- 3Laboratorio de Ecología Marina, Departamento de Sistemas y Procesos Naturales, Escuela Nacional de Estudios Superiores Unidad Mérida, Universidad Nacional Autónoma de México, Mérida, Mexico
Functional diversity measures help to understand the underlying mechanisms explaining the relationship between organisms and environment. This work examined the mesozooplankton community of an estuary under the hypothesis that the upper (< 25 psu) and lower (> 25 psu) zones are functionally different in terms of feeding strategies, trophic groups, camouflage, buoyancy, and defense against predators, due to the salinity gradient. We used the ‘community-weighted mean trait values’ (CWM) and a fuzzy correspondence analysis (FCA) in combination with three functional indices (FRic: functional richness, FEve: functional evenness, FDiv: functional divergence) to test our hypothesis. Zooplankton samples were taken in the tropical Sontecomapan estuary, southern Gulf of Mexico, in June 2018 and October 2019. A total of 21 zooplankton groups were recognized, all of them present in the lower estuary. Results showed significant differences (p< 0.05) in the FRic and FDiv values between the two zones, as well in the CWM metric. Results of the FCA showed that the lower estuary was characterized by herbivorous filter feeders that use watery bodies, flattened body forms, mucus houses, or ‘wings’ for buoyancy; highly transparent bodies (associated with gelatinous body structure) for camouflage and predators’ avoidance and, shells or carapaces as antipredator tactics. The upper estuary was characterized by omnivorous cruising predators that use their appendages or swim bladders for buoyancy, with ‘medium’ and ‘low’ levels of transparency, associated with chitinous and skin-protected body structures, which provide alternative ways for camouflage and spines or fast swimming behavior to protect from predators. These findings evidenced that the upper and lower zones of an estuary exhibit differences in the function of the zooplankton community and shed new light on the comprehension of estuaries’ ecological function.
Introduction
Estuaries are partially enclosed coastal systems in which the unique combination of marine and fluvial waters leads to a variety of habitats. The mixture of salty and fresh waters offers high levels of nutrients, placing estuaries among the most productive water bodies on the planet (McLusky and Elliott, 2004). Primary productivity in estuaries is mainly due to the plants bordering the systems, rather than photosynthetic processes in the water itself. At times, phytoplankton cell concentration is relatively low, but higher compared to that in the open sea (Dobson and Frid, 1998).
Estuaries display highly variable hydrographic properties due to their own dynamic nature, in which the tides, fluvial discharges, and winds combine to drive the spatial pattern of salinity, temperature, turbidity, and other hydrological parameters affecting the zooplankton distribution (Benfield, 2013). Usually, salinity is the key factor determining the spatial distribution of estuarine zooplankton and organisms have a range of salinities in which they can survive (Benfield, 2013; Vinagre and Costa, 2014). Based on the salinity intrusion, one can distinguish a lower estuarine zone characterized by a free connection with the open sea, and an upper estuarine zone with an important amount of freshwater, with an intermediate mixing zone (Nayak and Noronha-D’Mello, 2018). Most biota in estuaries includes marine organisms that can tolerate brackish waters to a certain extent, thus regulating the structure of communities, the species interactions, and some other communities’ attributes such as trophic structure (Sanvicente-Añorve et al., 2002; Mateus et al., 2008; Vinagre and Costa, 2014) and functional traits.
Functional traits refer to the morphological, physiological, behavioral, or phenological characteristics that control the response of organisms to environmental features and their effects on ecosystem properties (Violle et al., 2007; Díaz et al., 2013). Therefore, functional diversity is the variety and distribution of functional traits in a community (Lavorel et al., 2008). Species having similar functional traits can be grouped into functional groups, that is, organisms with a similar response to environmental conditions and/or similar effect on ecosystem function (Benedetti et al., 2016); key functional traits are those few traits that well describe its fitness and can be classified regarding their type and function in the ecosystem (Litchman et al., 2013). In the pelagic environment, zooplankters exhibit high variability in form, size, motility, feeding strategies, and reproductive modes, characteristics that determine their fitness in the ecosystem (Sanvicente-Añorve et al., 2006; Saiz, 2009; Litchman et al., 2013). The analysis of functional traits in a community has been proposed as an effective tool to characterize ecosystem functioning by linking organisms to ecosystems (Pomerleau et al., 2015; Hébert et al., 2016). Furthermore, the examination of functional traits reveals ecosystem processes not evident by taxonomic analyses (Barnett et al., 2007). Hence, studies have demonstrated differences in the ecosystem function along eutrophication or trophic state gradients by analyzing the functional attributes of zooplankters (Obertegger and Manca, 2011; Goździejewska et al., 2021).
Sontecomapan is a small lagoon located on the eastern Mexican coast facing the Gulf of Mexico. This coastal estuarine system offers diverse habitats for feeding, protection, and reproduction of commercial and non-commercial invertebrates and vertebrates. Local fisheries include crabs, shrimps, oysters, snappers, and mullets, and the vegetation surrounding the lagoon provides important resting places for resident and migratory birds (Monroy-Ojeda and Isern, 2013; González-Fierro and Ponce-Vélez, 2018). The lagoon is permanently linked with the sea by a single narrow connection in its northeast section. The intrusion of marine water into the lagoon interplays with the tides and river runoffs leading to variable salinity gradients throughout the year (López-Portillo et al., 2017). Studies regarding zooplankton functional traits mainly focus on freshwater habitats and crustaceans, probably due to the high abundance and wide geographical distribution of these animals (Barnett et al., 2007; Vogt et al., 2013; Hébert and Beisner, 2021). Recently, studies in marine waters have offered an integrative approach to the function of zooplankton communities (Pomerleau et al., 2015; Li et al., 2022). In estuaries, field observations indicated that major changes in composition and diversity of zooplankters occur at 25 psu salinity level (Li et al., 2006). In this study, we examined the whole mesozooplankton community and their associated functional traits to test the hypothesis that the upper (< 25 psu) and lower (> 25 psu) zones of the Sontecomapan estuary function in a different manner due to the salinity gradient. Functional traits here examined incorporate several aspects of the zooplankton ecology allowing them to survive as plankton, and include feeding ecology, buoyancy, camouflage, and defense tactics against predators.
Materials and methods
Study area
The Sontecomapan lagoon is located in the southern Gulf of Mexico, between 18° 30.3’ – 18° 33.9’ N, and 94° 59.1’ – 95° 2.4’ W (Figure 1). Three distinct meteorological conditions can be distinguished in the region: the ‘nortes’ season, from November to February, characterized by strong cold winds from the North with intense sporadic rainfall; the dry season, from March to early June; the rainy season, from late June to October, in which the lagoon receives continuous freshwater inflow from the rivers and runoff, due to pluvial precipitation (Aké-Castillo and Vázquez, 2008; Esquivel-Herrera and Soto-Castor, 2018).
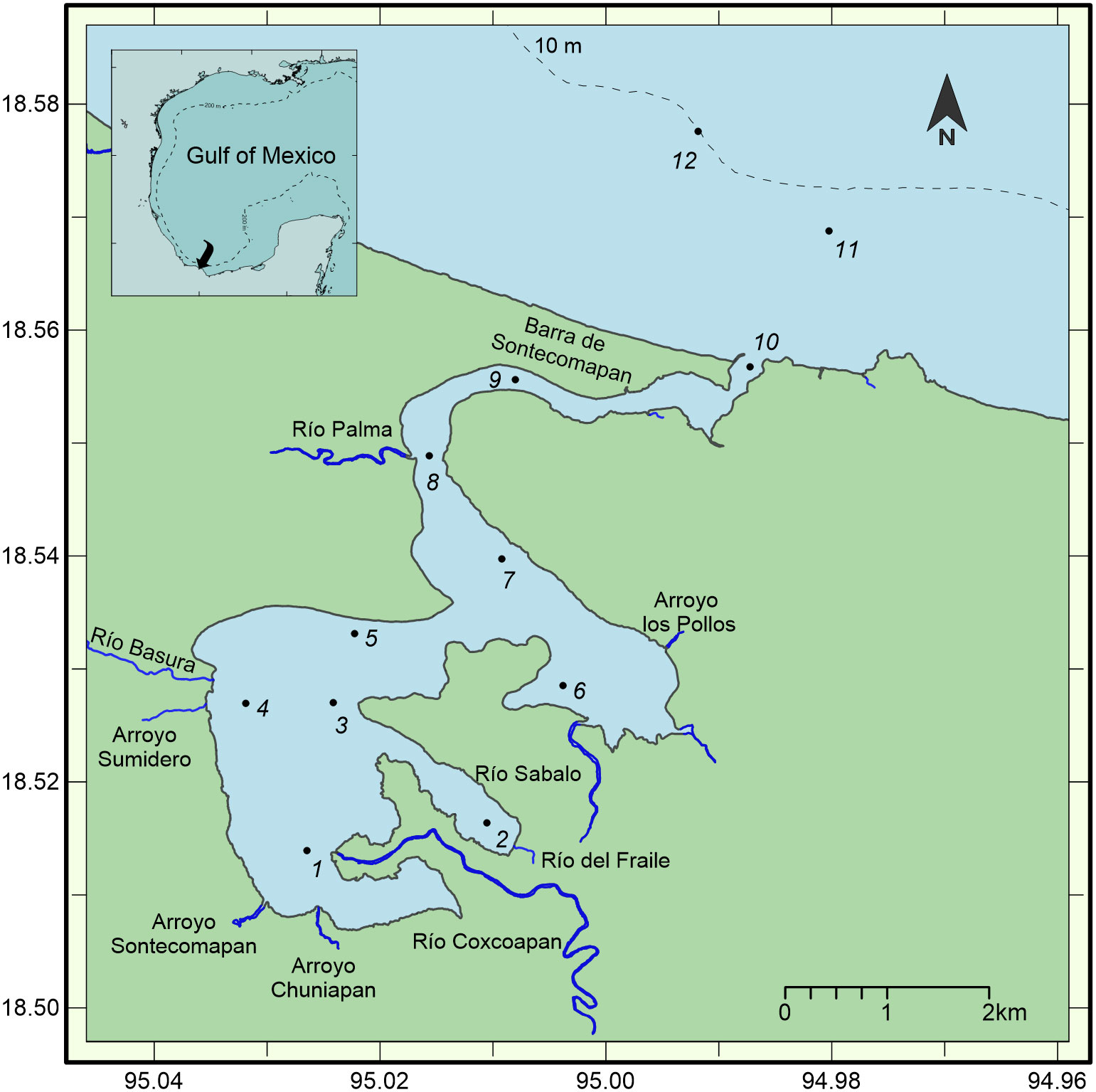
Figure 1 Study area and location of sampling stations in the Sontecomapan lagoon in June 2018 and October 2019.
The lagoon is a small shallow (1.5 m) water body separated by the sea by a single narrow entrance. It is about 7 km in length and 5 km wide and feeds from several rivers and streams, such as Palma, Basura, Chuniapan, and Coxcoapan (Figure 1). The lagoon exhibits diverse environments (quasi stagnant waters, mangrove forests, wetlands) due to its geomorphology and variable marine water intrusion throughout the year, varying from a marine condition during the dry season to a freshwater condition during the rainy season (Esquivel-Herrera and Soto-Castor, 2018).
Field and laboratory work
Zooplankton sampling was carried out in June 2018 (dry season) and October 2019 (rainy season). Sampling stations were distributed along the lagoon and in the adjacent marine zone; eleven stations were sampled in June (stations 1 to 11), and twelve in October (stations 1 to 12) (Figure 1). Zooplankton samples were obtained during the daytime in a small boat with an outboard motor using a conical net of 1.6 m in length, 50 cm in diameter and 333 µm mesh size, equipped with a mechanical flowmeter to estimate the volume of filtered water. Samples were collected from circular surface (0-60 cm) tows for five minutes at a speed of two knots. Afterward, samples were fixed in a 4% solution of formaldehyde in seawater neutralized with sodium borate. At each sampling station, the temperature and salinity of the water were measured using a YSI 85 salinometer-conductivity probe, with accuracies of ± 0.01°C in temperature and ± 0.01 in salinity.
In the laboratory, all zooplankters were sorted and classified into major groups according to specialized literature (Lalli and Gilmer, 1989; Gasca and Suárez, 1996; Boltovskoy, 1999a; Boltovskoy, 1999b; Johnson and Allen, 2012; Castellani and Edwards, 2017). Zooplankters were classed into taxonomic categories broader than the family level; however, for some groups, the dominant species were identified.
Data analysis
The functional trait composition of the zooplankton community was analyzed through the so-called ‘community-weighted mean trait values’ (CWM) metric (Garnier et al., 2004). The CWM measure is constructed using two matrices: a matrix containing data on the composition and abundance of taxa, and a matrix including the relevant taxa’ functional traits for the ecological problem of interest (Ricotta and Moretti, 2011). This measure is computed as:
where CWM is the community-weighted mean value of a given functional trait, pi is the relative abundance of taxon i, xi is the trait value for taxon i, and S is the number of taxa. The functional trait matrix included eight traits and 38 categories related to the feeding ecology, buoyancy, camouflage, antipredator tactics, and body characteristics (Table 1).
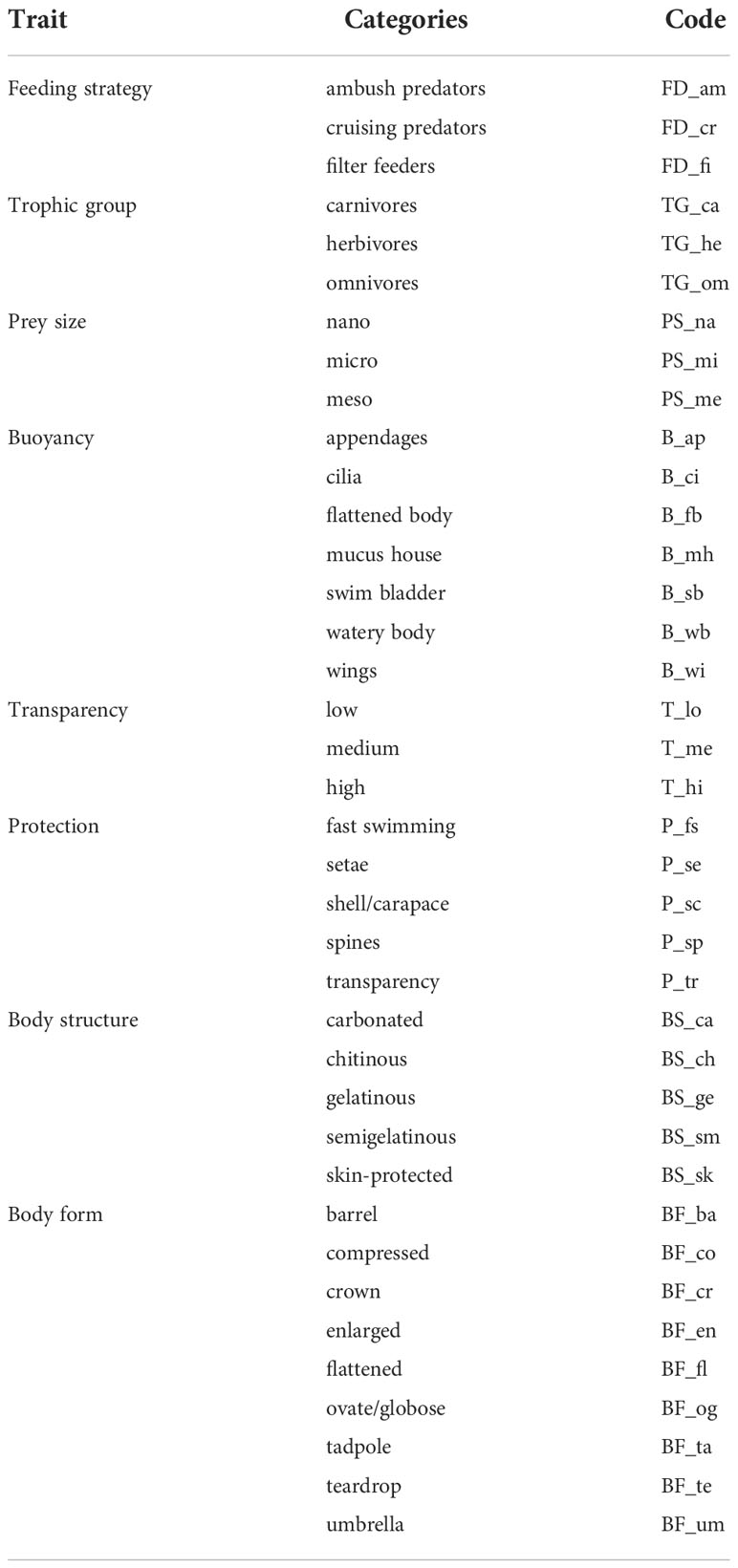
Table 1 Functional traits and associated categories used in the functional characterization of zooplankters.
Concerning feeding strategies, three basic modes were included in the functional trait matrix. The ‘filter-feeding’ strategy comprises organisms that feed by passing the water through a specialized filter structure or a sieve composed of setae or other appendages; for example, thaliaceans and cladocerans. The ‘ambush feeding’ strategy includes animals that remain motionless most of the time and rely on prey movement to encounter it, with or without remote detection; for example, pteropods, copepods, and chaetognaths. The ‘cruise feeding’ strategy contains organisms that swim actively and remotely detect their prey by hydrodynamical, chemical, and/or visual cues; for instance, fish larvae (Viitasalo et al., 1998; Kiørboe, 2011; Saiz, 2009).
Data on the abundance of the zooplankton groups across the sampling sites of the two sampling periods were transformed and treated employing the Bray-Curtis dissimilarity index; the resultant symmetric matrix was represented through a non-metric multidimensional scaling (nMDS). As well, a PERMANOVA test was also applied to the zooplankton abundance-by-stations and CWM matrices to test differences between seasons and/or the upper and lower estuaries. These analyses were performed with the PRIMER 7 software (Clarke et al., 2014).
Three primary components of functional diversity (functional richness FRic, functional evenness FEve, and functional divergence FDiv indices) were also calculated (Mason et al., 2005; Laliberté and Legendre, 2010). From these indices, only FEve and FDiv vary between 0 and 1. Functional richness can be defined as the amount of functional space occupied by the species assemblage; it is independent of abundance. Low FRic values indicate that some potentially available resources are not being exploited. Functional evenness may be seen as how regularly the abundance of species is distributed in the functional space. Low FEve values imply that some parts of the niche are utilized but subexploited. Functional divergence represents how far high abundant species are from the centroid of the functional space. High FDiv values indicate a high degree of niche differentiation in the dominant species (Mouchet et al., 2010; Córdova-Tapia and Zambrano, 2015). In practice, a Principal Coordinates Analysis (PCoA) is performed to represent the species distribution in a multidimensional functional space. The input data of this analysis is a species-by-species distance matrix and the resulting PCoA axes represent the new “traits” that are used in the estimation of the three functional indices, but calculations are not trivial. The input matrix is constructed using the Gower distance index, which allows the mixing of qualitative, quantitative, and binary traits (Villéger et al., 2008). FRic may correspond to species richness because they are based only on the presence of species, whereas FDiv and FEve may correspond to diversity and evenness indices since they incorporate abundances; however, the association between trait diversity and taxonomic indices is not always straightforward (Weiher, 2011; Pomerleau et al., 2015). Several functional diversity indices exist in the literature, but some of them are highly correlated; the three indices here analyzed (FRic, FDiv, and FEve) are independent and complementary to each other (Villéger et al., 2008; Mouchet et al., 2010). Afterward, major functional groups of zooplankton taxa were identified based on a cluster dendrogram illustrating the trait similarities among zooplankters. These calculations were made with the FD library of the R Software.
Finally, a Biological Trait Analysis was performed to detect changes along the estuarine gradient. One approach to this procedure involves the application of a fuzzy correspondence analysis (FCA) to the trait matrix (Chevenet et al., 1994; Bremner et al., 2006). This procedure assigns a score (0 to 3) to each taxon and trait category as follows: 0 being no affinity for the category, and 3 being the higher affinity (Bremner et al., 2006). An estimation of the contribution of each functional trait (bounded between 0 and 1) to the global variability was also made (Pavoine et al., 2009). These calculations were made with the ADE4 library of the R Software. Taxa-by-stations matrix and scored input data used in this study can be found at https://hdl.handle.net/20.500.12201/11347.
Results
Hydrological conditions and zooplankton groups
Temperature values ranged between 25.7 and 30.1°C in June 2018, and between 25.7 and 29.1°C in October 2019. Salinity varied between 18.2 and 32.5 psu in June 2018, and between 2.3 and 34 psu in October 2019, with the highest values towards the mouth and the coastal area (Figure 2). Location of both upper and lower estuaries varied between seasons; hence, the lower estuary (> 25 psu) comprised stations from the channel and outside the lagoon (stations 8 to 11) in October, whereas, in June, only the stations outside the lagoon (11 and 12) corresponded to the lower estuary.
The zooplankton community was represented by 21 major taxa. All of the taxa were registered in the lower estuary, but some of them (thaliaceans, amphipods, heteropods, and larvae of brachiopods and polychaetes) were absent from the upper estuary. The medusae and stomatopod and echinoderm larvae had their major abundance in salinities above 23.2 psu; the pteropods, mysids, ostracods, bryozoan larvae, appendicularias, and molluscan larvae were mainly distributed in salinities above 16.2 psu; the cladocerans, chaetognaths and luciferid larvae were most abundant in salinities above 8.4 psu. Finally, the copepods, luciferids, and larvae of decapods and fishes had a wide distribution in both parts of the estuary; fish larvae were the only group with a higher abundance in the upper estuary (Table 2). The number of taxa increased with raising salinity, from four taxa at 2.3 psu to 20 taxa at 32.5 psu.
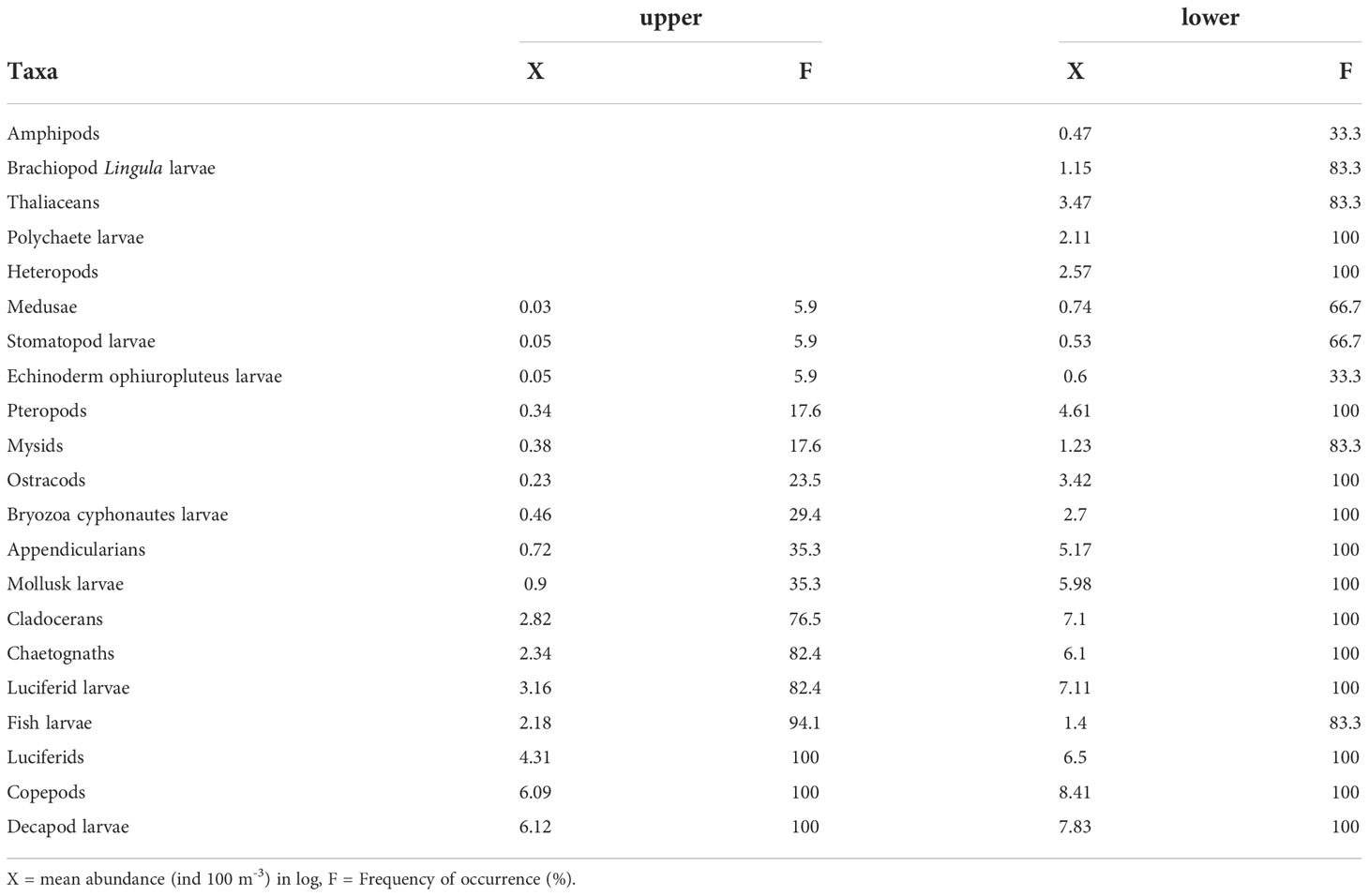
Table 2 Zooplankton groups registered in the upper (< 25 psu) and lower (> 25 psu) zones of the Sontecomapan estuary.
For some groups, we recognized the dominant species or a more specific taxon. Hence, Acartia tonsa (about 80% of the total copepod abundance) was the dominant species for copepods, Parasagitta friderici for chaetognaths, Atlanta lesueurii for heteropods, and Limacina trochiformis for pteropods. The luciferids were represented by Belzebub faxoni and the amphipods by Lestrigonus bengalensis. Within the thaliaceans we recognized doliodids but mostly salps; the larvae of meroplanktonic polychaetes mostly belonged to the family Spionidae.
Zooplankton functional traits
The PERMANOVA results indicated significant differences in the zooplankton composition between the upper and lower estuaries (p< 0.05); however, no differences were detected between seasons (p > 0.05). The representation of sampling stations in an nMDS space illustrates this results (Figure 3). As well, the CWM metric, treated through a PERMANOVA, showed significant differences in the functional traits between the upper and lower estuaries (p< 0.05), but no differences between seasons (p > 0.05).
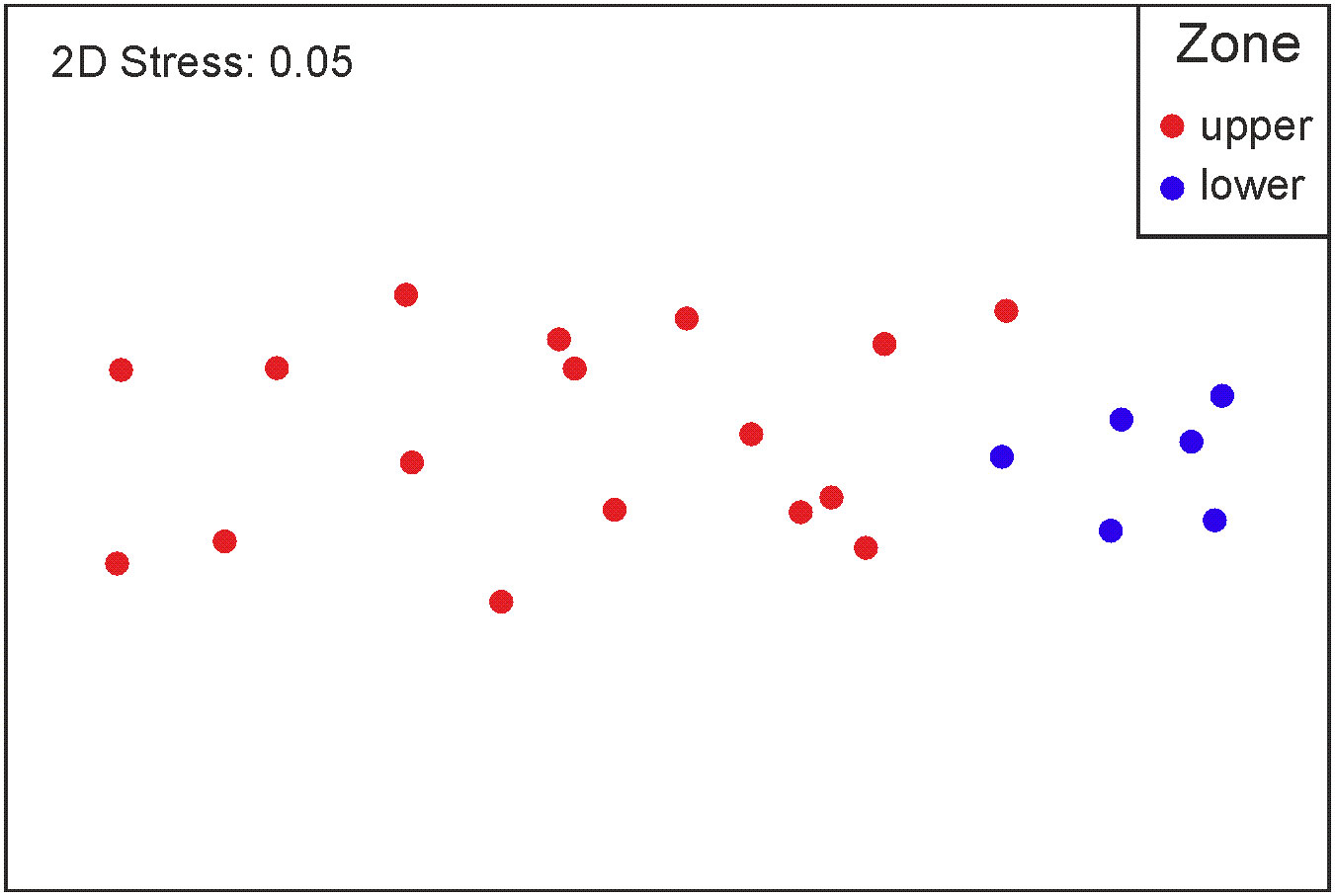
Figure 3 Results of a nMDS showing the ordination of the sampling stations in the Sontecomapan estuary during the two sampling periods.
Results of the FCA showed clear discrimination between the sampling stations of the lower and upper estuaries. In fact, the first axis may represent the salinity gradient along the estuary, with the high-salinity stations (> 25 psu) to the right (Figure 4). Among the functional categories associated with the lower estuary are: filter feeders (feeding strategy), herbivores (trophic group), wings, cilia, and flattened or watery bodies (buoyancy), and setae, shell/carapace, and transparency (protection). These functional categories showed a higher proportion in the lower estuary (Figure 5). In turn, among the characteristics related to the upper zone are: cruising predators (feeding strategy), omnivores (trophic group), appendages and swim bladder (buoyancy), and spines and fast swimming (protection). The ambush feeding strategy, located near the center of the plane, seemed to have an intermediate position and, the carnivores, located to the right side of the plane (Figure 4) showed a slightly higher proportion in the lower estuary (Figure 5).
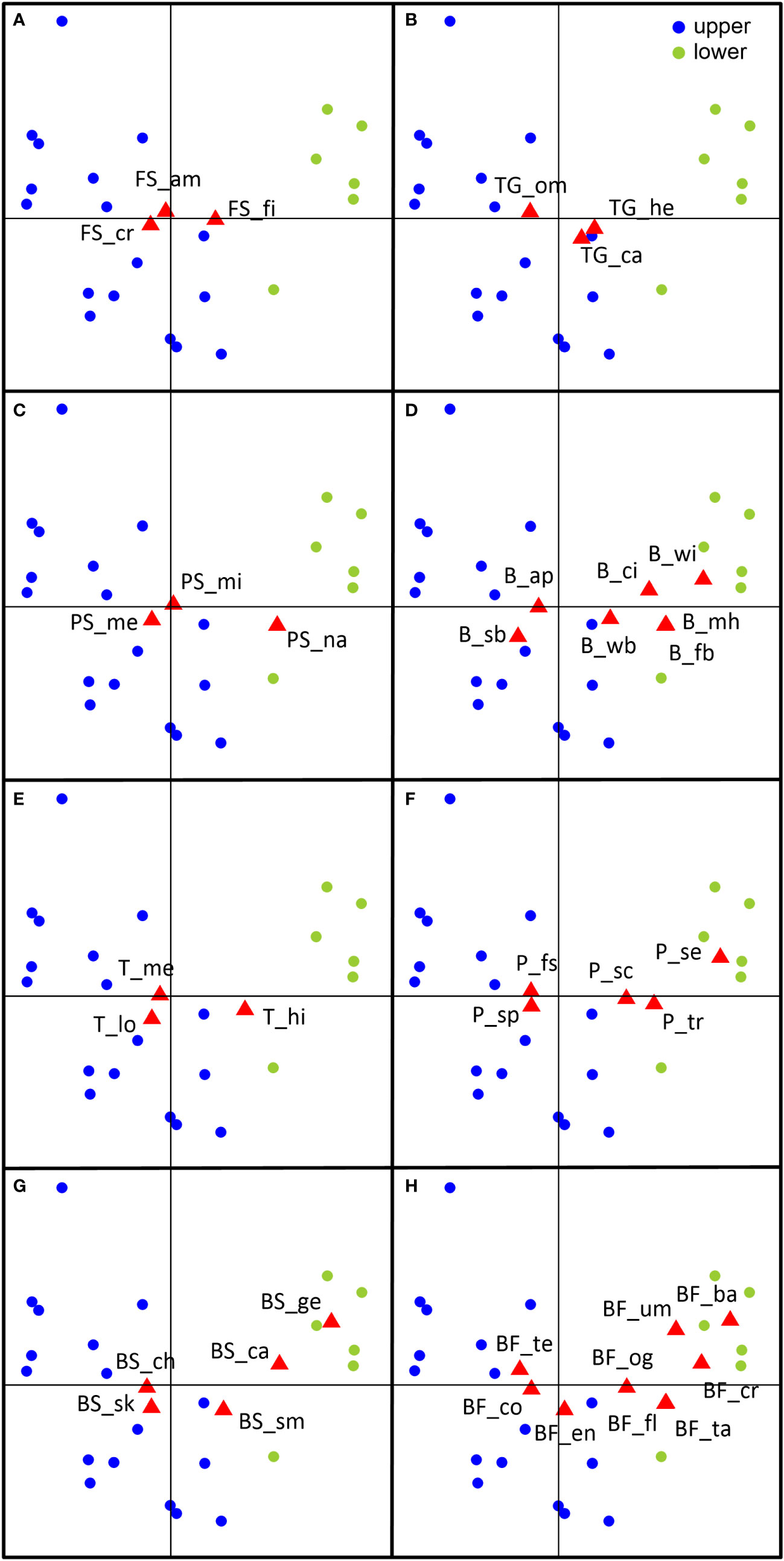
Figure 4 Results of the fuzzy correspondence analysis showing the ordination of sampling stations and trait categories of the Sontecomapan estuary. (A) Feeding strategy, (B) Trophic group, (C) Prey size, (D) Buoyancy, (E) Transparency, (F) Protection, (G) Body structure, (H) Body form. Abbreviations are in Table 1.
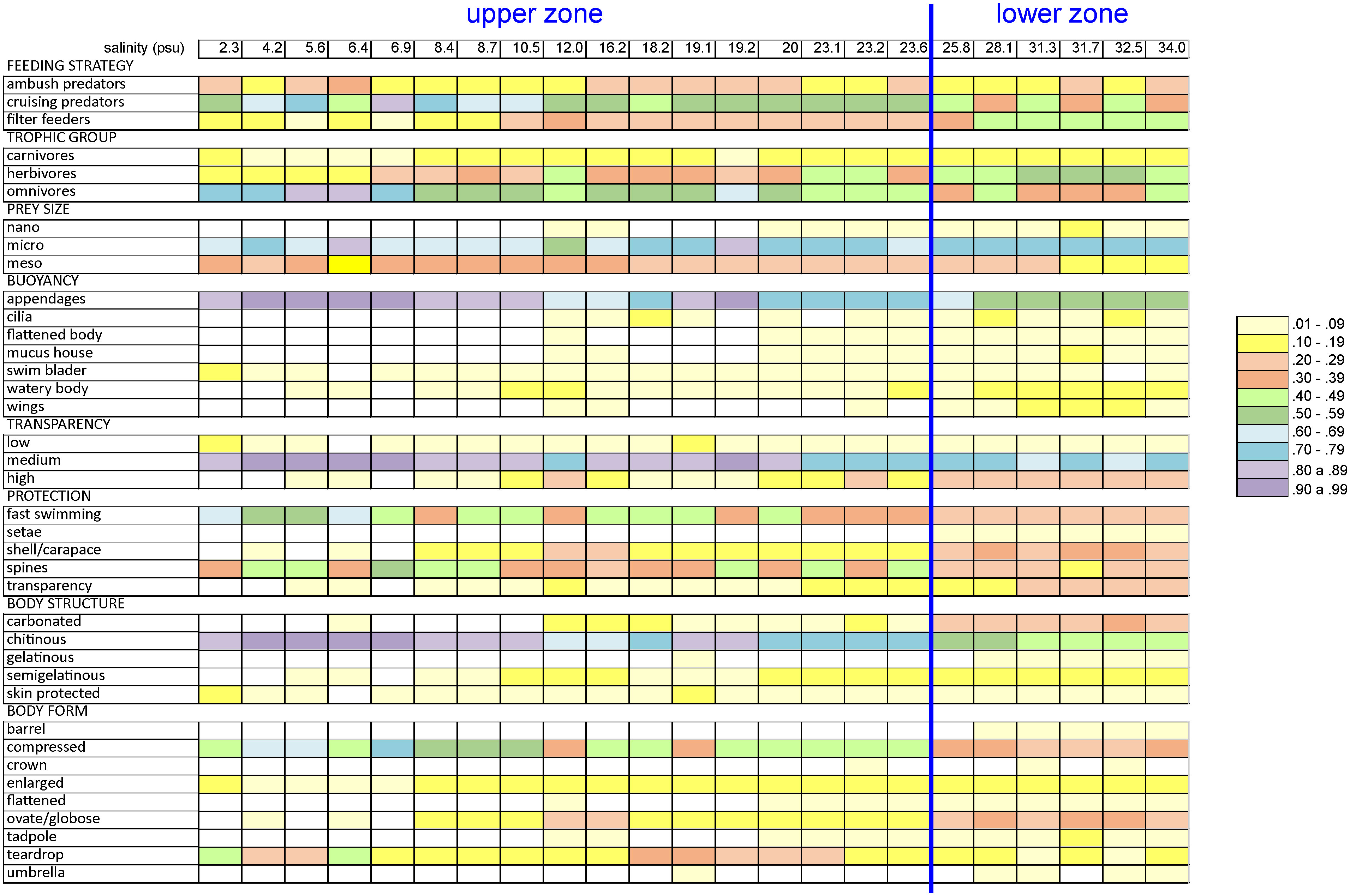
Figure 5 Proportions of the eight functional traits and their corresponding categories related to the salinity values of the Sontecomapan estuary.
The FCA also indicated that major contributions to the global variability were done by the prey size, body structure, and body form (Table 3); however, this result should be taken with caution because all the traits are related and provide complementary information linked to the ecological function of zooplankters. For instance, a gelatinous body structure can be related to highly-transparent bodies and, a compressed body form may be associated with fast swimming behavior.
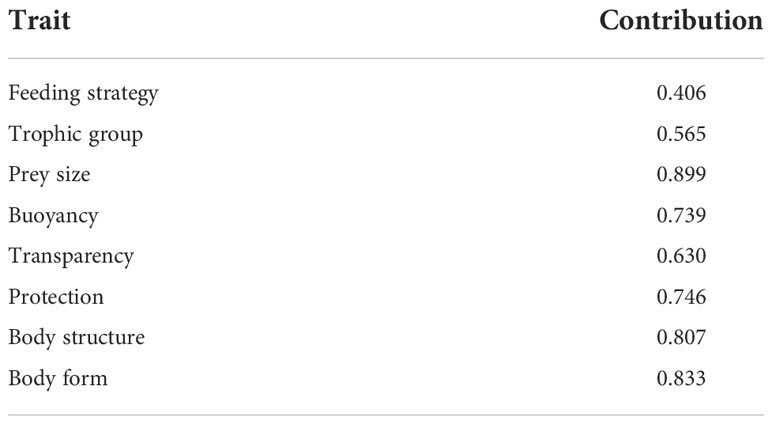
Table 3 Contribution of each functional trait to the global variability of the structure represented in the FCA.
The cluster dendrogram showed two major groups (Figure 6). In general, Group 1 was characterized by herbivorous filter-feeding organisms, with high or medium transparency, and that use the wings, flattened or watery bodies for buoyancy, and the shell/carapaces or transparent bodies for protection. Among the members of this group are the thaliaceans, chaetognaths, medusae, heteropods, pteropods, ostracods, and cladocerans. Group 2 was characterized by cruising predators, some of them omnivores, with medium or low transparency, and that use the appendages for buoyancy and the spines or fast swimming behavior for protection against predators. The luciferids, mysids, copepods, decapod, polychaete, and fish larvae are among the members of this group (Figure 6). As seen, the characteristics of Group 1 are more related to those defined for the lower estuary according to the results of the FCA (Figure 4), whereas Group 2 displays characteristics more related to the upper estuary.
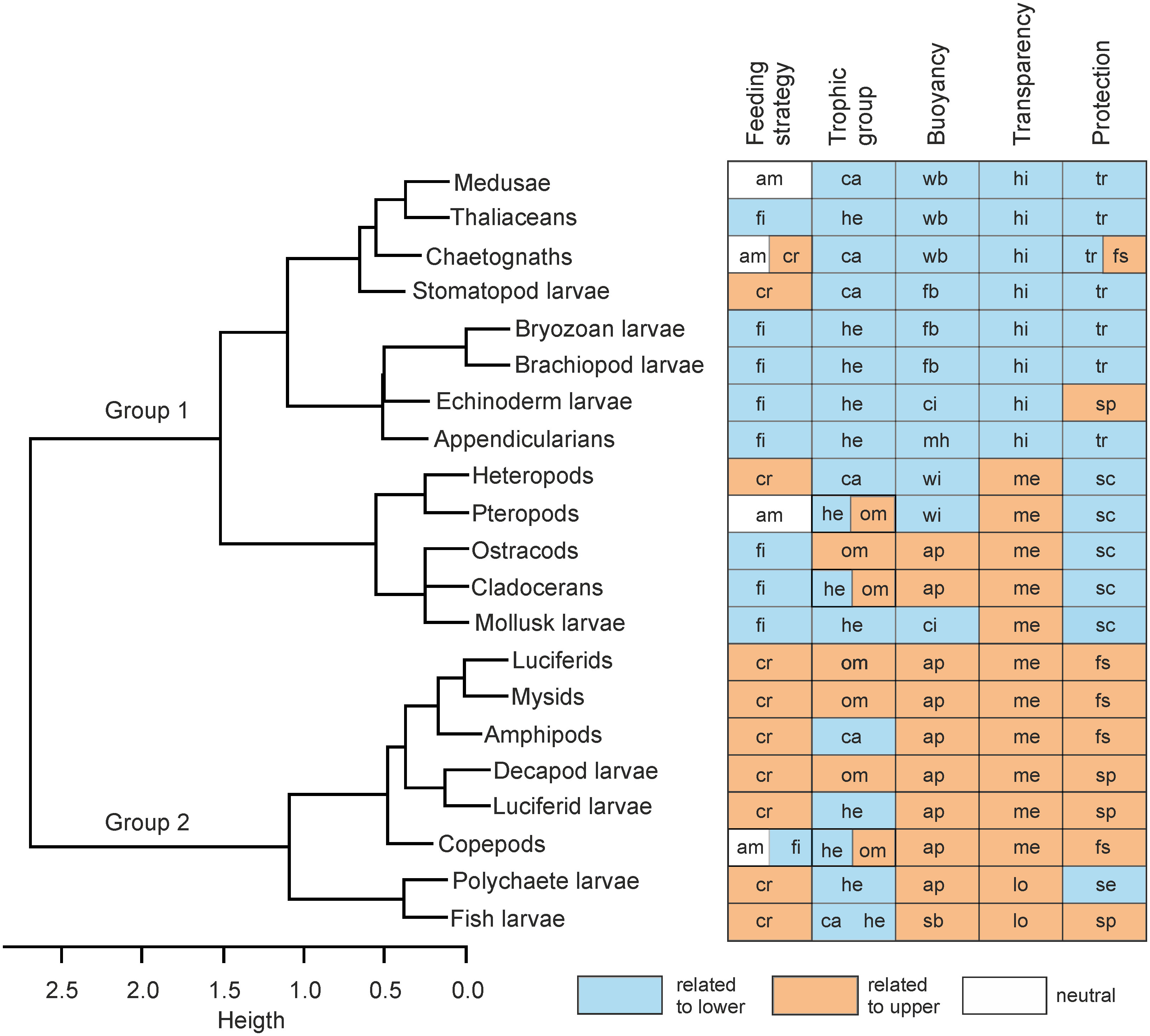
Figure 6 Dendrogram based on trait similarities among zooplankters collected in the Sontecomapan estuary. Abbreviations are in Table 1. Colors indicate the relationship of each category to either the upper or lower estuaries according to the results of the FCA.
The functional indices FRic and FDiv values showed significant differences (t-test, p< 0.05) between the upper and lower zones of the estuary, but no differences were detected in the FEve (Figure 7). Seasonal changes were not statistically significant (p > 0.05).
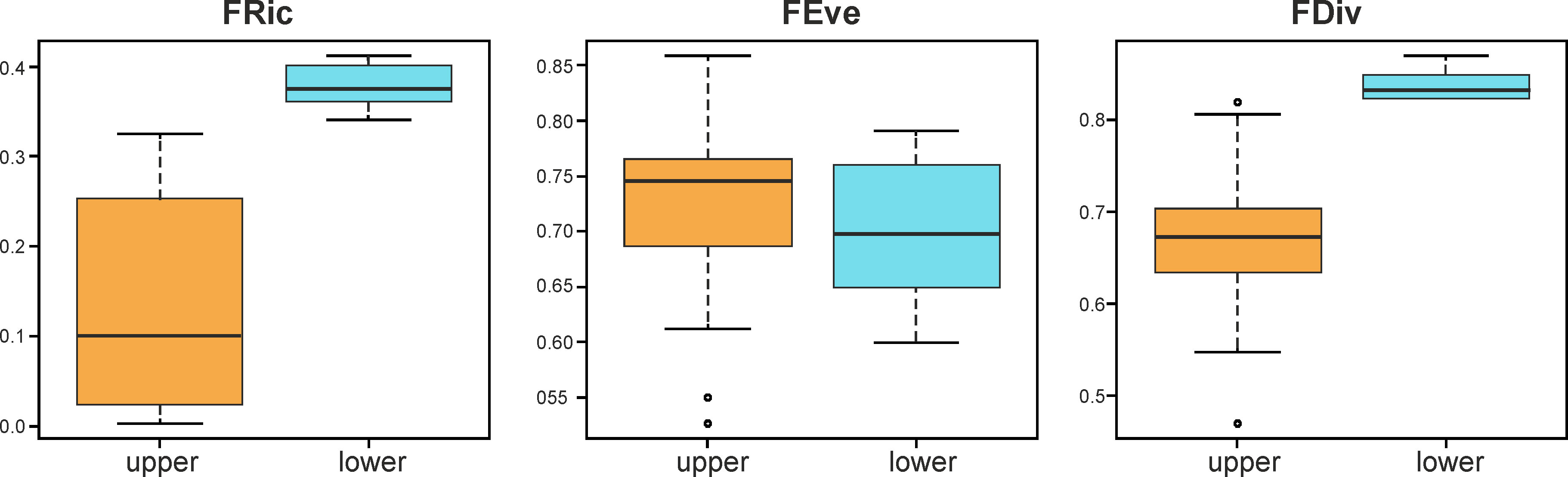
Figure 7 Boxplot of the functional richness (FRic), functional evenness (FEve), and functional divergence (FDiv) values in the upper (< 25 psu) and lower (> 25 psu) zones of an estuary.
Discussion
Differences in environmental conditions between the coastal marine zone and the inner estuary lead to a variation in functional traits and diversity of the zooplankton community. Functional richness (FRic) showed higher values in the lower estuary (Figure 7). Rare taxa in the community and their associated functional traits have a high influence on this index since its estimation does not consider the abundance of taxa (van der Linden et al., 2017). In this study, some traits were exclusively or more frequently encountered in the lower estuary, such as the gelatinous body structure, the presence of wing or mucus houses as structures for buoyancy, as well as the umbrella, barrel, and flattened body forms. These traits (associated with the thaliaceans, heteropods, medusae, or stomatopods) have deep implications for the function of the zooplankton community, as it is discussed in the following section. The loss of species with different combinations of functional traits will cause a decrease in functional richness (Mouillot et al., 2013). The functional divergence (FDiv) values decrease when salinity decreases (Figure 7). This index represents how abundance is spread along the functional trait axes: when the most abundant species have different functional traits, the FDiv value is high (Villéger et al., 2008). For instance, the chaetognaths and the cladocerans, two important zooplankton groups in the lower estuary (Table 2), exhibit distinct functional traits. The chaetognaths are carnivorous ambush or active predators, consuming mainly copepods; they transfer energy from micro- and mesozooplankton to predators at higher position in the pelagic food webs (Kehayias et al., 2005; Saiz, 2009; Sanvicente-Añorve et al., 2020). In turn, the cladocerans are herbivorous filter feeders, they are active algal grazers and constitute a relevant energy link between primary producers and planktonic predators; sometimes, they help control the phytoplankton blooms (Arunpandi et al., 2020; Hébert and Beisner, 2021). Ecologically, a high functional divergence value is indicative of a high degree of niche differentiation and, in consequence, of low resource competition (Mason et al., 2005). We cannot prove this statement, but our results indicated that all the traits here considered were found in the lower estuary (Figure 5): the higher the possibilities to exploit a resource, the lower the competitive interactions.
The zooplankton attributes here incorporated showed functional differences in the feeding ecology, buoyancy, camouflage, and defense against predators between upper and lower estuaries, as we show below.
Feeding strategies
For feeding, zooplankters exhibit a wide variety of morphological, behavioral, and physiological traits (Sanvicente-Añorve et al., 2006; Kiørboe, 2011; Litchman et al., 2013). Thus, it is not always evident to assign a single functional trait to a given species since feeding strategies may change during its development or it can switch depending on food composition or environmental factors (Kiørboe, 2011; Wilken et al., 2013); this problem can be solved using the fuzzy coding procedure (Chevenet et al., 1994). This study revealed differences in the feeding ecology (feeding strategies and trophic structure) between the upper and lower zones of an estuary.
Cruising predators were related to the upper estuary (Figure 4). Fish and decapod larvae and luciferids (all in Group 2) were the most important representants of this trophic guild. Fish larvae use their highly developed visual capabilities to detect prey. Under optimal conditions, their visual range is longer than the perception distance of zooplankters that notice their prey by hydrodynamic signals (Kiørboe, 2011). In general, all fish larvae display similar hunting strategies: once the prey is detected, the larva decreases its swimming velocity to approach the prey. The predator bends its body into an S-shape and attacks the prey with the mouth open to create a suction flow (Holzman and Wainwright, 2009; Kiørboe, 2011). Together with vision, luciferids use chemo- and mechanoreceptors to perceive their prey; they can sense the hydrodynamic disturbances caused by the swimming appendages of the prey (Vega-Pérez et al., 1996). Mechanisms of capturing prey in decapod larvae are poorly studied. In some species, the predator flexes the abdomen to pin the prey to its ventral side and then uses the feeding appendages to eat (Johnson and Allen, 2012). Decapod larvae have well-developed functional eyes used to avoid predation (Charpentier and Cohen, 2015), and probably, to detect their prey.
Filter-feeders were associated with the lower estuary (Figure 4) and were represented by the tunicates (appendicularians and thaliaceans), cladocerans, ostracods, and molluscan larvae (all in Group 1). Appendicularians use an external mucous structure called ‘house’ to filter and concentrate food particles from the water by beating the tail; afterward, particles are captured by an internal pharyngeal filter for ingestion (Conley et al., 2018). The feeding strategy employed by appendicularians enables the ingestion of particles in the pico- and nanoplankton sizes that are not commonly used by other filter-feeders, so they can transfer the energy from the picoplankton to higher trophic levels (Lambert, 2005; Matos et al., 2021). Thus, a major proportion of nanoparticles can potentially be ingested in the lower estuary (Figure 5). In the thaliaceans (salps and doliodids), feeding takes place by filtering the water through a mucous mesh where food is captured and moved to the esophagus. Their barrel-shaped body is fundamental for pumping water: during the process, the water enters by the oral siphon and exits by the atrial one (Henschke et al., 2016; Conley et al., 2018). Larvae of meroplanktonic mollusks use their cilia to swim or generate micro-currents to feed (Chan et al., 2013). In cladocerans, thoracic legs are provided with rows of setae and spines that serve for pumping, filtering, and handling food; legs beat rhythmically and particles stick to the flat surfaces and setae rows and then pass to the mouth (Dodson et al., 2009). Ostracods can produce feeding currents by beating their vibratory plates, although direct attacks on prey are also possible (Lochhead, 1968). The ecological significance of these feeding mechanisms has not been evaluated. Suspended organic matter and other particles in estuaries represent a survival risk for filter-feeders since suspended material may clog the filtering structures (Nogueira et al., 2018). This could be the reason for a higher proportion of filter-feeders in the marine-influenced area.
The ambush feeding strategy had an intermediate position (Figure 4), with similar proportions in both parts of the estuary (Figure 5). Copepods and chaetognaths, with a wide distribution in the study area, were among the most important representants of this group. Acartia tonsa, the dominant copepod species, detects its prey by hydrodynamic signals. Once the prey is detected, the copepod lunges forward and orients in a way that the prey is placed ventral to the feeding appendages; a vacuum is then created, and the prey is sucked (Kiørboe, 2011). This species is capable of switching its feeding strategy from ambush to filter-feeding and vice-versa, depending on food composition (Kiørboe et al., 1996). Chaetognaths have developed different foraging tactics depending on their buoyant properties. Species with neutral buoyancy wait immobile for their prey and once detected, they perform quick jumps to catch the prey; species with negative buoyancy tend to be more active predators alternating short periods of swimming and sinking for searching prey (Saiz, 2009).
Trophic groups
Herbivores were related to the lower estuary, according to the results of the FCA (Figure 4). The appendicularians, thaliaceans (salps and doliolids), pteropods, and cladocerans, all members of Group 1 (Figure 6), were among the most important representatives of this trophic level. Field observations showed that appendicularians exhibit a seasonal abundance pattern coincident with the phytoplankton bloom; however, the bacterioplankton is also an important part of their diet (Capitanio et al., 2018). As well, for salps and doliolids, the phytoplankton constitutes their main food and phytoplankton blooms can trigger some ecological processes (Lambert, 2005; Henschke et al., 2016). Based on the analysis of gut and fecal pellets, thecosomatous pteropods have been commonly considered herbivorous organisms feeding upon phytoplankton; however, they also consume dinoflagellates, radiolarians, foraminiferans, and tintinnids (Lalli and Gilmer, 1989; Gilmer and Harbison, 1991). Cladocerans mostly consume algae, but they can also eat protozoans and nauplii larvae; food can be rejected if it contains toxic alga or is unpalatable (Dodson et al., 2009). In situ cultures of luciferids evidenced that larvae eat upon phytoplankton showing selectivity by certain algae (Zimmerman, 1973).
The omnivores were associated with the upper estuary (Figure 4) and were represented by the copepods, adult luciferids, and decapod larvae, all in Group 2 (Figure 6). From several in situ experiments realized in a harbor in California, Kleppel (1992) concluded that A. tonsa eats on both phytoplankton and microzooplankton and that selectivity was infrequent. Luciferids can consume microalgae and small zooplankters, as evidenced by the laboratory experiments and the morphological analysis of their feeding appendages (Lee et al., 1992). Field observations indicated that food availability is the most important factor determining the distribution of Belzebub faxoni (Sanvicente-Añorve et al., 2021), the only luciferid species here registered. Laboratory experiments revealed that decapod larvae are generally omnivorous; while some early larvae catch microalgae and other small particles, late larval forms grasp food items with their appendages (Johnson and Allen, 2012). Due to the broad diet of omnivores they can survive in highly variable environments, such as estuaries.
Carnivores had little higher proportions towards the lower estuary (Figure 5). Chaetognaths and fish larvae, with a wide distribution in the study area, were the most abundant members of this trophic group. Generally, fish larvae feed on a variety of micro- and mesozooplankton organisms, with larvae and adult copepods as their main prey (Hunter, 1981; Jackson and Lenz, 2016). For chaetognaths, the primary food consists of copepods, but they can also consume gelatinous organisms (Marazzo et al., 1997; Giesecke and Gonzalez, 2008; Sanvicente-Añorve et al., 2020). Other carnivorous organisms, such as stomatopod larvae, heteropods, and amphipods, mostly represented in the lower estuary (Table 2), accounted for the small differences between the two zones.
Buoyancy
For buoyancy, results of the FCA showed that the presence of appendages and swim bladders were related to the upper estuary (Figure 4). These functional categories characterized the members of Group 2 (Figure 6). A manner to increase frictional resistance to water is to modify the shape of the body or to develop spines, appendages, or other body projections (Molloy and Cowling, 1999), which results in an increase in body surface area without increasing density. Many pelagic crustaceans have developed this buoyancy mechanism to remain in a certain position in the water column. Copepods, for instance, use their long antennae to slow their sinking: antennae can spread to increase water resistance when the animal is sinking, or fold to diminish drag when it is swimming up (Alexander, 1990). Luciferids increase the frictional resistance to sinking by using their flabellate uropods as well as their numerous abdominal and thoracic appendages (Sanvicente-Añorve et al., 2021). Besides body projections zooplankters have developed several strategies to stay buoyant in the waters, such as the use of gas-filled sacs or swim bladders. Many teleostean fishes use this mechanism to control their buoyancy in the water column. While growing, a fish larva develops dense body structures (bone, cartilage, muscle) resulting in changes in buoyancy and sinking speed during its developmental stage (Alexander, 1990; Lindsey et al., 2010). By inflating the swim bladder with gas, the density of the larva decreases allowing the animal to attain a neutral buoyancy (Lindsey et al., 2010).
The lower estuary was characterized by flattened forms, watery bodies, and the use of mucus houses and wings for buoyancy (Figures 4, 6). Similar to body projections, flattened forms also increase the surface area-to-volume ratio to avoid sinking, such as in larvae of stomatopods, bryozoans, and brachiopods. The most conspicuous morphological characteristic in stomatopod larvae is a large dome-shaped or flattened carapace that encloses a great portion of the body and helps the larvae to remain in the water column. The carapace is equipped with a rostrum and long spines that provide additional buoyancy and protection from predators (Haug et al., 2016). Another strategy to avoid sinking consists of trying to emulate the density of the seawater. For instance, gelatinous animals have evolved to match the density of their watery bodies to that of the surrounding water (Alexander, 1990). Salps, medusae, and heteropods maintain their buoyancy by exchanging heavy ions, such as sulfates, with lighter but osmotically similar ions, such as chlorides (Molloy and Cowling, 1999). A similar situation is for chaetognaths (Kapp, 1991). Other morphological characteristics to provide buoyancy are some external structures (mucous houses in appendicularians) and the ‘hydrofoils’ (wings in holoplanktonic mollusks). As stated, appendicularians secret an external “house” around their bodies which serves for filter-feeding and provides buoyancy to these small solitary organisms (Holland, 2016). Some organisms must swim to remain in the plankton (Molloy and Cowling, 1999). In pteropods, the weight of the shell causes the animal to sink. Among the morphological modifications of pteropods to planktonic life is that a portion of the foot has been modified in the form of two swimming wings (Lalli and Gilmer, 1989). The rhythmic movement of the wings allows the animal to swim and avoid sinking (Molloy and Cowling, 1999; Manno et al., 2017). Besides, the deployment of a mucous web used to trap its food enables pteropods to hang motionless in the water column (Harbison and Gilmer, 1992; Manno et al., 2017).
Camouflage and defense against predators
In this study, the ‘high’ category of transparency was related to the lower estuary (Figure 4); highly transparent organisms, such as thaliaceans, medusae, chaetognaths and appendicularians, were classed in Group 1 (Figure 6). In accordance, gelatinous and semi-gelatinous animals registered higher proportions in the lower zone (Figure 5). Probably, the main advantage of gelatinous material is the transparency it provides. By keeping the light reflection to a minimum, gelatinous organisms hide from predators and, at the same time, stalk their own prey (Parker, 1999; Johnsen, 2000). The incorporation of seawater into the body of gelatinous organisms results in similar refractive indices between the animals and the surrounding marine environment, resulting in similar light transmission characteristics (Herring, 2002). This may explain why the marine-influenced area of the estuary had a major proportion of highly-transparent organisms (Figure 5).
The ‘medium’ and ‘low’ categories of transparency were more related to the upper estuary (Figure 4). Organisms with a medium transparency level were widely distributed in the study area (classed in Groups 1 and 2) but showed a higher proportion in the upper part (Figure 5). Chitinous and skin-protected organisms are respectively related to those levels of transparency. An effective camouflage in aquatic habitats consists in mimicking the lighting effects in the environment. This phenomenon can be observed in chitinous animals, in which light is reflected by alternating layers of high- and low-density chitin of different refractive indices (Parker, 1999). Several terrestrial and marine species exhibit this kind of camouflage (Parker et al., 1998a; Parker et al., 1998b) but copepods or luciferids, common crustaceans in this study, have not been yet analyzed. Skin seems to be a problematic organ because it always reflects some light, making the organisms visible to predators; the problem can be solved by reducing the amount of skin and then, the reflection of light (Johnsen, 2000). This seems to be the case with fish larvae and polychaete larvae.
In the lower estuary, protective characteristics against predators are the high transparency in the animals above discussed, the use of shells or carapaces (in pteropods, heteropods, ostracods, cladocerans, and larvae of meroplanktonic mollusks), and the setae (in polychaete larvae) (Figure 4). Most of these organisms belong to Group 1 (Figure 6). The physical and chemical characteristics of the water in estuaries affect the calcifying processes during the shell formation of organisms. The availability of calcium carbonate for shell growth in the lower part of estuaries is higher than in the upper part due to higher salinity and alkalinity conditions (Waldbusser et al., 2011). This may explain the higher proportion of calcified organisms in the marine-influenced part of the Sontecomapan estuary. In the case of polychaete larvae, evidence suggests that they can use their long bristles or setae to deter predators having different feeding mechanisms (Pennington and Chia, 1984).
In the upper estuary, common protective characteristics were the use of spines (in fish and decapod larvae) and a fast swimming escape behavior (in some crustaceans and chaetognaths) (Figure 4). These protective features are common in organisms of Group 2 (Figure 6). In response to predation, crustacean larvae have developed diverse morphological defenses, such as long spines. The carapace and abdomen of decapod larvae are armed with a variable number of spines that limit predators with small gape sizes to prey upon and, in some cases, the spines can be poisonous to predators (Østergaard et al., 2005; Bashevkin and Morgan, 2020). Similarly, the spines of fishes can ‘increase’ their body dimensions reducing the capture success of predators or making the fish difficult to eat (Price et al., 2015). Among crustaceans, fast escape behavior is associated with copepods, luciferids, and mysids (Buskey et al., 2002; Johnson and Allen, 2012). Body in luciferids and mysids are laterally compressed allowing them to be good swimmers. For instance, when swimming, mysids can suddenly make abdominal flexions to change direction and evade predators (Johnson and Allen, 2012). Copepods exhibit a strong potential to detect and escape from predators. The calanoid Acartia tonsa avoids contact with its predators by making repeated jumps at an average velocity of 75.8 mm s-1 in females, and 61.7 mm s-1 in males (Suchman and Sullivan, 1998).
Conclusions
For years, researchers have demonstrated the changes in the community composition and structure in estuaries and coastal lagoons based on the degree of marine water intrusion. In this study, we also evidenced differences in the zooplankton ecosystem function between the upper (< 25 psu) and lower (> 25 psu) zones of an estuary, through the analysis of three functional indices (FRic, FEve, and FDiv), the ‘community-weighted mean trait values’ metric (CWM) and a fuzzy correspondence analysis (FCA). The functional richness (FRic) and functional divergence (FDiv) showed significantly higher values in the lower estuary. High FRic values indicated that zooplankters with rare or extreme functional traits are present, and their loss will result in a decrease in the FRic values; high FDiv values indicated that the most abundant zooplankters have extreme functional traits. Ecologically, high FRic values may indicate that available resources can be exploited in a variety of ways, whereas high FDiv values may show a high level of niche differentiation and then, low competition among organisms.
The CWM and the FCA showed that the lower estuary can be mostly characterized by herbivorous filter-feeding organisms that use watery or flattened bodies, cilia, or wings for buoyancy, highly-transparent bodies for camouflage, and shells or carapaces as protection against predators. The upper estuary was mainly characterized by omnivorous cruising predators that use the appendages or swim bladders for buoyancy, and spines or fast swimming behavior to protect from predators. This study provides new information on the ecological function of zooplankters in estuaries.
Data availability statement
The original contributions presented in the study are included in the article/supplementary materials. Further inquiries can be directed to the corresponding author.
Author contributions
LS-A conceptualized the study. LS-A and MA-M were involved in the funding acquisition. MS-C and EL-S performed the laboratory analysis. LS-A, MA-M, and EG-C analyzed the data. LS-A, MS-C, and EL-S contributed to constructing the zooplankton functional traits database. All authors read and approved the original draft.
Funding
This study was financially supported by the Universidad Nacional Autónoma de México through the Programa de Apoyo a Proyectos de Investigación e Innovación Tecnológica (UNAM-PAPIIT IN206720 project).
Acknowledgments
The authors gratefully acknowledge Rosamond Coates, director of the Estación de Biología los Tuxtlas of the Instituto de Biología (UNAM) for the valuable support we received during the fieldwork. The authors also thank Faustino Zavala-García for his assistance with the graphics and Mario Martínez-Mayén who helped us with sampling processing. A special thanks goes to the two reviewers whose comments greatly improved the manuscript.
Conflict of interest
The authors declare that the research was conducted in the absence of any commercial or financial relationships that could be construed as a potential conflict of interest.
Publisher’s note
All claims expressed in this article are solely those of the authors and do not necessarily represent those of their affiliated organizations, or those of the publisher, the editors and the reviewers. Any product that may be evaluated in this article, or claim that may be made by its manufacturer, is not guaranteed or endorsed by the publisher.
References
Aké-Castillo J. A., Vázquez G. (2008). Phytoplankton variation and its relation to nutrients and allochthonous organic matter in a coastal lagoon on the gulf of Mexico. Estuar. Coast. Shelf Sci. 78, 705−714. doi: 10.1016/j.ecss.2008.02.012
Alexander R. M. (1990). Size, speed and buoyancy adaptations in aquatic animals. Am. Zool. 30, 189−196. doi: 10.1093/icb/30.1.189
Arunpandi N., Jyothibabu R., Jagadeesan L., Albin K. J., Savitha K. M. M., Parthasarathi S. (2020). Impact of salinity on the grazing rate of a cladocera (Latonopsis australis) in a large tropical estuarine system. Environ. Monit. Assess. 192 (2), 1−13. doi: 10.1007/s10661-020-8068-x
Barnett A. J., Finlay K., Beisner B. E. (2007). Functional diversity of crustacean zooplankton communities: towards a trait-based classification. Freshw. Biol. 52, 796−813. doi: 10.1111/j.1365-2427.2007.01733.x
Bashevkin S. M., Morgan S. G. (2020). “Predation and competition,” in The natural history of the crustacea: developmental biology and larval ecology, volume 7. Eds. Anger K., Harzsch S., Thiel M. (Oxford: Oxford University Press), 360−383. doi: 10.1093/oso/9780190648954.001.0001
Benedetti F., Gasparini S., Ayata S. D. (2016). Identifying copepod functional groups from species functional traits. J. Plankton Res. 38, 159−166. doi: 10.1093/plankt/fbv096
Benfield M. C. (2013). “Estuarine zooplankton,” in Estuarine ecology. Eds. Day J. W., Crump B. C., Kemp W. M., Yáñez-Arancibia A. (Hoboken: Wiley-Blackwell), 285−302. doi: 10.1002/9781118412787
Bremner J., Rogers S. I., Frid C. L. J. (2006). Methods for describing ecological functioning of marine benthic assemblages using biological traits analysis (BTA). Ecol. Indic. 6 (3), 609−622. doi: 10.1016/j.ecolind.2005.08.026
Buskey E. J., Lenz P. H., Hartline D. K. (2002). Escape behavior of planktonic copepods in response to hydrodynamic disturbances: high speed video analysis. Mar. Ecol. Prog. Ser. 235, 135−146. doi: 10.3354/meps235135
Capitanio F. L., Spinelli M. L., Presta M. L., Aguirre G. E., Cervetto G., Pájaro M., et al. (2018). “Ecological role of common appendicularian species from shelf waters off Argentina,” in Plankton ecology of the southwestern Atlantic. Eds. Hoffmeyer M. S., Sabatini M. E., Brandini F. P., Calliari D. L., Santinelli N. H. (Cham: Springer International Publishing), 201−218. doi: 10.1007/978-3-319-77869-3_10
Castellani C., Edwards M. (2017). Marine plankton: a practical guide to ecology, methodology, and taxonomy (Oxford: Oxford University Press). doi: 10.1093/oso/9780199233267.001.0001
Chan K. Y. K., Jiang H., Padilla D. K. (2013). The swimming speed of larval snail does not correlate with size and ciliary beat frequency. PloS One 8, e82764. doi: 10.1371/journal.pone.0082764
Charpentier C. L., Cohen J. H. (2015). Chemical cues from fish heighten visual sensitivity in larval crabs through changes in photoreceptor structure and function. J. Exp. Biol. 218, 3381−3390. doi: 10.1242/jeb.125229
Chevenet F., Dolédec S., Chessel D. (1994). A fuzzy coding approach for the analysis of long-term ecological data. Freshw. Biol. 31 (3), 295−309. doi: 10.1111/j.1365-2427.1994.tb01742.x
Clarke K. R., Gorley R. N., Somerfield P. J., Warwick R. M. (2014). Change in marine communities: an approach to statistical analysis and interpretation (Plymouth: PRIMER-E, Ltd).
Conley K. R., Lombard F., Sutherland K. R. (2018). Mammoth grazers on the ocean’s minuteness: a review of selective feeding using mucous meshes. Proc. R. Soc B 285, 20180056. doi: 10.1098/rspb.2018.0056
Córdova-Tapia F., Zambrano L. (2015). La diversidad funcional en la ecología de comunidades. Ecosistemas 24 (3), 78–87. doi: 10.7818/ECOS.2015.24-3.10
Díaz S., Purvis A., Cornelissen J. H. C., Mace G. M., Donoghue M. J., Ewers R. M., et al. (2013). Functional traits, the phylogeny of function, and ecosystem service vulnerability. Ecol. Evol. 3, 2958−2975. doi: 10.1002/ece3.601
Dodson S., Cáceres C. E., Rogers D. C. (2009). “Cladocera and other Branchiopoda,” in Ecology and classification of north American freshwater invertebrates. Eds. Thorp J. H., Covich A. P. (London: Elsevier Academic Press), 773−827. doi: 10.1016/c2009-0-02669-5
Esquivel-Herrera A., Soto-Castor R. (2018). “A multivariate approach to the spatial and temporal water characterization parameters at the tropical Sontecomapan lagoon, Mexico,” in Ecology of the Sontecomapan lagoon, Veracruz. Eds. Castellanos-Páez M. E., Esquivel-Herrera A., Aldeco-Ramírez J., Pagano M. (Mexico City: IRD Editions, UAM-X), 11−33.
Garnier E., Cortez J., Billès G., Navas M. L., Roumet C., Debussche M., et al. (2004). Plant functional markers capture ecosystem properties during secondary succession. Ecology 85, 2630−2637. doi: 10.1890/03-0799
Gasca R., Suárez E. (1996). Introducción al estudio del zooplancton marino (Chetumal: ECOSUR-CONACYT).
Giesecke R., Gonzalez H. E. (2008). Reproduction and feeding of Sagitta enflata in the Humboldt current system off Chile. ICES J. Mar. Sci. 65, 361−370. doi: 10.1093/icesjms/fsn030
Gilmer R. W., Harbison G. R. (1991). Diet of Limacina helicina (Gastropoda: Thecosomata) in Arctic waters in midsummer. Mar. Ecol. Prog. Ser. 77, 125−134. doi: 10.3354/MEPS077125
González-Fierro A., Ponce-Vélez G. (2018). “Metal pollution in aquatic ecosystems: a case study on Sontecomapan coastal lagoon (State of Veracruz) considered as a reference of pristine ecosystems,” in Ecology of the Sontecomapan lagoon, Veracruz. Eds. Castellanos-Páez M. E., Esquivel-Herrera A., Aldeco-Ramírez J., Pagano M. (Mexico City: IRD Editions, UAM-X), 257−293.
Goździejewska A. M., Koszałka J., Tandyrak R., Grochowska J., Parszuto K. (2021). Functional responses of zooplankton communities to depth, trophic status, and ion content in mine pit lakes. Hydrobiologia 848, 2699−2719. doi: 10.1007/s10750-021-04590-1
Harbison G. R., Gilmer R. W. (1992). Swimming, buoyancy and feeding in shelled pteropods: a comparison of field and laboratory observations. J. Moll. Stud. 58, 337–339. doi: 10.1093/mollus/58.3.337
Haug C., Ahyong S. T., Wiethase J. H., Olesen J., Haug J. T. (2016). Extreme morphologies of mantis shrimp larvae. Nauplius 24, e2016020. doi: 10.1590/2358-2936e2016020
Hébert M. P., Beisner B. E. (2021). “Functional trait approaches for the study of metazooplankton ecology,” in Zooplankton ecology. Eds. Teodósio M. A., Barbosa A. B. (Boca Raton: CRC Press), 3−27. doi: 10.1201/9781351021821
Hébert M. P., Beisner B. E., Maranger R. (2016). A meta-analysis of zooplankton functional traits influencing ecosystem function. Ecology 97, 1069−1080. doi: 10.1890/15-1084.1
Henschke N., Everett J. D., Richardson A. J., Suthers I. M. (2016). Rethinking the role of salps in the ocean. Trends. Ecol. Evol. 31, 720−733. doi: 10.1016/j.tree.2016.06.007
Holzman R., Wainwright P. C. (2009). How to surprise a copepod: strike kinematics reduce hydrodynamic disturbance and increase stealth of suction-feeding fish. Limnol. Oceanogr. 54, 2201−2212. doi: 10.4319/lo.2009.54.6.2201
Hunter J. R. (1981). “Feeding ecology and predation of marine larvae,” in Marine fish larvae: morphology, ecology and relation to fisheries. Ed. Lasker R. (Seattle: University of Washington Press), 34−77.
Jackson J. M., Lenz P. H. (2016). Predator-prey interactions in the plankton: larval fish feeding on evasive copepods. Sci. Rep. 6, 1−11. doi: 10.1038/srep33585
Johnson W. S., Allen D. M. (2012). Zooplankton of the Atlantic and gulf coasts: a guide to their identification and ecology (Baltimore: Johns Hopkins University Press). doi: 10.1353/book.19394
Kapp H. (1991). Some aspects of buoyancy adaptations of chaetognaths. Helgolander Meeresunters. 45, 263−267. doi: 10.1007/BF02365646
Kehayias G., Michaloudi E., Koutrakis E. (2005). Feeding and predation impact of chaetognaths in the north Aegean Sea (Strymonikos and Ierissos gulfs). J. Mar. Biol. Assoc. U. K. 85 (6), 1525−1532. doi: 10.1111/j.1469-185X.2010.00148.x
Kiørboe T. (2011). How zooplankton feed: mechanisms, traits and trade‐offs. Biol. Rev. (2022) 86, 311–339. doi: 10.1111/j.1469-185X.2010.00148.x
Kiørboe T., Saiz E., Viitasalo M. (1996). Prey switching behaviour in the planktonic copepod Acartia tonsa. Mar. Ecol. Prog. Ser. 143, 65−75. doi: 10.3354/meps143065
Kleppel G. S. (1992). Environmental regulation of feeding and egg production by Acartia tonsa off southern California. Mar. Biol. 112, 57−65. doi: 10.1007/BF00349728
Laliberté E., Legendre P. (2010). A distance-based framework for measuring functional diversity from multiple traits. Ecology 91, 299−305. doi: 10.1890/08-2244.1
Lalli C., Gilmer R. W. (1989). Pelagic snails. the biology of holoplanktonic gastropod mollusks (Stanford: Stanford University Press).
Lambert G. (2005). Ecology and natural history of the protochordates. Can. J. Zool. 83, 34−50. doi: 10.1139/z04-156
Lavorel S., Grigulis K., McIntyre S., Williams N. S. G., Garden D., Dorrough J., et al. (2008). Assessing functional diversity in the field methodology matters! Funct. Ecol. 22, 134−147. doi: 10.1111/j.1365-2435.2007.01339.x
Lee W. Y., Omori M., Peck R. W. (1992). Growth, reproduction and feeding behavior of the planktonic shrimp, Lucifer faxoni Borradaile, off the Texas coast. J. Plankton Res. 14, 61−69. doi: 10.1093/plankt/14.1.61
Li K. Z., Yin J. Q., Huang L. M., Tan Y. H. (2006). Spatial and temporal variations of mesozooplankton in the pearl river estuary, China. Estuar. Coast. Shelf Sci. 67 (4), 543−552. doi: 10.1016/j.ecss.2005.12.008
Li Y., Ge R., Chen H., Zhuang Y., Liu G., Zheng Z. (2022). Functional diversity and groups of crustacean zooplankton in the southern Yellow Sea. Ecol. Indic 136, 108699. doi: 10.1016/j.ecolind.2022.108699
Lindsey B. W., Smith F. M., Croll R. P. (2010). From inflation to flotation: contribution of the swimbladder to whole-body density and swimming depth during development of the zebrafish (Danio rerio). Zebrafish 7, 85−96. doi: 10.1089/zeb.2009.0616
Litchman E., Ohman M. D., Kiørboe T. (2013). Trait-based approaches to zooplankton communities. J. Plankton Res. 35, 473−484. doi: 10.1093/plankt/fbt019
Lochhead J. H. (1968). The feeding and swimming of Conchoecia (Crustacea, Ostracoda). Biol. Bull. 134, 456−464. doi: 10.2307/1539863
López-Portillo J., Lara-Domínguez A. L., Vázquez G., Aké-Castillo J. A. (2017). Water quality and mangrove-derived tannins in four coastal lagoons from the gulf of Mexico with variable hydrologic dynamics. J. Coast. Res. 77, 28−38. doi: 10.2112/S177-004.1
Manno C., Bednaršek N., Tarling G. A., Peck V. L., Comeau S., Adhikari D., et al. (2017). Shelled pteropods in peril: assessing vulnerability in a high CO2 ocean. Earth-Sci. Rev. 169, 132−145. doi: 10.1016/j.earscirev.2017.04.005
Marazzo A., Machado C. F., Nogueira C. S. R. (1997). Notes on feeding of Chaetognatha in Guanabara bay, Brazil. J. Plankton Res. 19, 819−828. doi: 10.1093/plankt/19.7.819
Mason N. W., Mouillot D., Lee W. G., Wilson J. B. (2005). Functional richness, functional evenness and functional divergence: the primary components of functional diversity. Oikos 111, 112−118. doi: 10.1111/j.0030-1299.2005.13886.x
Mateus M., Mateus S., Baretta J. W. (2008). “Basic concepts of estuarine ecology”,” in Perspectives on integrated coastal zone management in south America. Eds. Neves R., Baretta J. W., Mateus M. (Lisbon: IST Press), 3−14. doi: 10.13140/2.1.4497.0562
Matos A., Ledoux J. B., Domínguez-Pérez D., Almeida D., Antunes A. (2021). “Omics advances in the study of zooplankton: big data for small drifting organisms,” in Zooplankton ecology. Eds. Teodósio M. A., Barbosa A. B. (Boca Raton: CRC Press), 264−277. doi: 10.1201/9781351021821
McLusky D. S., Elliott M. (2004). “The estuarine environment,” in The estuarine ecosystem: ecology, threats and management, ”. Eds. McLusky D. S., Elliott M. (New York: Oxford University Press), 1−18. doi: 10.1093/acprof:oso/9780198525080.003.0001
Molloy P. J., Cowling M. J. (1999). Buoyancy mechanisms of marine organisms: lessons from nature. Underw. Technol. 24, 41−49. doi: 10.3723/175605499783259785
Monroy-Ojeda A., Isern S. G. (2013). Noteworthy bird records in Sontecomapan, Veracruz, México. Acta Zool. Mex. 29 (3), 666−676. doi: 10.21829/azm.2013.2931605
Mouchet M. A., Villéger S., Mason N. W., Mouillot D. (2010). Functional diversity measures: an overview of their redundancy and their ability to discriminate community assembly rules. Funct. Ecol. 24 (4), 867–876. doi: 10.1111/j.1365-2435.2010.01695.x
Mouillot D., Graham N. A., Villéger S., Mason N. W., Bellwood D. R. (2013). A functional approach reveals community responses to disturbances. Trends Ecol. Evol. 28, 167−177. doi: 10.1016/j.tree.2012.10.004
Nayak G. N., Noronha-D’Mello C. A. (2018). Estuarine mudflat and mangrove sedimentary environments along central west coast of India. SF J. Environ. Earth Sci. 1 (1), 1013.
Nogueira M., Nascimento L. S. D., Maciel P. V., Tilbert S., Oliveira L. D. (2018). “Diversity, species composition and assemblage dynamics of estuarine gelatinous and semi-gelatinous zooplankton from Brazil,” in Plankton ecology of the southwestern Atlantic. Eds. Hoffmeyer M. S., Sabatini M. E., Brandini F. P., Calliari D. L., Santinelli N. H. (Cham: Springer International Publishing), 375−412. doi: 10.1007/978-3-319-77869-3_18
Obertegger U., Manca M. (2011). Response of rotifer functional groups to changing trophic state and crustacean community. J. Limnol. 70, 231−238. doi: 10.3274/JL11-70-2-07
Østergaard P., Munk P., Janekarn V. (2005). Contrasting feeding patterns among species of fish larvae from the tropical Andaman Sea. Mar. Biol. 146, 595−606. doi: 10.1007/s00227-004-1458-8
Parker A. R., Mckenzie D. R., Ahyong S. T. (1998a). A unique form of light reflector and the evolution of signalling in Ovalipes (Crustacea: Decapoda: Portunidae). Proc. R. Soc B 265, 861−867. doi: 10.1098/rspb.1998.0371
Parker A. R., Mckenzie D. R., Large M. C. (1998b). Multilayer reflectors in animals using green and gold beetles as contrasting examples. J. Exp. Biol. 201, 1307−1313. doi: 10.1242/jeb.201.9.1307
Pavoine S., Vallet J., Dufour A. B., Gachet S., Daniel H. (2009). On the challenge of treating various types of variables: application for improving the measurement of functional diversity. Oikos 118 (3), 391−402. doi: 10.1111/j.1600-0706.2008.16668.x
Pennington J. T., Chia F. S. (1984). Morphological and behavioral defenses of trochophore larvae of Sabellaria cementarium (Polychaeta) against four planktonic predators. Biol. Bull. 167, 168−175. doi: 10.2307/1541345
Pomerleau C., Sastri A. R., Beisner B. E. (2015). Evaluation of functional trait diversity for marine zooplankton communities in the northeast subarctic Pacific ocean. J. Plankton Res. 37 (4), 712–726. doi: 10.1093/plankt/fbv045
Price S. A., Friedman S. T., Wainwright P. C. (2015). How predation shaped fish: the impact of fin spines on body form evolution across teleosts. Proc. R. Soc B 282, 20151428. doi: 10.1098/rspb.2015.1428
Ricotta C., Moretti M. (2011). CWM and Rao’s quadratic diversity: a unified framework for functional ecology. Oecologia 167, 181−188. doi: 10.1007/s00442-011-1965-5
Saiz E. (2009). “Swimming dynamics of zooplankton,” in Marine ecology. Eds. Duarte C. M., Lot-Helgueras A. (Paris: UNESCO), 319−338.
Sanvicente-Añorve L., Chiappa-Carrara X., Ocaña-Luna A. (2002). Spatio-temporal variation of ichthyoplankton assemblages in two lagoon systems of the Mexican Caribbean. Bull. Mar. Sci. 70, 19−32.
Sanvicente-Añorve L., Hernández-González J., Lemus-Santana E., Hermoso-Salazar M., Violante-Huerta M. (2021). Population structure and seasonal variability of two luciferid species (Decapoda: Sergestoidea) in the western gulf of Mexico. Diversity 13, 301. doi: 10.3390/d13070301
Sanvicente-Añorve L., Sierra-Zapata S., Lemus-Santana E., Ruiz-Boijseauneau I., Soto-González L. (2020). Feeding activity of Flaccisagitta enflata (Chaetognatha) upon copepods in the southern gulf of Mexico. Cah. Biol. Mar. 61, 1−7. doi: 10.21411/CBM.A.D2D370A8
Sanvicente-Añorve L., Soto L. A., Espinosa-Fuentes M. L., Flores-Coto C. (2006). Relationship patterns between ichthyoplankton and zooplankton: a conceptual model. Hydrobiologia 559, 11−22. doi: 10.1007/s10750-005-1323-x
Suchman C. L., Sullivan B. K. (1998). Vulnerability of the copepod Acartia tonsa to predation by the scyphomedusa Chrysaora quinquecirrha: effect of prey size and behavior. Mar. Biol. 132, 237−245. doi: 10.1007/s002270050389
van der Linden P., Marchini A., Smith C. J., Dolbeth M., Simone L. R. L., Marques J. C., et al. (2017). Functional changes in polychaete and mollusc communities in two tropical estuaries. Estuar. Coast. Shelf Sci. 187, 62−73. doi: 10.1016/j.ecss.2016.12.019
Vega-Pérez L. A., Ara K., Liang T. H., Pedreira M. M. (1996). Feeding of the planktonic shrimp Lucifer faxoni Borradaile 1915 (Crustacea: Decapoda) in the laboratory. Rev. Bras. Oceanogr. 44, 1−8. doi: 10.1590/S1413-77391996000100001
Viitasalo M., Kiørboe T., Flinkman J., Pedersen L. W., Visser A. W. (1998). Predation vulnerability of planktonic copepods: consequences of predator foraging strategies and prey sensory abilities. Mar. Ecol. Prog. Ser. 175, 129−142. doi: 10.3354/meps175129
Villéger S., Mason N. W., Mouillot D. (2008). New multidimensional functional diversity indices for a multifaceted framework in functional ecology. Ecology 89, 2290−2301. doi: 10.1890/07-1206.1
Vinagre C., Costa M. J. (2014). Estuarine-coastal gradient in food-web network structure and properties. Mar. Ecol. Prog. Ser. 503, 11−21. doi: 10.3354/meps10722
Violle C., Navas M. L., Vile D., Kazakou E., Fortunel C., Hummel I., et al. (2007). Let the concept of trait be functional! Oikos 116, 882−892. doi: 10.1111/j.2007.0030-1299.15559.x
Vogt R. J., Peres-Neto P. R., Beisner B. E. (2013). Using functional traits to investigate the determinants of crustacean zooplankton community structure. Oikos 122, 1700−1709. doi: 10.1111/j.1600-0706.2013.00039.x
Waldbusser G. G., Voigt E. P., Bergschneider H., Green M. A., Newell R. I. E. (2011). Biocalcification in the eastern oyster (Crassostrea virginica) in relation to long-term trends in Chesapeake bay pH. Estuar. Coast. 34, 221–231. doi: 10.1007/s12237-010-9307−0
Weiher E. (2011). “A primer of trait and functional diversity,” in Biological diversity: frontiers in measurement and assessment. Eds. Magurran A. E., McGill B. J. (Oxford: Oxford University Press), 175–193. doi: 10.1086/666756
Wilken S., Huisman J., Naus-Wiezer S., Van Donk E. (2013). Mixotrophic organisms become more heterotrophic with rising temperature. Ecol. Lett. 16, 225−233. doi: 10.1111/ele.12033
Keywords: functional diversity, functional traits, zooplankton, feeding strategies, trophic groups, camouflage, buoyancy, defense against predators
Citation: Sanvicente-Añorve L, Sánchez-Campos M, Alatorre-Mendieta M, Lemus-Santana E and Guerra-Castro E (2022) Zooplankton functional traits in a tropical estuarine system: Are lower and upper estuaries functionally different? Front. Mar. Sci. 9:1004193. doi: 10.3389/fmars.2022.1004193
Received: 27 July 2022; Accepted: 18 November 2022;
Published: 01 December 2022.
Edited by:
Alberto Basset, University of Salento, ItalyReviewed by:
Akash R. Sastri, Fisheries and Oceans Canada (DFO), CanadaPablo Muniz, Universidad de la República, Uruguay
Copyright © 2022 Sanvicente-Añorve, Sánchez-Campos, Alatorre-Mendieta, Lemus-Santana and Guerra-Castro. This is an open-access article distributed under the terms of the Creative Commons Attribution License (CC BY). The use, distribution or reproduction in other forums is permitted, provided the original author(s) and the copyright owner(s) are credited and that the original publication in this journal is cited, in accordance with accepted academic practice. No use, distribution or reproduction is permitted which does not comply with these terms.
*Correspondence: Laura Sanvicente-Añorve, lesa@unam.mx