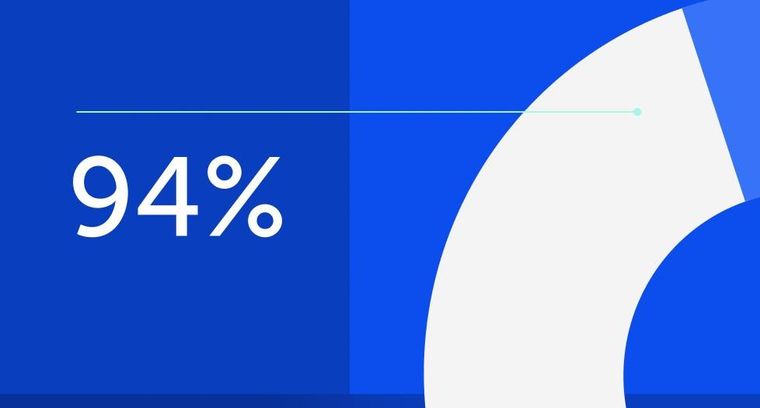
94% of researchers rate our articles as excellent or good
Learn more about the work of our research integrity team to safeguard the quality of each article we publish.
Find out more
ORIGINAL RESEARCH article
Front. Mar. Sci., 01 December 2022
Sec. Coral Reef Research
Volume 9 - 2022 | https://doi.org/10.3389/fmars.2022.1002924
This article is part of the Research TopicInnovative Approaches to Coral Reef Science by Early Career ResearchersView all 15 articles
Behavioral responses are considered sensitive and effective indicators of organism stress. As the demand for standardized coral toxicity tests grows, innovative tools that allow for automatic and quantitative measurements of these behaviors may complement ecotoxicological studies. The rapid growth of competitive marine algae in aquaculture systems is a major issue for generating coral spat for reef restoration, and the application of non-toxic antifouling (AF) coatings might effectively mitigate this issue. While these coatings do not appear to be toxic to sensitive coral larvae, their potential to affect larval mobility has not been tested. In this context, we tested the effect of three recently-developed and potentially non-toxic AF coatings: (i) antiadhesive, (ii) cerium dioxide (CeO2-x) nanoparticle, and (iii) encapsulated biocide dichlorooctylisothiazolinone (DCOIT) on the swimming velocity and activity of Acropora millepora coral larvae for potential use in reef-restoration activities. The behavior of 32 coral larvae per AF treatment were recorded, each for 25 min, in a self-constructed dark box with two camera recording sets in parallel. The tracking analysis was performed with the software Noldus EthoVision XT. The mean larval swimming velocity on control tiles of 93.1 ± 5.6 mm min-1 (and activity of 62.8 ± 5.2%) was nearly 2-fold faster (higher) than on the antiadhesive, (CeO2-x) nanoparticle and DCOIT coatings, respectively. Larvae exposed to the DCOIT-coated tiles remained almost stationary. Although the underlying cause and consequence of these results require further investigation, tracking of coral larval swimming behavior was identified as a reliable and feasible method for assessing potential non-lethal responses to AF coatings. As changes in behavior could have significant consequences for larval survival and settlement, they are important endpoints to consider, and the quantification of behavioral responses may be a meaningful and sensitive tool. Therefore, we recommend the use of behavioral studies for coral larval assessments in ecotoxicology as a valuable endpoint. For methodological standardization and implementation, our study also features a detailed guide for video-processing and track analysis of A. millepora coral larvae in EthoVision.
Tropical coral reefs are under increasing pressure (Gardner et al., 2003; Bruno and Selig, 2007; De’Ath et al., 2012) from climate change as well as increased human activities in coastal areas (Hughes et al., 2010; Pandolfi et al., 2011; Hughes et al., 2017). While heat stress events can cause a rapid decline in coral cover (Cornwall et al., 2021), local impacts to coral reefs can exacerbate coral mortality through multi-stressor events. For instance, antifoulant releases following ship groundings have resulted in some of the most contaminated coral reefs, with TBT (tributyltin) and Cu (copper) being highly potent inhibitors of coral larval settlement and survival, both of which are key processes in reef recovery (Negri et al., 2002). Therefore, the development of safe, non-toxic AF coatings could reduce persistent harm following such accidents.
Coral health and survival can also be affected by competition with other benthic organisms (Hughes et al., 2007; Vermeij and Sandin, 2008; Vermeij et al., 2009), including macroalgae, which can be favored by excess nutrients (Karcher et al., 2020; (Adam et al., 2021). The interaction between corals and fouling organisms, particularly filamentous algae can be detrimental to larval settlement and to the survival and growth of coral recruits and the success of adult corals (Carpenter and Edmunds, 2006; Box and Mumby, 2007; Linares et al., 2012). Competition between rapidly growing algae and newly settled corals in aquaculture represents a problem for coral restoration projects, where maximizing the survival of corals is critical to the feasibility of upscaling restoration towards ecologically meaningful outcomes (Randall et al., 2020). Non-toxic AF applications may prove beneficial for improving survival in coral aquaculture and restoration, an approach being developed to boost reef recovery. The application of a wax AF coating on coral settlement devices to control the benthic community composition around newly settled corals (i.e. ‘spat’) was found to reduce mortality (Tebben et al., 2014), which could increase the likelihood of coral survival to size-escape thresholds and consequently improve the success of sexual coral-propagation techniques (Randall et al., 2020). A recent study also demonstrated the effectiveness of two non-toxic AF coatings (same coatings as in this study) to reduce algal growth on coral settlement surfaces, a first step towards controlling fine-scale competition with benthic organisms in coral aquaculture (Roepke et al., 2022). While these AF coatings did not affect coral larval settlement, further work is required to assess their potential influence on other aspects of larval behavior such as mobility which might represent a sensitive response relevant to the application of AF coatings for shipping and in aquaculture.
Innovative non-toxic AF coatings include silicon-based sol-gel coatings which exhibit antiadhesive surface properties (herein referred to as antiadhesive coating) that prevent the attachment of fouling organisms and facilitate their release at high water velocities. The antiadhesive effect is achieved through low surface energies (with weak molecular attraction), amphiphilic properties (both hydrophilic and hydrophobic properties) and a precisely tuned surface roughness designed to inhibit primary fouling (Detty et al., 2014). Some studies have shown effective AF properties of the biocide dichlorooctylisothiazolinone (DCOIT, Kathon 930, C-9 or DCOI) encapsulated in silica nanocapsules (in this study referred to as DCOIT coating), which decreases the toxicity towards organisms (Maia et al., 2015; Dos Santos et al., 2020). Other recently developed non-biocidal AFs are based on nanoparticles (NPs), which disrupt bacterial cell-to-cell communication (i.e. quorum sensing) to inhibit the formation of biofilms (herein referred to as nanoparticle coating) and mitigate or delay colonization by algae (Korschelt et al., 2018b). One example includes cerium (Ce3+/Ce4+)-modified sites across the high surface area of NPs, which enhance the catalytic oxidation of halides, resulting in the formation of biocidal compounds that combat biofilm formation or the formation of signaling molecules involved in intracellular communication (Herget et al., 2017a; Korschelt et al., 2018a; Herget et al., 2018).
Studies on potential coral stressors and the effects of toxicants have garnered interest in the scientific and public community in recent years as the awareness of threats to tropical coral reef health increases. Over two decades ago, copper toxicity was tested for its inhibitory effect on coral gamete fertilization and larvae settlement (Reichelt-Brushett and Harrison, 2000; Negri and Heyward, 2001). Since then, assays have been used to identify toxicity thresholds for petroleum (Nordborg et al., 2018), AF paint (Negri et al., 2002), pesticides (Ross et al., 2015), and most recently, sunscreens and UV filters (Miller et al., 2021; Moeller et al., 2021; Pawlowski et al., 2021; Miller et al., 2022). However, toxicity assays often differ in methodology and experimental responses or endpoints among studies. For example, some studies have used lethal concentrations (i.e. Kwok and Ang, 2013), while other studies report sublethal endpoints including gene expression biomarkers of heat stress (Louis et al., 2017; Ishibashi et al., 2018), oxidative stress (Cima et al., 2013; Olsen et al., 2013; Marangoni et al., 2019), phototoxicity (Overmans et al., 2018), symbiont density (Cunning and Baker, 2013), bleaching response (Siebeck et al., 2006), and growth (Wijgerde et al., 2020). Standardized tests would enable the validation of coral-toxicity data related to different compounds or pathogens that are known or suspected to negatively affect individual coral species or coral ecosystems. Furthermore, robust and standardized tests, as suggested by a recent study on coral larval settlement and survival responses to UV filters (Miller et al., 2022), could help to predict biochemical, physiological and behavioral tolerance thresholds in corals (Marangoni et al., 2019; Parkinson et al., 2019) and therefore substantially improve risk assessments and help guide coral restoration efforts.
Behavioral changes that exceed the normal range of functional responses including locomotion, habitat selection, feeding, predator avoidance, competition and reproduction, have the potential to reduce fitness and survival of individuals and populations (Bridges, 1997). Behavioral end-points in aquatic ecotoxicology are characterized by short response times, high sensitivity, and are usually non-invasive, allowing for repeated measurements and time-dependent data collection (Gerhardt, 2007). For example, reductions in swimming and feeding behavior can be more sensitive than biochemical or whole organism responses (Harayashiki et al., 2016; Harayashiki et al., 2018). These endpoints may be the first detectable responses of an organism to environmental perturbations, suggesting that they could be highly valuable early indicators of stress (Reichelt-Brushett and Harrison, 2004; Bertram et al., 2022). Yet, behavioral ecotoxicology has received less attention due to a lack of user-friendly tools for quantitative data acquisition (Faimali et al., 2017). As a result, little is known about behavioral stress responses in cnidarians (Ianna et al., 2020), and most studies to date have been based on tedious manual analysis of observational data such as manually tracing swimming pathways and image analysis (Reichelt-Brushett and Harrison, 2004; Kwok and Ang, 2013; Antonio-Martínez et al., 2020; Ianna et al., 2020). Some more advanced approaches have been applied to tracking coral larvae movement including the reconstruction from film using Matlab Image Processing toolbox (Martínez-Quintana et al. (2015)). However, the recent development of inexpensive and more widely available technology has recently renewed interest in quantifying behavior and investigating relationships between behavioral and physiological activities in aquatic organisms (Faimali et al., 2017). The application of more user-friendly automatic image acquisition systems should improve the speed and reliability of accurately measuring and quantifying behavioral end-points for application in ecotoxicology (Kane et al., 2005; Bertram et al., 2022).
The software EthoVision XT (Noldus Information Technology) has been used in many aquatic behavioral studies, especially with zebrafish larvae (Groneberg et al., 2015; Tudorache et al., 2015; Thia et al., 2020). In this study, EthoVision XT was used for the first time to assess the behavior of coral larvae, including the quantification of swimming activity and velocity, in response to three recently-developed and potentially non-toxic AF coatings (antiadhesive, cerium dioxide nanoparticles, and encapsulated DCOIT). Our objectives were to (1) explore the swimming behavior of the coral larvae in response to the AF coating treatments, and (2) test the feasibility of the experimental setup, including its adaptation for coral larvae, with the software EthoVision XT. This behavioral study aimed to contribute to the suite of sub-lethal ecotoxicological protocols available to identify more universal and sensitive responses to environmental challenges for coral larvae.
Three different AF coating variants were prepared on 4 x 4 cm poly (methyl methacrylate) (PMMA) tiles, a common material used in aquaculture: (i) antiadhesive coating, (ii) DCOIT coating, and (iii) CeO2 nanoparticle coating. To achieve good coating application, all tiles were cleaned by rinsing with ethanol followed by a surface activation using microwave plasma (Creaetch 250 Plasma MV, working pressure 10 Pa in air, activation time 2.5 min., Creavac GmbH), prior to coating. PMMA was selected as an inert and transparent substrate to allow tracking of larvae, having the advantage over glass of better AF adhesion.
At low surface energies in the range of 20-25 mN/m, organisms usually form poor attachments to the surface, so removal by incident flow is often possible (Detty et al., 2014). To generate low surface energy coatings, a SiO2 coating solution (‘sol’) based on modified silicon alcoholates was prepared following the work of Sokolova et al. (2012) and Detty et al. (2014). For sol preparation, 0.675 g n-octadecyl-trimethoxysilane (CAS 3069-42-9, abcr GmbH, Karlsruhe, Germany), 3.675 g tridecafluoro-1,1,2,2-tetrahydrooctyltriethoxysilane (CAS 51851-37-7, abcr GmbH, Karlsruhe, Germany), 22.4 g n-octyltriethoxysilane (CAS 2943-75-1, abcr GmbH, Karlsruhe, Germany), and 18.75 g tetraethylorthosilicate (TEOS, CAS 78-10-4 abcr GmbH, Karlsruhe) were mixed with 59.5 mL of ethanol and 11.4 mL of HCl (0.1 M) and stirred for 24 h at room temperature for hydrolysis and sol formation. Coatings were applied by dip-coating, dried under ambient conditions and then cured at 100°C for one hour. The final coating on the PMMA tiles was as translucent as the control tiles without any coating.
Hydrophobic pyrogenic silicic acid powder (0.1 g) was mixed with a solution of 0.5 g of dichlorooctylisothiazolinone (DCOIT; C11H17Cl2NOS; TCI America; CAS RN 64359-81-5; product number D4157) in 2 mL of ethanol. The mixture was then left overnight to evaporate the ethanol. Two different sols were used in sequence to deposit the DCOIT loaded-silicic-acid powder on the PMMA tiles. First, 20 mL of a hydrophilic sol made from TEOS and 3-glycidyloxypropyl-triethoxysilane (9 mL TEOS, 1 mL 3-glycidyloxypropyl-triethoxysilane, 60 mL H2O and 30 mL 0.01M HCl) were intensively mixed by sonication (UP100H, Hielscher Ultrasonics GmbH, Teltow, Germany) with 0.1 g of the DCOIT loaded powder. The mixture was applied to the PMMA tiles by dip-coating, dried and solidified by annealing at 100°C for one hour. To achieve slower DCOIT release from the coatings, a second layer using a hydrophobic sol (as described in 2.1.1.) was applied on top. As for the primary coating, 0.1 g DCOIT loaded powder was mixed with 20 mL of the hydrophobic sol shortly before coating. To estimate the extractable DCOIT content from the coating, ethanol was used for extraction. Photometrically, a DCOIT content of 3.2 mg cm-2 was measured. The final coating was similarly translucent to the control and antiadhesive coated tiles.
Cerium oxide nanoparticles (NPs) were manufactured according to Ji et al. (2012) and the ‘Supporting Information’ in Herget et al. (2017b). In short, 100 mL of a 9.0 M NaOH solution was poured into 20 mL of a rapidly stirred 0.05 M solution of cerium(III) nitrate hexahydrate. After stirring for 30 min, the suspension was transferred into a stainless steel autoclave with a Teflon insert and heated to 100°C for 24 hours. The lilac product was separated from the turbid suspension by centrifugation (3000 rpm, 10 min) and washed two times with MilliQ-water. After drying at 60°C overnight, the resulting yellow product was ground in a vibrating ball mill for two minutes at 30 rpm. The milling yielded agglomerates of the size< 20 µm. Before adding the cerium oxide powder to the coating solutions, the powder was dispersed in water and a pH of about 7.5 was adjusted. Coatings were prepared using two different sols. Coating solution 1: 100 mL TEOS, 420 mL ethanol (96%) and 20 mL 0.01 M HCl were mixed and stirred overnight at room temperature for hydrolysis. An acidic silica nanosol with a solids content of about 6 wt% was formed. Coating solution 2: 75g TEOS, 25 g 3-glycidyloxypropyl-triethoxysilane, 420 mL ethanol (96%) and 20 mL 0.01 M HCl were mixed and stirred overnight at room temperature for hydrolysis. The solids content of the sol was also about 6 wt.%. The PMMA tiles were coated 3 times. 1st coat: dip coating of the tiles by using a mixture of 1 g cerium oxide powder dispersed within 30 mL of coating solution 1. 2nd and 3rd coat: coating application by felt using 1 g cerium oxide dispersed within 30 mL of coating solution 2. The combination of an initial dip coating using a SiO2-sol with cerium oxide and the subsequent overcoats using an epoxy-modified sol with cerium oxide gave good adhesion and uniformity of the coatings. Test coatings showed a coating weight of 0.9 mg cm-2. The amount of ceria NPs in the coatings solutions was 35.7 wt%. In total, approximately 0.32 mg of ceria NPs were embedded per cm2. The final coating on the tiles was slightly opaque compared with the other coatings and control.
Gravid colonies (25–40 cm diameter) of the scleractinian coral Acropora millepora (Ehrenberg, 1834) were collected from Falcon Island (18° 45.796’S 146° 31.926’E; 2 m depth) in November 2019 under permit G12/35236.1, issued by the Great Barrier Reef Marine Park Authority to the Australian Institute of Marine Science (AIMS). Colonies were transported to the National Sea Simulator (SeaSim) at AIMS and maintained at ambient temperature in outdoor 1700 L flow-through holding tanks until spawning. On 15th November 2019, spawning occurred and the coral gametes from eight A. millepora parental colonies were collected and fertilized en masse. Symbiont-free larval cultures were maintained at densities < 500 larvae per L, indoors, in flow-through rearing tanks supplied with 0.4 μm filtered natural seawater at 27.0 ± 0.5°C (as described in Negri and Heyward, 2000; Nordborg et al., 2018). Flow-through seawater (1.5 turnovers per day) and an air stone ring at the base of each tank provided saturating aeration and created a gentle curtain of bubbles to keep larvae away from a submerged cylindrical mesh filter (100 µm) at the outflow. The average water quality parameters during larval culture were (range): pH 8.06 (8.01-8.13), salinity 35.9 (35.4-36.4), NH4 0.19 (0.13-0.27) µmol/L, PO4 0.08 (0.06-0.11) µmol/L, NO3 1.80 (1.25-2.93) µmol/L, SiO2 2.91 (2.44-4.31) µmol/L, alkalinity 2291 (2267-2321) µmol/Kg and dissolved inorganic carbon 2010 (1984-2052) µmol/Kg. Each day, a sub-sample of larvae in the rearing tanks was tested for settlement competency on 3D-printed polylactic acid (PLA) discs. 13 days after fertilization, larvae were found competent to settle and the experiment commenced. Immediately prior to each filming session (total of 8 sessions on the same day), new larvae from the same batch and rearing tank were collected.
To investigate the effects of the AF coatings on larval behavior, coral larvae were filmed while moving freely on top of AF coated (antiadhesive, nanoparticle, DCOIT) and uncoated (control) PMMA tiles (approx. 4 x 4 cm) in a laboratory experiment. Prior to the experiment, all tiles were rinsed with fresh 1-μm filtered natural seawater to avoid any particle contamination on the surfaces. Silicone sealing rings with an inner diameter of 15 mm were then placed on top of the PMMA tiles and filled with fresh 650 μL of 1-μm filtered natural seawater, creating small “pools” that kept the larvae on the tiles above the AF coatings (Figure 1). The silicone rings self-sealed against the PMMA tiles. Room temperature was kept at 28.5°C which maintained water temperature in the pools at a stable 27.0°C throughout the experiment. Two silicone rings, each holding a single 13-day-old larva, were placed on each tile. Shortly prior to each filming session, larvae were gently pipetted into the previously filled pools with as little excess water from the pipette. Four tiles, one for each treatment, were arranged to be filmed at the same time by one camera (Figure 1; Olympus TG-5). Each filming session included two sets of 4 tiles (1 tile per treatment), each filmed by a single camera. This totaled two cameras and 8 tiles per session (and 2 tiles per treatment per session) (Figure 2). Tiles were coated on both sides and each side was used in a single trial (16 tiles per treatment). By conducting eight filming sessions of 28 minutes each, the tracks of 32 larvae were recorded for each treatment. After each filming session, silicone rings were rinsed with filtered seawater and applied on top of new tiles from the same treatment. New larvae from the rearing tank were introduced to fresh filtered seawater in each test. To prevent light reflections on the water surface and to increase the contrast between the larvae and the background for filming, the translucent tiles were placed inside a dark box on top of a light table. The light table was made from translucent white diffusive plastic and was lit from below by two LED light bars (ARLEC Model UC450, 4W, Cool White), providing equal lighting for all tiles (Figure 2). To provide an even surface for the tiles, a thick (2 cm) transparent PMMA block was placed on top of the light table. Both cameras were placed at an equal distance above the tiles (top-down view) and optical camera zoom was used to frame the tiles in the videos, ensuring no distortion around the edges. Recordings of both cameras were started simultaneously. The cameras were set to record at a resolution of 1920x1080 pixels (1080p/Full HD) and 25 frames per second. The recordings stopped automatically after 28 minutes when a file size of about four gigabytes was reached (limit of FAT32 formatted SD cards).
Figure 1 Tile setup beneath one camera. Actual image (A) of one tile setup (here: DCOIT tile). Schematic diagram (B) with coral larvae (a), silicone sealing ring (b), PMMA tile (c).
Figure 2 Filming setup including both cameras (a), light bars (b), light table (c), PMMA block (d), both tile setups (e) and the dark box (f). The 4 tile colors notionally represent tiles of each of the 4 treatment types (control, DCOIT, antiadhesive, and nanoparticle).
The video editing program XMedia Recode (version 3.4.8.3) (Dörfler, 2019) was used for post-production of the video files. The first two and the last one minute of each video were cut to acquire clips with a duration of 25 minutes. This procedure ensured a steady video quality by eliminating possible effects from camera handling and allowed the larvae a couple minutes of acclimation. Based on literature (Faimali et al., 2017), and given the lack of information for coral larval swimming video-tracking analyses, 25 minutes of behavior observations was selected, allowing substantial observation durations and multiple tests with larvae of the same age. Before track-analysis, the video files were post-processed to enhance the contrast between the larvae and the background (see Supplementary Manual on GitHub for details). Subsequently, videos were converted from the original format (.mov) to .avi video-container-format for further application in the tracking software. EthoVision® XT (version 10.1.856; Noldus, Wageningen, Netherlands; Spink et al., 2001) was used to analyze larval behavior. The software generated data (every tenth of a second for each individual larva) of the distance moved (in mm) and the swimming activity (moving/not moving). “Minimal distance moved” in the EthoVision software’s “Track Smoothing Profiles” tab was set to record track changes only when the larvae moved more than 0.25 mm (direct distance; see Supplementary Manual on GitHub). The velocity of each larva while moving was calculated by dividing the total distance a larva travelled by the duration of time spent moving during the trial (as measured by EthoVision). The threshold velocity of the larvae considered “moving” was set to 0.033 mm s-1 (~1.98 mm min-1), whereas the threshold for “not moving” was a velocity below 0.02 mm s-1 (~1.21 mm min-1). If a larva travelled more than 1.98 mm min-1 initially, but lost speed below this threshold, “moving” was still detected. Below 1.21 mm min-1, however, no movement was measured. These settings suppressed noise by ensuring recordings of actual larval movements and minimizing “jitter of detail” video effects, that could have biased the data.
All acquired tracks were checked, and where necessary, corrected manually to ensure high data accuracy (see Supplementary Manual on GitHub here: https://github.com/drefeld/CoralLarvaeTracking.git). EthoVision XT created result summaries of the trial statistics (raw data for the calculation of the velocity while moving) and group statistics, which were transferred to Microsoft Excel 2019.
All statistical analyses were performed in R version 4.1.1 (R Core Team, 2021) and the data were manipulated and visualized using packages of the ‘tidyverse’ (Wickham et al., 2019) and ‘car’ (Fox and Weisberg, 2019). To investigate differences in larval swimming velocity and activity amongst the AF treatments, linear mixed effects models (LMM) were fitted using the package ‘nlme’ (Pinheiro et al., 2021).
Swimming velocity while moving , and swimming activity (time moving (in percent)) were used as response variables. The swimming activity was log-transformed (after adding a small constant), and the swimming velocity was square-root transformed to stabilize heteroscedasticity in the residuals. Treatment was always included as the sole fixed effect. Weights were adjusted to allow for unequal variances among treatments.
The filming “session” was used as a proxy for time of day and included in each model as a random effect to account for possible variation due to diurnal patterns of larval behavior. Likelihood-ratio tests between the LMMs with “session” and baseline generalized least squares (GLS) models without random effects revealed that including the variable “session” significantly improved model performance in all cases (p< 0.05). Adding the “position” of the rings on the coated tiles (inner/outer corner of tile) as a random effect did not significantly improve model fit and was therefore omitted from all models.
Restricted maximum likelihood (REML) estimation was used for all models and statistical significance of the treatment effect was assessed using the ‘anova’ function included in ‘nlme’ (Pinheiro et al., 2021). The model was diagnosed and the satisfaction of model assumptions was checked using standard R model diagnostic plots with normalized residuals. Pairwise post-hoc tests based on estimated marginal means were run with the package ‘emmeans’ (Lenth, 2021) to find differences between each treatment pair. The p-value was adjusted for multiple testing with the Tukey method. Tests were performed on the log-/sqrt-scale and back-transformed for visualization. (Figures 3, 4). An RMarkdown (.Rmd) script that shows the analysis of the track data in RStudio, can be downloaded online on GitHub: https://github.com/drefeld/CoralLarvaeTracking.git.
Figure 3 Average larval swimming velocity (mm min-1) in each treatment and control (n = 32 larvae per treatment). Numbers below bars indicate mean velocity ± SE in the corresponding treatment. Error bars represent SEM. Asterisks indicate statistically significant differences based on pairwise post-hoc tests with estimated marginal means (Supplementary Tables 1, 3; *p< 0.05, **p< 0.01, ***p< 0.001).
Figure 4 Average larval swimming activity (moving/not moving; in %) in each treatment and control (n=32), as indicated by the percentages (rounded) in the bars. Asterisks indicate statistically significant differences based on pairwise post-hoc tests with estimated marginal means (Supplementary Tables 2, 4; *p< 0.05, **p< 0.01, ***p< 0.001).
Larval swimming velocity differed among AF treatments (LMM: χ2(3) = 94.7, p< 0.001). With a mean velocity of 93.1 ± 5.6 mm min-1 (mean ± SE; Table 1), A. millepora larvae moved significantly faster on the control tiles than on all the AF tiles: larvae on the antiadhesive-coated tiles averaged 58 ± 5 mm min-1 (p< 0.001), while the nanoparticle-coated tiles averaged 48.1 ± 3.8 mm min-1 (p< 0.001) and the DCOIT-coated tiles averaged 37.7 ± 1.5 mm min-1 (p< 0.001). Larval swimming velocity did not differ between the antiadhesive-coated and nanoparticle-coated tiles (p = 0.278). Velocity was lower on the DCOIT-coated tiles than on the antiadhesive- (p< 0.001) and nanoparticle-coated tiles (p = 0.034) (Figure 3; Table 1). Pairwise statistical results of swimming velocity data can be viewed in the Supplementary Table 3.
Table 1 Mean swimming velocity (mm min-1) and activity (%; moving) ± SE (standard error), velocity ranges (mm min-1; min. – max.) and mean distance travelled (mm) ± SE of Acropora millepora larvae per treatment (Control, Nanoparticles, Antiadhesive, DCOIT) on the coated PMMA tiles.
Larval activity (time spent moving vs. not moving) also significantly differed among AF treatments (LMM: χ2(3) = 285.43, p< 0.001), and followed a similar pattern to swimming velocity. The larvae on the control tiles spent the majority of their time swimming (moving) (62.8 ± 5.2% (mean ± SE); Table 1), and moved significantly more than on the antiadhesive-coated tiles (22.9 ± 5.2%; p< 0.001), the nanoparticle-coated tiles (14.1 ± 3.4%; p< 0.001) and the DCOIT-coated tiles (p< 0.001) (Figure 4; Table 1). On the DCOIT-coated tiles, larvae were predominantly still, moving an average of only 1.5 ± 0.4% of the trial duration (Table 1). Pairwise statistical results of swimming activity data are available in the Supplementary Table 4.
The effects of innovative AF coatings on marine species, especially corals, have been rarely evaluated since their introduction after 2008, when TBT was globally prohibited (Dafforn et al., 2011). Here, three coatings with reportedly high AF efficacy and low potential toxicity (Detty et al., 2014; Maia et al., 2015; Herget et al., 2017a) were tested on the swimming activity and velocity of Acropora millepora coral larvae. In all three antifouling treatments, larvae moved significantly slower and in general less often than in the control treatment. As the methodology of track-analysis with the software EthoVision XT is well-established for other aquatic organisms (i.e. zebrafish and amphipods), it was an additional goal to test the reliability and feasibility of this method with coral larvae. A comprehensive guide for video-processing and analysis is provided (see Supplementary Manual on GitHub (https://github.com/drefeld/CoralLarvaeTracking.git)). Our results document the potential of this method to identify behavioral effects in coral larvae, although the underlying cause of the changes to swimming behavior requires further investigation.
Like planulae of most coral species (Harrison and Wallace, 1990), A. millepora larvae are very active swimmers and exhibited typical straight, curved and spiraled swimming patterns with peridic stopping on the PMMA. The significantly higher motility of the larvae in the control treatment could be explained by the relatively smooth PMMA surface properties, which likely provided a featureless environment for the larvae who were consequently less likely to stop and explore; these smooth tiles did not offer any potentially attractive cues for settlement, nor was there any microtopography available. Therefore, larvae maintained ‘normal’ or ‘typical’ swimming and searching behavior in the absence of chemical and physical settlement cues. In this context, the high motility observed in the control could be considered ‘normal’ and indicative of active larval swimming, in the absence of settlement cues. It was not possible for the technique to distinguish between slow swimming and ‘crawling’ behavior. Crawling, which implies a slow swimming speed behavior with random swimming stops in between would have been tracked as “not moving” once the velocity of the larva was below 1.21 mm min-1 (to eliminate jitter/noise). However, if a larvae travelled slowly or crawled for a substantial period, this would be captured in the mean velocity metric and activity data of the larvae in the treatments. Future studies could test for changes to normal swimming behavior in response to AF treatments on other biologically inert materials.
By contrast, all the AF treatments tested caused a reduction in swimming speed and larval activity in comparison to the PMMA control tiles. In the absence of an inductive cue, reduced motility could be explained by either toxic action of the antifoulant ingredient (DCOIT, CeO2), other biochemical interactions of the coatings with the larvae, or characteristics of the AF coating surface properties that confuse larval behavior, resulting in cessation of searching, or both (Negri et al., 2002), either of which could ultimately result in recruitment failure. However, further research is needed to characterize ‘normal’ behavior in the presence of settlement cues, which would also likely reduce swimming speed and activity, as the larvae probe the substrate, attach and metamorphose (Randall et al., 2020). Indeed, this expected reduction in swimming activity in response to settlement cues could be similar to the swimming speeds and activity seen in some AF treatments. Therefore, normal swimming behavior on different materials in the presence and absence of settlement cues requires further characterization.
The antiadhesive coating showed a much milder effect on the motility of the larvae than the DCOIT-coating. Sol-gel does not contain a biocide, so the inhibition of swimming is likely to be physical, and the reduced motility of the larvae compared with the control could be a result of different surface properties. This coating type is characterized by an amphiphilic surface with both hydrophilic and hydrophobic properties and interferes with fouling in multiple ways. For example, diatoms bind through hydrophilic proteins, while barnacles bind through hydrophobic adhesive proteins (Finlay et al., 2010); hence, amphiphilic coatings can either reduce or amplify bioadhesion and protein adsorption (Detty et al., 2014). Reduced motility of the A. millepora larvae may have been due to recognition of surface properties attractive for settlement (although no attachment or metamorphosis occurred in the short timeframe of the assays), or unlikely and unknown toxic effects of the sol-gel coating onto the larvae. Any disadvantageous surface properties of the coating and any unlikely potential for toxicity should be investigated further by assessing the survival of recruits on, and adjacent to, the sol-gel.
The rationale behind the encapsulation of DCOIT in the coating is the reduction of direct biocidal interactions with coating components, the control of leaching rate, and thus, a decrease in the absolute quantity of biocide needed to prepare a formulation with identical AF efficacy. Ultimately, the lifetime of the coating is increased and potential environmental threats are reduced (Maia et al., 2012; Maia et al., 2015; Martins et al., 2017; Gutner-Hoch et al., 2018; Figueiredo et al., 2020). However, the strong effect of this treatment on larval behavior indicates that this coating may cause stress, although the mechanism underpinning this behavioral change is unknown. Future studies could address the potential toxicity of this coating with different biocide concentrations (incl. EC50 values) on physiological parameters (e.g. respiration) coupled with biochemical markers (e.g. enzymatic oxidative stress). When a toxicant is present, it requires energy to metabolize and counteract, which could take energy away from other functions. In order to reduce energy expenditure, a larva may reduce its swimming velocity and overall activity, and try to settle and metamorphose into a recruit as soon as possible (Kwok et al., 2016). However, DCOIT can also damage cellular structures and functions as it diffuses easily through membranes and cell walls and can also cause a blockage of the antioxidant defense system, leading to oxidative stress (Eom et al., 2019). Campos et al. (2022) found oxidative stress, lipid peroxidation, damaged lysosomal membranes and increased histopathological pathologies in the neotropical oyster Crassostrea brasiliana in response to DCOIT at environmentally relevant concentrations. Negative effects of DCOIT in bivalves (Fonseca et al., 2020), including decreased activity of GST (glutathione S-transferase), SOD (superoxide dismutase) and CAT (catalase) in the gills and digestive gland have also been reported (da Silva et al., 2021). To date, very few studies have assessed the effects of encapsulated DCOIT in tropical marine species (Dos Santos et al., 2020; de Campos et al., 2022). Only one study by Ferreira et al. (2021) compared the effects of free (non-integrated in a coating) and encapsulated DCOIT on a soft coral, Sarcophyton glaucum. The authors found reduced toxicity of encapsulated DCOIT on the coral fragments compared with free DCOIT, as measured by coral polyp retraction, reduced photosynthetic efficacy and the elevated production of oxidative-stress markers (Ferreira et al., 2021). Relative to the negative control, encapsulated DCOIT still caused some toxicity. The extreme and significant reduction of A. millepora larval motility (velocity and activity) observed here is most likely driven by the DCOIT’s toxicity, rather than the surface roughness. Ultimately, a potential consequence of substantially reduced velocity and activity could be a reduction in larval dispersal and the disruption of the coral’s life cycle by limiting a larva’s ability to seek a suitable habitat for settlement. This toxicity-based hypothesis could be tested with control experiments, in which larvae have no direct contact with the coating, and experiments that test the same surface coating (texture and composition) but without DCOIT incorporation.
The CeO2 nanoparticle’s antifouling property is created by the enzymatic activity of the nanoparticles which catalyze the oxidation of halides in presence of hydrogen peroxide (H2O2) to hypohalous acids which can subsequently react to halogenated organic compounds. Hypohalous acids combat biofilm formation through their biocidal activity or by disrupting the formation of signaling molecules involved in intracellular communication (Herget et al., 2017a; Herget et al., 2018). In our study, the significant reduction of velocity and activity due to the nanoparticle-coated PMMA, reveals an effect of either the amphiphilic surface properties of the sol-gel coating (as suggested for the antiadhesive coating), the surface structure/porosity with incorporated CeO2 nanoparticles (NPs), the oxidative properties of the nanoparticles, or some combination thereof. The effects of CeO2 nanoparticles have not been previously tested on corals; however, recent research suggests that other nanoparticles used in cosmetics can have negative effects. For example, Tang et al. (2017) found ZnO nanoparticles (common in sunscreens) to affect lipid profiles in Seriatopora caliendrum through glycerophosphocholine (GPC) profiling and concluded a mechanical disturbance due to the presence of ZnO nanoparticles. Corinaldesi et al. (2018) reported that uncoated ZnO nanoparticles induced severe coral bleaching, while two coated forms of TiO2 nanoparticles had no negative effect on Acropora spp. over a 48h exposure. Another study (Fel et al., 2019), found a negative impact of ZnO on the photosystem II of the algal symbionts (the functionality was reduced by 38% compared to the controls). However, as only a few studies are available, and methodologies differ, and assumptions and hypotheses need to be further evaluated, these results should be treated cautiously (Mitchelmore et al., 2021; Moeller et al., 2021; Miller et al., 2022).
We tested the catalytic activity of the NPs in their unconsolidated form and assumed their activity in the coating would still be present. Thus, the exact catalytic activity of the coating remains unclear and conclusions concerning its effect on larval motility require validation. We recommend testing the activity of NPs prior to incorporating them into the coating in future experiments to disentangle whether the chemical effect of the NPs plays a role. However, nothing is known of the effects or the mechanism of action of CeO2 nanoparticles on corals. Our study also did not include an inert particle/surface control (for the encapsulated DCOIT particles and CeO2 nanoparticles) and observed effects are therefore difficult to attribute to either physical coating effects or chemical effects.
In addition to our identification of two efficient antifouling coatings with robust levels of coral settlement in Roepke et al. (2022), we propose future studies on settlement, survival and growth on these antifouling coatings, as well as behavioral studies and measures of biochemical markers in response to these coatings. Approaches to settle larvae directly onto restoration deployment substrates adjacent to, or surrounded by these coatings, may offer protection against overgrowth by algae.
Compared with past studies of coral larval swimming behavior (Faimali et al., 2017), EthoVision XT provides useful and user-friendly tools to quantify behavior. An important consideration in adopting this software package, however, is the license cost, and alternative freeware applications, such as trackR (Garnier), pathtrackr in R (Harmer and Thomas, 2019) or idtracker.ai in Python (Romero-Ferrero et al., 2019) may also be suitable and warrant testing. A comparison of these methodologies could identify the most affordable and promising video-tracking methodology for standardization with coral larvae. EthoVision is able to process longer videos, without the need to subsample files or investigate still images, providing a more holistic representation of the observed behavior. Here, “session” as a random factor was found to improve model performance, which suggests that diurnal patterns of the larvae could have affected the measurements. Therefore, we recommend several recording setups in parallel to cover high replicate measurements during small time intervals. While it requires time to troubleshoot and correct the tracking parameters for each video file, the program allows for high throughput, complex data collection and reproducibility. Therefore, EthoVision XT and other similar programs should be considered to complement existing physiological studies and expand the field of behavioral ecotoxicology in marine organisms. With software options that allow diverse experimental setups for different species, automated tracking could represent a powerful tool to detect early signs of stress, providing scientists with standardized techniques for application across taxa to identify “universal responses” to environmental challenges. We recommend the use of cameras with fast loading rechargeable batteries to ensure a steady, smooth recording process. Also, cameras with AC energy supplies and parallel recording mode could be even more suitable than those used here. Finally, tethered recordings that feed to direct computer storage could also speed-up recording and analysis processes.
Results from our behavioral ecotoxicological study of Acropora millepora larvae show that all antifoulant treatments significantly affected the swimming velocity and activity of larvae, albeit to a different degree. By reducing the swimming velocity and activity of the larvae to almost zero, the DCOIT treatment showed the strongest effect. The swimming velocities and activities of larvae exposed to the antiadhesive- and nanoparticle-coated tiles were similar, and were clearly reduced compared to the control. The results suggest three alternative interpretations of the coatings’ effect on larval motility: the physical surface topographies of the coatings could have caused the larvae to move more slowly, the antifouling treatments interacted biochemically with the larvae, or the coatings had a potentially toxic effect on the larvae, or some combination of the effects. Before these coatings are applied in coral aquaculture or restoration, further information on their antifouling efficacy and effects on settlement (Roepke et al., 2022), survival and growth are necessary. Moreover, it is suggested to test the chemical stability and AF activity duration of the coatings on different materials over different time scales, under ex situ and in situ conditions, and on different coral species and developmental stages (gametes, early and late larvae, spat, recruits, adults).
The sensitivity of automated behavioral data collection was demonstrated with this movement tracking method and setup and we recommend its application in future studies. Our study encompasses a guided manual for the use of the software EthoVision with coral larvae, providing scientists with a powerful tool to detect the first indication of stress in early coral life stages. The method can be used to provide a benchmark for larval behavior under normal conditions, and to rapidly and reliably quantify deviations in swimming behavior due to chemical or physical challenges. Moreover, swimming velocity and activity represent ecologically relevant parameters to assess effects of contaminants at environmental concentrations that do not cause mortality. The results should be verified by more studies on the same antifouling coatings with particle controls, as well as other environmentally relevant toxic compounds, and across coral species.
The track analysis manual, a RMarkdown (.Rmd) script of the track data, a knitted HTML version of the RMarkdown including all results and figures, and supplementary tables can be found on GitHub (https://github.com/drefeld/CoralLarvaeTracking.git). The raw data supporting the conclusions of this article will be made available by the authors, without undue reservation.
LR conceptualized and coordinated the study, with guidance provided by DB, US, CR, AN, and AK; LR and DB performed the experiments, analyzed the data and prepared figures/tables; LR wrote the manuscript; CR and AN substantively revised the manuscript. All authors contributed to the article and approved the submitted version.
This work was partially funded by the Australian Institute of Marine Science, the University of Bremen and graduate school GLOMAR, the AiF (German Federation of Industrial Research Associations) and the DSM (German Foundation Marine Conservation) and was supported by the Reef Restoration and Adaptation Program, which is funded by a partnership between the Australian Government’s Reef Trust and the Great Barrier Reef Foundation.
The authors would like to thank Constanze von Waldthausen for her technical support at ZMT, and Florita Flores and Peter Thomas-Hall for their guidance and lab support at AIMS. The authors also thank the staff at the National Sea Simulator at AIMS for assistance with experimental setup and field collections. We acknowledge the Bindal People as the Traditional Owners where this work took place. We pay our respects to their Elders past, present and emerging and we acknowledge their continuing spiritual connection to their land and sea country.
The authors declare that the research was conducted in the absence of any commercial or financial relationships that could be construed as a potential conflict of interest.
The reviewer IM declared a shared affiliation with the authors CR and AN to the handling editor at the time of review.
All claims expressed in this article are solely those of the authors and do not necessarily represent those of their affiliated organizations, or those of the publisher, the editors and the reviewers. Any product that may be evaluated in this article, or claim that may be made by its manufacturer, is not guaranteed or endorsed by the publisher.
The Supplementary Material for this article can be found online at: https://github.com/drefeld/CoralLarvaeTracking.git
Adam T. C., Burkepile D. E., Holbrook S. J., Carpenter R. C., Claudet J., Loiseau C., et al. (2021). . Landscape-scale patterns of nutrient enrichment in a coral reef ecosystem: implications for coral to algae phase shifts. Ecol. Applications 31 (1), e02227. doi: 10.1002/eap.2227
Antonio-Martínez F., Henaut Y., Vega-Zepeda A., Cerón-Flores A. I., Raigoza-Figueras R., Cetz-Navarro N. P., et al. (2020). Leachate effects of pelagic sargassum spp. on larval swimming behavior of the coral acropora palmata. Sci. Rep. 10, 1–13. doi: 10.1038/s41598-020-60864-z
Bertram M. G., Martin J. M., McCallum E. S., Alton L. A., Brand J. A., Brooks B. W., et al. (2022). Frontiers in quantifying wildlife behavioural responses to chemical pollution. Biol. Rev. 46, 1346–1364. doi: 10.1111/brv.12844
Box S. J., Mumby P. J. (2007). Effect of macroalgal competition on growth and survival of juvenile Caribbean corals. Mar. Ecol. Prog. Ser. 342, 139–149. doi: 10.3354/meps342139
Bridges C. M. (1997). Tadpole swimming performance and activity affected by acute exposure to sublethal levels of carbaryl. Environ. Toxicol. Chem. 16, 1935–1939. doi: 10.1002/etc.5620160924
Bruno J. F., Selig E. R. (2007). Regional decline of coral cover in the indo-pacific: Timing, extent, and subregional comparisons. PloS One 2, e711. doi: 10.1371/journal.pone.0000711
Campos B. G., de Fontes M. K., Gusso-Choueri P. K., Marinsek G. P., Nobre C. R., Moreno B. B., et al. (2022). A preliminary study on multi-level biomarkers response of the tropical oyster crassostrea brasiliana to exposure to the antifouling biocide DCOIT. Mar. Pollut. Bull. 174, 113241. doi: 10.1016/j.marpolbul.2021.113241
Carpenter R. C., Edmunds P. J. (2006). Local and regional scale recovery of diadema promotes recruitment of scleractinian corals. Ecol. Lett. 9, 268–277. doi: 10.1111/j.1461-0248.2005.00866.x
Cima F., Ferrari G., Ferreira N. G. C., Rocha R. J. M., Serôdio J., Loureiro S., et al. (2013). Preliminary evaluation of the toxic effects of the antifouling biocide Sea-nine 211TM in the soft coral sarcophyton cf. glaucum (Octocorallia, alcyonacea) based on PAM fluorometry and biomarkers. Mar. Environ. Res. 83, 16–22. doi: 10.1016/j.marenvres.2012.10.004
Corinaldesi C., Marcellini F., Nepote E., Damiani E., Danovaro R. (2018). Impact of inorganic UV filters contained in sunscreen products on tropical stony corals (Acropora spp.). Sci. Total Environ. 637–638, 1279–1285. doi: 10.1016/j.scitotenv.2018.05.108
Cornwall C. E., Comeau S., Kornder N. A., Perry C. T., van Hooidonk R., DeCarlo T. M., et al. (2021). Global declines in coral reef calcium carbonate production under ocean acidification and warming. Proc. Natl. Acad. Sci. U.S.A. 118, e2015265118. doi: 10.1073/pnas.2015265118
Cunning R., Baker A. C. (2013). Excess algal symbionts increase the susceptibility of reef corals to bleaching. Nat. Clim. Change 3, 259–262. doi: 10.1038/nclimate1711
Dafforn K. A., Lewis J. A., Johnston E. L. (2011). Antifouling strategies: History and regulation, ecological impacts and mitigation. Mar. pollut. Bull. 62, 453–465. doi: 10.1016/j.marpolbul.2011.01.012
da Silva A. R., Guerreiro A., da S., Martins S. E., Sandrini J. Z. (2021). DCOIT unbalances the antioxidant defense system in juvenile and adults of the marine bivalve amarilladesma mactroides (Mollusca: Bivalvia). Comp. Biochem. Physiol. Part - C Toxicol. Pharmacol. 250, 109169. doi: 10.1016/j.cbpc.2021.109169
De’Ath G., Fabricius K. E., Sweatman H., Puotinen M. (2012). The 27-year decline of coral cover on the great barrier reef and its causes. Proc. Natl. Acad. Sci. U.S.A. 109, 17995–17999. doi: 10.1073/pnas.1208909109
de Campos B. G., do Prado e Silva M. B. M., Avelelas F., Maia F., Loureiro S., Perina F., et al. (2022). Toxicity of innovative antifouling additives on an early life stage of the oyster crassostrea gigas: Short- and long-term exposure effects. Environ. Sci. pollut. Res. 29, 27534–27547. doi: 10.1007/s11356-021-17842-3
Detty M. R., Ciriminna R., Bright F. V., Pagliaro M. (2014). Environmentally benign sol-gel antifouling and foul-releasing coatings. Acc. Chem. Res. 47, 678–687. doi: 10.1021/ar400240n
Dörfler S. (2019) XMedia recode 3.4.8.3. Available at: https://www.xmedia-recode.de/en/version.php.
Eom H. J., Haque M. N., Nam S. E., Lee D. H., Rhee J. S. (2019). Effects of sublethal concentrations of the antifouling biocide Sea-nine on biochemical parameters of the marine polychaete perinereis aibuhitensis. Comp. Biochem. Physiol. Part - C Toxicol. Pharmacol. 222, 125–134. doi: 10.1016/j.cbpc.2019.05.001
Faimali M., Gambardella C., Costa E., Piazza V., Morgana S., Estévez-Calvar N., et al. (2017). Old model organisms and new behavioral end-points: Swimming alteration as an ecotoxicological response. Mar. Environ. Res. 128, 36–45. doi: 10.1016/j.marenvres.2016.05.006
Fel J. P., Lacherez C., Bensetra A., Mezzache S., Béraud E., Léonard M., et al. (2019). Photochemical response of the scleractinian coral stylophora pistillata to some sunscreen ingredients. Coral Reefs 38, 109–122. doi: 10.1007/s00338-018-01759-4
Ferreira V., Pavlaki M. D., Martins R., Monteiro M. S., Maia F., Tedim J., et al. (2021). Effects of nanostructure antifouling biocides towards a coral species in the context of global changes. Sci. Total Environ. 799, 149324. doi: 10.1016/j.scitotenv.2021.149324
Figueiredo J., Loureiro S., Martins R. (2020). Hazard of novel anti-fouling nanomaterials and biocides DCOIT and silver to marine organisms. Environ. Sci. Nano 7, 1670–1680. doi: 10.1039/d0en00023j
Finlay J. A., Bennett S. M., Brewer L. H., Sokolova A., Clay G., Gunari N., et al. (2010). Barnacle settlement and the adhesion of protein and diatom microfouling to xerogel films with varying surface energy and water wettability. Biofouling 26, 657–666. doi: 10.1080/08927014.2010.506242
Fonseca V. B., Guerreiro A., da S., Vargas M. A., Sandrini J. Z. (2020). Effects of DCOIT (4,5-dichloro-2-octyl-4-isothiazolin-3-one) to the haemocytes of mussels perna perna. Comp. Biochem. Physiol. Part - C Toxicol. Pharmacol. 232, 108737. doi: 10.1016/j.cbpc.2020.108737
Fox J., Weisberg S. (2019). An R companion to applied regression. 3rd ed. (Sage Publications). Available at: https://uk.sagepub.com/en-gb/eur/an-r-companion-to-applied-regression/book246125.
Gardner T. A., Côté I. M., Gill J. A., Grant A., Watkinson A. R. (2003). Long-term region-wide declines in Caribbean corals. Science 301, 958–960. doi: 10.1126/science.1086050
Garnier S. trackR - multi-object tracking with r. Available at: https://swarm-lab.github.io/trackR/.
Gerhardt A. (2007). Aquatic behavioral ecotoxicology - prospects and limitations. Hum. Ecol. Risk Assess. 13, 481–491. doi: 10.1080/10807030701340839
Groneberg A. H., Herget U., Ryu S., De Marco R. J. (2015). Positive taxis and sustained responsiveness to water motions in larval zebrafish. Front. Neural Circuits 9. doi: 10.3389/fncir.2015.00009
Gutner-Hoch E., Martins R., Oliveira T., Maia F., Soares A., Loureiro S., et al. (2018). Antimacrofouling efficacy of innovative inorganic nanomaterials loaded with booster biocides. J. Mar. Sci. Eng. 6, 6. doi: 10.3390/jmse6010006
Harayashiki C. A. Y., Reichelt-Brushett A., Cowden K., Benkendorff K. (2018). Effects of oral exposure to inorganic mercury on the feeding behaviour and biochemical markers in yellowfin bream (Acanthopagrus australis). Mar. Environ. Res. 134, 1–15. doi: 10.1016/j.marenvres.2017.12.018
Harayashiki C. A. Y., Reichelt-Brushett A. J., Liu L., Butcher P. (2016). Behavioural and biochemical alterations in penaeus monodon post-larvae diet-exposed to inorganic mercury. Chemosphere 164, 241–247. doi: 10.1016/j.chemosphere.2016.08.085
Harmer A. M. T., Thomas D. B. (2019). Pathtrackr: An r package for video tracking and analysing animal movement. Methods Ecol. Evol. 10, 1196–1202. doi: 10.1111/2041-210X.13200
Harrison P. L., Wallace C. C. (1990). “Reproduction, dispersal and recruitment of scleractinian corals,” in Coral reefs (Ecosystems of the world; 25). Ed. Dubinsky Z. (New York: Elsevier Science Publishing Company), 133–207.
Herget K., Frerichs H., Pfitzner F., Tahir M. N., Tremel W. (2018). Functional enzyme mimics for oxidative halogenation reactions that combat biofilm formation. Adv. Mater. 30, 1–28. doi: 10.1002/adma.201707073
Herget K., Hubach P., Pusch S., Deglmann P., Götz H., Gorelik T. E., et al. (2017a). Haloperoxidase mimicry by CeO2-x nanorods combats biofouling. Adv. Mater. 29, 1–28. doi: 10.1002/adma.201603823
Herget K., Hubach P., Pusch S., Deglmann P., Götz H., Gorelik T. E., et al. (2017b). Supporting information: Haloperoxidase mimicry by CeO2-x nanorods combats biofouling. Adv. Mater. 29, 1603823. doi: 10.1002/adma.201603823
Hughes T. P., Graham N. A. J., Jackson J. B. C., Mumby P. J., Steneck ,. R. S. (2010). Rising to the challenge of sustaining coral reef resilience. Trends Ecol. Evol. 25, 633–642. doi: 10.1016/j.tree.2010.07.011
Hughes T. P., Kerry J. T., Álvarez-Noriega M., Álvarez-Romero J. G., Anderson K. D., Baird A. H., et al. (2017). Global warming and recurrent mass bleaching of corals. Nature 543, 373–377. doi: 10.1038/nature21707
Hughes T. P., Rodrigues M. J., Bellwood D. R., Ceccarelli D., Hoegh-Guldberg O., McCook L., et al. (2007). Phase shifts, herbivory, and the resilience of coral reefs to climate change. Curr. Biol. 17, 360–365. doi: 10.1016/j.cub.2006.12.049
Ianna M. L., Reichelt-Brushett A., Howe P. L., Brushett D. (2020). Application of a behavioural and biochemical endpoint in ecotoxicity testing with exaiptasia pallida. Chemosphere 257, 127240. doi: 10.1016/j.chemosphere.2020.127240
Ishibashi H., Minamide S., Takeuchi I. (2018). Identification and characterization of heat shock protein 90 (HSP90) in the hard coral acropora tenuis in response to irgarol 1051. Mar. pollut. Bull. 133, 773–780. doi: 10.1016/j.marpolbul.2018.06.014
Ji Z., Wang X., Zhang H., Lin S., Meng H., Sun B., et al. (2012). Designed synthesis of CeO2 nanorods and nanowires for studying toxicological effects of high aspect ratio nanomaterials. ACS Nano 6, 5366–5380. doi: 10.1021/nn3012114
Kane A. S., Salierno J. D., Brewer S. K. (2005). “Fish models in behavioral toxicology: Automated techniques, updates and perspectives,” in Methods in aquatic toxicology (Boca Raton, Florida: Lewis Publishers), 559–590. Available at: http://aquaticpath.phhp.ufl.edu/pubs/images/AqToxMethods-Ch32.pdf.
Karcher D. B., Roth F., Carvalho S., El-Khaled Y. C., Tilstra A., Kürten B., et al. (2020). Nitrogen eutrophication particularly promotes turf algae in coral reefs of the central red Sea. PeerJ 2020, 1–25. doi: 10.7717/peerj.8737
Korschelt K., Schwidetzky R., Pfitzner F., Strugatchi J., Schilling C., Von Der Au M., et al. (2018a). CeO2-: X nanorods with intrinsic urease-like activity. Nanoscale 10, 13074–13082. doi: 10.1039/c8nr03556c
Korschelt K., Tahir M. N., Tremel W. (2018b). A step into the future: Applications of nanoparticle enzyme mimics. Chem. - A Eur. J. 24, 9703–9713. doi: 10.1002/chem.201800384
Kwok C. K., Ang P. O. (2013). Inhibition of larval swimming activity of the coral (Platygyra acuta) by interactive thermal and chemical stresses. Mar. pollut. Bull. 74, 264–273. doi: 10.1016/j.marpolbul.2013.06.048
Kwok C. K., Lam K. Y., Leung S. M., Chui A. P. Y., Ang P. O. (2016). Copper and thermal perturbations on the early life processes of the hard coral platygyra acuta. Coral Reefs 35, 827–838. doi: 10.1007/s00338-016-1432-1
Lenth R. V. (2021) Emmeans: estimated marginal means. Available at: https://cran.r-project.org/package=emmeans.
Linares C., Cebrian E., Coma R. (2012). Effects of turf algae on recruitment and juvenile survival of gorgonian corals. Mar. Ecol. Prog. Ser. 452, 81–88. doi: 10.3354/meps09586
Louis Y. D., Bhagooli R., Kenkel C. D., Baker A. C., Dyall ,. S. D. (2017). Gene expression biomarkers of heat stress in scleractinian corals: Promises and limitations. Comp. Biochem. Physiol. Part - C Toxicol. Pharmacol. 191, 63–77. doi: 10.1016/j.cbpc.2016.08.007
Maia F., Silva A. P., Fernandes S., Cunha A., Almeida A., Tedim J., et al. (2015). Incorporation of biocides in nanocapsules for protective coatings used in maritime applications. Chem. Eng. J. 270, 150–157. doi: 10.1016/j.cej.2015.01.076
Maia F., Tedim J., Lisenkov A. D., Salak A. N., Zheludkevich M. L., Ferreira M. G. S. (2012). Silica nanocontainers for active corrosion protection. Nanoscale 4, 1287–1298. doi: 10.1039/c2nr11536k
Marangoni L. F., de B., Dalmolin C., Marques J. A., Klein R. D., Abrantes D. P., et al. (2019). Oxidative stress biomarkers as potential tools in reef degradation monitoring: A study case in a south Atlantic reef under influence of the 2015–2016 El Niño/Southern oscillation (ENSO). Ecol. Indic. 106, 105533. doi: 10.1016/j.ecolind.2019.105533
Martínez-Quintana A., Bramanti L., Viladrich N., Rossi S., Guizien K. (2015). Quantification of larval traits driving connectivity: the case of corallium rubrum (L. 1758). Mar. Biol. 162, 309–318. doi: 10.1007/s00227-014-2599-z
Martins R., Oliveira T., Santos C., Kuznetsova A., Ferreira V., Avelelas F., et al. (2017). Effects of a novel anticorrosion engineered nanomaterial on the bivalve: Ruditapes philippinarum. Environ. Sci. Nano 4, 1064–1076. doi: 10.1039/c6en00630b
Miller I. B., Moeller M., Kellermann M. Y., Nietzer S., Di Mauro V., Kamyab E., et al. (2022). Towards the development of standardized bioassays for corals: Acute toxicity of the UV filter benzophenone-3 to scleractinian coral larvae. Toxics 10, 244. doi: 10.3390/toxics10050244
Miller I. B., Pawlowski S., Kellermann M. Y., Petersen-Thiery M., Moeller M., Nietzer S., et al. (2021). Toxic effects of UV filters from sunscreens on coral reefs revisited: regulatory aspects for “reef safe” products. Environ. Sci. Eur. 33, 74. doi: 10.1186/s12302-021-00515-w
Mitchelmore C. L., Burns E. E., Conway A., Heyes A., Davies I. A. (2021). A critical review of organic ultraviolet filter exposure, hazard, and risk to corals. Environ. Toxicol. Chem. 40, 967–988. doi: 10.1002/etc.4948
Moeller M., Pawlowski S., Petersen-Thiery M., Miller I. B., Nietzer S., Heisel-Sure Y., et al. (2021). Challenges in current coral reef protection – possible impacts of UV filters used in sunscreens, a critical review. Front. Mar. Sci. 8. doi: 10.3389/fmars.2021.665548
Negri A. P., Heyward A. J. (2000). Inhibition of fertilization and larval metamorphosis of the coral acropora millepora (Ehrenberg 1834) by petroleum products. Mar. pollut. Bull. 41, 420–427. doi: 10.1016/S0025-326X(00)00139-9
Negri A. P., Heyward A. J. (2001). Inhibition of coral fertilisation and larval metamorphosis by tributyltin and copper. Mar. Environ. Res. 51, 17–27. doi: 10.1016/S0141-1136(00)00029-5
Negri A. P., Smith L. D., Webster N. S., Heyward ,. A. J. (2002). Understanding ship-grounding impacts on a coral reef: Potential effects of anti-foulant paint contamination on coral recruitment. Mar. pollut. Bull. 44, 111–117. doi: 10.1016/S0025-326X(01)00128-X
Nordborg F. M., Flores F., Brinkman D. L., Agustí S., Negri A. P. (2018). Phototoxic effects of two common marine fuels on the settlement success of the coral acropora tenuis. Sci. Rep. 8, 1–12. doi: 10.1038/s41598-018-26972-7
Olsen K., Ritson-Williams R., Ochrietor J. D., Paul V. J., Ross C. (2013). Detecting hyperthermal stress in larvae of the hermatypic coral porites astreoides: The suitability of using biomarkers of oxidative stress versus heat-shock protein transcriptional expression. Mar. Biol. 160, 2609–2618. doi: 10.1007/s00227-013-2255-z
Overmans S., Nordborg M., Díaz-Rúa R., Brinkman D. L., Negri A. P., Agustí S. (2018). Phototoxic effects of PAH and UVA exposure on molecular responses and developmental success in coral larvae. Aquat. Toxicol. 198, 165–174. doi: 10.1016/j.aquatox.2018.03.008
Pandolfi J. M., Connolly S. R., Marshall D. J., Cohen A. L. (2011). Projecting coral reef futures under global warming and ocean acidification. Science 333, 418–422. doi: 10.1126/science.1204794
Parkinson J. E., Baker A. C., Baums I. B., Davies S. W., Grottoli A. G., Kitchen S. A., et al. (2020). Molecular tools for coral reef restoration: Beyond biomarker discovery. Conserv. Lett., 13, e12687. doi: 10.1111/conl.12687
Pawlowski S., Moeller M., Miller I. B., Kellermann M. Y., Schupp P. J., Petersen-Thiery M. (2021). UV Filters used in sunscreens–a lack in current coral protection? Integr. Environ. Assess. Manage. 17, 926–939. doi: 10.1002/ieam.4454
Pinheiro J., Bates D., Debroy S., Sarkar D., R Core Team (2021) Linear and nonlinear mixed effects models contact. linear nonlinear mix. eff. model. Available at: https://cran.r-project.org/package=nlme.
Randall C. J., Negri A. P., Quigley K. M., Foster T., Ricardo G. F., Webster N. S., et al. (2020). Sexual production of corals for reef restoration in the anthropocene. Mar. Ecol. Prog. Ser. 635, 203–232. doi: 10.3354/MEPS13206
R Core Team (2021) R: A language and environment for statistical computing. Available at: https://www.r-project.org/.
Reichelt-Brushett A. J., Harrison P. L. (2000). The effect of copper on the settlement success of larvae from the scleractinian coral acropora tenuis. Mar. pollut. Bull. 41, 385–391. doi: 10.1016/S0025-326X(00)00131-4
Reichelt-Brushett A. J., Harrison P. L. (2004). Development of a sublethal test to determine the effects of copper and lead on scleractinian coral larvae. Arch. Environ. Contam. Toxicol. 47, 40–55. doi: 10.1007/s00244-004-3080-7
Roepke L. K., Brefeld D., Soltmann U., Randall C. J., Negri A. P., Kunzmann A. (2022). Antifouling coatings can reduce algal growth while preserving coral settlement. Sci. Rep. 12, 15935. doi: 10.1038/s41598-022-19997-6
Romero-Ferrero F., Bergomi M. G., Hinz R. C., Heras F. J. H., de Polavieja G. G. (2019). Idtracker.Ai: Tracking all individuals in small or Large collectives of unmarked animals. Nat. Methods 16, 179–182. doi: 10.1038/s41592-018-0295-5
Ross C., Olsen K., Henry M., Pierce R. (2015). Mosquito control pesticides and sea surface temperatures have differential effects on the survival and oxidative stress response of coral larvae. Ecotoxicology 24, 540–552. doi: 10.1007/s10646-014-1402-8
Dos Santos J. V. N., Martins R., Fontes M. K., Galv B., Bruni M., Maia F. (2020). Can encapsulation of the biocide DCOIT affect the anti-fouling efficacy and toxicity on tropical bivalves? Appl. Sci. (Switzerland) 10, 1–12. doi: 10.3390/app10238579
Siebeck U. E., Marshall N. J., Klüter A., Hoegh-Guldberg O. (2006). Monitoring coral bleaching using a colour reference card. Coral Reefs 25, 453–460. doi: 10.1007/s00338-006-0123-8
Sokolova A., Bailey J. J., Waltz G. T., Brewer L. H., Finlay J. A., Fornalik J., et al. (2012). Spontaneous multiscale phase separation within fluorinated xerogel coatings for fouling-release surfaces. Biofouling 28, 143–157. doi: 10.1080/08927014.2012.659244
Spink A. J., Tegelenbosch R. A. J., Buma M. O. S., Noldus L. P. J. J. (2001). The EthoVision video tracking system - a tool for behavioral phenotyping of transgenic mice. Physiol. Behav. 73, 731–744. doi: 10.1016/S0031-9384(01)00530-3
Tang C. H., Lin C. Y., Lee S. H., Wang W. H. (2017). Membrane lipid profiles of coral responded to zinc oxide nanoparticle-induced perturbations on the cellular membrane. Aquat. Toxicol. 187, 72–81. doi: 10.1016/j.aquatox.2017.03.021
Tebben J., Guest J. R., Sin T. M., Steinberg P. D., Harder T. (2014). Corals like it waxed: Paraffin-based antifouling technology enhances coral spat survival. PloS One 9, 1–8. doi: 10.1371/journal.pone.0087545
Thia E., Chou P. H., Chen P. J. (2020). In vitro and in vivo screening for environmentally friendly benzophenone-type UV filters with beneficial tyrosinase inhibition activity. Water Res. 185, 116208. doi: 10.1016/j.watres.2020.116208
Tudorache C., Ter Braake A., Tromp M., Slabbekoorn H., Schaaf M. J. M. (2015). Behavioral and physiological indicators of stress coping styles in larval zebrafish. Stress 18, 121–128. doi: 10.3109/10253890.2014.989205
Vermeij M. J. A., Sandin S. A. (2008). Density-dependent settlement and mortality structure the earliest life phases of a coral population. Ecology 89, 1994–2004. doi: 10.1890/07-1296.1
Vermeij M. J. A., Smith J. E., Smith C. M., Vega Thurber R., Sandin S. A. (2009). Survival and settlement success of coral planulae: Independent and synergistic effects of macroalgae and microbes. Oecologia 159, 325–336. doi: 10.1007/s00442-008-1223-7
Wickham H., Averick M., Bryan J., Chang W., McGowan L., François R., et al. (2019). Welcome to the tidyverse. J. Open Source Software 4, 1686. doi: 10.21105/joss.01686
Keywords: locomotion, motility, biomarker, ecotoxicology, video-tracking systems, aquatic toxicology, coral toxicity
Citation: Roepke LK, Brefeld D, Soltmann U, Randall CJ, Negri AP and Kunzmann A (2022) Applying behavioral studies to the ecotoxicology of corals: A case study on Acropora millepora. Front. Mar. Sci. 9:1002924. doi: 10.3389/fmars.2022.1002924
Received: 25 July 2022; Accepted: 14 November 2022;
Published: 01 December 2022.
Edited by:
Iva Popovic, The University of Queensland, AustraliaReviewed by:
Amanda Reichelt-Brushett, Southern Cross University, AustraliaCopyright © 2022 Roepke, Brefeld, Soltmann, Randall, Negri and Kunzmann. This is an open-access article distributed under the terms of the Creative Commons Attribution License (CC BY). The use, distribution or reproduction in other forums is permitted, provided the original author(s) and the copyright owner(s) are credited and that the original publication in this journal is cited, in accordance with accepted academic practice. No use, distribution or reproduction is permitted which does not comply with these terms.
*Correspondence: Lisa K. Roepke, bGlzYS5yb2Vwa2VAbGVpYm5pei16bXQuZGU=
Disclaimer: All claims expressed in this article are solely those of the authors and do not necessarily represent those of their affiliated organizations, or those of the publisher, the editors and the reviewers. Any product that may be evaluated in this article or claim that may be made by its manufacturer is not guaranteed or endorsed by the publisher.
Research integrity at Frontiers
Learn more about the work of our research integrity team to safeguard the quality of each article we publish.