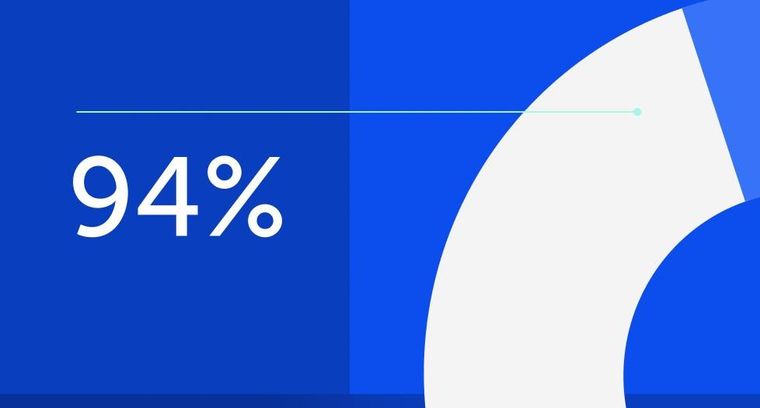
94% of researchers rate our articles as excellent or good
Learn more about the work of our research integrity team to safeguard the quality of each article we publish.
Find out more
ORIGINAL RESEARCH article
Front. Mar. Sci., 12 January 2023
Sec. Marine Conservation and Sustainability
Volume 9 - 2022 | https://doi.org/10.3389/fmars.2022.1000687
This article is part of the Research TopicIdentifying and Comparing Important Areas for Marine Sustainable Use and ConservationView all 19 articles
Connectivity plays a key role in the effectiveness of MPA networks ensuring metapopulation resilience through gene flow and recruitment effect. Yet, despite its recognized importance for proper MPA network functioning, connectivity is not often assessed and is very seldomly used in marine spatial planning. Here, we combined biophysical modelling with graph theory to identify Mediterranean marine reserves that support connectivity between different ecoregions through stepping-stone processes, thus preventing network fragmentation, and those that have an important role as propagule source areas contributing to the recruitment and rescue effects. We identified 19 reserves that play a key role towards the functioning of the network, serving either as stepping-stones or as propagule sources, yet with distinct patterns between ecological groups with contrasting propagule duration (PD). The Côte D’Azur marine reserves are important both as stepping-stones and propagule sources for several ecological groups. Also, key is the Capo Rizzuto and Plemmirio marine reserves due to their role as stepping stones between different marine ecoregions, particularly for species with longer PD (Pisces, Crustacea and Echinodermata). These results provide stakeholders and managers with crucial information for the implementation and management of an efficient marine reserve network in the Mediterranean.
The implementation of marine protected area (MPA) networks has been encouraged as these can accommodate key features, such as replication and representativity, without the need to encompass very large areas (Gaines et al., 2010). A successful network of MPAs should operate synergistically with its benefits being more than just the sum of the individual benefits of single MPAs (Hamilton et al., 2010; Grorud-Colvert et al., 2014; Roff, 2014). In this sense, connectivity plays a key role in ensuring metapopulation resilience (Kininmonth et al., 2011) and the successful functioning of an MPA network (Magris et al., 2014). Marine connectivity, i.e., the exchange of individuals among geographically separated populations, is strongly influenced by species’ behavioural and demographic characteristics (e.g., propagule duration, migratory patterns) as well as oceanographic processes (Magris et al., 2018; Balbar and Metaxas, 2019). Well-connected MPAs are able to facilitate metapopulation persistence and reverse species decline (Bonin et al., 2016), improve MPA effectiveness (Goetze et al., 2021), and better cope with climate change and stochastic events such as heatwaves or environmental disasters (Micheli et al., 2012; Bates et al., 2019).
The Convention on Biological Diversity Aichi target 11 states that conservation should be based on effectively and equitably managed, ecologically representative, and well-connected systems of protected areas (CBD, 2010). Most connectivity studies have focused on single species (e.g., Nolasco et al., 2022) or several species of the same taxonomic group (e.g., Faillettaz et al., 2018) with very few studies exploring MPA connectivity for a wide range of taxonomic groups and/or large geographic scales (but see Romero-Torres et al., 2018; Roberts et al., 2021). The incorporation of connectivity in marine spatial conservation planning has become more accessible with the development of systematic conservation planning frameworks that allow the inclusion of such data (Balbar and Metaxas, 2019; Daigle et al., 2020; Virtanen et al., 2020). Various tools have been used to estimate connectivity (for a review, see Calò et al., 2013). Depending on the spatial and temporal scale of the study, and on the species’ life stage, these can include population genetics (Jenkins and Stevens, 2018), natural tags (e.g., otoliths, Gillanders, 2005) and biotelemetry (e.g., Abecasis et al., 2009). A more recent approach to estimate the extent to which metapopulations are connected is the use of bio-physical models coupled with centrality measures from graph theory, which can predict potential connectivity at large spatial and temporal scales (Ospina-Alvarez et al., 2020). These models simulate the dispersal of propagules advected by ocean currents adding biological traits such as propagule duration (PD) or larval mortality to increase the realism of connectivity processes (Treml et al., 2012; Assis et al., 2018). The use of centrality indices from graph theory allows the identification and sorting of nodes (MPAs) in a network according to their ecological relevance (Ospina-Alvarez et al., 2020; Keeley et al., 2021). Two centrality indices can provide relevant information in the context of networks of protected areas: the Betweenness and the Out-Strength. The Betweenness centrality identifies the nodes that act as bridges or stepping-stones and thus preventing network fragmentation (Freeman, 1977; Ospina-Alvarez et al., 2020). The Out-Strength centrality identifies the nodes with the highest probability of spreading their propagules to other nodes thus acting as essential spawning habitats (source areas) for the network (Barrat et al., 2004; Ospina-Alvarez et al., 2020), translating into a higher recruitment effect. Understanding the overall structure of the MPA network and knowing the connectivity role each MPA plays is key to the successful planning and management of MPA networks (Balbar and Metaxas, 2019; Ospina-Alvarez et al., 2020).
The Mediterranean Sea is a marine biodiversity hotspot facing some of the highest anthropogenic impacts (Myers et al., 2000; Coll et al., 2012). Yet, current conservation management measures are insufficient and largely ineffective (Pérez-Ruzafa et al., 2017; Claudet et al., 2020). The existing MPAs are unevenly distributed across regions and underperforming as a network (Calò et al., 2013; Claudet et al., 2020). Indeed, low connectivity has been anticipated for the European marine reserves network, particularly striking for species with limited dispersal capacities, such as ecosystem structuring species of corals and seagrasses (Assis et al., 2021b) and some fish species (Andrello et al., 2013), highlighting the possible conservation implications. Although potential connectivity was identified for the Western Mediterranean marine reserves it is practically nonexistent among the Eastern Mediterranean marine reserves (Assis et al., 2021b). In such a context of low connectivity, identifying key MPAs acting as connectivity hubs promoting crucial links between otherwise isolated regions and/or areas acting as important propagule sources for the network provides stakeholders and managers crucial information for the implementation and management of an efficient marine reserve network.
Considering international initiatives calling for highly protected and well-connected MPAs (Convention on Biological Diversity Aichi Target 11), here, we identify the key Mediterranean marine reserves enhancing stepping-stone connectivity and increasing recruitment effect for distinct biodiversity groups. The estimates were aimed at the strongest protection level provided by marine reserves (MPAs with the strongest protection level, i.e., fully protected), owing to the higher conservation value for biodiversity (e.g., higher density of organisms; Giakoumi et al., 2017; Sala and Giakoumi, 2017) and to the lower anthropogenic impact (e.g., trawling; Sala and Giakoumi, 2017) relative to additional areas with lower protection levels (Zupan et al., 2018). Together, these traits allow reducing/removing confounding effects while inferring connectivity estimates (Eigaard et al., 2016; Sala and Giakoumi, 2017; Dureuil et al., 2018; Assis et al., 2021b). Our findings provide key insights on the individual role of marine reserves, particularly important in the scope of MPA resource management, and climate change adaptation and resilience (Almany et al., 2009; Pascual et al., 2017).
The Atlas of Marine Protection (Marine Conservation Institute, 2020) and the Database of Marine Protected Areas in the Mediterranean (MAPAMED, 2022) were used as a starting point for the compilation of the marine reserves database, as these contain the most up to date information on MPAs. To ensure that every existing marine reserve was included a further web search was conducted for each country as in Zupan et al. (2018). For each marine reserve, we gathered information on its location, size, shape, and marine ecoregion as defined in Spalding et al. (2007).
To examine the potential connectivity of Mediterranean marine reserves across multiple taxa and ecological groups, we considered four ecological groups based on the similarity of their PD following Assis et al. (2021b). Group 1 (G1) as representative of Cnidaria, Tunicata and Porifera (mean PD of 2 days), group 2 (G2) representing Macroalgae and Seagrass (mean PD of 6 days), group 3 (G3) representing Bryozoa, Mollusca and Polychaeta (mean PD of 17 days) and group 4 (G4) representing Pisces, Crustacea and Echinodermata (mean PD of 36 days). Empirical PD data was compiled from published literature as in Assis et al. (2021b). To ensure a representative sample and given the low number of species with PD information this compilation comprised marine species from all oceans.
Estimates of connectivity between the Mediterranean marine reserves were inferred with a bio-physical numerical model (Assis et al., 2015; Buonomo et al., 2017; Assis et al., 2021a) that simulated the spatial and temporal distribution of drifting propagules (i.e., planktonic dispersal stages such as larvae, spores, gametes, seeds and drifting fragments) with information on ocean currents derived from the Hybrid Coordinate Ocean Model (HYCOM), a high-resolution hindcast of three-dimensional velocity fields (spatial resolution of 0.08°, approximately 7 km in the Mediterranean Sea, with 40 depth levels). The HYCOM model is forced by wind stress, wind speed, precipitation, and heat flux (Chassignet et al., 2007) and assimilates data from an array of satellites, bathythermographs, Argo floats and moored buoys (for additional information please refer to Chassignet et al., 2007; see Supplementary Figure S1 for a representation of the surface circulation in the Mediterranean Sea). Within its spatial resolution, orders of magnitude lower than reserve distances, it mimics key oceanographic processes such as meandering currents, oceanic eddies, filaments, and fronts (Lett et al., 2008). Currently, there is no validation of the HYCOM model for the Mediterranean Sea against empirical observations (like in Domingues et al., 2012; Nolasco et al., 2018; Nolasco et al., 2022), however, studies comparing its performance elsewhere (e.g., Southern Pacific and Northern Atlantic) found accurate estimates of the prevalent oceanographic features (Fossette et al., 2012; Kendall et al., 2013). When used in bio-physical modelling, such as the current approach, HYCOM has recurrently explained the patterns of population connectivity estimated from independent genetic data of macroalgae, mussels, limpets, fish, crustaceans, echinoderms, and seagrass (Assis et al., 2015; Assis et al., 2018, Assis et al., 2022; Klein et al., 2016; Cunha et al., 2017; Ntuli et al., 2020). In particular, one of the studies, focusing on the Mediterranean Sea, showed HYCOM explaining > 75% of the variability found in population genetic data of a macroalgae (Buonomo et al., 2017).
The bio-physical model released individual particles simulating the passive drifting propagules from sites located 1km apart at the borders of each reserve every 24 hours, throughout a complete year. In the simulation, landmass was defined with a high-resolution polygon (Haklay and Weber, 2008). The geographic position of each particle was determined every hour of simulation by using bilinear interpolation over the eastward and northward components of the HYCOM’s velocity fields. The particles drifted until eventually ended up on a reserve or land (e.g., Assis et al., 2015; Assis et al., 2018; Buonomo et al., 2017; Cunha et al., 2017). Given the general absence of comprehensive information on key ecological traits for most species (e.g., fecundity, larval mortality, larval vertical migrations, and onset of swimming abilities) only the species PD was considered. To account for interannual variability, simulations were run individually for each year for a 10-year period (2008-2017) and a connectivity matrix between all pairs of sites was produced by the aggregated trajectories. Asymmetrical pairwise probabilities of connectivity between reserves were determined by dividing the number of particles released from reserve i that reached reserve j, by the total number of particles released from reserve i, thus accounting for the relative effect of reserve size, as larger reserves imply a greater amount of source sites, and therefore higher contribution to the connectivity estimates.
A graph theory approach (also referred to as network analysis) was implemented to infer and visualize stepping-stone connectivity processes between reserves (e.g., Assis et al., 2021b; Buonomo et al., 2017). To this end, the reserves defined the nodes of the graph and the pairwise connectivity matrix, averaged for the 10-years of simulation, defined the strength of its edges. Individual results were produced per ecological group, sharing similar dispersal ecology. To address the role of each marine reserve towards the functioning of the network we estimated two centrality measures of the graph, namely Betweenness and Out-Strength. To facilitate interpretation, centrality measures were normalized between 0 and 1, with 1 representing the highest values. For the Betweenness measures this normalization was performed by considering the maximum and minimum values retrieved by component (cluster), to capture the less/more central reserves, at the scale of each network aggregation. Reserves whose Betweenness or Out-Strength values were above the 95th percentile of the distribution were considered as key.
Two additional metrics were computed to characterize network structure, namely, number of components (clusters) and the relative size of the largest component. The first represents the number of disconnected subgraphs, while the second represents the ratio between the number of reserves existing in the largest component (subgraph) and the number of reserves in the entire graph (Urban & Keitt, 2001; David et al., 2022). Lastly, to examine the potential effect of losing one or more reserves (e.g., through perturbation) in network structure we performed an analysis of sequential reserve (node) deletion (Urban & Keitt, 2001; David et al., 2022), which allowed testing the importance of individual reserves to the overall coherence of the network. To this end, reserves (and all associated oceanographic connections) were removed iteratively from the network (69 iterations; considering the total number of reserves) under 3 scenarios: (1) from the highest to the lowest betweenness centrality, from the highest to the lowest out-strength centrality and randomly with 999 permutations, with no replacement. On each iteration, the number of components and relative size of the largest component metrics were computed.
All the analyses were performed in R (Team, 2021), graph analyses were produced using the ‘igraph’ package (Csardi and Nepusz, 2006).
We identified a total of 70 marine reserves in the Mediterranean Sea, distancing on average 1187.63± 772.18 km from each other (median: 1040.56km) and summing an area of 644.46 km2 representing less than 0.2% of the Mediterranean Sea (Table S1). Most of the marine reserves are located in Western Mediterranean European countries with very few in North African countries or in the Eastern Mediterranean (Table S1). This is also evident when assessing their distribution by marine ecoregions with the vast majority located in the Western Mediterranean (65.7%), and few marine reserves in the Adriatic (8.6%), Aegean (7.1%), Alboran (7.1%), Ionian (8.6%), and Levantine seas (2.9%), and none in the Tunisian plateau (Table S1).
The biophysical modelling estimating connectivity over a 10-year period released a total of 3,280,394 particles from 898 sites located at the edges of marine reserves, resulting in 1,263,927 connectivity events (38.52% of all possible events). Increasing PD per ecological group produced more distant connectivity events between reserves. Average distances of connectivity spanned from 6.95km (range: 0-44.07km), 10.68km (range: 0-186.12km), 20.01km (range: 0-530.79km) and 46.73km (range: 0-695.53km), for G1, G2, G3 and G4, respectively. Such contrasting distances driven by the PD of the 4 ecological groups correlated with the potential connectivity between reserves, with the two groups with shorter PD (G1 and G2) structuring a fragmented network (Figures 1, 2; Supplementary Figures S2-S5) with 9 and 15 components and relative size of the largest component of 0.13 and 0.11, respectively. The two groups with longer PD (G3 and G4) are able to link reserves at larger spatial scales and structuring a more coherent network (Figures 1, 2; Supplementary Figures S6–S9) with 3 and 6 components and relative size of the largest component of 0.75 and 0.94, respectively.
Figure 1 Betweenness centrality estimated for the Mediterranean network of marine reserves. Reserves are depicted as circles, with their size and colour reflecting the normalized value of betweenness. Higher betweenness, above the 95th percentile of the distribution of values, is indicated in each legend by a vertical line in the colour scale. Isolated reserves are shown in white. Mean propagule duration (± standard deviation) is shown per ecological group.
Figure 2 Out-strength centrality estimated for the Mediterranean network of marine reserves. Reserves are depicted as circles, with their size and colour reflecting the normalized value of out-strength. Higher out-strength, above the 95th percentile of the distribution of values, is indicated in each legend by a vertical line in the colour scale. Isolated reserves are shown in white. Mean propagule duration (± standard deviation) is shown per ecological group.
Graph theory using pairwise connectivity estimates showed that 19 out of 70 reserves (~27%) play a key role towards the functioning of the network (above 95th percentile of Betweenness or Out-strength), acting as stepping-stones, propagule source areas or both, for one or more ecological groups (Table 1). The marine reserves with the highest Betweenness centrality, contributing for stepping-stone connectivity, are spread throughout the Ligurian and Tyrrhenian Seas for the low dispersive groups G1 and G2, which in general display poor network connectivity (Table 1; Figure 1). As the network expands with increasing PD, new links are formed and the reserves with highest Betweenness centrality are found in the Balearic Islands and the Ionian Sea. Particularly, the Plemmirio and Capo Rizzuto reserves play an important role for G4 (Table 1), supporting stepping-stone connectivity between the western and eastern Mediterranean basins (Figure 1). The marine reserves with the highest Out-Strength centrality (Table 1; Figure 2), are located in Gibraltar and the Ligurian and Tyrrhenian Seas for G1 and G2. For G3 and G4, highest Out-Strength centrality was found in the Ligurian Sea, particularly in the Côte D’Azur area (Calanques, Port Cros and Cote Bleue reserves; Table 1), where the network of reserves is denser and therefore more connectivity events occurred (Table 1; Figure 2).
Table 1 Marine reserves on the 95th percentile for Betweenness (B), Out-Strength (O) and both (BO) for each of the ecological groups (G1 - Cnidaria, Tunicata and Porifera; G2 - Macroalgae and Seagrass; G3 - Bryozoa, Mollusca and Polychaeta; G4 - Pisces, Crustacea and Echinodermata).
The tests examining the potential effect of losing reserves in network structure allowed us to verify that reserves with higher betweenness and out-strength centrality are particularly important to the overall coherence of the network. When these reserves were iteratively removed from the network, the number of components (i.e., isolated subgraphs) increased and the size of the largest component decreased in a more pronounced way than when reserves were removed randomly, particularly for G3 and G4 (Figure 3; Supplementary Figures S10–S15).
Figure 3 Effect of removing marine reserves (nodes) on (A) the number of components of the network (i.e., number of clusters) and (B) the relative size of the largest component, under the 3 scenarios of sequential node deletion from the highest to the lowest betweenness centrality (highBetw.), from the highest to the lowest out-strength centrality (highOut-S.), and randomly with 999 permutations, with no replacement (Random). Network tests are related to G4 (Pisces, Crustacea, and Echinodermata). Independent figures for the additional groups (G1, G2, and G3) are available in supplementary information (Figures S10-S15).
Using theoretical bio-physical modelling coupled with graph theory, we evaluated the current network of marine reserves in the Mediterranean Sea and identified 19 reserves that can play a key role towards the functioning of the network, serving either as stepping-stones linking biogeographic regions or as propagule sources securing gene flow along the network, yet with distinct patterns for the different ecological groups. For instance, the reserves found in the central region of Côte D’Azur (Ligurian Sea) can support connectivity, either as stepping-stones or propagule sources, for numerous ecological groups with contrasting PD, while the Capo Rizzuto and Plemmirio reserves (Ionian Sea) are key hubs supporting connectivity between the western and eastern Mediterranean basins, particularly for biodiversity groups with longer PD. In general, groups with lower dispersal potential tend to be more isolated and would benefit from a denser network of marine reserves, as only 0.2% of the Mediterranean Sea is fully protected. The expansion of the current network to include currently unprotected areas that may hold high biodiversity, could improve connectivity, and should be considered for future conservation and management strategies.
Previous studies had already identified the Mediterranean Sea poor network connectivity (Andrello et al., 2013; Ospina-Alvarez et al., 2020; Assis et al., 2021b), particularly for low dispersal ecosystem structuring species like macroalgae, seagrasses and corals (Assis et al., 2021b). These connectivity patterns expose the uneven distribution of reserves throughout the Mediterranean Sea, with a low number in the Adriatic, Ionian, Aegean and Levantine Sea and a total lack of reserves in the Tunisian plateau, leading to a highly fragmented and poorly connected network, adding to the lack of habitat representativity (Abdulla et al., 2009). Thus, this generally low potential connectivity of the Mediterranean network of marine reserves undermines its overall resilience and reveals its poor capacity to perform as a proper network.
Against this background of poor connectivity, we identified those marine reserves that may standout in supporting connectivity, acting as stepping-stones between different components (high Betweenness centrality), hence securing links between different regions (Ospina-Alvarez et al., 2020) and/or acting as propagule source (high Out-strength centrality) hence representing spawning areas that play an important role towards recruitment and rescue effects (Eriksson et al., 2014; Ospina-Alvarez et al., 2020; Roberts et al., 2021). We found that 19 of the 70 Mediterranean marine reserves presented such key roles (Betweenness or Out-strength value above 95th percentile of the distribution) for at least one group of species (Table 1). However, the spatial extent of the links depended on the PD. For species with low PD (G1 and G2), connectivity links were limited in spatial scale, occurring almost exclusively with nearby marine reserves, thus failing to ensure links between different oceanographic regions. This is the case of the Gibraltar marine reserves where their proximity to each other and the local oceanographic characteristics, favour the exchange of propagules between them for the G1 and G2 species. An increase in the number of (stepping-stone) marine reserves is required to guarantee overall connectivity, particularly across different oceanographic regions. Adding new sites to expand the current network of marine reserves should be done by identifying sites that are highly connected to, but currently are not part of, a marine reserve (Magris et al., 2018). This would be crucial in transitional areas between marine ecoregions, especially in those underrepresented (e.g., Tunisian plateau, Levantine Sea) to ensure gene flow. Additionally, enhancing connectivity of G1 and G2 ecological groups, which include species such as gorgonians, sponges, and seagrass, is particularly relevant given that these species form essential habitats for other biodiversity (Pascual et al., 2017; Magris et al., 2018). In contrast, for species with longer PD, propagule flow between different marine ecoregions (e.g., Western Mediterranean, Ionian and Adriatic Seas) was partially ensured, preventing further network fragmentation. The marine reserves acting as connectivity hubs have a greater probability of spreading the genes of the local populations throughout the network thus ensuring a higher gene flow (Pascual et al., 2017). For example, the marine reserves of Capo Rizzuto and Plemmirio possibly assume a key role for connecting metapopulations between the Ionian and the Adriatic and Western Mediterranean regions, respectively. The simulated scenarios where marine reserves were sequentially removed, representing hypothetical perturbations, emphasised the role of these marine reserves acting as key connectivity hubs towards the coherence of the Mediterranean network. When marine reserves with high betweenness (or high out-strength) were removed from the network this resulted in a drastic fragmentation of the network, compared to random removal of reserves.
The approach used in the present study provided key knowledge unknown until now, however, it may have some degree of oversimplification that needs to be addressed. First, marine reserves are not the exclusive source of propagules or stepping-stone locations of marine biodiversity throughout the Mediterranean Sea, as regions outside protected areas may have an important role as well. Thus, our results provide a what-if scenario, where marine reserves are the unique source and sink locations serving connectivity (Assis et al., 2021b). Only considering such effective conservation areas is somewhat unrealistic; however, the approach is supported if we consider the increasing rate of potential threats to marine biodiversity and the need to provide adequate protection and recover ecosystems, i.e., highly protected and well-connected MPA networks. In fact, Dureuil et al. (2018) found that 59% of European MPAs are commercially trawled, precluding the expected benefits of MPA implementation such as increased productivity and spillover. This is particularly true in the Mediterranean Sea, where (over)fishing may be targeting the larger individuals that are the main spawners and source of propagules (Cardinale and Arrhenius, 2000; Vielmini et al., 2017). Secondly, despite integrating key ecological traits (i.e., propagule duration) and oceanographic processes (e.g., fronts and eddies; Manel et al., 2019), as well as the actual spatial arrangement of marine reserves, it did not consider population dynamics or larvae behaviour (Anadón et al., 2013; Faillettaz et al., 2018). For example, spawning seasonality is known to affect propagule dispersal and consequently connectivity (Kough and Paris, 2015; Torrado et al., 2021). Additionally, some species exhibit ontogenetic shifts in habitat use and/or large migrations during adult phases (e.g., Abecasis et al., 2009; Vandeperre et al., 2014). Our goal was to provide an overall view across a wide range of taxa and therefore it would be impractical to consider population dynamics (e.g., fecundity, size structure, spawning seasonality, propagule mortality) or larvae movements (e.g., vertical migrations, chemical attraction) given the general lack and inter- and intra-specific variability of these parameters (e.g., Endo et al., 2019), as well as the challenges imposed on the modelling task. Numerous studies using the same bio-physical modelling approach systematically provided evidence on how propagule duration alone coupled with oceanographic transport can explain independent genetic and demographic data, from macroalgae and corals, to fish and invertebrates (e.g., Buonomo et al., 2017; Lourenço et al., 2017; Assis et al., 2018; Nicastro et al., 2020; Ntuli et al., 2020). This is particularly true for low dispersive structuring species, such as those represented by G1 and G2, but not only, as the propagule phase represents the major dispersive phase for most marine species (Calò et al., 2013). Lastly, we assume that the ecosystem health of the marine reserves is intact allowing them to fulfil the potential connectivity roles identified by the bio-physical model. If that is not the case, then connectivity patterns among the Mediterranean network of marine reserves can be compromised.
Network connectivity and its effectiveness to provide ecological benefits can be compromised by inadequate management or poor enforcement (Edgar et al., 2014; Gill et al., 2017). Therefore, ensuring that the reserves identified here benefit from adequate compliance is key to ensure the entire network efficiency. For example, the marine reserves in the Côte D’Azur area play a key role as propagule sources for most ecological groups. Thus, ensuring their adequate management and strong enforcement is fundamental for maintaining gene flow along the network. The 19 marine reserves identified as key connectivity hubs (Table 1) present a medium to high enforcement level (Guidetti et al., 2008; Giakoumi et al., 2017; Di Lorenzo et al., 2020) fulfilling two key attributes, no-take and well enforced, that are known to influence MPA performance (Claudet et al., 2008; Di Franco et al., 2016). Increasing the size of these reserves could contribute to further maximizing network connectivity, as larger marine reserves are able not only to supply but also to capture more propagules emanating from other marine reserves (Assis et al., 2021b). Local stressors such as marine invasions (Giakoumi and Pey, 2017) and water pollution (Abessa et al., 2018), as well as global climate change (Hannah et al., 2002) can further compromise the effectiveness of the network and should be managed and controlled a priori, as impacts may be impossible to prevent once established (Abessa et al., 2018). For example, if the marine reserve of Capo Rizzuto is affected by some disaster and its role is compromised this could lead to connectivity loss between the Ionian and Adriatic Seas for G4 (fish, echinoderms and crustaceans) eventually resulting in biodiversity loss (species and genetic). Therefore, safeguarding the identified key reserve areas might be particularly relevant to ensure network resilience in the long-term, as these can function as propagule sources to replenish other regions in case of catastrophic events causing mass mortalities, such as localized pollution or heatwaves (Eriksson et al., 2014; Bonin et al., 2016; Fung et al., 2017). Climate change is expected to impact network connectivity (Lima et al., 2021) and therefore its efficiency, by altering species distributions (Ramos Martins et al., 2021) and by affecting propagule duration and ocean hydrodynamics (Connor et al., 2007). Thus, the inclusion of connectivity and its potential shifts due to climate change in conservation planning is highly recommended (Andrello et al., 2013; Álvarez-Romero et al., 2018).
Until recently, conservation prioritization in the Mediterranean Sea was based on estimates of biodiversity distribution and anthropogenic threats (Myers et al., 2000; Coll et al., 2012), while connectivity was overlooked. Here, we identify the key connectivity hubs (stepping-stones and propagule sources) of the current network of the Mediterranean reserves. In line with the ongoing efforts to increase protection to 30% by 2030 and considering the low reserve coverage of the Mediterranean Sea and the fragmentation of the current reserve network, future studies should focus on identifying additional areas (currently unprotected) that may be key in improving network connectivity. Including such areas in the network, particularly in the Eastern and Southern Mediterranean, could contribute to a more efficient and resilient network. The overall Mediterranean connectivity should then be included in systematic conservation planning to optimize the design and efficiency of the network (Schill et al., 2015; Balbar and Metaxas, 2019), transforming it from a group of isolated MPAs to a truly functional reserve network (Albert et al., 2017; Roberts et al., 2021).
The datasets presented in this study can be found in online repositories. The names of the repository/repositories and accession number(s) can be found below: Biophysical model available as open source at: http://github.com/jorgeassis/biophysicalModelling. Vector shapefile for biophysical modelling with digitized polygons of the Mediterranean marine reserves available at: 10.6084/m9.figshare.21670658.
Conceptualization: DA, EF, and JA. Data curation: BC and EF. Data analysis: DA, EF, and JA. Funding acquisition: DA. DA led the writing with support from EF and JA. All authors contributed to the article and approved the submitted version.
This study was funded by the European Maritime and Fisheries Fund and the MAR2020 program through project REDAMP (MAR-01.04.02-FEAMP-0015), the Horizon Europe Framework Programme through project MPAEurope (HORIZON-CL6-2021-BIODIV-01-12), and by the Foundation for Science and Technology (FCT) through projects UID/Multi/04326/2020, PTDC/BIA-CBI/6515/2020, transitional standards DL57/2016/CP1361/CT0035 and DL57/2016/CP1361/CT0036, and fellowship SFRH/BD/144878/2019.
The authors declare that the research was conducted in the absence of any commercial or financial relationships that could be construed as a potential conflict of interest.
All claims expressed in this article are solely those of the authors and do not necessarily represent those of their affiliated organizations, or those of the publisher, the editors and the reviewers. Any product that may be evaluated in this article, or claim that may be made by its manufacturer, is not guaranteed or endorsed by the publisher.
The Supplementary Material for this article can be found online at: https://www.frontiersin.org/articles/10.3389/fmars.2022.1000687/full#supplementary-material
Abdulla A., Gomei M., Hyrenbach D., Notarbartolo-Di-Sciara G., Agardy T. (2009). Challenges facing a network of representative marine protected areas in the Mediterranean: prioritizing the protection of underrepresented habitats. ICES J. Mar. Sci. 66, 22–28. doi: 10.1093/icesjms/fsn164
Abecasis D., Bentes L., Erzini K. (2009). Home range, residency and movements of Diplodus sargus and Diplodus vulgaris in a coastal lagoon: Connectivity between nursery and adult habitats. Estuarine Coast. Shelf Sci. 85, 525–529. doi: 10.1016/j.ecss.2009.09.001
Abessa D. M. S., Albuquerque H. C., Morais L. G., Araújo G. S., Fonseca T. G., Cruz A. C. F., et al. (2018). Pollution status of marine protected areas worldwide and the consequent toxic effects are unknown. Environ. pollut. 243, 1450–1459. doi: 10.1016/j.envpol.2018.09.129
Albert C. H., Rayfield B., Dumitru M., Gonzalez A. (2017). Applying network theory to prioritize multispecies habitat networks that are robust to climate and land-use change. Conserv. Biol. 31, 1383–1396. doi: 10.1111/cobi.12943
Almany G. R., Connolly S. R., Heath D. D., Hogan J. D., Jones G. P., Mccook L. J., et al. (2009). Connectivity, biodiversity conservation and the design of marine reserve networks for coral reefs. Coral Reefs 28, 339–351. doi: 10.1007/s00338-009-0484-x
Álvarez-Romero J. G., Munguía-Vega A., Beger M., Mar Mancha-Cisneros M., Suárez-Castillo A. N., Gurney G. G., et al. (2018). Designing connected marine reserves in the face of global warming. Global Change Biol. 24, e671–e691. doi: 10.1111/gcb.13989
Anadón J. D., Del Mar Mancha-Cisneros M., Best B. D., Gerber L. R. (2013). Habitat-specific larval dispersal and marine connectivity: implications for spatial conservation planning. Ecosphere 4, art82. doi: 10.1890/ES13-00119.1
Andrello M., Mouillot D., Beuvier J., Albouy C., Thuiller W., Manel S. (2013). Low connectivity between Mediterranean marine protected areas: A biophysical modeling approach for the dusky grouper epinephelus marginatus. PloS One 8, e68564. doi: 10.1371/journal.pone.0068564
Assis J., Failler P., Fragkopoulou E., Abecasis D., Touron-Gardic G., Regalla A., et al. (2021a). Potential biodiversity connectivity in the network of marine protected areas in Western Africa. Front. Mar. Sci. 8. doi: 10.3389/fmars.2021.765053
Assis J., Fragkopoulou E., Serrão E. A., Horta E Costa B., Gandra M., Abecasis D. (2021b). Weak biodiversity connectivity in the European network of no-take marine protected areas. Sci. Total Environ. 773, 145664. doi: 10.1016/j.scitotenv.2021.145664
Assis J., Serrão E.Á., Coelho N. C., Tempera F., Valero M., Alberto F. (2018). Past climate changes and strong oceanographic barriers structured low-latitude genetic relics for the golden kelp laminaria ochroleuca. J. Biogeogr. 45, 2326–2336. doi: 10.1111/jbi.13425
Assis J., Zupan M., Nicastro K. R., Zardi G. I., Mcquaid C. D., Serrão E. A. (2015). Oceanographic conditions limit the spread of a marine invader along southern African shores. PloS One 10, e0128124. doi: 10.1371/journal.pone.0128124
Assis J. (2022). Biophysical modelling framework to estimate oceanographic connectivity, GitHub repository. Available at: https://github.com/jorgeassis/biophysicalModelling.
Balbar A. C., Metaxas A. (2019). The current application of ecological connectivity in the design of marine protected areas. Global Ecol. Conserv. 17, e00569. doi: 10.1016/j.gecco.2019.e00569
Barrat A., Barthélemy M., Pastor-Satorras R., Vespignani A. (2004). The architecture of complex weighted networks. Proc. Natl. Acad. Sci. United States America 101, 3747. doi: 10.1073/pnas.0400087101
Bates A. E., Cooke R. S. C., Duncan M. I., Edgar G. J., Bruno J. F., Benedetti-Cecchi L., et al. (2019). Climate resilience in marine protected areas and the ‘Protection paradox’. Biol. Conserv. 236, 305–314. doi: 10.1016/j.biocon.2019.05.005
Bonin M. C., Harrison H. B., Williamson D. H., Frisch A. J., Saenz-Agudelo P., Berumen M. L., et al. (2016). The role of marine reserves in the replenishment of a locally impacted population of anemonefish on the great barrier reef. Mol. Ecol. 25, 487–499. doi: 10.1111/mec.13484
Buonomo R., Assis J., Fernandes F., Engelen A. H., Airoldi L., Serrão E. A. (2017). Habitat continuity and stepping-stone oceanographic distances explain population genetic connectivity of the brown alga cystoseira amentacea. Mol. Ecol. 26, 766–780. doi: 10.1111/mec.13960
Calò A., Félix-Hackradt F. C., Garcia J., Hackradt C. W., Rocklin D., Treviño Otón J., et al. (2013). A review of methods to assess connectivity and dispersal between fish populations in the Mediterranean Sea. Adv. Oceanogr. Limnol. 4, 150–175. doi: 10.4081/aiol.2013.5342
Cardinale M., Arrhenius F. (2000). The relationship between stock and recruitment: are the assumptions valid? Mar. Ecol. Prog. Ser. 196, 305–309. doi: 10.3354/meps196305
CBD (2010). Decision adopted by the Conference of the Parties to the Convention on Biological Diversity at its tenth meeting. Decision X/2. Strategic plan for biodiversity 2011–2020, Aichi, Japan. Available at: https://www.cbd.int/doc/decisions/cop-10/cop-10-dec-02-en.pdf.
Chassignet E. P., Hurlburt H. E., Smedstad O. M., Halliwell G. R., Hogan P. J., Wallcraft A. J., et al. (2007). The HYCOM (HYbrid coordinate ocean model) data assimilative system. J. Mar. Syst. 65, 60–83. doi: 10.1016/j.jmarsys.2005.09.016
Claudet J., Loiseau C., Sostres M., Zupan M. (2020). Underprotected marine protected areas in a global biodiversity hotspot. One Earth 2, 380–384. doi: 10.1016/j.oneear.2020.03.008
Claudet J., Osenberg C. W., Benedetti-Cecchi L., Domenici P., García-Charton J.-A., Perez-Ruzafa A., et al. (2008). Marine reserves: size and age do matter. Ecol. Lett. 11, 481–489. doi: 10.1111/j.1461-0248.2008.01166.x
Coll M., Piroddi C., Albouy C., Ben Rais Lasram F., Cheung W. W. L., Christensen V., et al. (2012). The Mediterranean Sea under siege: spatial overlap between marine biodiversity, cumulative threats and marine reserves. Global Ecol. Biogeogr. 21, 465–480. doi: 10.1111/j.1466-8238.2011.00697.x
Connor M. I., Bruno J. F., Gaines S. D., Halpern B. S., Lester S. E., Kinlan B. P., et al. (2007). Temperature control of larval dispersal and the implications for marine ecology, evolution, and conservation. Proc. Natl. Acad. Sci. 104, 1266. doi: 10.1073/pnas.0603422104
Csardi G., Nepusz T. (2006). The igraph software package for complex network research. InterJournal Complex Syst. 1695. Available at: https://igraph.org.
Cunha R. L., Assis J. M., Madeira C., Seabra R., Lima F. P., Lopes E. P., et al. (2017). Drivers of cape Verde archipelagic endemism in keyhole limpets. Sci. Rep. 7, 41817. doi: 10.1038/srep41817
Daigle R. M., Metaxas A., Balbar A. C., Mcgowan J., Treml E. A., Kuempel C. D., et al. (2020). Operationalizing ecological connectivity in spatial conservation planning with marxan connect. Methods Ecol. Evol 11, 570–579. doi: 10.1111/2041-210X.13349
David C. L., Marzloff M. P., Knights A. M., Cugier P., Nunes F. L. D., Cordier C., et al. (2022). Connectivity modelling informs metapopulation structure and conservation priorities for a reef-building species. Diversity Distributions 28, 2056–2070. doi: 10.1111/ddi.13596
Di Franco A., Thiriet P., Di Carlo G., Dimitriadis C., Francour P., Gutiérrez N. L., et al. (2016). Five key attributes can increase marine protected areas performance for small-scale fisheries management. Sci. Rep. 6, 38135. doi: 10.1038/srep38135
Di Lorenzo M., Guidetti P., Di Franco A., Calò A., Claudet J. (2020). Assessing spillover from marine protected areas and its drivers: A meta-analytical approach. Fish Fisheries 21, 906–915. doi: 10.1111/faf.12469
Domingues C. P., Nolasco R., Dubert J., Queiroga H. (2012). Model-derived dispersal pathways from multiple source populations explain variability of invertebrate larval supply. PloS One 7, e35794. doi: 10.1371/journal.pone.0035794
Dureuil M., Boerder K., Burnett K. A., Froese R., Worm B. (2018). Elevated trawling inside protected areas undermines conservation outcomes in a global fishing hot spot. Science 362, 1403–1407. doi: 10.1126/science.aau0561
Edgar G. J., Stuart-Smith R. D., Willis T. J., Kininmonth S., Baker S. C., Banks S., et al. (2014). Global conservation outcomes depend on marine protected areas with five key features. Nature 506, 216–220. doi: 10.1038/nature13022
Eigaard O. R., Bastardie F., Breen M., Dinesen G. E., Hintzen N. T., Laffargue P., et al. (2016). Estimating seabed pressure from demersal trawls, seines, and dredges based on gear design and dimensions. ICES J. Mar. Sci. 73, i27–i43. doi: 10.1093/icesjms/fsw116
Endo C., Gherardi D. F. M., Pezzi L. P., Lima L. N. (2019). Low connectivity compromises the conservation of reef fishes by marine protected areas in the tropical south Atlantic. Sci. Rep. 9, 8634. doi: 10.1038/s41598-019-45042-0
Eriksson A., Elías-Wolff F., Mehlig B., Manica A. (2014). The emergence of the rescue effect from explicit within- and between-patch dynamics in a metapopulation. Proc. R. Soc. B: Biol. Sci. 281, 20133127. doi: 10.1098/rspb.2013.3127
Faillettaz R., Paris C. B., Irisson J.-O. (2018). Larval fish swimming behavior alters dispersal patterns from marine protected areas in the north-Western Mediterranean Sea. Front. Mar. Sci. 5. doi: 10.3389/fmars.2018.00097
Freeman L. C. (1977). A set of measures of centrality based on betweenness. Sociometry 40, 35–41. doi: 10.2307/3033543
Fossette S., Putman N. F., Lohmann K. J., Marsh R., Hays G. C. (2012). A biologist's guide to assessing ocean currents: a review. Mar. Ecol. Prog. Ser. 457, 285–301. doi: 10.3354/meps09581
Fung E., Imbach P., Corrales L., Vilchez S., Zamora N., Argotty F., et al. (2017). Mapping conservation priorities and connectivity pathways under climate change for tropical ecosystems. Climatic Change 141, 77–92. doi: 10.1007/s10584-016-1789-8
Gaines S. D., White C., Carr M. H., Palumbi S. R. (2010). Designing marine reserve networks for both conservation and fisheries management. Proc. Natl. Acad. Sci. 107, 18286–18293. doi: 10.1073/pnas.0906473107
Giakoumi S., Pey A. (2017). Assessing the effects of marine protected areas on biological invasions: A global review. Front. Mar. Sci. 4. doi: 10.3389/fmars.2017.00049
Giakoumi S., Scianna C., Plass-Johnson J., Micheli F., Grorud-Colvert K., Thiriet P., et al. (2017). Ecological effects of full and partial protection in the crowded Mediterranean Sea: a regional meta-analysis. Sci. Rep. 7, 8940. doi: 10.1038/s41598-017-08850-w
Gillanders B. M. (2005). Using elemental chemistry of fish otoliths to determine connectivity between estuarine and coastal habitats. Estuarine Coast. Shelf Sci. 64, 47–57. doi: 10.1016/j.ecss.2005.02.005
Gill D. A., Mascia M. B., Ahmadia G. N., Glew L., Lester S. E., Barnes M., et al. (2017). Capacity shortfalls hinder the performance of marine protected areas globally. Nature 543, 665–669. doi: 10.1038/nature21708
Goetze J. S., Wilson S., Radford B., Fisher R., Langlois T. J., Monk J., et al. (2021). Increased connectivity and depth improve the effectiveness of marine reserves. Global Change Biol. 27, 3432–3447. doi: 10.1111/gcb.15635
Grorud-Colvert K., Claudet J., Tissot B. N., Caselle J. E., Carr M. H., Day J. C., et al. (2014). Marine protected area networks: Assessing whether the whole is greater than the sum of its parts. PloS One 9, e102298. doi: 10.1371/journal.pone.0102298
Guidetti P., Milazzo M., Bussotti S., Molinari A., Murenu M., Pais A., et al. (2008). Italian Marine reserve effectiveness: Does enforcement matter? Biol. Conserv. 141, 699–709. doi: 10.1016/j.biocon.2007.12.013
Haklay M., Weber P. (2008). OpenStreetMap: User-generated Street maps. IEEE Pervasive Computing 7, 12–18. doi: 10.1109/MPRV.2008.80
Hamilton S. L., Caselle J. E., Malone D. P., Carr M. H. (2010). Incorporating biogeography into evaluations of the channel islands marine reserve network. Proc. Natl. Acad. Sci. 107, 18272–18277. doi: 10.1073/pnas.0908091107
Hannah L., Midgley G. F., Millar D. (2002). Climate change-integrated conservation strategies. Global Ecol. Biogeogr. 11, 485–495. doi: 10.1046/j.1466-822X.2002.00306.x
Jenkins T. L., Stevens J. R. (2018). Assessing connectivity between MPAs: Selecting taxa and translating genetic data to inform policy. Mar. Policy 94, 165–173. doi: 10.1016/j.marpol.2018.04.022
Keeley A. T. H., Beier P., Jenness J. S. (2021). Connectivity metrics for conservation planning and monitoring. Biol. Conserv. 255, 109008. doi: 10.1016/j.biocon.2021.109008
Kendall M. S., Poti M., Wynne T. T., Kinlan B. P., Bauer L. B. (2013). Consequences of the life history traits of pelagic larvae on interisland connectivity during a changing climate. Mar. Ecol. Prog. Ser. 489, 43–59. doi: 10.3354/meps10432
Kininmonth S., Beger M., Bode M., Peterson E., Adams V. M., Dorfman D., et al. (2011). Dispersal connectivity and reserve selection for marine conservation. Ecol. Model. 222, 1272–1282. doi: 10.1016/j.ecolmodel.2011.01.012
Klein M., Teixeira S., Assis J., Serrão E. A., Gonçalves E. J., Borges R. (2016). High interannual variability in connectivity and genetic pool of a temperate clingfish matches oceanographic transport predictions. PloS One 11, e0165881. doi: 10.1371/journal.pone.0165881
Kough A. S., Paris C. B. (2015). The influence of spawning periodicity on population connectivity. Coral Reefs 34, 753–757. doi: 10.1007/s00338-015-1311-1
Lett C., Verley P., Mullon C., Parada C., Brochier T., Penven P., et al. (2008). A Lagrangian tool for modelling ichthyoplankton dynamics. Environ. Model. Software 23, 1210–1214. doi: 10.1016/j.envsoft.2008.02.005
Lima L. S., Gherardi D. F. M., Pezzi L. P., Passos L. G. D., Endo C. A. K., Quimbayo J. P. (2021). Potential changes in the connectivity of marine protected areas driven by extreme ocean warming. Sci. Rep. 11, 10339. doi: 10.1038/s41598-021-89192-6
Lourenço C. R., Nicastro K. R., Mcquaid C. D., Chefaoui R. M., Assis J., Taleb M. Z., et al. (2017). Evidence for rangewide panmixia despite multiple barriers to dispersal in a marine mussel. Sci. Rep. 7, 10279. doi: 10.1038/s41598-017-10753-9
Magris R. A., Andrello M., Pressey R. L., Mouillot D., Dalongeville A., Jacobi M. N., et al. (2018). Biologically representative and well-connected marine reserves enhance biodiversity persistence in conservation planning. Conserv. Lett. 11, e12439. doi: 10.1111/conl.12439
Magris R. A., Pressey R. L., Weeks R., Ban N. C. (2014). Integrating connectivity and climate change into marine conservation planning. Biol. Conserv. 170, 207–221. doi: 10.1016/j.biocon.2013.12.032
Manel S., Loiseau N., Andrello M., Fietz K., Goñi R., Forcada A., et al (2019) Long-distance benefits of marine reserves: Myth or reality? Trends Ecol. Evol 34, 342–354. doi: 10.1016/j.tree.2019.01.002
MAPAMED (2022). MAPAMED, the database of MArine Protected Areas in the MEDiterranean. 2019 edition, version 2. SPA/RAC and MedPAN. Available at: https://www.mapamed.org/.
Marine Conservation Institute (2020). MPAtlas (Seattle, WA). Available at: www.mpatlas.org.
Micheli F., Saenz-Arroyo A., Greenley A., Vazquez L., Espinoza Montes J. A., Rossetto M., et al. (2012). Evidence that marine reserves enhance resilience to climatic impacts. PloS One 7, e40832. doi: 10.1371/journal.pone.0040832
Myers N., Mittermeier R. A., Mittermeier C. G., Da Fonseca G. A. B., Kent J. (2000). Biodiversity hotspots for conservation priorities. Nature 403, 853–858. doi: 10.1038/35002501
Nicastro K. R., Assis J., Serrão E. A., Pearson G. A., Neiva J., Valero M., et al. (2020). Congruence between fine-scale genetic breaks and dispersal potential in an estuarine seaweed across multiple transition zones. ICES J. Mar. Sci. 77, 371–378. doi: 10.1093/icesjms/fsz179
Nolasco R., Dubert J., Acuña J. L., Aguión A., Cruz T., Fernandes J. N., et al. (2022). Biophysical modelling of larval dispersal and population connectivity of a stalked barnacle: implications for fishery governance. Mar. Ecol. Prog. Ser. 694, 105–123. doi: 10.3354/meps14097
Nolasco R., Gomes I., Peteiro L., Albuquerque R., Luna T., Dubert J., et al. (2018). Independent estimates of marine population connectivity are more concordant when accounting for uncertainties in larval origins. Sci. Rep. 8, 2641. doi: 10.1038/s41598-018-19833-w
Ntuli N. N., Nicastro K. R., Zardi G. I., Assis J., Mcquaid C. D., Teske P. R. (2020). Rejection of the genetic implications of the “Abundant centre hypothesis” in marine mussels. Sci. Rep. 10, 604. doi: 10.1038/s41598-020-57474-0
Ospina-Alvarez A., De Juan S., Alós J., Basterretxea G., Alonso-Fernández A., Follana-Berná G., et al. (2020). MPA network design based on graph theory and emergent properties of larval dispersal. Mar. Ecol. Prog. Ser. 650, 309–326. doi: 10.3354/meps13399
Pascual M., Rives B., Schunter C., Macpherson E. (2017). Impact of life history traits on gene flow: A multispecies systematic review across oceanographic barriers in the Mediterranean Sea. PloS One 12, e0176419. doi: 10.1371/journal.pone.0176419
Pérez-Ruzafa A., García-Charton J. A., Marcos C. (2017). North East Atlantic vs. Mediterranean marine protected areas as fisheries management tool. Front. Mar. Sci. 4. doi: 10.3389/fmars.2017.00245
Ramos Martins M., Assis J., Abecasis D. (2021). Biologically meaningful distribution models highlight the benefits of the Paris agreement for demersal fishing targets in the north Atlantic ocean. Global Ecol. Biogeogr. 30, 1643–1656. doi: 10.1111/geb.13327
Roberts K. E., Cook C. N., Beher J., Treml E. A. (2021). Assessing the current state of ecological connectivity in a large marine protected area system. Conserv. Biol. 35, 699–710. doi: 10.1111/cobi.13580
Roff J. C. (2014). Networks of marine protected areas – the demonstrability dilemma. Aquat. Conservation: Mar. Freshw. Ecosyst. 24, 1–4. doi: 10.1002/aqc.2429
Romero-Torres M., Treml E. A., Acosta A., Paz-García D. A. (2018). The Eastern tropical pacific coral population connectivity and the role of the Eastern pacific barrier. Sci. Rep. 8, 9354. doi: 10.1038/s41598-018-27644-2
Sala E., Giakoumi S. (2017). No-take marine reserves are the most effective protected areas in the ocean. ICES J. Mar. Sci 75, 1166–1168. doi: 10.1093/icesjms/fsx059
Schill S. R., Raber G. T., Roberts J. J., Treml E. A., Brenner J., Halpin P. N. (2015). No reef is an island: Integrating coral reef connectivity data into the design of regional-scale marine protected area networks. PloS One 10 (12), e0144199. doi: 10.1371/journal.pone.0144199
Spalding M. D., Fox H. E., Allen G. R., Davidson N., Ferdaña Z. A., Finlayson M., et al. (2007). Marine ecoregions of the world: A bioregionalization of coastal and shelf areas. BioScience 57, 573–583. doi: 10.1641/B570707
Team, R (2021) R: A language and environment for statistical computing (Vienna, Austria: R Foundation for Statistical Computing).
Torrado H., Mourre B., Raventos N., Carreras C., Tintoré J., Pascual M., et al. (2021). Impact of individual early life traits in larval dispersal: A multispecies approach using backtracking models. Prog. Oceanogr. 192, 102518. doi: 10.1016/j.pocean.2021.102518
Treml E. A., Roberts J. J., Chao Y., Halpin P. N., Possingham H. P., Riginos C. (2012). Reproductive output and duration of the pelagic larval stage determine seascape-wide connectivity of marine populations. Integr. Comp. Biol. 52, 525–537. doi: 10.1093/icb/ics101
Urban D., Keitt T. (2001). Landscape connectivity: a graph-theoretic perspective. Ecology 82, 1205–1218. doi: 10.1890/0012-9658(2001)082[1205:LCAGTP]2.0.CO;2
Vandeperre F., Aires-Da-Silva A., Fontes J., Santos M., Serrão Santos R., Afonso P. (2014). Movements of blue sharks (Prionace glauca) across their life history. PloS One 9, e103538. doi: 10.1371/journal.pone.0103538
Vielmini I., Perry A. L., Cornax M. J. (2017). Untying the Mediterranean Gordian knot: A twenty first century challenge for fisheries management. Front. Mar. Sci. 4. doi: 10.3389/fmars.2017.00195
Virtanen E. A., Moilanen A., Viitasalo M. (2020). Marine connectivity in spatial conservation planning: analogues from the terrestrial realm. Landscape Ecol. 35, 1021–1034. doi: 10.1007/s10980-020-00997-8
Keywords: marine protected areas (MPAs), biodiversity conservation, resource management, propagule dispersal, stepping-stone, recruitment
Citation: Abecasis D, Fragkopoulou E, Claro B and Assis J (2023) Biophysical modelling and graph theory identify key connectivity hubs in the Mediterranean marine reserve network. Front. Mar. Sci. 9:1000687. doi: 10.3389/fmars.2022.1000687
Received: 22 July 2022; Accepted: 21 December 2022;
Published: 12 January 2023.
Edited by:
Leslie New, Ursinus College, United StatesReviewed by:
Henrique Queiroga, University of Aveiro, PortugalCopyright © 2023 Abecasis, Fragkopoulou, Claro and Assis. This is an open-access article distributed under the terms of the Creative Commons Attribution License (CC BY). The use, distribution or reproduction in other forums is permitted, provided the original author(s) and the copyright owner(s) are credited and that the original publication in this journal is cited, in accordance with accepted academic practice. No use, distribution or reproduction is permitted which does not comply with these terms.
*Correspondence: David Abecasis, ZGF2aWRiZWNhc0BnbWFpbC5jb20=
Disclaimer: All claims expressed in this article are solely those of the authors and do not necessarily represent those of their affiliated organizations, or those of the publisher, the editors and the reviewers. Any product that may be evaluated in this article or claim that may be made by its manufacturer is not guaranteed or endorsed by the publisher.
Research integrity at Frontiers
Learn more about the work of our research integrity team to safeguard the quality of each article we publish.