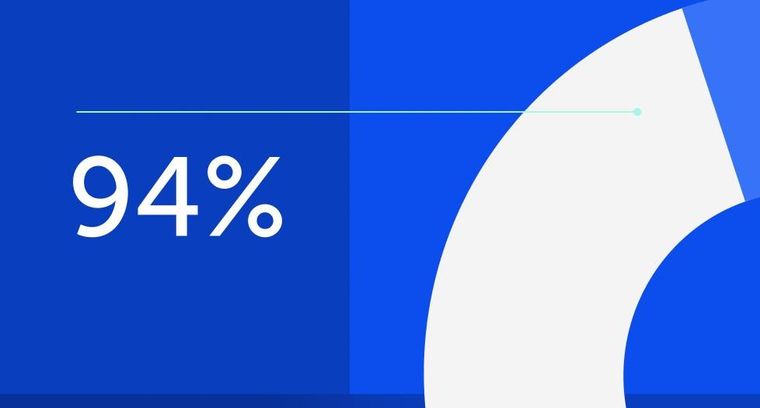
94% of researchers rate our articles as excellent or good
Learn more about the work of our research integrity team to safeguard the quality of each article we publish.
Find out more
ORIGINAL RESEARCH article
Front. Mar. Sci., 23 December 2021
Sec. Marine Molecular Biology and Ecology
Volume 8 - 2021 | https://doi.org/10.3389/fmars.2021.821019
This article is part of the Research TopicMarine Biological Materials: Functional Mechanisms and Environmental Impacts from the Molecular to the Macro-ScaleView all 11 articles
The increasing underwater noise generated by anthropogenic activities has been widely recognized as a significant and pervasive pollution in the marine environment. Marine mussels are a family of sessile bivalves that attach to solid surfaces via the byssal threads. They are widely distributed along worldwide coastal areas and are of great ecological and socio-economic importance. Studies found that anthropogenic noise negatively affected many biological processes and/or functions of marine organisms. However, to date, the potential impacts of anthropogenic noise on mussel byssal attachment remain unknown. Here, the thick shell mussels Mytilus coruscus were exposed to an ambient underwater condition (∼50 dB re 1 μPa) or the playbacks of pile-driving noise (∼70 or ∼100 dB re 1 μPa) for 10 days. Results showed that the noise significantly reduced the secretion of byssal threads (e.g., diameter and volume) and weakened their mechanical performances (e.g., strength, extensibility, breaking stress, toughness and failure location), leading to a 16.95–44.50% decrease in mussel byssal attachment strength. The noise also significantly down-regulated the genes expressions of seven structural proteins (e.g., mfp-1, mfp-2, mfp-3, mfp-6, preCOL-P, preCOL-NG, and preCOL-D) of byssal threads, probably mediating the weakened byssal attachment. Given the essential functions of strong byssal attachment, the findings demonstrate that the increasing underwater anthropogenic noise are posing a great threat to mussel population, mussel-bed community and mussel aquaculture industry. We thus suggest that future work is required to deepen our understanding of the impacts of anthropogenic noise on marine invertebrates, especially these with limited locomotion ability, like bivalves.
The underwater noise generated by anthropogenic activities such as shipping, oil and gas exploration, and the installation of renewable energy devices, has now been widely recognized as a significant and pervasive pollution in the marine environment (Duarte et al., 2021). Anthropogenic noise undoubtedly alters the acoustic signature of marine ecosystems and poses a great threat to marine organisms (Jerem and Mathews, 2021). What’s worse, given the growing levels of human activities, the underwater anthropogenic noise levels likely continue to increase in the foreseeable future (Duarte et al., 2021). Over the past decades, the potential impacts of anthropogenic noise on marine organisms have raised global attention (Peng et al., 2015; Popper et al., 2020). Studies demonstrate that a wide variety of biological processes and physiological functions of marine organisms can be negatively affected by anthropogenic noise, such as acoustic communication (Di Iorio and Clark, 2010; Alves et al., 2021), auditory sensitivity (Kastelein et al., 2018; Vieira et al., 2021), foraging behavior (Purser and Radford, 2011; Wale et al., 2013), antipredator behavior (Simpson et al., 2015; Kok et al., 2021), reproduction (de Jong et al., 2018; Smott et al., 2018) and embryonic development (de Soto et al., 2013; Nedelec et al., 2015). However, to date, most studies were conducted on marine mammals and fishes (Erbe et al., 2018; Popper and Hawkins, 2019), and the effects of anthropogenic noise on marine invertebrates, especially bivalves, received much less attention (Di Franco et al., 2020; Wale et al., 2021).
Marine bivalves, one class of benthic invertebrate, are able to detect substrate-borne and water-borne sound through contact with both substrate and surrounding water (Roberts et al., 2015; Vazzana et al., 2016; Charifi et al., 2017). Among marine invertebrates, bivalves are likely to be particularly vulnerable to anthropogenic noise, since their benthic habit and limited locomotion ability make them unable to escape from a noise area (Day et al., 2017; Vazzana et al., 2020). More importantly, marine bivalves are of great ecological and socio-economic importance, such as: (1) using as a source of human food, (2) increasing biodiversity of local marine ecosystem through creating habitats for other organisms, (3) improving seawater quality by filtering the water and removing particulates within, (4) attenuating global warming and ocean acidification through carbon fixation, and (5) mitigating ocean eutrophication through filtering large quantities of organic matter from the water column and the production of biodeposits (Vaughn and Hoellein, 2018; Suplicy, 2020; van der Schatte Olivier et al., 2020). Limited available studies show that anthropogenic noise disrupts the larval development of the New Zealand scallop Pecten novaezelandiae (de Soto et al., 2013), causes physiological harm and/or alters behavior in adult marine bivalves, including the razor clam Sinonovacula constricta (Peng et al., 2016), the Mediterranean mussel Mytilus galloprovincialis (Vazzana et al., 2020), the blood clam Tegillarca granosa (Shi et al., 2019), the blue mussel M. edulis (Wale et al., 2019), and the scallop P. fumatus (Day et al., 2017). However, there are species-specific and ontogenetic variations of noise-induced changes (Wale et al., 2021). Despite the current advance, the full extent to which anthropogenic noise affects marine bivalves is still poorly understood. Regarding their critical ecological roles and socio-economic importance, it is urgent to deepen our understanding of the effects of anthropogenic noise on marine bivalves.
Marine mussels are a family of sessile bivalve species that are widely distributed along worldwide coastal areas (Zhao et al., 2020). They attach to a wide array of substrata with their strong holdfast structure, the byssal threads (Waite, 1983). The byssal thread includes three parts: (1) the proximal region, which has a corrugated surface and is highly extensible; (2) the distal region, that is smoother, stiffer and approximately twice the length of the proximal region; and (3) the adhesive plaque, which adheres to the substrate (Bell and Gosline, 1996). Marine mussels can also attach to one another via byssal threads, and thereby form mussel aggregates or mussel beds, thus improving stability and protection against predation and environmental perturbations, increasing fertilization success, and providing habitats for other organisms (Borthagaray and Carranza, 2007; Liu et al., 2011; Christensen et al., 2015; Kong et al., 2019). A strong byssal attachment is thus essential for mussel survival, self-defense, reproduction and their ecological functions (Bandara et al., 2013; Sui et al., 2017; Shang et al., 2019). Hence, any potential impacts of anthropogenic noise on byssal attachment could have fundamental ecological implications for marine mussels and ecosystems. Given this, an understanding of noise effects in this regard is crucial. However, to date, the potential impacts of anthropogenic noise on mussel byssal attachment remain unknown.
Due to its ecological and economical importance, and wide distribution along the coastal areas of China, Korea and Japan (Li et al., 2015; Sui et al., 2015), the thick shell mussel M. coruscus was chosen and used as a testing organism in this study. A laboratory-based experiment was performed to investigate the effects of anthropogenic noise (specifically playback of pile-driving noise, an impulsive sound source) on byssal thread number, morphology and mechanical performance, and thereby the overall byssal attachment strength of mussel individual. Importantly, the expression levels of seven key genes encoding structural proteins of byssal threads, including four mussel foot proteins (mfp-1, mfp-2, mfp-3, and mfp-6) and three precursor collagen proteins (preCOL-P, preCOL-NG, and preCOL-D), were also determined to reveal the underlying molecular responses of marine mussels to anthropogenic noise.
Adult thick shell mussels M. coruscus with similar size (shell length of 102.1 ± 5.5 mm) were collected from Shengsi Islands, Zhejiang, China (30°73′ N and 122°45′ E) and directly transported to the Qingjiang Station of Zhejiang Mariculture Research Institute. The mussels were transported in air, mimicking the natural condition during low tide. Following carefully cleaning of epibionts without damaging their shells, the mussels were acclimated in filtered and UV-irradiated natural seawater (temperature of 22.5 ± 0.4°C, pH of 8.09 ± 0.06, and salinity of 23.8 ± 0.4 PSU) with continuous aeration for 2 weeks. The mussels were fed twice daily (e.g., at 08:00∼08:30 and 19:00∼19:30) with the microalgae Platymonas subcordiformis to satiation. Excess food and feces were removed daily with seawater change (e.g., at 18:00∼19:00), in which two thirds of the seawater was removed by siphoning, followed by refilling with filtered and UV-irradiated seawater.
Following acclimation, mussels were randomly assigned to a control and two anthropogenic noise input groups. The ambient condition without any additional anthropogenic sound input serviced as the control, while the underwater sound pressure levels of the two anthropogenic noise input groups were set at ∼70 and ∼100 dB re 1 μPa to mimic the underwater conditions with different degrees of anthropogenic sound, according to previous studies (Hildebrand, 2009; Slabbekoorn et al., 2010; Peng et al., 2016). The pile-driving sound recorded previously (Solan et al., 2016) was used as the source of anthropogenic noise input in this study. For the two anthropogenic noise input groups, mussels were exposed to playbacks of the pile-driving noise at respective underwater sound pressure levels. The experiment was performed with circular buckets (diameter of 60 cm, depth of 70 cm) filled with filtered and UV-irradiated natural seawater with a depth of 50 cm. For all groups, the circular bucket had a circular glass chamber (diameter of 20 cm, depth of 10 cm) centered on its floor, and each contained two individual mussels (Figure 1). The reason why each chamber contained only two mussels was to avoid the formation of mussel aggregate and its potential effects on sound propagation and the underwater sound pressure level.
Figure 1. Schematic diagram of the experimental setup and the positions where underwater acoustic conditions were determined.
A submersible loudspeaker (UW-30, Electro-Voice, Indiana, United States; frequency response 0.1–10 kHz; impedance 8 ohms; power-handling capacity 30 watts) was positioned in the center of the circular tank at a depth of 20 cm below the water surface and oriented toward the floor to generate the desired acoustic conditions (Figure 1). The submersible loudspeaker was driven using a power amplifier player (AV-296, SAST, Guangdong, China; power-handling capacity 150 watts). The action acoustic conditions were determined using the acoustics recording unit, which included a bioacoustics recorder (Song Meter SM2+, Wildlife Acoustics, MA, United States; 96 kHz sampling rate), and a calibrated omni-directional hydrophone (HIT-96-MIN, High Tech; Long Beach, Mississippi, United States; flat frequency response 0.02–30 kHz, sensitivity −164 dB re 1 V/μPa). To test whether there were any variations of the underwater sound pressure levels at different positions, the hydrophone was placed near the bottom of the circular bucket at 5 cm, 15 cm, and 25 cm away from the center (Figure 1). Acquired acoustic data were analyzed with the Soundscape Analysis Software SACS V1.0 (Register number: 2014SR216788), and underwater sound pressure levels were obtained following the described method (Peng et al., 2016). During the entire experimental period, mussels were fed twice daily, and seawater was continuously aerated and renewed daily as described above. The seawater parameters, including temperature, pH and salinity, were kept as described above. Since the control and two anthropogenic noise input groups were conducted simultaneously in a laboratory, the experimental circular buckets were equipped with anti-vibration shielding to avoid the potential effects of the high sound pressure level noise on the acoustic conditions of other groups. The experiment plan was replicated five times with different individuals for each time and under identical experimental conditions. The experiment lasted for 10 days, and no animal mortality was observed.
Following exposure, one of the two mussels in the circular glass chamber was randomly selected, dissected and immediately frozen in liquid nitrogen for further gene expression analysis. After carefully removing the existing byssal threads without damaging foot organ, the other mussel was allowed to regrow for 24 h to secret new byssal threads. During the 24 h incubation, the experimental conditions were identical with those of the 10-day experimental period.
After regrowing for 24 h, the full-length byssal threads of mussels were carefully collected according to the method described previously (Zhao et al., 2017). Briefly, newly secreted byssal threads were cautiously removed from the substrate one by one. Mussels were dissected by severing the adductor muscles. The full-length byssal threads were then carefully excised at their attachment points to foot. After collection, the number of newly secreted byssal threads per mussel was manually counted. The length (mm) of each byssal thread was determined using a digital Vernier caliper with a precision of 0.01 mm. Digital image of each byssal thread was obtained using a CCD camera mounted on a microscope (BX53, Olympus, Japan). Diameters of three independent locations of the byssal thread were measured through image analysis using the free-access software ImageJ Version 1.51j81, and the mean value of them was used as the diameter (d; μm) of the byssal thread. Byssal thread was assumed to be cylindrical (Bell and Gosline, 1996), and the cross-sectional area (mm2) of each byssal thread was calculated from the obtained diameter using the equation: cross-sectional area = π × (d/2)2. The volume (mm3) of each byssal thread was calculated by multiplying the cross-sectional area by the length.
After quantitative analysis, mechanical properties of byssal thread were determined using a universal testing machine (AGS-J, Shimadzu, Japan) following the methods described previously (Zhao et al., 2017). The adhesive plaque was glued to a metal stub secured in the lower platform of the testing machine, while the proximal end of byssal thread was sandwiched between cardboard and further clamped at the upper grip of the testing machine. Tensile test of byssal thread was conducted in air at room temperature at a loading rate of 10 mm/min until failure occurred, mimicking the natural air exposure condition during low tide.
The breaking force (N), also named thread strength, was determined as the force required to break a single byssal thread. The breaking strain (%), also called thread extensibility was measured as the extension at failure, divided by the unstressed thread length. The breaking stress (N/mm2) was calculated as the value of breaking force divided by cross-sectional area. The toughness (J/cm3) was measured as the energy absorbed per unit volume until thread failure occurred. The location of failure (e.g., proximal, distal or adhesive plaque) was noted, and failure occurrence at each location was expressed as the percentage of the number of threads tested. When failure occurred at the grip, the corresponding data were discarded from analysis to avoid underestimating the actual mechanical properties (Bell and Gosline, 1996; Moeser and Carrington, 2006).
According to previous studies (Bell and Gosline, 1996; Zhao et al., 2017; Shi et al., 2020), the overall mussel attachment strength was estimated as the potential maximal attachment strength (N) with the formula: attachment = average thread strength × thread number. Prior to the estimation, the average thread strength was calculated as the mean value of all thread strengths for a given mussel individual.
Following dissection, the foot tissue was immediately frozen in liquid nitrogen and stored at −80°C upon use. Total RNA was extracted using TRIzol Reagent (Invitrogen, 15596018) following the manufacturer’s protocol. DNA contamination in the RNA sample was removed by treating with DNase I (Invitrogen, 18047019). The RNA quality was checked by 1.0% formaldehyde-denatured agarose gel electrophoresis. The RNA concentration was quantified using a NanoDrop 1000 spectrophotometer (Thermo Fisher Scientific). The cDNA was synthesized with the high-quality total RNA using the M-MLV First Strand Kit (Invitrogen, C28025-032) according to the manufacturer’s protocol. Real-time PCR was conducted using a CFX96 Real-Time System (Bio-Rad, United States) with a total reaction volume of 10 μL containing 5 μL of SsoFast EvaGreen Supermix (Bio-Rad, 1725201AP), 0.5 μL of each primer (10 μM), 2 μL of cDNA template and 2 μL of double-distilled water. The cycle conditions were 95°C for 5 min, 40 cycles of 94°C for 20 s, 61°C for 20 s, and 72°C for 20 s. The specificity of each amplification reaction was verified by analyzing the melting curve. The relative gene expression level was calculated using the 2–ΔΔCt method (Livak and Schmittgen, 2001) with the 18S rRNA gene as the internal reference. The expression of 18S rRNA has been verified to be stable under ambient and different noise exposure conditions. The gene-specific primers using in this study were listed in Table 1.
One-way ANOVAs were performed to show the effects of position (i.e., distance to the center of the concentric circle vertically below the sound source) on the underwater sound pressure level, and the effects of anthropogenic noise on byssal thread production, morphology, mechanical parameters and gene expression levels. Post-hoc Tukey’s multiple tests were conducted to compare differences among groups. Prior to analysis, homogeneity of variance and normality of data were verified with the Levene’s test and Shapiro-Wilk’s tests, respectively. Chi-square test was performed to compare failure occurrences at each location. Data are presented as the mean ± SD, and a p-value less than 0.05 was considered to indicate statistically significance.
One-way ANOVAs showed that the distance to the center of the concentric circle vertically below the sound source had no significant effect on the underwater sound pressure levels near the bottom of the circular bucket, indicating no significant difference between the underwater sound pressure levels at 5, 15, and 25 cm away from the center (Table 2). As shown in Table 2, the underwater sound pressure level of the control group was ∼55 dB re 1 μPa that was much lower than those of the two anthropogenic noise input groups. Additionally, the actual underwater sound pressure levels of the two anthropogenic noise input groups were generally consistent with the nominal values that set at ∼70 and ∼100 dB re 1 μPa. Given this situation, experimental results were therefore analyzed and reported with nominal underwater sound pressure levels.
Table 2. The underwater sound pressure levels (dB re 1 μPa) near the bottom of the circular bucket at 5, 15, and 25 cm away from the center of the concentric circle vertically below the sound source (n = 10 per distance, mean ± SD).
The average number of newly secreted byssal threads per mussel was slightly reduced from 46.6 (100%) to 43.4 (93.13%) and 41 (87.98%) after the 10-day exposure to the 70 and 100 dB re 1 μPa anthropogenic noise, but no significant effect was observed [F(2, 12) = 3.47, p = 0.06] (Figure 2A). The length of newly secreted byssal thread was also not significantly affected after the 10-day exposure [F(2, 12) = 2.9, p = 0.09] (Figure 2B). However, a significant effect of anthropogenic noise on the diameter of the byssal thread was detected [F(2, 12) = 6.71, p = 0.01]. The diameter of mussels exposed to 70 and 100 dB re 1 μPa noise decreased to approximately 97.18 and 93.50% of that of the control, respectively (Figure 2C). Compared with the control, the average volume of the byssal thread was significantly declined to about 87.09 and 80.40% of that of the control upon 70 and 100 dB re 1 μPa noise exposure, respectively [F(2, 12) = 11.22, p = 0.002] (Figure 2D).
Figure 2. Effects of anthropogenic noise exposure on the (A) number, (B) thread length, (C) thread diameter, and (D) volume of byssal threads newly produced by the thick shell mussel Mytilus coruscus (n = 5 per group, mean ± SD). Means not sharing the same superscript are significantly different (Tukey’s HSD, p < 0.05).
The breaking force of byssal thread was significantly reduced for mussels exposed to the 70 and 100 dB re 1 μPa anthropogenic noise [F(2, 12) = 66.67, p = 3.17 × 10–7], which were approximately 89.27 and 63.41% of that of the control (Figure 3A). The breaking stress of byssal thread was significantly decreased after the 10-day exposure [F(2, 12) = 21.62, p = 1.05 × 10–4]. Compared to control, the breaking stress was declined by about 5.57 and 27.84% for mussels exposed to 70 and 100 dB re 1 μPa anthropogenic noise, respectively (Figure 3B). The breaking strain of byssal thread was also significantly reduced by anthropogenic noise exposure [F(2, 12) = 39.58, p = 5.20 × 10–6]. The breaking strain of mussels exposed to 70 and 100 dB re 1 μPa anthropogenic noise were significantly decreased to approximately 80.27 and 65.93% of that of the control, respectively (Figure 3C). Similarly, the toughness of byssal thread was significantly affected by anthropogenic noise exposure as well [F(2, 12) = 75.70, p = 1.57 × 10–7]. The toughness of the 70 and 100 dB re 1 μPa anthropogenic noise exposure groups were remarkably declined to approximately 76.06 and 48.07% of that of the control, respectively (Figure 3D).
Figure 3. Effects of anthropogenic noise exposure on the (A) breaking force, (B) breaking stress, (C) breaking strain, and (D) toughness of byssal threads newly produced by the thick shell mussel Mytilus coruscus (n = 5 per group, mean ± SD). Means not sharing the same superscript are significantly different (Tukey’s HSD, p < 0.05).
As shown in the Figure 4, the thread failure occurred at either the proximal region or the adhesive plaque. The occurrence of thread failure located in the adhesive plaque was significantly increased by anthropogenic noise exposure (χ2 = 35.92, df = 2, p < 0.0001). For the control group, only 24% thread failure occurred in the adhesive plaque, while it was increased to approximately 34 and 64% for the 70 and 100 dB re 1 μPa anthropogenic noise exposure groups, respectively.
Figure 4. Effects of anthropogenic noise exposure on failure occurrence (%) at each location of byssal thread newly produced by the thick shell mussel Mytilus coruscus (n = 5 per group, χ2 = 35.92, df = 2, p < 0.0001).
The overall mussel attachment strength was significantly weakened by anthropogenic noise exposure [F(2, 12) = 64.31, p = 3.86 × 10–7] (Figure 5). Compared to the control, the potential maximal attachment strength of mussels treated with 70 and 100 dB re 1 μPa anthropogenic noise was significantly decreased by 16.95 and 44.50%, respectively.
Figure 5. Effects of anthropogenic noise exposure on the potential maximal attachment strength of the thick shell mussel Mytilus coruscus (n = 5 per group, mean ± SD). Means not sharing the same superscript are significantly different (Tukey’s HSD, p < 0.05).
One-way ANOVAs showed that the expression levels of mfp-1, mfp-2, mfp-3, and mfp-6 were significantly affected by anthropogenic noise exposure (Figures 6A–D). Compared to the control, the expression levels of mfp-1 were significantly decreased by 34.81 and 46.28% for mussels exposed to 70 and 100 dB re 1 μPa anthropogenic noise, respectively (Figure 6A). Although the fold-change varied, the expression patterns of mfp-2, mfp-3, and mfp-6 were similar to that of mfp-1, showing the down-regulation upon anthropogenic noise exposure (Figures 6B–D). Significant effects of anthropogenic noise on the expression levels of preCOL-P, preCOL-NG, and preCOL-D were observed as well (Figures 6E–G). The expression levels of preCOL-P of mussels exposed to 70 and 100 dB re 1 μPa anthropogenic noise were significantly reduced to 48.81 and 28.95% of that of control, respectively (Figure 6E). Similarly, for mussels treated by 70 and 100 dB re 1 μPa anthropogenic noise, the expression levels of preCOL-NG were, respectively, decreased to 93.69 and 48.47%, and the expression levels of preCOL-D were, respectively, down-regulated to 68.16 and 50.07%, compared to those of control (Figures 6F,G).
Figure 6. Effects of anthropogenic noise exposure on the expression levels of genes encoding structural proteins of byssal threads, including (A) mfp-1, (B) mfp-2, (C) mfp-3, (D) mfp-6, (E) preCOL-P, (F) preCOL-NG and (G) preCOL-D in the thick shell mussel Mytilus coruscus (n = 5 per group, mean ± SD). Means not sharing the same superscript are significantly different (Tukey’s HSD, p < 0.05).
Our results suggest that exposure to playbacks of the pile-driving noise had significant negative effects on byssal thread production and mechanical performances of the thick shell mussel M. coruscus, leading to reductions in the diameter, average volume, strength (i.e., breaking force), extensibility (i.e., breaking strain), breaking stress and toughness of individual byssal thread. Additionally, byssal threads of control mussels were commonly broken at the proximal region, while those of noise-treated mussels were largely fractured at the adhesive plaque, indicating the weakest part of byssal thread shifted from the proximal region to the adhesive plaque upon exposure to playbacks of the pile-driving noise. These noise-induced changes led to a 16.95–44.50% decrease in the overall byssal attachment strength, greatly limiting mussels’ ability to attach firmly on substratum. On the one hand, the weakened byssal attachment would undoubtedly increase dislodgement risk of mussel individuals in natural habitats and commercial suspension cultures (O’Donnell et al., 2013), and subsequently make them more vulnerable to predators and environmental turbulence (e.g., waves) at the individual level. On the other hand, the weakened byssal attachment might also hinder the formation and maintenance of mussel beds, and in turn impair the recruitment, anti-predation capability and ecological functions of mussel population. We therefore conclude that anthropogenic noise (e.g., the pile-driving noise) has negative impacts on both mussel individuals and population through hampering byssal attachment, which in turn pose a great threat to mussel bed communities and the mussel aquaculture industry.
The noise-induced decline in byssal thread production (i.e., the decrease in the diameter and volume of byssal thread, and the down-regulation of genes encoding structural proteins of byssal thread) might be due to limited energy availability. It has been shown that the energetic cost of byssal thread production is substantial, requiring 8–10% of a mussel’s total energy expenditure (Hawkins and Bayne, 1985; Lurman et al., 2013; Roberts et al., 2021). However, studies have suggested that anthropogenic noise adversely affected the feeding behavior and metabolism activity of marine bivalves, including mussels, which seems to result in energy deficiency (Peng et al., 2016; Spiga et al., 2016; Shi et al., 2019; Wale et al., 2019; Vazzana et al., 2020). For example, exposure to ship noise playbacks led to a 12% reduction in oxygen consumption and an 84% decrease in filtration rate of the blue mussel M. edulis (Wale et al., 2019). Under this circumstance, energy availability could be limited and mussels must allocate a relatively few energy to biological processes that are less critical for individual survival (Lachance et al., 2011; Shang et al., 2021). We suggest that upon exposure to the pile-driving noise, mussels tended to limit the energy allocation to byssal attachment, thus leading to the down-regulation of genes expression levels of byssal proteins and the decrease in byssal thread diameter and volume of the thick shell mussel M. corucus in this study. It is worth noting that there was no significant alteration in byssal thread number and length. However, these were not opposite to the results described above. As known, to attach firmly on the substrate, mussel individuals must produce enough byssal threads angled away from the shell to the ideal places where they want to anchor (Bell and Gosline, 1996). However, the down-regulation of genes expression levels of byssal proteins and the decrease in thread volume suggest that less byssal proteins were synthesized, indicating there was insufficient materials for mussel individuals to produce byssal threads like before. Under this circumstance, decreasing thread diameter but keeping thread number and length should be an adaptive strategy to meet the lowest requirements for mussel attachment.
The noise-induced reduction in mechanical performances of byssal thread can be attributed to a series of reasons. Morphological, the decrease in mechanical properties could be firstly owing to the morphological alterations of byssal thread. For example, the reduction in strength (i.e., breaking force), extensibility (i.e., breaking strain) and toughness might result from the thinner individual byssal thread. However, given the negative correlation between breaking stress and thread diameter, breaking stress was still decreased with the thread diameter reducing. This suggests that except morphological alterations, there must be other reasons contributing to the reduction in mechanical performances of byssal threads. Our results show that the mRNA levels of genes encoding structural proteins of byssal threads, including four mussel foot proteins (mfp-1, mfp-2, mfp-3, and mfp-6) and three precursor collagen proteins (preCOL-P, preCOL-NG, and preCOL-D), were significantly down-regulated upon exposure to the pile-driving noise. As known, the down-regulation of genes expression levels may not guarantee the reduction in translation of byssal proteins, but taken together with the decrease in thread volume, we suggest that levels of the four mussel foot proteins (mfp-1, mfp-2, mfp-3, and mfp-6) and the three precursor collagen proteins (preCOL-P, preCOL-NG, and preCOL-D) were, to some extent, down-regulated. It has been proven that the protective cuticle of byssal thread is comprised solely of mfp-1, while the inner collagenous core is mainly made of preCOL-P, preCOL-NG, and preCOL-D (∼81% of the dry weight) (Silverman and Roberto, 2010). The other identified mussel foot proteins, including mfp-2, mfp-3, and mfp-6, are confined exclusively to the adhesive plaque (Lee et al., 2011). The preCOL-P is essential for the remarkable extensibility at the proximal region, and the preCOL-D provides the excellent stiffness property at the distal region of the byssal thread (Bandara et al., 2013). The preCOL-NG, distributed uniformly throughout the proximal and distal region, is considered to mediate the interaction between preCOL-P and preCOL-D, and thereby combines the remarkable extensibility and high strength properties of byssal thread (Bandara et al., 2013). These evidences show that the four mussel foot proteins (mfp-1, mfp-2, mfp-3, and mfp-6) and the three precursor collagen proteins (preCOL-P, preCOL-NG, and preCOL-D) are critical for the mechanical performances of byssal threads. Regarding this, the down-regulation of structural proteins of byssal threads should be one reason underlying the weakened mechanical properties and the shift of the weakest part of byssal thread.
It has been revealed that the mechanoreceptors within mussel foot participate in byssal threads secretion and adhesion (LaCourse and Northrop, 1978; Amini et al., 2017). Briefly, once mussel foot probes an ideal substrate, the mechanoreceptors and their downstream signal transduction pathways would be activated. The mussel then starts to fabricate byssal threads and subsequently attaches on the substrate. Importantly, the mechanoreceptors are also highly sensitive to mechanical waterborne vibration or acoustic particle motion and thus essential for mussel sound detection (Roberts et al., 2015; Vazzana et al., 2016). These suggest that mechanoreceptors mediate both mussel byssal attachment and sound detection. Thus, it is reasonable to infer that there might be any cross-talk between the two signal transduction pathways. In this way, the biological function of the mechanoreceptors in byssal attachment may be interfered by the coexisting noise. In turn, the process of byssal threads secretion and adhesion was disrupted, finally leading to the observed reduction in byssal thread production and mechanical performances in this study. In a word, the dysfunction, if any, of the mechanoreceptors might be one reason mediating the noise-induced alteration in mussel byssal attachment. Of course, empirical evidences are required to validate this assumption.
In conclusion, this study found that 10-day continuous exposure to playbacks of the pile-driving noise significantly weakened gene expression of byssal proteins, secretion of byssal threads and their mechanical performances, which eventually led to a 16.95–44.50% decrease in the overall mussel byssal attachment strength (Figure 7). Given the essential functions of strong byssal attachment, these findings suggest that the increasing underwater anthropogenic noise is posing a great threat to mussel population, mussel-bed community and mussel aquaculture industry. We thus suggest that future work is needed to enhance our understanding of the impacts of anthropogenic noise on marine invertebrates, especially these with limited locomotion ability, like bivalves.
Figure 7. Schematic diagram showing the main findings and implications of this study. Exposure to playbacks of the pile-driving noise significantly weakened gene expression of byssal proteins, secretion of byssal threads and their mechanical performances, which eventually led to a 16.95–44.50% decrease in the overall mussel byssal attachment strength. In conclusion, anthropogenic noise (e.g., the pile-driving noise) has negative impacts on both mussel individuals and population through hampering byssal attachment, in turn posing a great threat to mussel-bed community and mussel aquaculture industry.
The original contributions presented in the study are included in the article/supplementary material, further inquiries can be directed to the corresponding author/s.
XZ and GL conceived and designed this study. XZ and SS performed the whole experiments and collected the data. XZ wrote the manuscript, while other authors reviewed and revised the manuscript. GL provided oversight of the project. All authors gave final approval for publication and contributed to data analysis and interpretation.
This work was funded by the Shandong Provincial Natural Science Foundation (No. ZR2020QC209), the National Natural Science Foundation of China (No. 42106195), the Central Public-interest Scientific Institution Basal Research Fund, CAFS (No. 2020TD12), and the Central Public-interest Scientific Institution Basal Research Fund, YSFRI, CAFS (No. 20603022021005).
The authors declare that the research was conducted in the absence of any commercial or financial relationships that could be construed as a potential conflict of interest.
All claims expressed in this article are solely those of the authors and do not necessarily represent those of their affiliated organizations, or those of the publisher, the editors and the reviewers. Any product that may be evaluated in this article, or claim that may be made by its manufacturer, is not guaranteed or endorsed by the publisher.
Alves, D., Vieira, M., Amorim, M. C. P., and Fonseca, P. J. (2021). Boat noise interferes with lusitanian toadfish acoustic communication. J. Exp. Biol. 224:jeb234849. doi: 10.1242/jeb.234849
Amini, S., Kolle, S., Petrone, L., Ahanotu, O., Sunny, S., Sutanto, C. N., et al. (2017). Preventing mussel adhesion using lubricant-infused materials. Science 357, 668–673. doi: 10.1126/science.aai8977
Bandara, N., Zeng, H., and Wu, J. (2013). Marine mussel adhesion: biochemistry, mechanisms, and biomimetics. J. Adhes. Sci. Technol. 27, 2139–2162. doi: 10.1080/01694243.2012.697703
Bell, E. C., and Gosline, J. M. (1996). Mechanical design of mussel byssus: material yield enhances attachment strength. J. Exp. Biol. 199, 1005–1017. doi: 10.1242/jeb.199.4.1005
Borthagaray, A. I., and Carranza, A. (2007). Mussels as ecosystem engineers: their contribution to species richness in a rocky littoral community. Acta Oecol. 31, 243–250. doi: 10.1016/j.actao.2006.10.008
Charifi, M., Sow, M., Ciret, P., Benomar, S., and Massabuau, J.-C. (2017). The sense of hearing in the Pacific oyster, Magallana gigas. PLoS One 12:e0185353. doi: 10.1371/journal.pone.0185353
Christensen, H. T., Dolmer, P., Hansen, B. W., Holmer, M., Kristensen, L. D., Poulsen, L. K., et al. (2015). Aggregation and attachment responses of blue mussels, Mytilus edulis—impact of substrate composition, time scale and source of mussel seed. Aquaculture 435, 245–251. doi: 10.1016/j.aquaculture.2014.09.043
Day, R. D., McCauley, R. D., Fitzgibbon, Q. P., Hartmann, K., and Semmens, J. M. (2017). Exposure to seismic air gun signals causes physiological harm and alters behavior in the scallop Pecten fumatus. Proc. Natl. Acad. Sci. U.S.A. 114, E8537–E8546. doi: 10.1073/pnas.1700564114
de Jong, K., Amorim, M. C. P., Fonseca, P. J., Fox, C. J., and Heubel, K. U. (2018). Noise can affect acoustic communication and subsequent spawning success in fish. Environ. Pollut. 237, 814–823. doi: 10.1016/j.envpol.2017.11.003
de Soto, N. A., Delorme, N., Atkins, J., Howard, S., Williams, J., and Johnson, M. (2013). Anthropogenic noise causes body malformations and delays development in marine larvae. Sci. Rep. 3:2831. doi: 10.1038/srep02831
Di Franco, E., Pierson, P., Di Iorio, L., Calò, A., Cottalorda, J. M., Derijard, B., et al. (2020). Effects of marine noise pollution on mediterranean fishes and invertebrates: a review. Mar. Pollut. Bull. 159:111450. doi: 10.1016/j.marpolbul.2020.111450
Di Iorio, L., and Clark, C. W. (2010). Exposure to seismic survey alters blue whale acoustic communication. Biol. Lett. 6, 51–54. doi: 10.1098/rsbl.2009.0651
Duarte, C. M., Chapuis, L., Collin, S. P., Costa, D. P., Devassy, R. P., Eguiluz, V. M., et al. (2021). The soundscape of the Anthropocene ocean. Science 371:eaba4658. doi: 10.1126/science.aba4658
Erbe, C., Dunlop, R., and Dolman, S. (2018). “Effects of noise on marine mammals,” in Effects of Anthropogenic Noise on Animals, eds H. Slabbekoorn, R. J. Dooling, A. N. Popper, and R. R. Fay (New York, NY: Springer), 277–309.
Hawkins, A. J. S., and Bayne, B. L. (1985). Seasonal variation in the relative utilization of carbon and nitrogen by the mussel mytilus edulis: budgets, conversion efficiencies and maintenance requirements. Mar. Ecol. Prog. Ser. 25, 181–188. doi: 10.3354/meps025181
Hildebrand, J. A. (2009). Anthropogenic and natural sources of ambient noise in the ocean. Mar. Ecol. Prog. Ser. 395, 5–20. doi: 10.3354/meps08353
Jerem, P., and Mathews, F. (2021). Trends and knowledge gaps in field research investigating effects of anthropogenic noise. Conserv. Biol. 35, 115–129. doi: 10.1111/cobi.13510
Kastelein, R. A., Helder-Hoek, L., Kommeren, A., Covi, J., and Gransier, R. (2018). Effect of pile-driving sounds on harbor seal (Phoca vitulina) hearing. J. Acoust. Soc. Am. 143, 3583–3594. doi: 10.1121/1.5040493
Kok, A., Hulten, D., Timmerman, K., Lankhorst, J., Visser, F., and Slabbekoorn, H. (2021). Interacting effects of short-term and long-term noise exposure on antipredator behaviour in sand gobies. Anim. Behav. 172, 93–102. doi: 10.1016/j.anbehav.2020.12.001
Kong, H., Clements, J. C., Dupont, S., Wang, T., Huang, X., Shang, Y., et al. (2019). Seawater acidification and temperature modulate anti-predator defenses in two co-existing Mytilus species. Mar. Pollut. Bull. 145, 118–125. doi: 10.1016/j.marpolbul.2019.05.040
Lachance, A.-A., Hennebicq, R., Myrand, B., Sévigny, J.-M., Kraffe, E., Marty, Y., et al. (2011). Biochemical and genetic characteristics of suspension-cultured mussels (Mytilus edulis) in relation to byssal thread production and losses by fall-off. Aquat. Living Resour. 24, 283–293. doi: 10.1051/alr/2011115
LaCourse, J. R., and Northrop, R. B. (1978). A preliminary study of mechanoreceptors within the anterior byssus retractor muscle of Mytilus edulis L. Biol. Bull. 155, 161–168. doi: 10.2307/1540873
Lee, B. P., Messersmith, P. B., Israelachvili, J. N., and Waite, J. H. (2011). Mussel-inspired adhesives and coatings. Annu. Rev. Mater. Res. 41, 99–132. doi: 10.1146/annurev-matsci-062910-100429
Li, L., Lu, W., Sui, Y., Wang, Y., Gul, Y., and Dupont, S. (2015). Conflicting effects of predator cue and ocean acidification on the mussel Mytilus coruscus byssus production. J. Shellfish Res. 34, 393–400. doi: 10.2983/035.034.0222
Liu, G., Stapleton, E., Innes, D., and Thompson, R. (2011). Aggregational behavior of the blue mussels Mytilus edulis and Mytilus trossulus: a potential pre-zygotic reproductive isolation mechanism. Mar. Ecol. 32, 480–487. doi: 10.1111/j.1439-0485.2011.00446.x
Livak, K. J., and Schmittgen, T. D. (2001). Analysis of relative gene expression data using real-time quantitative PCR and the 2−ΔΔCt method. Methods 25, 402–408. doi: 10.1006/meth.2001.1262
Lurman, G. J., Hilton, Z., and Ragg, N. L. C. (2013). Energetics of byssus attachment and feeding in the green-lipped mussel Perna canaliculus. Biol. Bull. 224, 79–88. doi: 10.1086/BBLv224n2p79
Moeser, G. M., and Carrington, E. (2006). Seasonal variation in mussel byssal thread mechanics. J. Exp. Biol. 209, 1996–2003. doi: 10.1242/jeb.02234
Nedelec, S. L., Simpson, S. D., Morley, E. L., Nedelec, B., and Radford, A. N. (2015). Impacts of regular and random noise on the behaviour, growth and development of larval Atlantic cod (Gadus morhua). Proc. R. Soc. B 282:20151943. doi: 10.1098/rspb.2015.1943
O’Donnell, M. J., George, M. N., and Carrington, E. (2013). Mussel byssus attachment weakened by ocean acidification. Nat. Clim. Change 3, 587–590. doi: 10.1038/nclimate1846
Peng, C., Zhao, X., and Liu, G. (2015). Noise in the sea and its impacts on marine organisms. Int. J. Env. Res. Public Health 12, 12304–12323. doi: 10.3390/ijerph121012304
Peng, C., Zhao, X., Liu, S., Shi, W., Han, Y., Guo, C., et al. (2016). Effects of anthropogenic sound on digging behavior, metabolism, Ca2 +/Mg2 + ATPase activity, and metabolism-related gene expression of the bivalve Sinonovacula constricta. Sci. Rep. 6:24266. doi: 10.1038/srep24266
Popper, A. N., and Hawkins, A. D. (2019). An overview of fish bioacoustics and the impacts of anthropogenic sounds on fishes. J. Fish Biol. 94, 692–713. doi: 10.1111/jfb.13948
Popper, A. N., Hawkins, A. D., and Thomsen, F. (2020). Taking the Animals’ perspective regarding anthropogenic underwater sound. Trends Ecol. Evol. 35, 787–794. doi: 10.1016/j.tree.2020.05.002
Purser, J., and Radford, A. N. (2011). Acoustic noise induces attention shifts and reduces foraging performance in three-spined sticklebacks (Gasterosteus aculeatus). PLoS One 6:e17478. doi: 10.1371/journal.pone.0017478
Roberts, E. A., Newcomb, L. A., McCartha, M. M., Harrington, K. J., LaFramboise, S. A., Carrington, E., et al. (2021). Resource allocation to a structural biomaterial: induced production of byssal threads decreases growth of a marine mussel. Funct. Ecol. 35, 1222–1239. doi: 10.1111/1365-2435.13788
Roberts, L., Cheesman, S., Breithaupt, T., and Elliott, M. (2015). Sensitivity of the mussel Mytilus edulis to substrate-borne vibration in relation to anthropogenically generated noise. Mar. Ecol. Prog. Ser. 538, 185–195. doi: 10.3354/meps11468
Shang, Y., Gu, H., Chang, X., Li, S., Sokolova, I., Fang, J. K. H., et al. (2021). Microplastics and food shortage impair the byssal attachment of thick-shelled mussel Mytilus coruscus. Mar. Environ. Res. 171:105455. doi: 10.1016/j.marenvres.2021.105455
Shang, Y., Wang, X., Kong, H., Huang, W., Hu, M., and Wang, Y. (2019). Nano-ZnO impairs anti-predation capacity of marine mussels under seawater acidification. J. Hazard. Mater. 371, 521–528. doi: 10.1016/j.jhazmat.2019.02.072
Shi, W., Guan, X., Sun, S., Han, Y., Du, X., Tang, Y., et al. (2020). Nanoparticles decrease the byssal attachment strength of the thick shell mussel Mytilus coruscus. Chemosphere 257:127200. doi: 10.1016/j.chemosphere.2020.127200
Shi, W., Han, Y., Guan, X., Rong, J., Du, X., Zha, S., et al. (2019). Anthropogenic noise aggravates the toxicity of cadmium on some physiological characteristics of the blood clam Tegillarca granosa. Front. Physiol. 10:377. doi: 10.3389/fphys.2019.00377
Silverman, H. G., and Roberto, F. F. (2010). “Byssus formation in Mytilus,” in Biological Adhesive Systems, eds J. von Byern and I. Grunwald (Vienna: Springer), 273–283.
Simpson, S. D., Purser, J., and Radford, A. N. (2015). Anthropogenic noise compromises antipredator behaviour in European eels. Glob. Change Biol. 21, 586–593. doi: 10.1111/gcb.12685
Slabbekoorn, H., Bouton, N., van Opzeeland, I., Coers, A., ten Cate, C., and Popper, A. N. (2010). A noisy spring: the impact of globally rising underwater sound levels on fish. Trends Ecol. Evol. 25, 419–427. doi: 10.1016/j.tree.2010.04.005
Smott, S., Monczak, A., Miller, M. E., and Montie, E. W. (2018). Boat noise in an estuarine soundscapea potential risk on the acoustic communication and reproduction of soniferous fish in the may river, South Carolina. Mar. Pollut. Bull. 133, 246–260. doi: 10.1016/j.marpolbul.2018.05.016
Solan, M., Hauton, C., Godbold, J. A., Wood, C. L., Leighton, T. G., and White, P. (2016). Anthropogenic sources of underwater sound can modify how sediment-dwelling invertebrates mediate ecosystem properties. Sci. Rep. 6:20540. doi: 10.1038/srep20540
Spiga, I., Caldwell, G. S., and Bruintjes, R. (2016). Influence of pile driving on the clearance rate of the blue mussel, Mytilus edulis (L.). Proc. Mtgs. Acoust. 27:040005. doi: 10.1121/2.0000277
Sui, Y., Hu, M., Huang, X., Wang, Y., and Lu, W. (2015). Anti-predatory responses of the thick shell mussel Mytilus coruscus exposed to seawater acidification and hypoxia. Mar. Environ. Res. 109, 159–167. doi: 10.1016/j.marenvres.2015.07.008
Sui, Y., Liu, Y., Zhao, X., Dupont, S., Hu, M., Wu, F., et al. (2017). Defense responses to short-term hypoxia and seawater acidification in the thick shell mussel Mytilus coruscus. Front. Physiol. 8:145. doi: 10.3389/fphys.2017.00145
Suplicy, F. M. (2020). A review of the multiple benefits of mussel farming. Rev. Aquacult. 12, 204–223. doi: 10.1111/raq.12313
van der Schatte Olivier, A., Jones, L., Vay, L. L., Christie, M., Wilson, J., and Malham, S. K. (2020). A global review of the ecosystem services provided by bivalve aquaculture. Rev. Aquacult. 12, 3–25. doi: 10.1111/raq.12301
Vaughn, C. C., and Hoellein, T. J. (2018). Bivalve impacts in freshwater and marine ecosystems. Annu. Rev. Ecol., Evol. 49, 183–208. doi: 10.1146/annurev-ecolsys-110617-062703
Vazzana, M., Celi, M., Maricchiolo, G., Genovese, L., Corrias, V., Quinci, E. M., et al. (2016). Are mussels able to distinguish underwater sounds? Assessment of the reactions of Mytilus galloprovincialis after exposure to lab-generated acoustic signals. Comput. Biochem. Physiol. Mol. Integr. Physiol. 201, 61–70. doi: 10.1016/j.cbpa.2016.06.029
Vazzana, M., Ceraulo, M., Mauro, M., Papale, E., Dioguardi, M., Mazzola, S., et al. (2020). Effects of acoustic stimulation on biochemical parameters in the digestive gland of mediterranean mussel Mytilus galloprovincialis (Lamarck, 1819). J. Acoust. Soc. Am. 147, 2414–2422. doi: 10.1121/10.0001034
Vieira, M., Beauchaud, M., Amorim, M. C. P., and Fonseca, P. J. (2021). Boat noise affects meagre (Argyrosomus regius) hearing and vocal behaviour. Mar. Pollut. Bull. 172:112824. doi: 10.1016/j.marpolbul.2021.112824
Waite, J. H. (1983). Adhesion in byssally attached bivalves. Biol. Rev. 58, 209–231. doi: 10.1111/j.1469-185X.1983.tb00387.x
Wale, M. A., Briers, R. A., and Diele, K. (2021). Marine invertebrate anthropogenic noise research – Trends in methods and future directions. Mar. Pollut. Bull. 173:112958. doi: 10.1016/j.marpolbul.2021.112958
Wale, M. A., Briers, R. A., Hartl, M. G. J., Bryson, D., and Diele, K. (2019). From DNA to ecological performance: effects of anthropogenic noise on a reef-building mussel. Sci. Total Environ. 689, 126–132. doi: 10.1016/j.scitotenv.2019.06.380
Wale, M. A., Simpson, S. D., and Radford, A. N. (2013). Noise negatively affects foraging and antipredator behaviour in shore crabs. Anim. Behav. 86, 111–118. doi: 10.1016/j.anbehav.2013.05.001
Zhao, X., Guo, C., Han, Y., Che, Z., Wang, Y., Wang, X., et al. (2017). Ocean acidification decreases mussel byssal attachment strength and induces molecular byssal responses. Mar. Ecol. Prog. Ser. 565, 67–77. doi: 10.3354/meps11992
Keywords: anthropogenic noise, mussel, byssal thread, attachment, mechanical performance, gene expression
Citation: Zhao X, Sun S, Shi W, Sun X, Zhang Y, Zhu L, Sui Q, Xia B, Qu K, Chen B and Liu G (2021) Mussel Byssal Attachment Weakened by Anthropogenic Noise. Front. Mar. Sci. 8:821019. doi: 10.3389/fmars.2021.821019
Received: 23 November 2021; Accepted: 06 December 2021;
Published: 23 December 2021.
Edited by:
Shiguo Li, Research Center for Eco-Environmental Sciences, Chinese Academy of Sciences (CAS), ChinaReviewed by:
Huaxin Gu, Shanghai Ocean University, ChinaCopyright © 2021 Zhao, Sun, Shi, Sun, Zhang, Zhu, Sui, Xia, Qu, Chen and Liu. This is an open-access article distributed under the terms of the Creative Commons Attribution License (CC BY). The use, distribution or reproduction in other forums is permitted, provided the original author(s) and the copyright owner(s) are credited and that the original publication in this journal is cited, in accordance with accepted academic practice. No use, distribution or reproduction is permitted which does not comply with these terms.
*Correspondence: Guangxu Liu, Z3Vhbmd4dV9saXVAemp1LmVkdS5jbg==; orcid.org/0000-0002-1039-7801
Disclaimer: All claims expressed in this article are solely those of the authors and do not necessarily represent those of their affiliated organizations, or those of the publisher, the editors and the reviewers. Any product that may be evaluated in this article or claim that may be made by its manufacturer is not guaranteed or endorsed by the publisher.
Research integrity at Frontiers
Learn more about the work of our research integrity team to safeguard the quality of each article we publish.