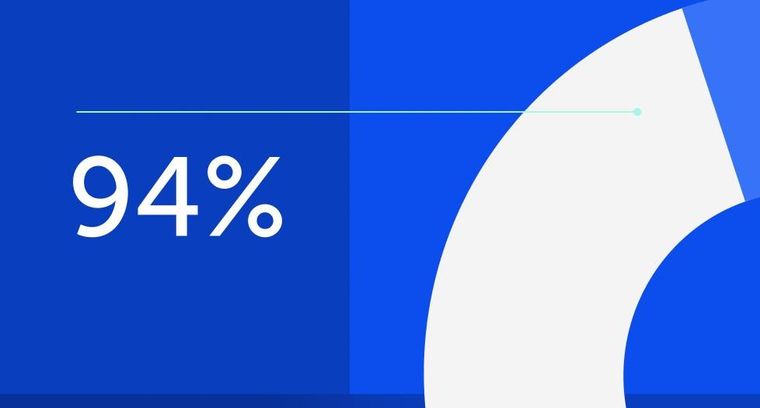
94% of researchers rate our articles as excellent or good
Learn more about the work of our research integrity team to safeguard the quality of each article we publish.
Find out more
ORIGINAL RESEARCH article
Front. Mar. Sci., 18 January 2022
Sec. Marine Ecosystem Ecology
Volume 8 - 2021 | https://doi.org/10.3389/fmars.2021.811533
This article is part of the Research TopicEcophysiology and Biogeochemistry of Marine Plants in the AnthropoceneView all 15 articles
Shallow coastal soft bottoms are important carbon sinks. Submerged vegetation has been shown to sequester carbon, increase sedimentary organic carbon (Corg) and thus suppress greenhouse gas (GHG) emissions. The ongoing regression of seagrass cover in many areas of the world can therefore lead to accelerated emission of GHGs. In Nordic waters, seagrass meadows have a high capacity for carbon storage, with some areas being recognized as blue carbon hotspots. To what extent these carbon stocks lead to emission of methane (CH4) is not yet known. We investigated benthic CH4 emission (i.e., net release from the sediment) in relation to seagrass (i.e. Zostera marina) cover and sedimentary Corg content (%) during the warm summer period (when emissions are likely to be highest). Methane exchange was measured in situ with benthic chambers at nine sites distributed in three regions along a salinity gradient from ∼6 in the Baltic Sea (Finland) to ∼20 in Kattegat (Denmark) and ∼26 in Skagerrak (Sweden). The net release of CH4 from seagrass sediments and adjacent unvegetated areas was generally low compared to other coastal habitats in the region (such as mussel banks and wetlands) and to other seagrass areas worldwide. The lowest net release was found in Finland. We found a positive relationship between CH4 net release and sedimentary Corg content in both seagrass meadows and unvegetated areas, whereas no clear relationship between seagrass cover and CH4 net release was observed. Overall, the data suggest that Nordic Zostera marina meadows release average levels of CH4 ranging from 0.3 to 3.0 μg CH4 m–2 h–1, which is at least 12–78 times lower (CO2 equivalents) than their carbon accumulation rates previously estimated from seagrass meadows in the region, thereby not hampering their role as carbon sinks. Thus, the relatively weak CH4 emissions from Nordic Z. marina meadows will not outweigh their importance as carbon sinks under present environmental conditions.
Methane (CH4) is a very potent greenhouse gas (GHG), with a global warming potential that is estimated to be 28–34 times higher than carbon dioxide (CO2) per mole of carbon over a 100-year period (Myhre et al., 2013). It has been estimated that about half of the global CH4 emissions generate from aquatic sources, although there is high variability between regions and ecosystems (Saunois et al., 2020; Rosentreter et al., 2021). Oceanic shelves, although marginal in area compared to deep oceans, contribute to about 75% of the CH4 emission from oceans worldwide (Bange et al., 1994). Methane in the marine environment is mainly produced in sediments during anaerobic degradation of organic matter by methanogenic archaea (Bakker et al., 2014; Wilson et al., 2020). The produced CH4 may be oxidized to CO2 in the sediment or released to the water column in solution by diffusion, via plant–tissue or as gas bubbles (Reeburgh, 2007; Jeffrey et al., 2019). Generally, only a small portion of the produced CH4 eventually reaches the atmosphere, since most CH4 is oxidized by microorganisms in the sediment and water column (Reeburgh, 2007). Seeping bubbles released from sediments lose most of their CH4 content during their passage through the water column and the extent of that loss depends on the bubble size and water depth (Weber et al., 2019). This explains why most marine CH4 emissions derive from the nearshore coastal environment, where there is less likelihood that the CH4 is oxidized before reaching the atmosphere (Weber et al., 2019).
Natural wetlands, i.e., vegetated ecosystems where the soil is water-saturated for most part of the year and which store large amount of carbon in their soils, account for 20–30% of the global yearly CH4 emissions and are thus the single largest non-anthropogenic source of CH4, adding up to 164 Tg yr–1 to the atmosphere (IPCC, 2001; Bridgham et al., 2013; He et al., 2015). In the coastal zone, vegetated habitats (i.e. saltmarshes, mangroves, and seagrass meadows) are estimated to emit around 4 Tg yr–1 (Al-Haj and Fulweiler, 2020). This emission level is hence low compared to their terrestrial counterparts, although greater than the release from open oceans, which are estimated to release between 0.4 and 1.8 Tg yr–1 (Rhee et al., 2009; Borges et al., 2016). Photosynthetically derived oxygen from submerged plants can potentially be used by methane-oxidizing bacteria (MOBs) in the sediment and water column, converting CH4 to CO2, and thereby hinder emission of CH4 to the atmosphere in these submerged vegetated ecosystems (Laanbroek, 2010). This may contrast with conditions in terrestrial and coastal wetlands where vegetation is emerged, i.e., in direct contact with the atmosphere, and therefore CH4 will be released without being processed in the water phase by MOBs (Laanbroek, 2010). Up to 90% of the CH4 produced in sediments with submerged vegetation can be reoxidized in the water column (King et al., 1990), but if the oxygen levels are low, due to for instance stagnant waters and during high consumption rates, the CH4 may be emitted to the atmosphere.
High organic loading and anoxic sediments provide conditions for long-term storage of refractory carbon. Consequently, coastal vegetated ecosystems such as saltmarshes, mangroves, and seagrass meadows are efficient sinks of atmospheric CO2 and referred to as blue carbon habitats (e.g., Mcleod et al., 2011; Duarte, 2017; Howard et al., 2017). However, the same conditions that make these habitats ideal for carbon storage also provide the potential for CH4 production (Al-Haj and Fulweiler, 2020). Conditions that favor methanogenesis could tip these coastal habitats from sinks to sources of CO2 and CH4 and thereby accelerate the greenhouse effect. It is therefore of great importance to understand the conditions governing the release of CH4 and other GHGs from these habitats. Studies from tidal saltmarshes show that CH4 emission is strongly salinity dependent, with significantly lower emissions at salinities over 18 (Poffenbarger et al., 2011), while in fresh- to brackish water marshes such a salinity-driven threshold has been reported to occur already at salinities above 10 (Wang et al., 2017). In peatlands, sulfate reduction inhibits methanogenesis and the release of CH4 is low (Dowrick et al., 2006). However, in anoxic marine sediments, sulfate reduction and methanogenesis may co-occur (Oremland and Taylor, 1978; Holmer and Kristensen, 1994; Sela-Adler et al., 2017).
Seagrass meadows have been reported to naturally emit low to moderate levels of CH4, ranging from 2–5 (Oremland, 1975) to 378 μg m–2 h–1 (Garcias-Bonet and Duarte, 2017). This is substantially lower than what has been observed in other marine habitats; for example, saltmarshes can emit over 10,000 μg m–2 h–1 (Whiting and Chanton, 1993). However, stressors (such as light reduction, habitat fragmentation, and warming) can dramatically increase CH4 emission in seagrass systems (Lyimo et al., 2018; Burkholz et al., 2020; George et al., 2020). Vegetation loss or alteration in macrophyte species composition may also stimulate methanogenesis in the sediment (Sutton-Grier and Megonigal, 2011; Lyimo et al., 2018; Al-Haj and Fulweiler, 2020).
In the Nordic region, seagrass meadows have high capacity for storing large amounts of carbon in the sediment, in particular, some sites along the Swedish Skagerrak coast are suggested to be global hotspots for blue carbon (Dahl et al., 2016; Röhr et al., 2018; Moksnes et al., 2021). It is previously known from the coastal southern Baltic Sea that CH4 emissions are positively correlated to the organic carbon content in sediments (Heyer and Berger, 2000). Therefore, it is of particular interest to study blue carbon habitats, such as seagrass meadows, that may store large amounts of organic carbon (Corg) in their sediments to understand the fate of stored carbon as potential sources for GHG emissions. No previous reports have, however, focused on CH4 emissions from Zostera marina beds in northern European coastal waters. In the current study, we aimed to investigate (i) the extent and variability in CH4 (g) emission from seagrass (Z. marina) dominated coastal soft bottom sediments in three regions along the salinity gradient from the Baltic Sea to the North Sea, (ii) to what degree vegetation cover (in terms of Z. marina) modifies CH4 (g) emission from the substrate, and (iii) whether CH4 (g) emission is correlated to the sedimentary organic carbon content.
Nordic coastal areas are of particular interest since they stretch from the Baltic Sea, which is a semi-enclosed water body and one of the largest brackish water areas in the world, to the marine environments of Skagerrak and Kattegat through the Danish straits (Storebælt, Lillebælt, and Öresund). The region is therefore characterized by a strong, large-scale salinity gradient from freshwater conditions (0–2) in the Bothnian Bay to marine conditions (∼34) in the North Sea (Helcom 2017-2018)1. Coastal shallow habitats in northern areas are deemed by climate scenario models to be exposed to faster warming than the global average, with an expected temperature increase ranging from 2°C in the southern part of the Baltic Sea to 4°C in the northern part by the end of this century (i.e., year 2100, Andersson et al., 2015), which may influence CH4 emissions from coastal blue carbon habitats in the future. Further, the coastal waters of the Baltic Sea, the Kattegat and Skagerrak are surrounded by nine countries and human activities in the area, adding pressure on the seagrass ecosystems (Boström et al., 2014). For instance, severe seagrass loss of about 60% has been reported on the Swedish west coast between 1980s and 2000s (Baden et al., 2003; Nyqvist et al., 2009). From some of these areas in Sweden where historical losses have occurred, it has been estimated that the resulting loss of carbon from the sediments could be up to 60 Mg C ha–1 (Moksnes et al., 2021).
The current study was carried out during a warm summer period (with water temperatures ranging from 20 to 23.5°C; see Supplementary Table 1) in August 2018 in Z. marina meadows and adjacent unvegetated areas within three regions, along the salinity gradient stretching from the Baltic Sea archipelago west of Turku in Finland (three sites) to the fjords east of Fyn Odense in Denmark (two sites) and the Gullmar Fjord on the Swedish Skagerrak coast (four sites) (see Figure 1 and Table 1). At the Finnish study area, Z. marina grows at the lower end of its salinity propagation limit (∼6), while the Danish sites have intermediate salinities (∼20) and the Swedish sites have salinities varying between 18 and 30 in the surface waters, with a yearly average of ∼26. Water temperatures were between 20 and 23.5°C in all study areas during the sampling period. In Finland, the sites are moderately exposed, and the sediment consists mainly of fine to coarse sand with low levels of organic content. In Denmark, the Nyborg site is exposed to easterly winds and the sediment is sandy with a low organic content, while the Holckenhavn Fjord is sheltered, and the sediment is siltier with a low organic content. In Sweden, the sites are situated in shallow bays exposed to different levels of hydrodynamic forces and the topmost layers in the sediments are sandy, silty, or muddy.
Figure 1. Map of sites (green dots, n = 2–4) in the three sampling regions (countries) with average methane emissions from sediments in seagrass meadows and unvegetated areas in each region as well as overall for all countries.
Table 1. Sampling design showing number of replicates, water depth and water temperature in seagrass meadows and adjacent unvegetated habitats at the different sampling sites, and mean salinity for each of the three sampling regions.
Incubation chambers, produced by transparent Plexiglas cores (inner diameter: 4.7 cm, height: 45 cm; Figure 2) containing an air-filled gas pocket with a gas-tight septum for extraction of gas samples, were placed in seagrass meadows (n = 6) and in adjacent unvegetated areas (n = 5 or 6) at 2–3 m water depth using scientific (SCUBA) diving. For details of the sampling regions and sites, see Table 1. The start time for incubations was set to around 10 am to catch the midday hours when productivity is expected to be highest. The chamber cores were pushed down 15 cm into the sediment, leaving a 20-cm water column and a 5-cm gas pocket (volume: 87 cm3) above the water in the chamber. A “start” gas sample (5 mL) was withdrawn from the pocket about 10 minutes after placing the chamber into the sediment and the time was noted. The incubations were conducted for about 5–6 h, whereafter an “end” gas sample was extracted from the gas pocket and the ending time was noted. The gas samples were directly transferred into gas-tight exetainers containing 58.3 mM zinc chloride (aq) for storage and to prevent any potential bacterial breakdown of CH4 until analysis. The exetainers were stored upside-down in the refrigerator (4°C) until analysis. At the Swedish sites, the development of CH4 release in the chambers was observed at several time points during the incubation period to follow and confirm linearity in the CH4 release.
Figure 2. Deployed incubation chamber in a seagrass meadow (left) and collection of a gas sample (top right). Illustration (bottom right) of the sampling methodology using an incubation chamber inserted 15 cm into sediment with a 20 cm water column above the sediment. On the top, a 5 cm air-pocket is connected to a gas-tight septum from where a gas-sample (including methane) released from the sediment could be collected (using a syringe). Gas-samples were extracted periodically after insertion from the chamber and stored in gas-tight exetainers until analyzed with gas-chromatography (GC). Photos: K. Gagnon.
Salinities and temperatures were measured at each site prior to incubations. In the seagrass sites, seagrass shoot density (shoots per m2) were either measured, in field, in quadrats (25 × 25 cm, n = 6) or measurements from previous studies (Gullström et al., 2012; Staveley et al., 2017) (see Supplementary Table 1) were used.
The gas in the collected exetainer samples were analyzed by means of headspace analysis and gas chromatography (GC). Briefly, 1 mL headspace was injected into a gas chromatographer (GC 8A, Shimadzu Corporation) equipped with a Porapak N column (80–100 mesh) and a flame ionization detector (FID). The carrier gas for the FID was nitrogen, while the fuel gas was hydrogen and the oxidant air. For calibration, certified standards at atmospheric concentration (1.9 ppm) and with 49.9 ppm CH4 (AirLiquide Gas AB) were used. Using the Ideal Gas Law (PV = nRT), the ppm concentrations were converted into molar concentrations (μmol CH4 L–1), which were plotted against incubation time. The CH4 emissions per surface area of the sediments were calculated as the total amount of CH4 accumulating over time within the gas-filled pocket of the incubation chamber and reported as μg CH4 m–2 h–1. Since measurements were only conducted during daytime, values were not extrapolated to full diurnal estimates.
After incubation, a sediment core was collected adjacent to each incubation chamber using a push corer (diameter: 4.7 cm, height: 60 cm). The cores were sliced into three different depth sections: 0–1 cm representing the oxidized zone, 1–15 cm representing the rhizosphere and below 15 cm representing the sediment without living seagrass. Sediment compression was accounted for in all cores by measuring the distance from the top of the core to the sediment surface, inside and outside the core after being inserted into the sediment (Glew et al., 2002).
Sediment core slices were weighed wet, homogenized and a subsample of 60 mL from each depth was then dried (60° C, ∼48 h) until constant weight, whereafter the dry bulk density (g DW cm–3) was calculated. The dry sediment samples were grinded to a powder using a Retch 400 mixing mill for subsequent carbon analyses. The total carbon and nitrogen content (% C and % N) in each sediment depth section was analyzed using a carbon–nitrogen elemental analyzer (Flash 2000, Thermo Fisher Scientific). Previous research in the studied regions have documented that the inorganic carbon content generally is low (<5%) and was therefore not accounted for Röhr et al. (2016); Dahl et al. (2020).
Variations in CH4 emission rates were compared between the different regions for the two habitat types, i.e., seagrass meadows vs. unvegetated areas separately, and then overall between seagrass meadows and unvegetated areas, using non-parametric Kruskal–Wallis tests (since with the log10[x + 1] transformation, homogeneity of variance was not achieved). A Bonferroni correction of the significance level was applied for multiple testing to limit the probability of Type 1 error (Holm, 1979). Potential relationships between CH4 emission and environmental variables such as sedimentary Corg content and seagrass shoot density, respectively were tested with linear regression analysis. All data analyses were performed in IBM SPSS statistics (version 27).
The CH4 emissions were generally low but varied substantially both within and between sites, resulting in a net release of CH4 to the air phase ranging from 0.3 to 1.8 μg CH4 m–2 h–1 at the Finnish sites to 2.0–2.5 μg CH4 m–2 h–1 at the Danish sites and 0.4–3.0 μg CH4 m–2 h–1 at the Swedish sites (Figures 1, 3). Pairwise comparisons showed that the overall CH4 emissions from seagrass meadows were significantly higher in both the Swedish and Danish sites when compared to the Finnish sites, while there was no significant difference between the Swedish and Danish sites (Table 2). For the unvegetated areas, CH4 emissions were significantly higher in the Swedish sites compared to the Finnish sites (Table 2). Overall, there was no difference in emissions between seagrass covered- and unvegetated sediments, even though differences between these habitat types occurred at some sites within each region (Table 2 and Figure 3).
Figure 3. Box-and-whisker-plot showing the variation of methane emission from the sediment to the gas-filled pocket in the incubation chambers of seagrass meadows and adjacent unvegetated areas in Finland (FIN), Denmark (DEN), and Sweden (SWE). The solid lines within the boxes indicate median values, the boxes represent the 25th to the 75th percentiles and the vertical whisker bars show the 5th and 95th percentiles of the data.
Table 2. Summary of non-parametric Kruskal–Wallis tests of methane emissions among regions within (seagrass meadows and unvegetated areas) and between habitats.
Methane emission increased along the salinity gradient (Figures 1, 4), although this pattern likely also is reflecting the inherent conditions of the three regions. The mean integrated (0-15 cm) organic carbon (Corg) content in the sediment varied between 0.1 and 6%, with the largest levels found in the Swedish sites (Supplementary Tables 1, 2). There were linear relationships between sedimentary Corg and CH4 emissions in seagrass meadows (Adj R2 = 0.12, p = 0.01, Supplementary Figure 1A) and in unvegetated areas (Adj R2 = 0.21, p < 0.01, Supplementary Figure 1B), respectively, although the adjusted R2 value were low indicating that there are other factors influencing the CH4 mean net release.
Figure 4. Box-and-whisker plot summarizing methane emissions from the sediment to the gas-filled pocket in the incubation chambers in seagrass meadows and adjacent unvegetated areas in relation to average salinities in the different regions. The solid lines within the boxes indicate median values, the boxes represent the 25th to the 75th percentiles and the vertical whisker bars show the 5th to the 95th percentiles of the data.
Seagrass meadows average shoot densities, as presented in previous works, varied from 101 to 652 shoots m–2 (Supplementary Table 1). There was no significant relationship between the average seagrass shoot density and CH4 emission in the current study.
This study shows that CH4 emissions from cold-temperate Nordic seagrass meadows are relatively low, both when compared to seagrass areas worldwide and when compared to other shallow-water habitats in the Nordic region. The amount of Corg stored in the sediment appeared to influence the emissions, as there was a positive correlation between CH4 emission and the sedimentary organic carbon content. The relatively low explanatory value suggests that besides the sedimentary Corg content, there must be other factors that are of major importance for methane release from these coastal sediments. Nevertheless, we found no significant influence of vegetation or salinity on CH4 emissions. The current study was conducted during the warm high-productive season, when also the net release of methane is expected to peak, and represents the first survey of methane emissions from seagrass meadow sediments in Nordic coastal waters.
Methane net release from Z. marina meadow sediments varied from 0.3 to 3.0 μg m–2 h–1. These values are in the lower range of what is reported from seagrass habitats globally, which can reach up to 378 μg m–2 h–1 (see Table 3). It is further drastically lower than reported emissions from other shallow-water habitats in Nordic waters like estuarine wetlands (8,583 μg m–2 h–1) or brackish-water reed (Phragmites) belts (15,200 μg m–2 h–1), (Table 3). The relatively low CH4 emission levels measured in our study agree well with those reported for coastal bare sediments (1.2–2.3 μg CH4 m–2 h–1) of the Baltic proper (Bonaglia et al., 2017). Those sediments had similar carbon content as on the Swedish west coast (5.5%) and similar salinities as in the Finnish areas (6.8), but were sampled deeper (50 m) and at much lower temperature (8.0°C) compared to our study (range: 20–23.5°C, Supplementary Table 1). The good agreement in rates may thus be explained by the fact that most of the CH4 generated deep inside the sediment is efficiently oxidized by the community of methane-oxidizing archaea and sulfate-reducing bacteria before it can reach the sediment water-interface (Orphan et al., 2001). Up to 90% of the CH4 produced in marine sediments are consumed already in the sediment phase (Reeburgh et al., 1993).
Table 3. Sediment methane (CH4) emission rates from seagrass meadows worldwide and other shallow-water habitats in Nordic waters, using sediment-to-air filled pocket chamber techniques, reported in the literature and in the current study.
Most studies where high emissions from seagrass habitats have been reported are from warmer (warm-temperate or tropical) waters (Table 3). This could be due to a temperature dependence of CH4 production as well as differences in organic matter quality and quantity, and in the microbial community composition. Temperature is known to significantly influence CH4 production both in tropical (Burkholz et al., 2020; George et al., 2020) and cold-temperate waters (Heyer and Berger, 2000). In the present study, water temperatures were similar across regions; nevertheless, differences in water temperature on local spatial (cm to m) and temporal (day to seasonal) scales could still influence the variation in emission rates, but this was not investigated here.
We found no significant influence of vegetation cover on CH4 emission from the sediments in the seagrass meadows, and unvegetated sediments had similar emissions as the vegetated areas. There are, however, reports that submerged vegetation, such as seagrasses and other rooted macrophytes, significantly influences the microbial composition and actions in the sediments (Jensen et al., 2007; Santos-Fonseca et al., 2015; Cúcio et al., 2016), with potential effects on the GHG balance. Seagrasses have been reported to reduce CH4 emissions by their photosynthetic oxygen production (Oremland, 1975; George et al., 2020) and also by symbiotic CH4 oxidizers associated with the plants.
In some coastal habitats, such as in tidal saltmarshes, CH4 emission is strongly affected by salinity, with lower emissions at higher salinities (Poffenbarger et al., 2011; Wang et al., 2017). It has been suggested that the higher levels of sulfate found in sediments of higher salinity will increase sulfate reduction, which in turn could inhibit CH4 production in vegetated habitats (e.g., Koebsch et al., 2018). Methane emissions from marine areas could hence be expected to be low. In contrast, we found the highest emission levels within the region with highest salinities, i.e., at the Swedish sites. When the organic carbon loading is high, it can be that the inhibitory effect of sulfate reduction plays a minor role and sulfate reduction and methanogenesis can co-occur (Holmer and Kristensen, 1994; Santos-Fonseca et al., 2015). Therefore, CH4 production can occur in marine areas with high organic carbon content, which is also confirmed in the current study.
Even though the emissions we measured from both vegetated and adjacent unvegetated sediments were low, the CH4 emissions partly counteract the seagrass meadows’ capacity to function as carbon sinks. The only published data on carbon accumulation rates for seagrasses in the Nordic area (Röhr et al., 2016) show annual mean values of 0.05 t C ha–1 yr–1 for Finland, and 0.35 t C ha–1 yr–1 for Denmark, while no accumulation data for Sweden has been published. Given in the same units and calculated as e-CO2 at a GWP100 of 34 (Myhre et al., 2013), the CH4 emissions in this study ranged from 0.0007 to 0.0040 in Finland, from 0.0045 to 0.0056 in Denmark, and from 0.0009 to 0.0067 t eCO2-C ha–1 yr–1 in Sweden. Thus, the carbon accumulation rate in Finland was between 12 and 75 times higher, and in Denmark between 63 and 78 times higher, than the estimated C emissions from CH4. We therefore conclude that the relatively weak emissions of CH4 from Nordic Z. marina meadows will not outweigh their importance as carbon sinks under present environmental conditions.
Climate simulations for the Baltic Sea ecosystems indicate a 2–4°C warming and a significant increase in precipitation by the year 2100 that may increase land runoff of allochthonous organic matter and decrease salinity (Andersson et al., 2015). This might have multifaceted effects on the seagrass systems. While healthy seagrass meadows contribute to mitigate the effects of runoff and capture part of the increased input of nutrients and organic matter, an increased organic content in the sediments might result in increased respiration and lower oxidation state of the sediment. A lower oxidation will in turn favor anaerobic respiration and might thus lead to increased production and emissions of CH4 and other GHGs. As temperatures are predicted to increase more drastically in the Nordic region than on a global scale (Andersson et al., 2015), this may accelerate CH4 emissions from blue carbon habitats such as seagrass meadows (Yvon-Durocher et al., 2011). It has previously been shown in tropical seagrass sediments that CH4 emissions more than doubled during high temperature stress (George et al., 2020). The Nordic seagrass systems, today functioning as effective sinks for atmospheric CO2, might thus be hampered by climate change effects so that their carbon capture capacity is reduced while their emission of GHGs is increased. This may eventually turn Nordic seagrass meadows from sinks to sources of CO2.
In conclusion, the relative low net release of methane from Nordic seagrass meadows presented in this study may reinforce their capacity as natural blue carbon sinks. To fully understand the extent of emissions of methane and other GHGs from Nordic coastal habitats, multiple spatial (from microhabitat to seascape level) and temporal (diurnal and seasonal) aspects should be considered in future studies.
The original contributions presented in the study are included in the article/Supplementary Material, further inquiries can be directed to the corresponding author.
MA, MB, SB, MG, CB, and MH conceived and designed the study. MA, MB, DD, MG, CB, and KG carried out the fieldwork. MA, SB, and MD carried out the lab work and analysis. MA and MB wrote the first draft of the manuscript with aid from MD and MG. All authors contributed to the final version of the manuscript.
The research was partly funded by the Bolin Centre for Climate Research. SB was supported by the Swedish Research Council Formas (grant number: 2017-01513). CB was funded by the bo Akademi University Foundation SR. KG was funded by the European Union’s Horizon 2020 Research and Innovation Programe as part of the project MERCES: Marine Ecosystem Restoration in Changing European Seas (grant number: 689518).
The authors declare that the research was conducted in the absence of any commercial or financial relationships that could be construed as a potential conflict of interest.
All claims expressed in this article are solely those of the authors and do not necessarily represent those of their affiliated organizations, or those of the publisher, the editors and the reviewers. Any product that may be evaluated in this article, or claim that may be made by its manufacturer, is not guaranteed or endorsed by the publisher.
Thanks to Nellie Stjärnkvist for assistance with laboratory analyses and also Lukas Meysick and Luca Rugiu for assistance in the field and with laboratory work.
The Supplementary Material for this article can be found online at: https://www.frontiersin.org/articles/10.3389/fmars.2021.811533/full#supplementary-material
Al-Haj, A. N., and Fulweiler, R. W. (2020). A synthesis of methane emissions from shallow vegetated coastal ecosystems. Glob. Change Biol. 26, 2988–3005. doi: 10.1111/gcb.15046
Andersson, A., Meier, H. E. M., Ripszam, M., Rowe, O., Wikner, J., Haglund, P., et al. (2015). Projected future climate change and Baltic Sea ecosystem management. Ambio 44, 345–356. doi: 10.1007/s13280-015-0654-8
Baden, S., Gullström, M., Lundén, B., Pihl, L., and Rosenberg, R. (2003). Vanishing seagrass (Zostera marina, L.) in Swedish coastal waters. Ambio 32, 374–377. doi: 10.1579/0044-7447-32.5.374
Bahlmann, E., Weinberg, I., Lavrič, J., Eckhard, T., Michaelis, W., Santos, R., et al. (2014). Tidal controls on trace gas dynamics in a seagrass meadow of the Ria Formosa lagoon (southern Portugal). Biogeosci. Discuss. 11, 10571–10603. doi: 10.5194/bg-12-1683-2015
Bakker, D. C. E., Bange, H. W., Gruber, N., Johannessen, T., Upstill-Goddard, R. C., Borges, A. V., et al. (2014). “Air-sea interactions of natural long-lived greenhouse gases (CO2, N2O, CH4) in a changing climate,” in Ocean-Atmosphere Interactions of Gases and Particles, eds P. S. Liss and M. T. Johnson (Berlin: Springer), doi: 10.1007/978-3-642-25643-1_3
Bange, H. W., Bartell, U. H., Rapsomanikis, S., and Andreae, M. O. (1994). Methane in the Baltic and North Seas and a reassessment of the marine emissions of methane. Glob. Biogeochem. Cycl. 8, 465–480. doi: 10.1029/94GB02181
Barber, T. R., and Carlson, P. R. Jr. (1993). “Effects of seagrass die-off on benthic fluxes and porewater concentrations of ∑ CO2, ∑ H2S, and CH4 in Florida Bay sediments,” in Biogeochemistry of Global Change: Radiatively Active Trace Gases, ed. R. S. Oremland (New York, NY: Chapman and Hall), 530–550. doi: 10.1007/978-1-4615-2812-8_29
Bonaglia, S., Brüchert, V., Callac, N., Vicenzi, A., Fru, E. C., and Nascimento, F. J. A. (2017). Methane fluxes from coastal sediments are enhanced by macrofauna. Sci. Rep. 7:13145. doi: 10.1038/s41598-017-13263-w
Borges, A. V., Champenois, W., Gypens, N., Delille, B., and Harlay, J. (2016). Massive marine methane emissions from near-shore shallow coastal areas. Sci. Rep. 6:27908. doi: 10.1038/srep27908
Boström, C., Baden, S., Bockelmann, A.-C., Dromph, K., Fredriksen, S., Gustafsson, C., et al. (2014). Distribution, structure and function of Nordic eelgrass (Zostera marina) ecosystems: implications for coastal management and conservation. Aquatic. Conserv. Mar. Freshw. Ecosyst. 24, 410–434. doi: 10.1002/aqc.2424
Bridgham, S. D., Cadillo-Quiroz, H., Keller, J. K., and Zhuang, Q. (2013). Methane emissions from wetlands: biogeochemical, microbial, and modeling perspectives from local to global scales. Glob. Change Biol. 19, 1325–1346. doi: 10.1111/gcb.12131
Burkholz, C., Garcias-Bonet, N., and Duarte, C. M. (2020). Warming enhances carbon dioxide and methane fluxes from Red Sea seagrass (Halophila stipulacea) sediments. Biogeosci. 17, 1717–1730. doi: 10.5194/bg-17-1717-2020
Cúcio, C., Engelen, A. H., Costa, R., and Muyzer, G. (2016). Rhizosphere microbiomes of European seagrasses are selected by the plant, but are not species specific. Front. Microbiol. 7:440. doi: 10.3389/fmicb.2016.00440
Dahl, M., Asplund, M. E., Deyanova, D., Franco, J. N., Koliji, A., Infantes, E., et al. (2020). High seasonal variability in sediment carbon stocks of cold–temperate seagrass meadows. J. Geophys. Res. Biogeosci. 125, 1–13. doi: 10.1029/2019JG005430
Dahl, M., Deyanova, D., Guetschow, S., Asplund, M. E., Lyimo, L. D., Karamfilov, V., et al. (2016). Sediment properties as important predictors of carbon storage in Zostera marina meadows: a comparison of four European areas. PLoS One 11:e0167493. doi: 10.1371/journal.pone.0167493
Deborde, J., Anschutz, P., Guérin, F., Poirier, D., Marty, D., Boucher, G., et al. (2010). Methane sources, sinks and fluxes in a temperate tidal Lagoon: the Arcachon lagoon (SW France). Estuar. Coast. Shelf Sci. 89, 256–266. doi: 10.1016/j.ecss.2010.07.013
Dowrick, D. J., Freeman, C., Lock, M. A., and Reynolds, B. (2006). Sulphate reduction and the suppression of peatland methane emissions following summer drought. Geoderma 132, 384–390. doi: 10.1016/j.geoderma.2005.06.003
Duarte, C. M. (2017). Reviews and syntheses: Hidden forests, the role of vegetated coastal habitats in the ocean carbon budget. Biogeosciences 14, 301–310. doi: 10.5194/bg-14-301-2017
Garcias-Bonet, N., and Duarte, C. M. (2017). Methane production by seagrass ecosystems in the Red Sea. Front. Mar. Sci. 4:340. doi: 10.3389/fmars.2017.00340
George, R., Gullström, M., Mtolera, M. S. P., Lyimo, T. J., and Björk, M. (2020). Methane emission and sulfide levels increase in tropical seagrass sediments during temperature stress: a mesocosm experiment. Ecol. Evol. 10, 1917–1928. doi: 10.1002/ece3.6009
Glew, J. R., Smol, J. P., and Last, W. M. (2002). “Sediment core collection and extrusion,” in Tracking Environmental Change Using lake Sediments, Developments in Paleoenvironmental Research, Vol. 1, eds W. M. Last and J. P. Smol (Dordrecht: Springer), 73–105. doi: 10.3791/54363
Gullström, M., Baden, S., and Lindegarth, M. (2012). Spatial patterns and environmental correlates in epiphytic assemblages of temperate seagrass (Zostera marina) meadows. Mar. Biol. 159, 413–425. doi: 10.1007/s00227-011-1819-z
He, S., Malfatti, S. A., McFarland, J. W., Anderson, F. E., Pati, A., Huntemann, M., et al. (2015). Patterns in wetland microbial community composition and functional gene repertoire associated with methane emissions. mBio 6:e00066-15. doi: 10.1128/mBio.00066-15
Heyer, J., and Berger, U. (2000). Methane emission from the coastal area in the southern Baltic Sea. Estuar. Coast. Shelf Sci. 51, 13–30. doi: 10.1006/ecss.2000.0616
Holmer, M., and Kristensen, E. (1994). Coexistence of sulfate reduction and methane production in an organic-rich sediment. Mar. Ecol. Prog. Ser. 107, 177–177. doi: 10.3354/meps107177
Howard, J., Sutton-Grier, A., Herr, D., Kleypas, J., Landis, E., Mcleod, E., et al. (2017). Clarifying the role of coastal and marine systems in climate mitigation. Front. Ecol. Environ. 15:42–50. doi: 10.1002/fee.1451
IPCC (2001). “Climate change 2001: the scientific basis,” in Contribution of Working Group I to the Third Assessment Report Of The Intergovernmental Panel on Climate Change, eds J. T. Houghton, Y. Ding, D. J. Griggs, M. Noguer, P. J. van der Linden, X. Dai, et al. (Cambridge: Cambridge University Press). doi: 10.1111/j.1461-0248.2005.00854.x
Jeffrey, L. C., Maher, D. T., Johnston, S. G., Kelaher, B. P., Steven, A., and Tait, D. R. (2019). Wetland methane emissions dominated by plant-mediated fluxes: contrasting emissions pathways and seasons within a shallow freshwater subtropical wetland. Limnol. Oceanogr. 64, 1895–1912. doi: 10.1002/lno.11158
Jensen, S. I., Kühl, M., and Priemé, A. (2007). Different bacterial communities associated with the roots and bulk sediment of the seagrass Zostera marina. FEMS Microbiol. Ecol. 62, 108–117. doi: 10.1111/j.1574-6941.2007.00373.x
King, G. M., Roslev, P., and Skovgaard, H. (1990). Distribution and rate of methane oxidation in sediments of the Florida Everglades. Appl. Environ. Microbiol. 56, 2902–2911. doi: 10.1128/aem.56.9.2902-2911.1990
Koch, S., Jurasinski, G., Koebsch, F., Koch, M., and Glatzel, S. (2014). Spatial variability of annual estimates of methane emissions in a phragmites australis (cav.) trin. ex steud. dominated restored coastal brackish fen. Wetlands 34, 593–602. doi: 10.1007/s13157-014-0528-z
Koebsch, F., Winkel, M., Liebner, S., Liu, B., Westphal, J., Schmiedinger, I., et al. (2018). Sulfate deprivation triggers high methane production in a disturbed and rewetted coastal peatland. Biogeosci. Discuss. 16, 1938–1953. doi: 10.5194/bg-2018-416
Laanbroek, H. J. (2010). Methane emission from natural wetlands: interplay between emergent macrophytes and soil microbial processes: a mini-review. Ann. Bot. 105, 141–153. doi: 10.1093/aob/mcp201
Liikanen, A., Silvennoinen, H., Karvo, A., Rantakokko, P., and Martikainen, P. J. (2009). Methane and nitrous oxide fluxes in two coastal wetlands in the northeastern Gulf of Bothnia. Baltic Sea. Bor. Environ. Res. 14, 351–368.
Lyimo, L. D., Gullström, M., Lyimo, T. J., Deyanova, D., Dahl, M., Hamisi, M. I., et al. (2018). Shading and simulated grazing increase the sulphide pool and methane emission in a tropical seagrass meadow. Mar. Poll. Bull. 134, 89–93. doi: 10.1016/j.marpolbul.2017.09.005
Mcleod, E., Chmura, G. L., Bouillon, S., Salm, R., Björk, M., Duarte, C. M., et al. (2011). A blueprint for blue carbon: toward an improved understanding of the role of vegetated coastal habitats in sequestering CO2. Front. Ecol. Environ. 9:552–560. doi: 10.1890/110004
Moksnes, P.-O., Röhr, M. E., Holmer, M., Eklöf, J. S., Eriander, L., Infantes, E., et al. (2021). Major impacts and societal costs of seagrass loss on sediment carbon and nitrogen stocks. Ecosphere 12:e03658. doi: 10.1002/ecs2.3658
Myhre, G., Shindell, D., and Pongratz, J. (2013). ““Anthropogenic and natural radiative forcing,” in Climate change 2013: The Physical Science Basis. Working Group I Contribution to the Fifth Assessment Report of the Intergovernmental Panel on Climate Change, ed. T. Stocker (Cambridge: Cambridge University Press), 659–740. doi: 10.1017/cbo9781107415324.018
Nyqvist, A., André, C., Gullström, M., Baden, S. P., and Åberg, P. (2009). Dynamics of seagrass meadows on the Swedish Skagerrak coast. Ambio 38, 85–88. doi: 10.1579/0044-7447-38.2.85
Oremland, R. S. (1975). Methane production in shallow-water, tropical marine sediments. Appl. Microbiol. 30, 602–608. doi: 10.1128/am.30.4.602-608.1975
Oremland, R. S., and Taylor, B. F. (1978). Sulfate reduction and methanogenesis in marine sediments. Geochim. Cosmochim. Acta. 42, 209–214. doi: 10.1016/0016-7037(78)90133-3
Orphan, V. J., Hinrichs, K.-U., Ussler Iii, W., Paull, C. K., Taylor, T., Sylva, S. P., et al. (2001). Comparative analysis of methane-oxidizing archaea and sulfate-reducing bacteria in anoxic marine sediments. Appl. Environ. Microbiol. 67, 1922–1934. doi: 10.1128/AEM.67.4.1922-1934.2001
Poffenbarger, H., Needelman, B., and Megonigal, P. (2011). Salinity influence on methane emissions from tidal marshes. Wetlands 31, 831–842. doi: 10.1007/s13157-011-0197-0
Reeburgh, W. S. (2007). Oceanic methane biogeochemistry. Chem. Rev. 107, 486–513. doi: 10.1021/cr050362v
Reeburgh, W. S., Whalen, S. C., and Alperin, M. J. (1993). “The role of methylotrophy in the global methane budget,” in Microbial Growth on C1 Compounds. Proceedings of the 7th International Symposium. American Society for Microbiology, eds J. C. Murrell and D. P. Kelly (Washington, DC: Intercept Press), 1–14. doi: 10.1038/s41564-019-0534-2
Rhee, T. S., Kettle, A. J., and Andreae, M. O. (2009). Methane and nitrous oxide emissions from the ocean: a reassessment using basin-wide observations in the Atlantic. J. Geophys. Res. 114:D12304. doi: 10.1029/2008JD011662
Röhr, M. E., Boström, C., Canal-Vergés, P., and Holmer, M. (2016). Blue carbon stocks in Baltic Sea eelgrass (Zostera marina) meadows. Biogeosciences 13, 6139–6153. doi: 10.5194/bg-13-6139-2016
Röhr, M. E., Holmer, M., Baum, J. K., Björk, M., Boyer, K., Chin, D., et al. (2018). Blue carbon storage capacity of temperate eelgrass (Zostera marina) meadows. Glob. Biogeochem. Cycl. 32, 1457–1475. doi: 10.1029/2018GB005941
Rosentreter, J. A., Borges, A. V., Deemer, B. R., Holgerson, M. A., Liu, S., Song, C., et al. (2021). Half of global methane emissions come from highly variable aquatic ecosystem sources. Nat. Geosci. 14, 225–230. doi: 10.1038/s41561-021-00715-2
Santos-Fonseca, A., Cardoso Marinho, C., and Assis Esteves, F. (2015). Aquatic macrophytes detritus quality and sulfate availability shape the methane production pattern in a dystrophic coastal lagoon. Amer. J. Plant Sci. 6, 1675–1684. doi: 10.4236/ajps.2015.610167
Saunois, M., Stavert, A. R., Poulter, B., Bousquet, P., Canadell, J. G., Jackson, R. B., et al. (2020). The global methane budget 2000–2017. Earth Syst. Sci. Data 12, 1561–1623. doi: 10.5194/essd-12-1561-2020
Sela-Adler, M., Ronen, Z., Herut, B., Antler, G., Vigderovich, H., Eckert, W., et al. (2017). Co-existence of methanogenesis and sulfate reduction with common substrates in sulfate-rich estuarine sediments. Front. Microbiol. 8:766. doi: 10.3389/fmicb.2017.00766
Staveley, T. A. B., Perry, D., Lindborg, R., and Gullström, M. (2017). Seascape structure and complexity influence temperate seagrass fish assemblage composition. Ecography 40, 936–946. doi: 10.1111/ecog.02745
Sutton-Grier, A. E., and Megonigal, J. P. (2011). Plant species traits regulate methane production in freshwater wetland soils. Soil Biol. Biochem. 43, 413–420. doi: 10.1016/j.soilbio.2010.11.009
Wang, C., Tong, C., Chambers, L. G., and Liu, X. (2017). Identifying the salinity thresholds that impact greenhouse gas production in subtropical tidal freshwater marsh soils. Wetlands 37, 559–571. doi: 10.1007/s13157-017-0890-8
Weber, T., Wiseman, N. A., and Kock, A. (2019). Global ocean methane emissions dominated by shallow coastal waters. Nat. Comm. 10:4584. doi: 10.1038/s41467-019-12541-7
Whiting, G., and Chanton, J. (1993). Primary production control of methane emission from wetlands. Nature 364, 794–795. doi: 10.1038/364794a0
Wilson, S. T., Al-Haj, A. N., Bourbonnais, A., Frey, C., Fulweiler, R. W., Kessler, J. D., et al. (2020). Ideas and perspectives: a strategic assessment of methane and nitrous oxide measurements in the marine environment. Biogeosci. 17, 5809–5828. doi: 10.5194/bg-17-5809-2020
Keywords: seagrass, greenhouse gas, blue carbon, nordic, Zostera marina
Citation: Asplund ME, Bonaglia S, Boström C, Dahl M, Deyanova D, Gagnon K, Gullström M, Holmer M and Björk M (2022) Methane Emissions From Nordic Seagrass Meadow Sediments. Front. Mar. Sci. 8:811533. doi: 10.3389/fmars.2021.811533
Received: 08 November 2021; Accepted: 15 December 2021;
Published: 18 January 2022.
Edited by:
Elva G. Escobar-Briones, National Autonomous University of Mexico, MexicoReviewed by:
Fernanda Adame, Australian Rivers Institute, Griffith University, AustraliaCopyright © 2022 Asplund, Bonaglia, Boström, Dahl, Deyanova, Gagnon, Gullström, Holmer and Björk. This is an open-access article distributed under the terms of the Creative Commons Attribution License (CC BY). The use, distribution or reproduction in other forums is permitted, provided the original author(s) and the copyright owner(s) are credited and that the original publication in this journal is cited, in accordance with accepted academic practice. No use, distribution or reproduction is permitted which does not comply with these terms.
*Correspondence: Maria E. Asplund, bWFyaWEuYXNwbHVuZEBndS5zZQ==; Mats Björk, bWF0cy5iam9ya0BzdS5zZQ==
Disclaimer: All claims expressed in this article are solely those of the authors and do not necessarily represent those of their affiliated organizations, or those of the publisher, the editors and the reviewers. Any product that may be evaluated in this article or claim that may be made by its manufacturer is not guaranteed or endorsed by the publisher.
Research integrity at Frontiers
Learn more about the work of our research integrity team to safeguard the quality of each article we publish.