- 1School of Earth Sciences, The Ohio State University, Columbus, OH, United States
- 2Department of Freshwater and Marine Ecology, Institute for Biodiversity and Ecosystem Dynamics, University of Amsterdam, Amsterdam, Netherlands
- 3Geography, Geology and Environmental Science, University of Wisconsin, Whitewater, WI, United States
- 4School of Marine Science and Policy, University of Delaware, Lewes, DE, United States
Coral reefs are among the most diverse and complex ecosystems in the world that provide important ecological and economical services. Increases in sea surface temperature linked to global climate change threatens these ecosystems by inducing coral bleaching. However, it is not fully known if natural intra- or inter-annual physiological variability is linked to bleaching resilience or recovery capacity of corals. Here, we monitored the coral physiology of three common Caribbean species (Porites divaricata, Porites astreoides, Orbicella faveolata) at six time points over 2 years by measuring the following traits: calcification, biomass, lipids, proteins, carbohydrates, chlorophyll a, algal endosymbiont density, stable carbon isotopes of the host and endosymbiotic algae, and the stable carbon and oxygen isotopes of the skeleton. The overall physiological profile of all three species varied over time and that of P. divaricata was consistently different from the two other coral species. Porites divaricata had higher energy reserves coupled with higher contributions of heterotrophically derived carbon to host tissues than both P. astreoides and O. faveolata. Consistently higher overall energy reserves and heterotrophic contributions to tissues appear to buffer against environmental stress, including bleaching events. Thus, natural physiological variability among coral species appears to be a stronger predictor of coral bleaching resilience than intra- or inter-annual physiological variability within a coral species.
Introduction
Coral reef ecosystems have important ecological and economic value, as they provide critical habitat for associated species and support billions of dollars each year to the global economy (e.g., Costanza et al., 2014; Hoegh-Guldberg, 2015; Spalding et al., 2017). Unfortunately, coral reef ecosystems are threatened by increasing seawater temperatures associated with global climate change, leading to rising frequency and intensity of coral bleaching events (e.g., Teneva et al., 2012; Frieler et al., 2013; Cziesielski et al., 2019). Specifically, coral bleaching is the loss of endosymbiotic algae (family Symbiodiniaceae) and/or their photosynthetic pigments, giving corals a pale or white appearance, altering their energy budget, and eventually leading to their death (e.g., Hoegh-Guldberg and Smith, 1989; Glynn, 1996; Downs et al., 2002; Grottoli et al., 2014; Suggett and Smith, 2020). While coral vulnerability to heat-induced bleaching is well documented, the underlying natural variability in physiology could contribute to coral susceptibility and/or resilience to heat waves.
Several physiological traits associated with resistance to, and recovery from, coral bleaching fluctuate on a seasonal basis (e.g., Fitt et al., 2000; Rodrigues and Grottoli, 2007; Hagedorn and Carter, 2015) and include tissue thickness (e.g., Loya et al., 2001; Thornhill et al., 2011; Levas et al., 2013), energy reserves (i.e., lipids, proteins, carbohydrates) (e.g., Rodrigues and Grottoli, 2007; Anthony et al., 2009; Grottoli et al., 2014; Schoepf et al., 2015), Symbiodiniaceae species switching or shuffling (e.g., Abrego et al., 2008; Grottoli et al., 2014; Cunning et al., 2015), and heterotrophic plasticity (e.g., Grottoli et al., 2006; Levas et al., 2013). However, the link between natural temporal variability in physiology and bleaching remains poorly understood.
Temporal variability of coral physiological traits in situ has been reported for Hawaiian, Florida, western Pacific and Australian corals. Differences among species and location are typically based on a limited number of physiological traits such as skeletal δ13C, tissue bleaching, algal density, biomass or calcification (e.g., Fairbanks and Dodge, 1979; Grottoli and Wellington, 1999; Marshall and Baird, 2000; Stimson et al., 2002; Thornhill et al., 2011; Ross et al., 2015, 2018). Markers of energy reserves such as tissue biomass, protein, and carbohydrates are typically lowest in the summer or fall (e.g., Fitt et al., 2000; Rodrigues and Grottoli, 2007; Thornhill et al., 2011; Levas et al., 2013), while lipids are typically highest in the summer (e.g., Ben-David-Zaslow and Benayahu, 1999; Oku et al., 2003; Towle et al., 2015). In addition, all of these energy reserves often vary among species and sites (e.g., Fitt et al., 2000; Thornhill et al., 2011; Hinrichs et al., 2013; Towle et al., 2015). However, natural intra- and inter-annual variability in Caribbean coral energy reserves has not been investigated. In addition, Symbioniaceae and chlorophyll a concentrations are often at their lowest in mid-to-late summer when temperature and irradiance are at their peak values, and vice versa in the winter (e.g., Fagoonee, 1999; Fitt et al., 2000; Thornhill et al., 2011). Most coral bleaching events occur in late summer or early fall (e.g., Hoegh-Guldberg, 1999; Baker et al., 2008; Courtney et al., 2018) when protein and carbohydrate energy reserves, Symbiodiniaceae, and chlorophyll a are naturally low (e.g., Fitt et al., 2000; Oku et al., 2003; Rodrigues and Grottoli, 2007; Thornhill et al., 2011). Experimental bleaching studies have revealed that some corals with high energy reserves and photosynthetic pigments tend to be more resistant to bleaching when exposed to heat stress (e.g., Grottoli et al., 2006, 2014; Schoepf et al., 2015). Thus, coral species with relatively higher concentrations of energy reserves and photosynthetic pigments overall, or with higher concentrations in late summer, may be more resistant to natural in situ bleaching as well.
Heterotrophy is a critical source of fixed carbon for corals, especially when bleached (e.g., Carriquiry et al., 1994; Grottoli et al., 2006, 2014; Hughes et al., 2010; Connolly et al., 2012). Isotopic and feeding studies indicate that the proportionate contribution of heterotrophically acquired carbon varies seasonally but not for all species (e.g., Porter, 1976; Carriquiry et al., 1994; Felis et al., 1998; Grottoli and Wellington, 1999; Rodrigues and Grottoli, 2006; Levas et al., 2013, 2016; Schoepf et al., 2015). Yet, natural intra- and inter-annual variability in Caribbean coral heterotrophy has not yet been investigated. Lower heterotrophy before, during, and/or after bleaching has been linked to slower bleaching recovery (e.g., Grottoli et al., 2006, 2014; Rodrigues and Grottoli, 2006). Thus, corals with higher heterotrophic capacity and/or plasticity during the late summer, should be less susceptible to bleaching and likely to recover more quickly from thermal stress.
Finally, high coral calcification rates are often viewed as an indicator of coral health (e.g., Cortés and Risk, 1985; Guzmán et al., 1994), as this is an energetically costly process (e.g., Cohen and McConnaughey, 2002; Allemand et al., 2011). Calcification rates are typically higher in summer than in winter (e.g., Crossland, 1984; Lough and Barnes, 2000; Ross et al., 2018). Calcification increases with increasing temperatures up to an optimum (e.g., Jokiel et al., 1978; Marshall and Clode, 2004) and then decreases or stops when temperatures rise above a certain threshold (e.g., Leder et al., 1991; Reynaud et al., 2003; Rodrigues and Grottoli, 2006; Jokiel, 2011). Further, calcification is typically enhanced due to higher light availability in summer months (see Gattuso et al., 1999; Allemand et al., 2011 for review). When bleached, coral calcification dramatically decreases or stops (e.g., Carilli et al., 2009; Grottoli et al., 2014; Hagedorn et al., 2016; D’Olivo and McCulloch, 2017; Fisch et al., 2019), as stressed corals prioritize energy allocation toward maintaining metabolic demand and minimizing energy reserve losses (e.g., Edmunds and Davies, 1989; Cabral-Tena et al., 2013). Thus, corals that grow more slowly overall, or slower during the summer and early fall, may be less susceptible to bleaching and/or recover more quickly as they may already be prioritizing energy reserves or other metabolic processes over calcification.
Overall, the intra- and inter-annual variability in coral physiology could underlie coral bleaching susceptibility, severity, and persistence during recovery. To the best of our knowledge, only one study to date has assessed several physiological traits (i.e., chlorophyll a, Symbiodiniaceae density, colony brightness, and skeletal density) over a 2-year survey in Pocillopora damicornis on the Great Barrier Reef (Cooper et al., 2008). However, more studies are needed that comprehensively assess multiple physiological and isotopic traits of corals in situ over multiple years, particularly for Caribbean corals. Here, we measured seven physiological traits (e.g., calcification, biomass, lipid, protein, carbohydrate, chlorophyll a, Symbiodinaceae density, and tissue and skeletal isotopes) in the three Caribbean coral species Porites divaricata (branching morphology), Porites astreoides (mounding/encrusting morphology), and Orbicella faveolata (mounding, formerly Montastraea faveolata (Budd et al., 2012) from a common garden from July 2009 to June 2011. Using a multivariate approach, we quantified coral overall physiological variability over time and among species and identified the trait (s) that underly variability. Understanding how key physiological traits of coral health vary among species and over time can help us assess how natural variability in coral physiology potentially modulates resistance and resilience of corals to bleaching.
Materials and Methods
Coral Collection and Experimental Design
Coral collection methods were previously described in Grottoli et al. (2014). Briefly, six ramets from nine healthy (no bleaching visible) genets of Porites divaricata, Porites astreoides, and Orbicella faveolata were collected from 2.4 to 7.9 m depth from a reef near Puerto Morelos, Mexico. They were mounted on labeled PVC tiles, buoyantly weighed (Jokiel et al., 1978), and redeployed in a common garden at 4.9 m depth on the back reef (20°52.8150N, 86°50.9890W) on July 2009 where submersible loggers recorded the seawater temperature every hour. One ramet per genet was pre-assigned to a collection time of July 2009 (0 month), September 2009 (1.5 months), June 2010 (11 months), July 2010 (12 months), September 2010 (13.5 months), and June 2011 (23 months). At each time interval, all surviving ramets per species assigned to that time point were collected and missing or dead ramets were recorded. Ramets were buoyantly weighed and daily calcification rates were calculated as the difference between weights at the start of the study and collection time, divided by the respective number of days elapsed since the last measurement, and standardized to surface area. Coral fragments were then frozen at −80°C and shipped to The Ohio State University on dry ice and stored at −80°C for further analysis.
Tissue Biomass, Symbiont Density, Chlorophyll a, and Energy Reserves
Tissue biomass was quantified using methods described by McLachlan et al. (2020) from ground corals. Symbiont density was measured according to methods described in Warner et al. (2006) using 6 replicate counts on a hemocytometer. Chlorophyll a pigments were extracted using a double extraction and quantified spectrophotometrically with the equations of Jeffrey and Humphrey (1975). Symbiont density, chlorophyll a, and tissue biomass were all standardized to colony surface area using the aluminum foil method (Marsh, 1970).
Total soluble lipids (henceforth referred as lipids) were chloroform extracted from a whole, ground coral (∼1 cm2) sub-sample from each fragment according to established methods (e.g., Grottoli et al., 2004; Rodrigues and Grottoli, 2007). Animal soluble protein and carbohydrate (henceforth referred as protein and carbohydrate, respectively) were extracted from a second whole, ground coral sub-sample according to established methods (DuBois et al., 1956; Smith et al., 1985). The energetic value of lipid, protein, and carbohydrate concentrations were calculated and reported in Joules (Gnaiger and Bitterlich, 1984) and standardized to the ash-free dry weight (Grottoli et al., 2014).
Stable Isotopic Analysis
Tissue C isotopic analyses were performed on separated animal host and endosymbiont fractions using established methods (e.g., Rodrigues and Grottoli, 2006; Hughes et al., 2010). In brief, coral tissue was removed using an airbrush and the algal and animal fractions were separated by centrifugation. Each sample was individually combusted in a Costech Elemental Analyzer coupled to a Finnigan Delta Plus Advantage stable isotope ratio mass spectrometer (SIRMS) via a Conflo III open split interface at The Ohio State University. The carbon isotopic composition of the animal host (δ13Ch) and algal endosymbiont (δ13Ce) were reported as the per mil deviation of the stable isotopes 13C:12C relative to Vienna-Peedee Belemnite Limestone standard (v-PDB). The difference between δ13Ch and δ13Ce (δ13Ch–e) was calculated to determine the relative contribution of photoautotrophic vs. heterotrophic carbon to the internal DIC pool (e.g., Muscatine, 1989; Rodrigues and Grottoli, 2006; Price et al., 2021). Repeated measurements of the commercial standard USGS-24 had a standard deviation of ± 0.04‰.
Skeletal C and O isotopic analyses were performed following the established method by Grottoli et al. (2017). Briefly, the coral fragments were cleaned of tissue with high-pressure tap water and dried at room temperature. No additional cleaning was performed as it can lead to uncorrectable isotopic fractionation of the sample (Grottoli et al., 2005). Using a Dremel tool with a diamond-tipped drill bit, the top 100–200 μm of skeletal material was shaved off the tip of each coral fragment at the point of maximum linear extension. Each coral skeletal sample was finely ground and a 80–100 μg subsample was analyzed for δ13C and δ18O using an automated Carbonate Kiel device coupled to a Finnigan Delta IV SIRMS at The Ohio State University. Samples were acidified under vacuum with 100% ortho-phosphoric acid, and the resulting CO2 was cryogenically purified and delivered to the mass spectrometer. The standard deviation of repeated measurements of an internal carbonate standard (n = 40) was ± 0.03‰ for δ13C and ± 0.05‰ for δ18O. All δ13C and δ18O values were reported as the per mil deviation relative to the Vienna Peedee Belemnite Limestone Standard (v-PDB). In general, increases in coral skeletal δ13C (δ13Cs) are linked to greater carbon contribution via photosynthesis and/or decreased heterotrophic carbon incorporated in the tissues, as the pool of inorganic carbon available for calcification through respiration is enriched (e.g., Grottoli and Wellington, 1999; Grottoli, 2002). δ18O variability results from temperature-induced kinetic fractionation (e.g., Weber and Woodhead, 1972; Kim and O’Neil, 1997) relative to seawater δ18O changes and salinity (Epstein et al., 1953). In corals, skeletal δ18O (δ18Os) increases as temperature decreases and seawater δ18O increases (e.g., McConnaughey, 1989; Leder et al., 1996). However, when corals are bleached, dramatic decreases in calcification can lead to reduced fractionation, enhance equilibration with seawater, and increases in δ13Cs and δ18Os (e.g., Rodrigues and Grottoli, 2006; Grottoli et al., 2017).
Statistical Analysis
The number of alive, dead, and missing coral ramets of each species was recorded at each time point. Differences in mortality among species at each time point were evaluated by a Fisher’s exact test (Supplementary Table 1).
All multivariate statistics were performed using Primer software V6. Coral physiological profiles (i.e., calcification, biomass, lipids, proteins, carbohydrates, chlorophyll a, Symbiodiniaceae density, and δ13Ch–e) were compared among species with a multivariate one-way analysis of similarity (ANOSIMs; Clarke, 1993; 9999 permutations). Temporal differences in intraspecific and interspecific coral physiological profiles were compared by two separate multi-variate one-way PERMANOVAs (9999 permutations). Similarity percentage analysis (SIMPER) were performed to identify which of the physiological traits contributed the most to the differences among species. The data were visualized using non-metric multidimensional scaling (NMDS) plots.
Univariate statistics were performed with R software (v3.4.3) to evaluate how each individual physiological trait differed among species, genets, and over time. Assumptions of normality were evaluated by the Shapiro-Wilk test, and homoscedasticity was assessed by the Bartlett test. The δ18Os and δ13Ce were normally distributed. None of the other physiological traits were normally distributed but did meet assumptions of homoscedasticity. Since no genet effects were found for any physiological trait, it was removed from the models. Physiological trait differences among species and time points were compared by non-parametric Kruskal-Wallis tests, except for δ18Os and δ13Ce where two-ways ANOVAs were performed. Differences among groups for each physiological trait were compared by Tukey Post hoc tests.
Results
Daily average seawater temperature on the reef ranged from 24.2 to 31.4°C with the lowest temperatures in January–March and the warmest temperatures in July–September (Figure 1). No severe heat stress events (Bleaching threshold SST 29.8°C, MMM + 1°C) were recorded by Coral Reef Watch in this region during our 2-year survey (Quintana Roo station (5 km), NOAA Coral Reef Watch, 2019). Nevertheless, seawater temperature exceeded 29.8°C for 63 days in 2009 and 42 days in 2010 (National Data Buoy Center, 2021). The same pattern was observed for seawater recorded on the reef with ≥ 29.8°C temperatures for 80 days in 2009 and more than 27 days in 2010 (temperature logger failed during July and August 2010, Figure 1). This induced mild paling of some O. faveolata ramets in September of 2009 but no paling for the two Porites species (Supplementary Table 1). Mild paling was also observed for some O. faveolata ramets in June 2010 (11 months), September 2010 (13.5 months) and June 2011 (23 months), and in P. divaricata ramets in September 2010 (13.5 months) and June 2011 (23 months) (Supplementary Table 1).
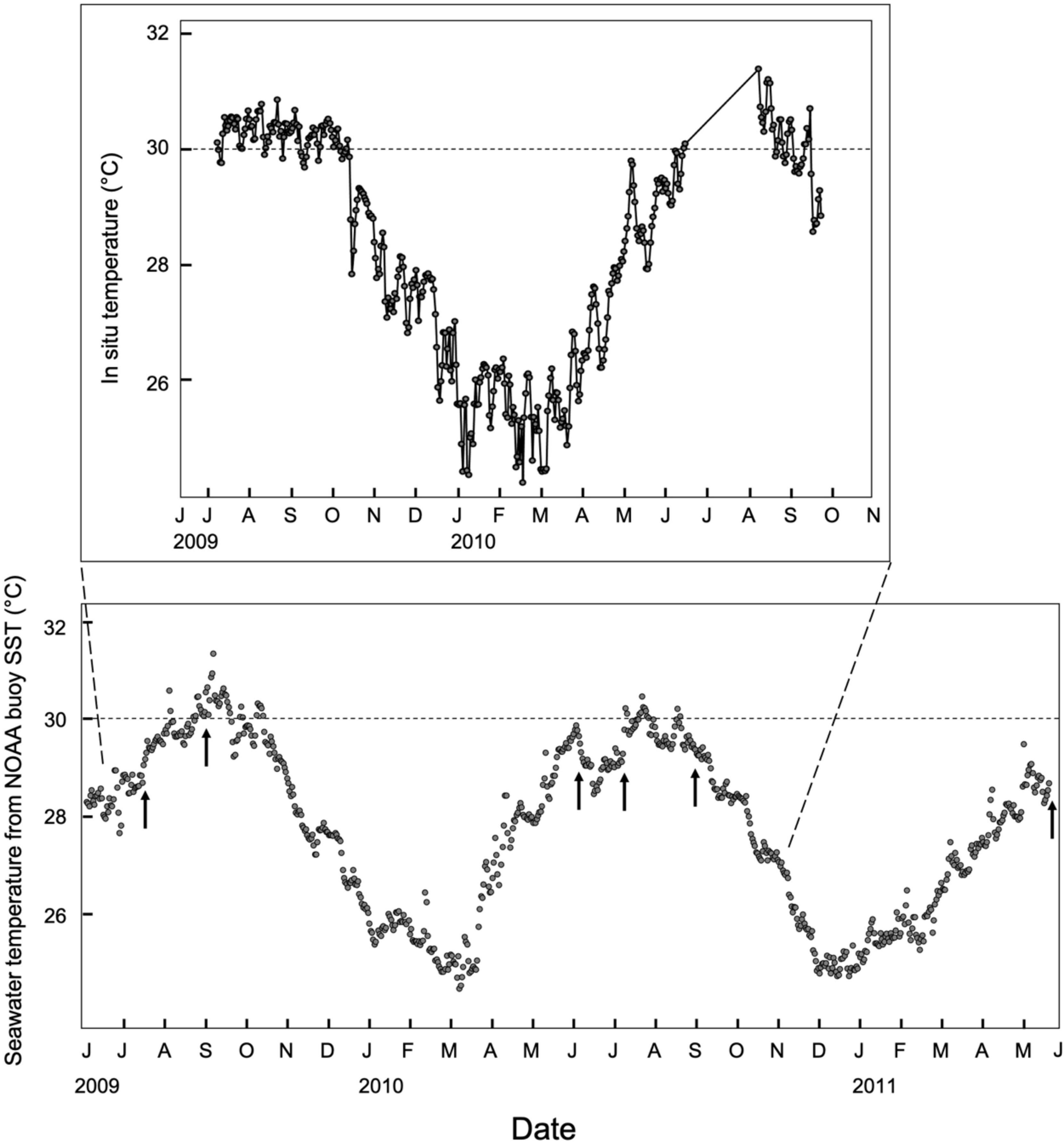
Figure 1. Average daily seawater temperature records between June 2009 and June 2011 from the NOAA buoy station 42058 central caribbean at 2 m depth (14°23′20″ N 74°48′10″ W) and inset with in situ temperature from temperature loggers deployed on the reef at the common garden beside the corals between June 2009 and November 2010 (20°52.8150N, 86°50.9890W). The local bleaching threshold (∼30°C) is represented by the dotted line. Months are indicated by their first letter and the year is indicated at the first listed month for that year. The sampling times are represented by the arrows.
While no mortality was observed for Porites coral fragments over time (Supplementary Figure 1 and Supplementary Table 1), two fragments of O. faveolata ramets died at 23 months (Supplementary Figure 1). Unfortunately, all the O. faveolata ramets from the 0-month timepoint were lost. In addition, the thin branches of P. divaricata ramets dislodged from the epoxy base over time resulting in one P. divaricata ramet being lost after 11 months, as well as four ramets after 12 months and five ramets after 13.5 months.
Variation in Physiological Profiles Among Species and Over Time
The physiological profile of P. divaricata differed significantly from that of P. astreoides and O. faveolata, though the latter two did not differ significantly from one other (Figure 2 and Table 1A). At least a third of the dissimilarity between species was due to total lipids and biomass, or biomass and carbohydrates (Table 1).
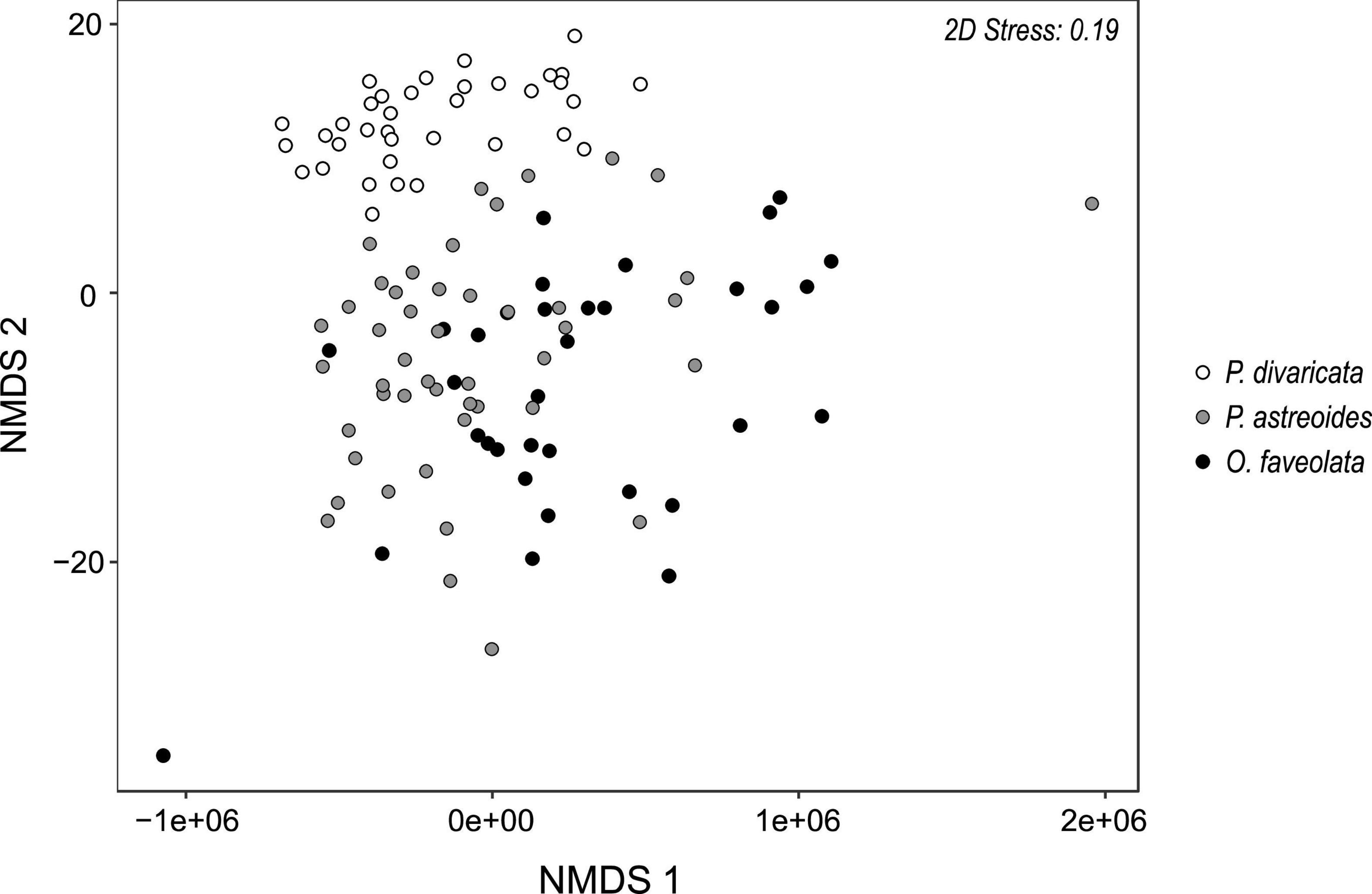
Figure 2. Multivariate NMDS plot (R = 0.33) for Porites divaricata (white), Porites astreoides (gray), and Orbicella faveolata (black) across all time points. Variables included in the analyses were calcification, lipids, proteins, carbohydrates, chlorophyll a, Symbiodiniaceae density, and δ13Ch–e. Corresponding ANOSIMs in Table 1.
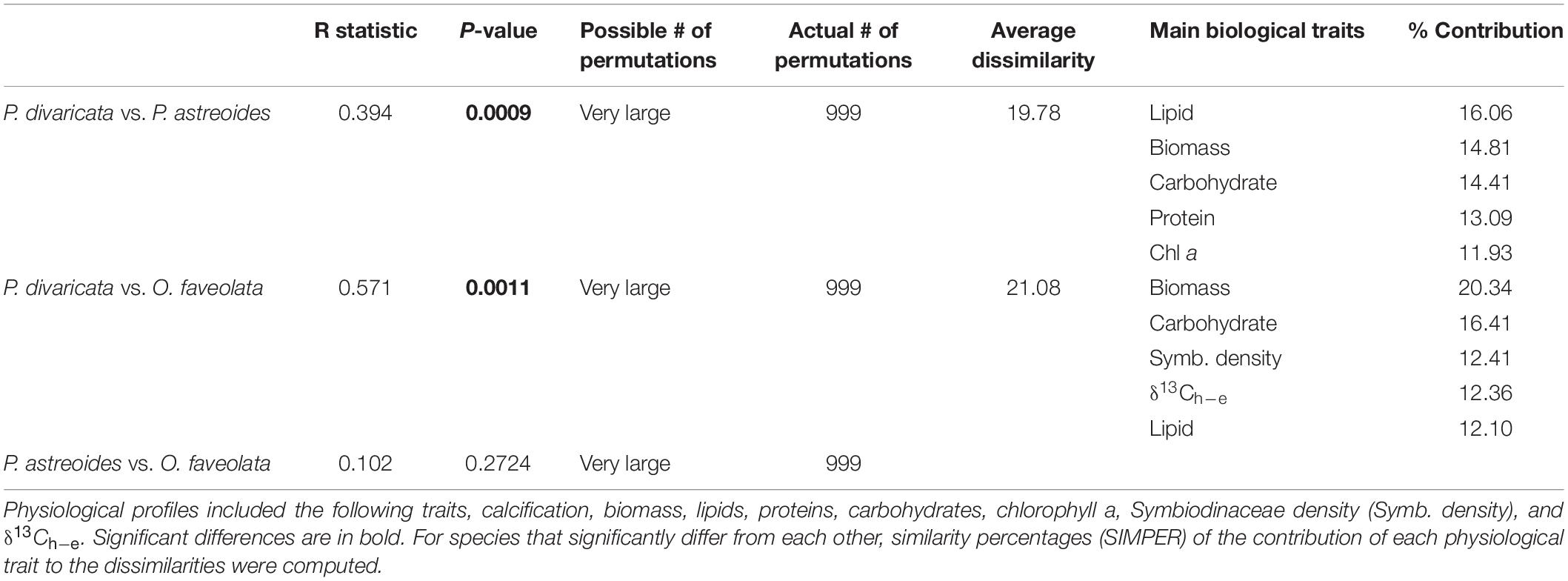
Table 1. Results of pair-wise tests of the one-way analysis of similarity (ANOSIM) of the physiological profiles of Porites divaricata, Porites astreoides, and Orbicella faveolata (global R = 0.33, p = 0.001).
The physiological profiles of each species also varied over time within and between years, with 93, 80, and 70% of time points significantly differing from each other within P. divaricata, P. astreoides, and O. faveolata, respectively (Table 2). The physiological profile of P. divaricata changed on intra- and interannual timescales and did not fluctuate on a predictable seasonal basis as the variability patterns differed between the observation years (Table 2). The physiological profiles of P. astreoides and O. faveolata also varied on intra- and interannual timescales, but their profiles returned to a common state in June of each years (i.e., no difference in their profiles at 11 and 23 months) (Table 2). Within each sampling month, the overall physiological profiles differed among two or more of the species during the first 11 months of the study (Figures 3A–C and Table 3). However, the physiological profiles of all three species always differed from each other throughout the entire second year of the study (Figures 3D–F and Table 3).
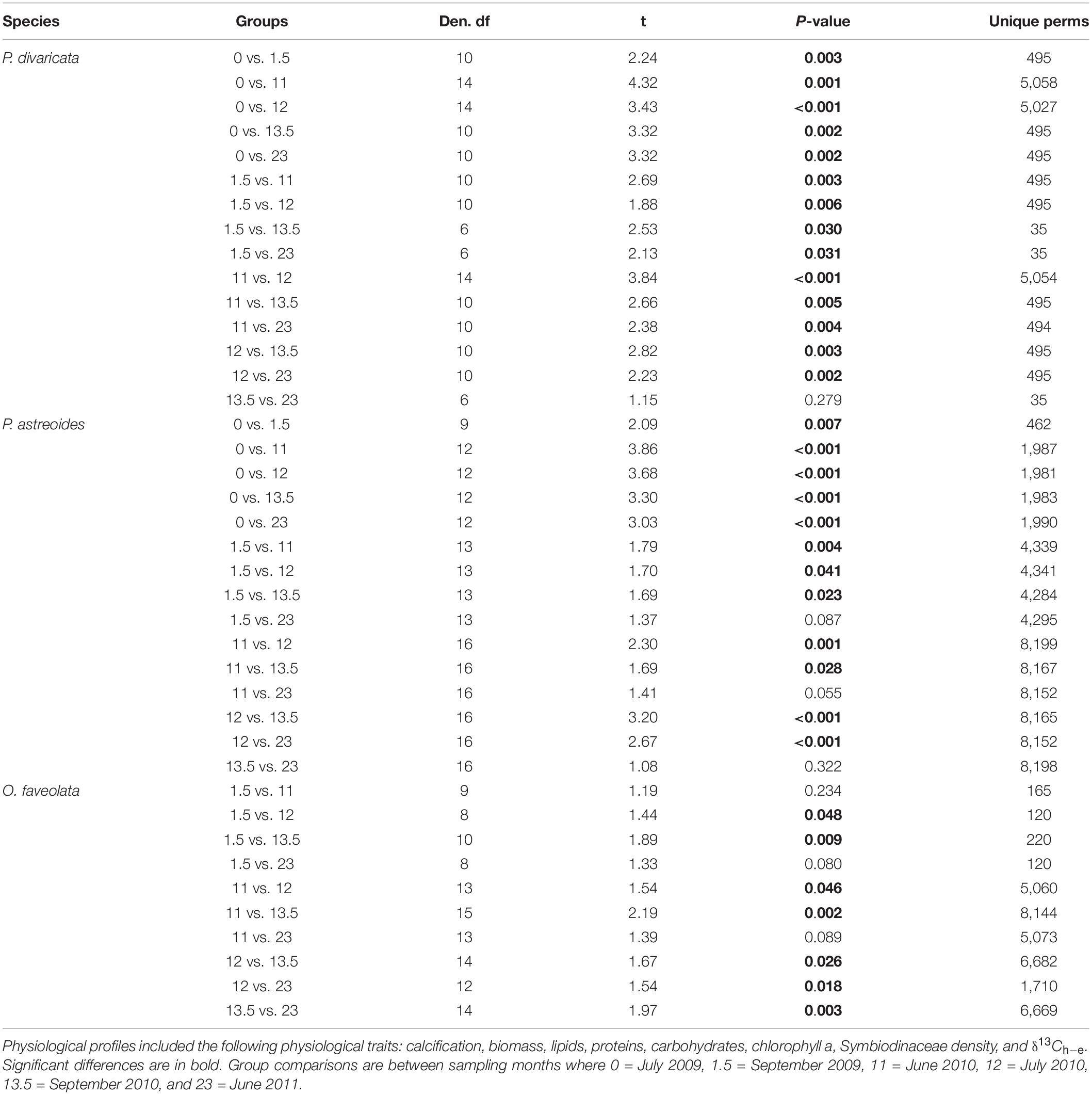
Table 2. Results of the pair-wise tests from the one-way PERMANOVAs testing the effect of time on coral physiological profiles of Porites divaricata, Porites astreoides, and Orbicella faveolata.
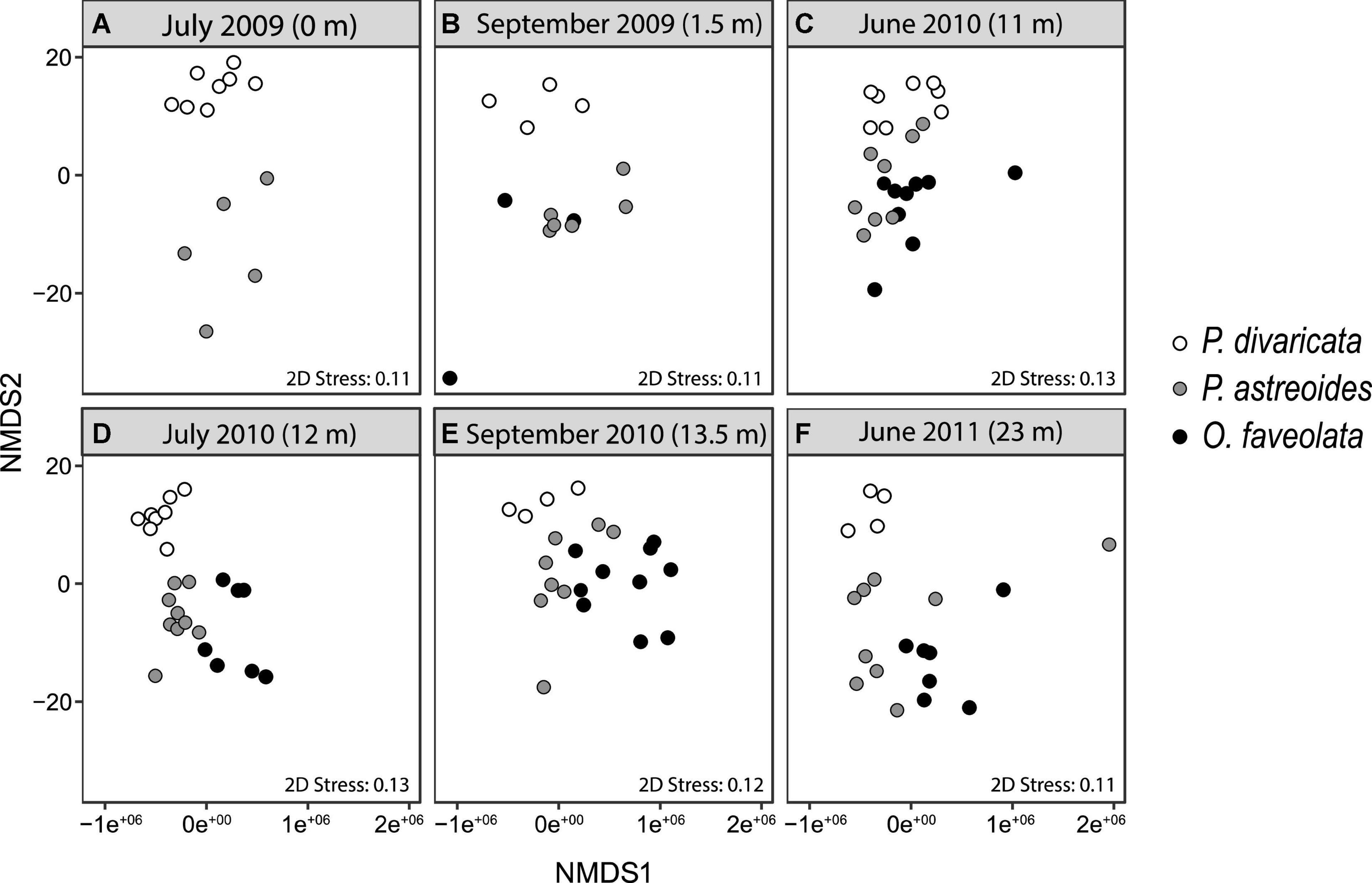
Figure 3. Multivariate NMDS plots for Porites divaricata (white), Porites astreoides (gray), and Orbicella faveolata (black) at (A–F) each time point (m = months). Variables included in the analyses were calcification, biomass, lipids, proteins, carbohydrates, chlorophyll a, Symbiodiniaceae density, and δ13Ch–e. Corresponding PERMANOVAs in Tables 2, 3.
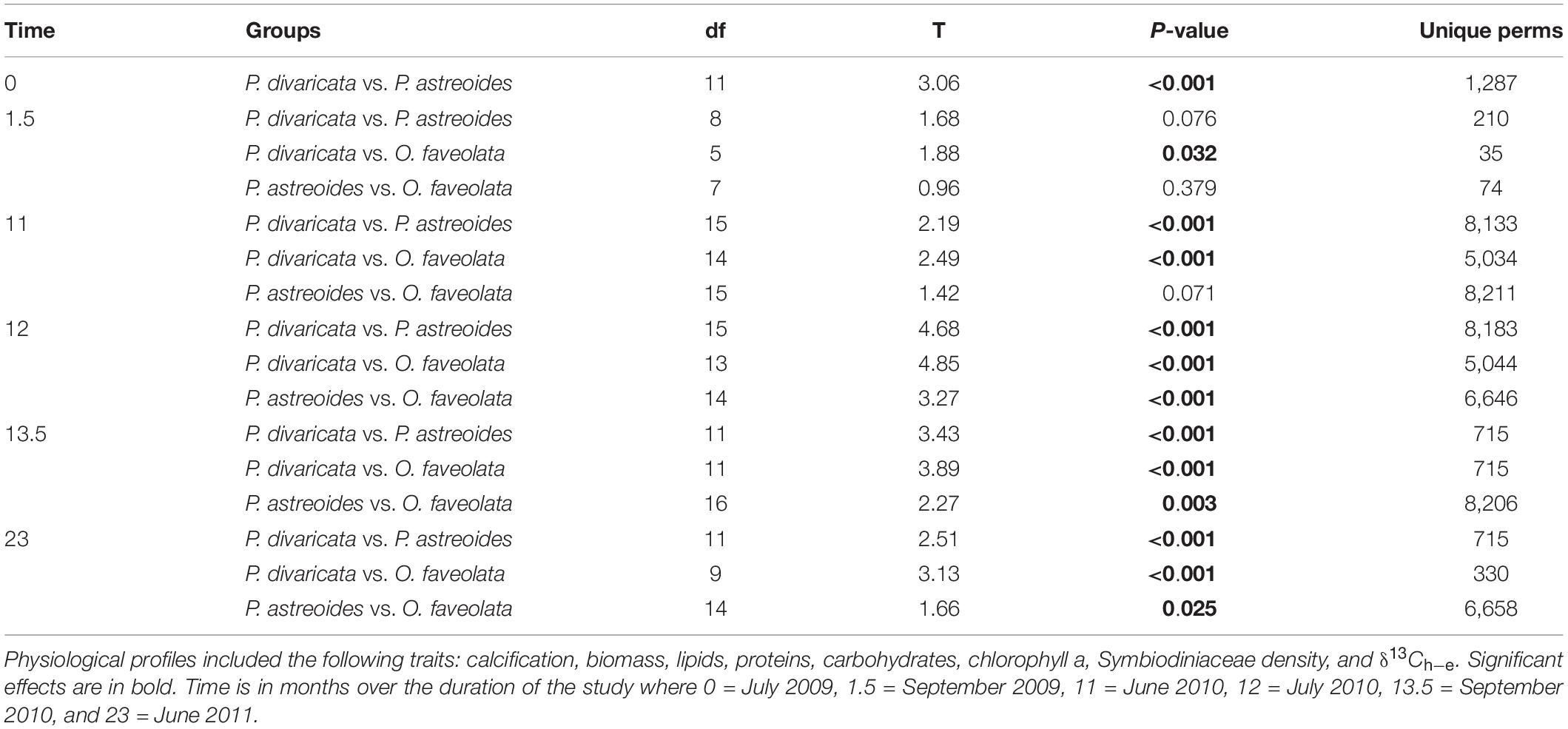
Table 3. Results of the pair-wises tests of the one-way PERMANOVAs testing differences among physiological profiles of coral species (Porites divaricata, Porites astreoides, Orbicella faveolata) within each time point.
Variation in Individual Physiological Traits Among Species and Over Time
For all species combined, there was a significant species by time interaction effect for the physiological traits of calcification, biomass, lipids, proteins, carbohydrates, chlorophyll a, Symbiodiniaceae density, and δ13Ch–e (Supplementary Table 2). Closer investigation revealed that carbohydrates was the only trait that differed between P. astreoides and O. faveolata (Supplementary Table 3) while P. divaricata differed from the other two species all traits except calcification and chlorophyll a (Supplementary Table 3).
Over time, calcification, biomass, Symbiodiniaceae density, and δ13Ch–e did not differ for P. divaricata (Figures 4A,B,G,H and Supplementary Table 4). In contrast, lipids, proteins, carbohydrates and chlorophyll a showed significant variability over time within and between year (Figures 4C–F and Supplementary Table 4). Lipid concentrations were generally higher the second year and proteins were higher at 13.5 months compared to 1.5 months (Figures 4C,D and Supplementary Table 4). Carbohydrates were higher at 0, 1.5, and 12 months compared to the rest of time points (Figure 4E and Supplementary Table 4) while chlorophyll a was highest at 0 months (late summer) and lowest at 11 months (early summer) (Figures 4E,F and Supplementary Table 4).
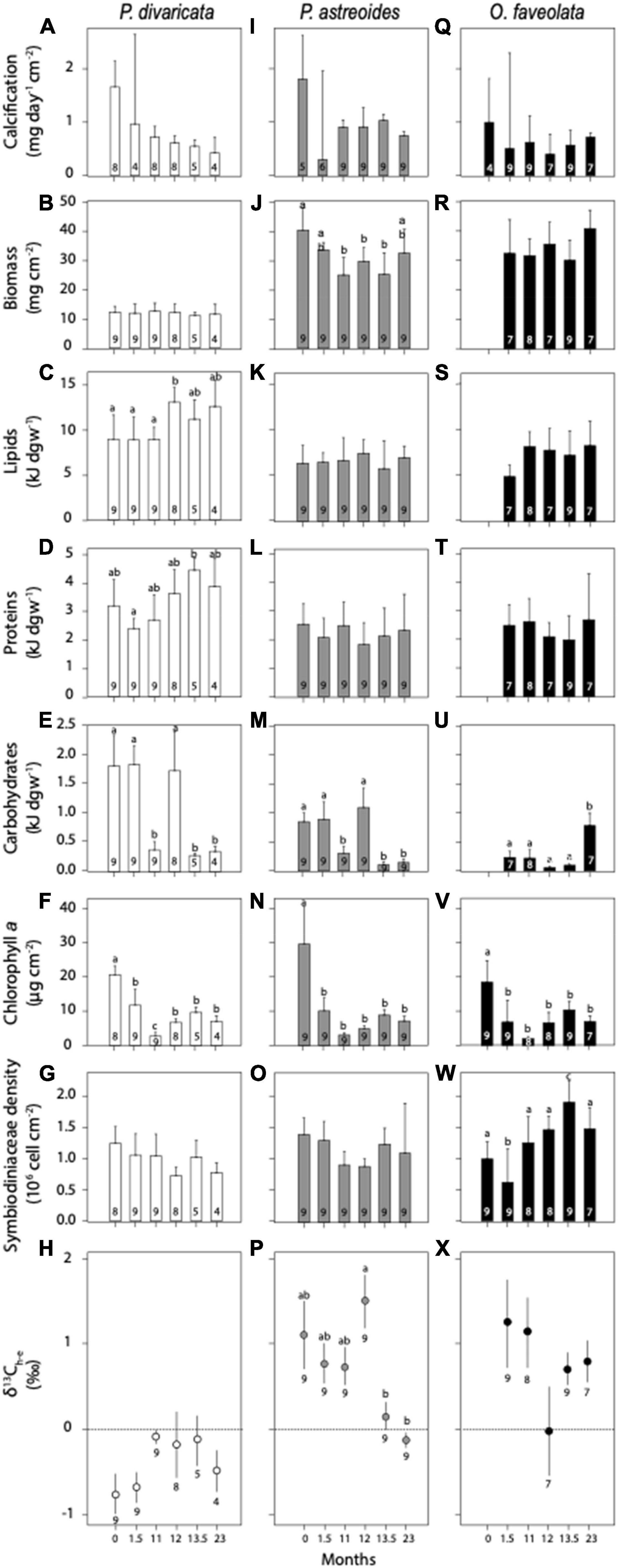
Figure 4. Average (± 1 SE) calcification, biomass, lipids, proteins, carbohydrates, chlorophyll a (Chl a), Symbiodiniaceae density, and δ13Ch–e of (A–H) Porites divaricata (white), (I–P) Porites astreoides (gray), (Q–X) Orbicella faveolata (black) at each time point (0 month = July 2009, 1.5 months = September 2009, 11 months = June 2010, 12 months = July 2010, 13.5 months = September 2010, and 23 months = June 2010). Averages that do not significantly differ from each other share similar letters or no letters. All Kruskal-Wallis, ANOVA, and Tukey post hoc results in Supplementary Tables 3, 4. Sample sizes are represented within each bar and below δ13Ch–e averages.
In P. astreoides, calcification, lipids, proteins, and symbiont density did not differ over time (Figures 4I,K,L,O and Supplementary Table 4). In contrast, biomass, carbohydrates, chlorophyll a and δ13Ch–e showed a significant temporal variability within and between years (Figures 4J,M,N,P and Supplementary Table 4). Biomass was higher at 0 months and lower at 11, 12, and 13.5 months (Figure 4J and Supplementary Table 4), and carbohydrate concentrations were higher at 0, 1.5, and 12 months (Figure 4M and Supplementary Table 4). Chlorophyll a was higher at 0 months (Figure 4N and Supplementary Table 4) and δ13Ch–e significantly declined over the course of the second year (Figure 4P and Supplementary Table 4).
Finally for O. faveolata, calcification, biomass, total lipids, proteins and δ13Ch–e did not differ over time (Figures 4Q–T,X and Supplementary Table 4). Carbohydrates were significantly higher and chlorophyll a significantly lower at the end of the study (Figures 4U,V and Supplementary Table 4), while Symbiodiniaceae density was the lowest at 1.5 months and highest at 13.5 months (Figure 4W).
Variation in Individual Isotopic Traits Among Species and Over Time
For all species combined, there was a significant species by time interaction effect for δ13Ce and δ18Os as well as significant species and time effects in δ13Ch and δ13Cs (Supplementary Table 5). Closer investigations revealed that P. divaricata differed isotopically from the other two species by having the lowest δ13Ch, δ13Cs, and δ13Os averages while P. astreoides had isotopically more depleted δ13Cs and δ13Os than O. faveolata (Figures 5, 6 and Supplementary Table 6). To investigate further, changes in each isotopic trait over time were evaluated within each species.
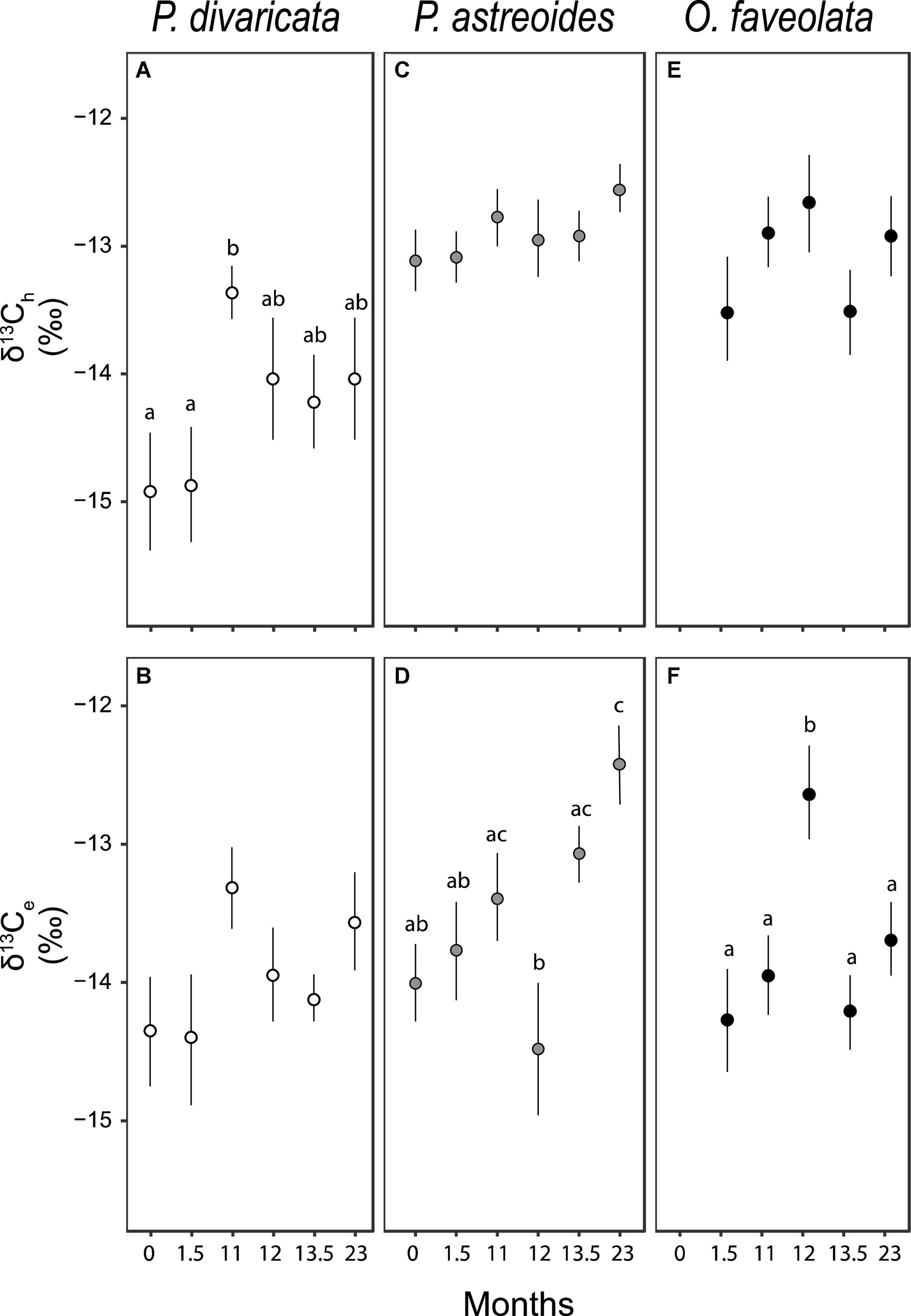
Figure 5. Average (± 1 SE) stable carbon isotopic values of (A,C,E) the animal host (δ13Ch) and (B,D,F) endosymbiont (δ13Ce) fractions of Porites divaricata (white), Porites astreoides (gray), and Orbicella faveolata (black) at each time point (0 month = July 2009, 1.5 months = September 2009, 11 months = June 2010, 12 months = July 2010, 13.5 months = September 2010, and 23 months = June 2010). Sample sizes range from 5 to 9 for each average. All Kruskal-Wallis, ANOVA and Tukey post hoc results in Supplementary Table 6.
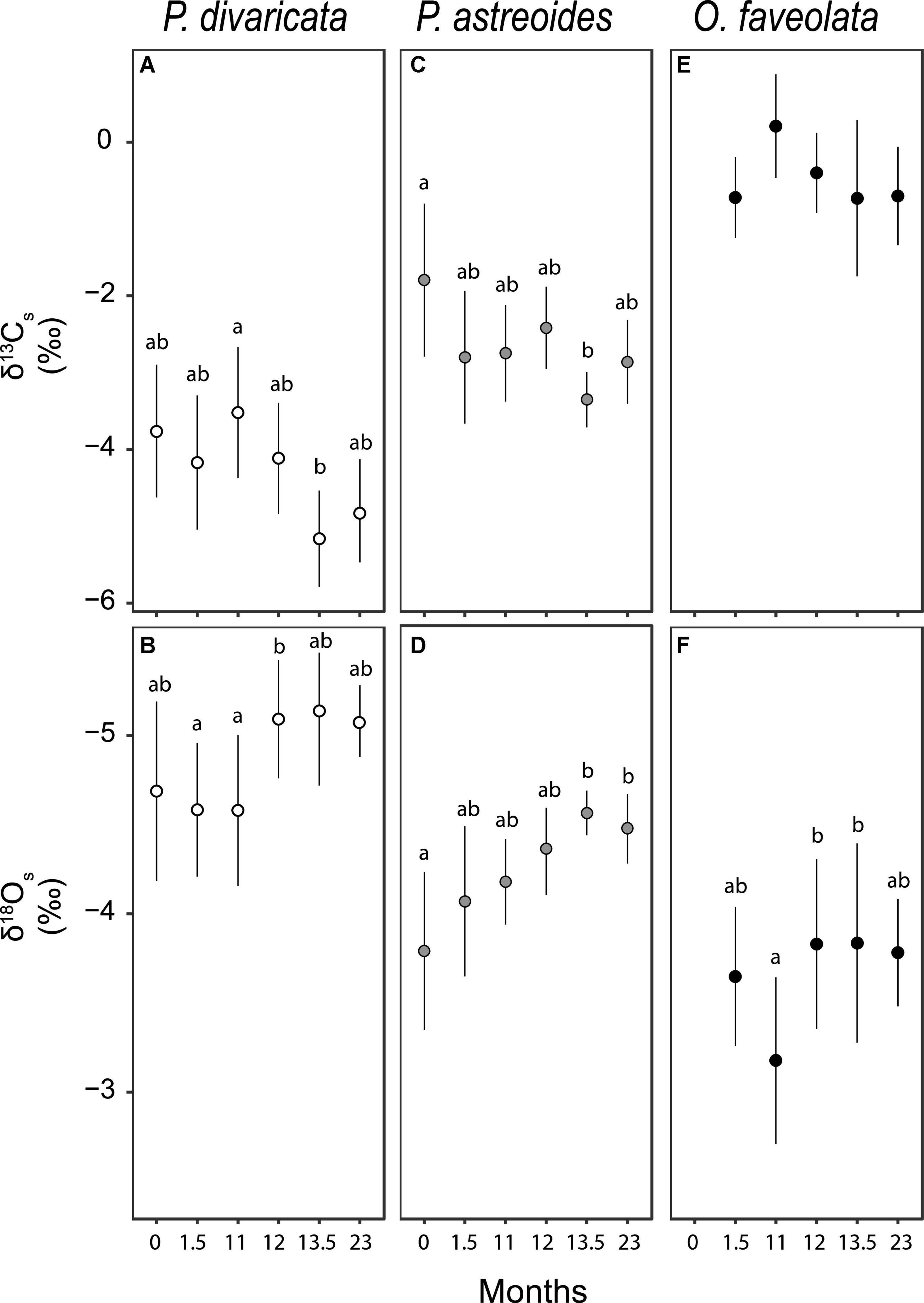
Figure 6. Average (± 1 SE) skeletal stable (A,C,E) carbon (δ13Cs) and (B,D,F) oxygen (δ18Os) isotopic values of Porites divaricata (white), Porites astreoides (gray) and Orbicella faveolata (black) at each time points (0 = July 2009, 1.5 = September 2009, 11 = June 2010, 12 = July 2010, 13.5 = September 2010, and 23 = June 2010). Sample sizes range from 5 to 9. Note that the y-axis values for δ18Os are inverted. All Kruskal-Wallis, ANOVA and Tukey post hoc results in Supplementary Table 6.
While the δ13Ch of P. divaricata differed over time with generally higher values in the second year, δ13Ce did not (Figures 5A,B and Supplementary Table 7). In contrast, δ13Ch in P. astreoides and O. faveolata did not change over time while their δ13Ce values did (Figures 5C–F and Supplementary Table 7). For P. astreoides,δ13Ce increased over the course of each year though only significantly so in the second year while δ13Ce was highest at 12 months in O. faveolata.
Porites divaricata δ13Cs exhibited a gradually declining trend over the course of the study, though significant differences were only detected between 11 and 13.5 months (Figure 6A and Supplementary Table 7). δ18Os also showed a gradually decreasing trend over the course of the study, though significant differences were only detected between 12 and 1.5 and 11 months (Figure 6B and Supplementary Table 7). In P. astreoides, δ13Cs and δ18Os generally decreased over time while in O. faveolataδ13Cs did not change over time and δ18Os was lowest at 11 months and highest at 12 and 13.5 months (Figures 6C–F and Supplementary Table 7).
Discussion
While variations in coral physiological profiles over time were observed within and among all three species, calcification rate was the only trait that did not fluctuate over the 2-year period nor among species. This suggested that these species were stable in their habitat during this 2-year survey despite natural environmental and other physiological fluctuations.
Porites divaricata Differed From Both Porites astreoides and Orbicella faveolata
Porites divaricata exhibited strong temporal variability and was distinctly different from the other two species at the physiology profile and individual trait levels throughout most of the study (Figures 2–4). This species contained the highest relative concentrations of lipid, protein and carbohydrate despite having the lowest biomass compared to P. astreoides and O. faveolata (Figures 4B–D,J–L,R–T), which is consistent with previous experimental studies using the same genets (e.g., Schoepf et al., 2015; Levas et al., 2018). Porites divaricata was the only species to exhibit temporal variability in all three of its energy reserves (i.e., lipids, protein, and carbohydrates) predominantly on an inter-annual basis (Figures 4C–E), suggesting that this species modulates these biomolecules in response to inter-annual variability in environmental conditions (e.g., heat wave events). The dynamic nature of lipids in Porites corals is well documented with post-bleaching catabolism of lipids in P. divaricata (e.g., Schoepf et al., 2015; Levas et al., 2018), seasonal variability in P. compressa lipids in Hawaii (Stimson, 1987), and intra and inter-annual variability in Porites sp. triacylglycerol (Carilli et al., 2012).
There was also noticeable temporal variability in chlorophyll a concentration with higher values the first year coupled with higher late summer concentrations compared to other time points (Figure 4F). This intra and inter- annual variability coupled with stable Symbiodiniaceae density indicates that P. divaricata modulates its chlorophyll a concentration per cell throughout the year most likely in response to seasonal temperature and light fluctuations (e.g., Brown et al., 1999; Hoogenboom et al., 2012). This suggests that P. divaricata is able to maintain their endosymbionts density across the range of temperatures experienced during the study. The trend in chlorophyll a was the same across all coral species, as they all show a cyclical pattern that most likely is driven by seasonal photoacclimation. In fact, all species appear to have photoacclimatized to the common garden location as suggested with the decrease in chlorophyll a after 1.5 months at the site (Figures 4F,N,V). Unfortunately, algal symbiont type was not monitored in our corals over time in our field study. However, algal symbiont type was quantified in ramets of the same coral genets in an accompanying bleaching experiment that showed that P. divaricata and O. faveolata shuffle their algal symbiont to favor more thermally tolerant ones following heat stress while P. astreoides cannot (Grottoli et al., 2014). Thus, photoacclimation in this field study occurred in all three species while algal symbiont types were probably shifting in two of the three species.
Porites divaricata δ13Ch–e and δ13Ch values were lower than in the other species (Figures 4H,P,X, 5A,C,E), suggesting that this coral incorporates a higher proportion of heterotrophically derived carbon that could be due to its higher uptake and/or lower release of dissolved organic carbon than the other two species (Levas et al., 2016). This is consistent with previous experimental studies showing the lowest δ13Ch values in non-bleached control P. divaricata compared to the other two coral species (Schoepf et al., 2015; Levas et al., 2018). Porites divaricata was also the only species with natural intra- and interannual variability in δ13Ch values (Figure 5A), suggesting that it has a greater capacity to modulate the proportion of heterotrophic derived carbon into its tissues on these time scales. Heterotrophic plasticity can lead to higher resilience in corals to global change (e.g., Grottoli et al., 2006; Hughes and Grottoli, 2013; Baumann et al., 2014; Conti-Jerpe et al., 2020), suggesting that P. divaricata may overcome thermal stress by increasing their heterotrophic acquisition of carbon. δ13Cs values of P. divaricata were the lowest compared to the other species (Figures 6A,C,E), which is also consistent with higher heterotrophic incorporation (e.g., Carriquiry et al., 1994; Grottoli and Wellington, 1999). While decreased light can also result in lower δ13Cs (e.g., Fairbanks and Dodge, 1979; Falkowski et al., 1984; Carriquiry et al., 1994; Grottoli and Wellington, 1999; Grottoli, 2002), all three species were exposed to the same light environment in the common garden, leaving only differences in heterotrophy as the most likely driver for the offset in δ13Cs in P. divaricata compared to the other two species (Figures 5, 6).
Porites astreoides and Orbicella faveolata
While the overall physiological profiles of P. astreoides and O. faveolata exhibited significant temporal variability, they typically did not differ from each other (Figures 2–5). This temporal variability was mostly driven by biomass, carbohydrates, chlorophyll a, and δ13Ch–e in P. astreoides and the same variables plus Symbiodiniaceae density in O. faveolata (Figure 4). In addition to photoacclimation to the common garden site (Figure 4N,V), P. astreoides exhibited stable Symbiodiniaceae density through the survey suggesting a potential capacity to maintain their endosymbiont density across the range of temperatures experienced during the study (Figure 4O). Orbicella faveolata was the only species to show intra- and interannual variability in both chlorophyll a and Symbiodiniaceae density (Figure 4W). This is consistent with Fitt et al. (2000) observations on O. faveolata, showing a seasonality in both chlorophyll a and Symbiodiniaceae density with lower values in late summer/early fall compared to spring/winter. Thus, O. faveolata photoacclimated its pigments to the common garden conditions but additional modulation of Symbiodinaceae may be a compensating response to the range of temperatures experienced during the study. However, at heat stress temperatures that induce severe bleaching, Symbiodinaceaea density and chlorophyll a dramatically decrease in both species thought O. faveolata recovers faster than P. astreoides following repeat bleaching (Grottoli et al., 2014; Schoepf et al., 2015; Levas et al., 2018). The field observations presented here confirm that O. faveolata was able to increase their Symbiodiniaceae density in response to two consecutive years of warm summers whereas P. astreoides cannot.
Carbohydrates were the only energy reserve that varied over time and among all three species with the lowest values observed for O. faveolata (Figures 4E,M,U). Interestingly, both Porites species displayed the same temporal pattern with lower values in early summer (June) which then increased during summer (July) and decreased again for fall (for September 2010). It seems that the carbohydrate values mostly followed temperature fluctuations in 2010. Previous experimental studies demonstrated that all three coral species catabolized carbohydrates in responses to bleaching inducing heat stress, though P. divaricata consistently had the highest concentrations compared to the other two species (e.g., Schoepf et al., 2015; Levas et al., 2018). The observed stable lipid and protein concentrations (Figures 4C–D,K–L,S–T) are consistent with other in situ and experimental data from P. astreoides and O. faveolata over time (e.g., Teece et al., 2011; Schoepf et al., 2015; Levas et al., 2018), but need to be considered with caution as we do not have results from O. faveolata at time 0 (July 2009).
P. astreoides and O. faveolata had higher δ13Ch–e values than P. divaricata (Figures 4H,P,X), suggesting that the two former species incorporated a lower proportion of heterotrophically derived carbon into its tissues. This is not consistent with observations from manipulative tank experiments where δ13Ch–e values were not always lowest for P. divaricata compared to P. astreoides and O. faveolata (e.g., Schoepf et al., 2015; Levas et al., 2018). This suggests that coral carbon budgets may change under experimental conditions or under more extreme heat stress conditions, and highlights the importance of field observations. Variation in δ13Ch–e was driven by temporal variability in δ13Ce values as δ13Ch did not vary over time (Figures 5C–F), suggesting a stronger influence of photosynthetically derived carbon to the pool of carbon in tissues relative to heterotrophically derived carbon in both species. This is very interesting since P. astreoides and O. faveolata host very different dominant species of Symbiodiniaceae (Grottoli et al., 2014).
Implications for Coral Bleaching and Resilience
This long-term in situ study highlights the significant natural variability in coral physiological profiles among coral species and over time. The physiological profile and isotopic signatures of P. divaricata were distinct from P. astreoides and O. faveolata throughout the study. Porites divaricata had the highest energy reserve stores (i.e., lipids, protein, carbohydrates) and the highest heterotrophic tissue contributions of all three species—two traits that are associated with lower susceptibility and higher resilience against bleaching (e.g., Grottoli et al., 2006; Anthony et al., 2009; Conti-Jerpe et al., 2020). While these traits varied on an intra-annual basis, they were not necessarily higher in late summer or early fall as would be predicted if corals modulated energy reserves and heterotrophy on a seasonal basis as a strategic mechanism to buffer themselves seasonally warm waters in late summer and possible thermal stress. However, the timing of spawning in these species may play a large role in the temporal variations in lipid levels. In fact, P. divaricata spawning peaks in spring while P. astreoides’ spawning season can last from January to September and O. faveolata from August to September (e.g., Szmant, 1986; McDermond, 2014). Thus, P. divaricata has a greater period of time to rebuild lipid levels lost during spawning before seawater temperatures reach maximum values in late summer. This may allow P. divaricata to be more competitive than P. astreoides and O. faveolata in a warming ocean where bleaching events can occur annually.
With comparable calcification rates among all species, there was also no apparent trade-off between skeletal growth and energy reserves or the relative contributions of photoautotrophic and heterotrophic carbon. Instead, consistently higher overall energy reserves and heterotrophic contributions to tissues in P. divaricata appear to buffer against intra- and interannual variability in environmental conditions, including bleaching events. However, the high variability in calcification among genets at 1.5 months when seawater temperature was the highest indicates that some individuals of all three species were stressed from the longer than usual warm period in 2009. Interestingly, despite a loss in symbiont density at 1.5 months, O. faveolata maintained calcification suggesting a high capacity to translocate carbon from the remaining Symbiodiniaceae or catabolism of lipid stores though additional research is needed to test this. Experimental evidence supports the buffering hypothesis (i.e., high energy reserves and heterotrophic capacity and/or plasticity) as P. divaricata was the most tolerant of the three species to repeated experimental bleaching stress with some capacity for shuffling to heat tolerant Symbiodiniaceae species as well (e.g., Grottoli et al., 2014; Schoepf et al., 2015; Levas et al., 2016). These strategies were also observed in manipulative experiments of bleached Hawaiian and Red Sea corals, where species with high energy reserves and/or heterotrophic carbon contributions are more tolerant to heat stress and/or recover more quickly (e.g., Grottoli et al., 2006; Rodrigues and Grottoli, 2007; Grottoli et al., 2017). Thus, variability in physiological profiles among coral species appears to be a better predictor for coral bleaching resilience than temporal, intra- and/or interannual physiological variability within a coral species.
Data Availability Statement
The datasets presented in this study can be found in online repositories. The names of the repository/repositories and accession number(s) can be found below: www.bco-dmo.org at www.bco-dmo.org/project/516103.
Author Contributions
AG and MW designed the study. LC did the statistical approach, analyzed the data, and draft the manuscript. AG, VS, SL, and MA participated in the fieldwork. VS and MA carried out laboratory analyses. All authors contributed to revising the manuscript.
Funding
This work was funded by the National Science Foundation with OCE#0825490 and OCE#1459536 to AG and OCE#0825413 to MW. Partial support was provided by the United States Geological Survey (Grant No. GR117846) to AG. All work undertaken in this study complied with the current laws of Mexico and the United States of America.
Conflict of Interest
The authors declare that the research was conducted in the absence of any commercial or financial relationships that could be construed as a potential conflict of interest.
Publisher’s Note
All claims expressed in this article are solely those of the authors and do not necessarily represent those of their affiliated organizations, or those of the publisher, the editors and the reviewers. Any product that may be evaluated in this article, or claim that may be made by its manufacturer, is not guaranteed or endorsed by the publisher.
Acknowledgments
We thank R. Iglesias-Prieto, A. Banaszak, S. Enriquez, R. Smith, and the staff of the Instituto de Ciencias del Mar y Limnologia, Universidad Nacional Autonoma de Mexico, in Puerto Morelos for their generous time and logistical support. We also thank T. Huey, D. Borg, E. Zebrowski, J. Scheuermann, M. McBride, J. Baumann, and I. Kuffner for help in the field and laboratory and for feedback.
Supplementary Material
The Supplementary Material for this article can be found online at: https://www.frontiersin.org/articles/10.3389/fmars.2021.811055/full#supplementary-material
References
Abrego, D., Ulstrup, K. E., Willis, B. L., and van Oppen, M. J. H. (2008). Species–specific interactions between algal endosymbionts and coral hosts define their bleaching response to heat and light stress. Proc. R. Soc. B 275, 2273–2282. doi: 10.1098/rspb.2008.0180
Allemand, D., Tambutté, É, Zoccola, D., and Tambutté, S. (2011). “Coral calcification, cells to reefs,” in Coral Reefs: An Ecosystem in Transition, eds Z. Dubinsky and N. Stambler (Dordrecht: Springer), 119–150. doi: 10.1007/978-94-007-0114-4_9
Anthony, K. R. N., Hoogenboom, M. O., Maynard, J. A., Grottoli, A. G., and Middlebrook, R. (2009). Energetics approach to predicting mortality risk from environmental stress: a case study of coral bleaching. Funct. Ecol. 23, 539–550. doi: 10.1111/j.1365-2435.2008.01531.x
Baker, A. C., Glynn, P. W., and Riegl, B. (2008). Climate change and coral reef bleaching: an ecological assessment of long-term impacts, recovery trends and future outlook. Estuar. Coast. Shelf Sci. 80, 435–471. doi: 10.1016/j.ecss.2008.09.003
Baumann, J., Grottoli, A. G., Hughes, A. D., and Matsui, Y. (2014). Photoautotrophic and heterotrophic carbon in bleached and non-bleached coral lipid acquisition and storage. J. Exp. Mar. Biol. Ecol. 461, 469–478. doi: 10.1016/j.jembe.2014.09.017
Ben-David-Zaslow, R., and Benayahu, Y. (1999). Temporal variation in lipid, protein and carbohydrate content in the Red Sea soft coral Heteroxenia fuscescens. J. Mar. Biol. Ass. 79, 1001–1006.
Brown, B. E., Dunne, R. P., Ambarsari, I., Le Tissier, M. D. A., and Satapoomin, U. (1999). Seasonal fluctuations in environmental factors and variations in symbiotic algae and chlorophyll pigments in four Indo-Pacific coral species. Mar. Ecol. Prog. Ser. 191, 53–69. doi: 10.3354/meps191053
Budd, A. F., Fukami, H., Smith, N. D., and Knowlton, N. (2012). Taxonomic classification of the reef coral family Mussidae (Cnidaria: Anthozoa: Scleractinia): classification of reef corals. Zool. J. Linn. Soc. 166, 465–529. doi: 10.1111/j.1096-3642.2012.00855.x
Cabral-Tena, R., Reyes-Bonilla, H., Lluch-Cota, S., Paz-García, D., Calderón-Aguilera, L., Norzagaray-López, O., et al. (2013). Different calcification rates in males and females of the coral Porites panamensis in the Gulf of California. Mar. Ecol. Prog. Ser. 476, 1–8.
Carilli, J., Donner, S. D., and Hartmann, A. C. (2012). Historical temperature variability affects coral response to heat stress. PLoS One 7:e34418. doi: 10.1371/journal.pone.0034418
Carilli, J. E., Norris, R. D., Black, B. A., Walsh, S. M., and McField, M. (2009). Local stressors reduce coral resilience to bleaching. PLoS One 4:e6324. doi: 10.1371/journal.pone.0006324
Carriquiry, J. D., Risk, M. J., and Schwarcz, H. P. (1994). Stable isotope geochemistry of corals from Costa Rica as proxy indicator of the El Niño/Southern Oscillation (ENSO). Geochim. Cosmochim. Acta 58, 335–351.
Clarke, K. R. (1993). Non-parametric multivariate analyses of changes in community structure. Aust. J. Ecol. 18, 117–143. doi: 10.1111/j.1442-9993.1993.tb00438.x
Cohen, A. L., and McConnaughey, T. A. (2002). Geochemical perspectives on coral mineralization. Rev. Min. Geochem. 54, 151–187. doi: 10.1515/9781501509346-011
Connolly, S. R., Lopez-Yglesias, M. A., and Anthony, K. R. N. (2012). Food availability promotes rapid recovery from thermal stress in a scleractinian coral. Coral Reefs 31, 951–960.
Conti-Jerpe, I. E., Thompson, P. D., Wong, C. W. M., Oliveria, N. L., Duprey, N. N., Moynihan, M. A., et al. (2020). Trophic strategy and bleaching resistance in reef-building corals. Sci. Adv. 6:eaaz5443. doi: 10.1126/sciadv.aaz5443
Cooper, T. F., Ridd, P. V., Ulstrup, K. E., Humphrey, C., Slivkoff, M., and Fabricius, K. E. (2008). Temporal dynamics in coral bioindicators for water quality on coastal coral reefs of the Great Barrier Reef. Mar. Freshw. Res. 59:703.
Cortés, J. N., and Risk, M. J. (1985). A reef under siltation stress: Cahuita, Costa Rica. Bull. Mar. Sci. 36:18.
Costanza, R., de Groot, R., Sutton, P., van der Ploeg, S., Anderson, S. J., Kubiszewski, I., et al. (2014). Changes in the global value of ecosystem services. Glob. Environ. Change 26, 152–158.
Courtney, T. A., De Carlo, E. H., Page, H. N., Bahr, K. D., Barro, A., Howins, N., et al. (2018). Recovery of reef-scale calcification following a bleaching event in Kāne’ohe Bay, Hawai’i: post-bleaching recovery of reef-scale calcification. Limnol. Oceanogr. 3, 1–9. doi: 10.1002/lol2.10056
Crossland, C. (1984). Seasonal variations in the rates of calcification and productivity in the coral Acropora formosa on a high-latitude reef. Mar. Ecol. Prog. Ser. 15, 135–140.
Cunning, R., Silverstein, R. N., and Baker, A. C. (2015). Investigating the causes and consequences of symbiont shuffling in a multi-partner reef coral symbiosis under environmental change. Proc. R. Soc. B 282:20141725. doi: 10.1098/rspb.2014.1725
Cziesielski, M. J., Schmidt-Roach, S., and Aranda, M. (2019). The past, present, and future of coral heat stress studies. Ecol. Evol. 9, 10055–10066. doi: 10.1002/ece3.5576
D’Olivo, J. P., and McCulloch, M. T. (2017). Response of coral calcification and calcifying fluid composition to thermally induced bleaching stress. Sci. Rep. 7:2207. doi: 10.1038/s41598-017-02306-x
Downs, C. A., Fauth, J. E., Halas, J. C., Dustan, P., Bemiss, J., and Woodley, C. M. (2002). Oxidative stress and seasonal coral bleaching. Free Radic. Biol. Med. 33, 533–543. doi: 10.1016/s0891-5849(02)00907-3
DuBois, M., Gilles, K. A., Hamilton, J. K., Rebers, P. A., and Fred, S. (1956). Colorimetric method for determination of sugars and related substances. Anal. Chem. 28, 350–356. doi: 10.1021/ac60111a017
Edmunds, P. J., and Davies, P. S. (1989). An energy budget for Porites porites (Scleractinia), growing in a stressed environment. Coral Reefs 8, 37–43. doi: 10.1007/bf00304690
Epstein, S., Buchsbaum, R., Lowenstam, H. A., and Urey, H. C. (1953). Revised carbonate-water isotopic temperature scales. Bull. Geol. Soc. Am. 64, 1315–1326. doi: 10.1130/0016-7606(1953)64[1315:rcits]2.0.co;2
Fagoonee, I. (1999). The dynamics of Zooxanthellae populations: a long-term study in the field. Science 283, 843–845. doi: 10.1126/science.283.5403.843
Fairbanks, R. G., and Dodge, R. E. (1979). Annual periodicity to the 18O/16O and 13C/12C ratios in the coral Montastrea annularis. Geochim. Cosmochim. Acta 43, 10009–11020.
Falkowski, P. G., Dubinsky, Z., Muscatine, L., and Porter, J. (1984). Light and the bioenergetics of a symbiotic coral. Bioscience 34, 705–709.
Felis, T., Patzold, J., Loya, Y., and Wefer, G. (1998). Vertical water mass mixing and plankton blooms recorded in skeletal stable carbon isotopes of a Red Sea coral. J. Geophys. Res. Atmos. 103, 30731–30739. doi: 10.1029/98jc02711
Fisch, J., Drury, C., Towle, E. K., Winter, R. N., and Miller, M. W. (2019). Physiological and reproductive repercussions of consecutive summer bleaching events of the threatened Caribbean coral Orbicella faveolata. Coral Reefs 38, 863–876.
Fitt, W. K., McFarland, F. K., Warner, M. E., and Chilcoat, G. C. (2000). Seasonal patterns of tissue biomass and densities of symbiotic dinoflagellates in reef corals and relation to coral bleaching. Limnol. Oceanogr. 45, 677–685.
Frieler, K., Meinshausen, M., Golly, A., Mengel, M., Lebek, K., Donner, S. D., et al. (2013). Limiting global warming to 2 °C is unlikely to save most coral reefs. Nat. Clim. Change 3, 165–170. doi: 10.1038/nclimate1674
Gattuso, J.-P., Allemand, D., and Frankignoulle, M. (1999). Photosynthesis and calcification at cellular, organismal and community levels in coral reefs: a review on interactions and control by carbonate chemistry. Am. Zool. 39, 160–183.
Glynn, P. W. (1996). Coral reef bleaching: facts, hypotheses and implications. Glob. Change Biol. 2, 495–509.
Gnaiger, E., and Bitterlich, G. (1984). Proximate biochemical composition and caloric content calculated from elemental CHN analysis: a stoichiometric concept. Oecologia 62, 289–298. doi: 10.1007/BF00384259
Grottoli, A. G. (2002). Effect of light and brine shrimp on skeletal ?13C in the Hawaiian coral Porites compressa: a tank experiment. Geochim. Cosmochim. Acta 66, 1955–1967. doi: 10.1016/s0016-7037(01)00901-2
Grottoli, A. G., Rodrigues, L. J., and Juarez, C. (2004). Lipids and stable carbon isotopes in two species of Hawaiian corals, Porites compressa and Montipora verrucosa, following a bleaching event. Mar. Biol. 145, 621–631.
Grottoli, A. G., Rodrigues, L. J., Matthews, K. A., Palardy, J. E., and Gibb, O. T. (2005). Pre-treatment effects on coral skeletal delta13C and delta18O. Chem. Geol. 221, 225–242.
Grottoli, A. G., Rodrigues, L. J., and Palardy, J. E. (2006). Heterotrophic plasticity and resilience in bleached corals. Nature 440, 1186–1189. doi: 10.1038/nature04565
Grottoli, A. G., Tchernov, D., and Winters, G. (2017). Physiological and biogeochemical responses of super-corals to thermal stress from the Northern Gulf of Aqaba, Red Sea. Front. Mar. Sci. 4:215. doi: 10.3389/fmars.2017.00215
Grottoli, A. G., Warner, M. E., Levas, S. J., Aschaffenburg, M. D., Schoepf, V., McGinley, M., et al. (2014). The cumulative impact of annual coral bleaching can turn some coral species winners into losers. Glob. Change Biol. 20, 3823–3833. doi: 10.1111/gcb.12658
Grottoli, A. G., and Wellington, G. M. (1999). Effect of light and zooplankton on skeletal 13C values in the eastern Paci?c corals Pavona clavus and Pavona gigantea. Coral Reefs 18, 29–41. doi: 10.1007/s003380050150
Guzmán, H., Burns, K., and Jackson, J. (1994). Injury, regeneration and growth of Caribbean reef corals after a major oil spill in Panama. Mar. Ecol. Prog. Ser. 105, 231–241.
Hagedorn, M., and Carter, V. L. (2015). Seasonal preservation success of the marine Dinoflagellate Coral Symbiont, Symbiodinium sp. PLoS One 10:e0136358. doi: 10.1371/journal.pone.0136358
Hagedorn, M., Carter, V. L., Lager, C., Camperio Ciani, J. F., Dygert, A. N., Schleiger, R. D., et al. (2016). Potential bleaching effects on coral reproduction. Reprod. Fertil. Dev. 28:1061. doi: 10.1071/rd15526
Hinrichs, S., Patten, N. L., Allcock, R. J. N., Saunders, S. M., Strickland, D., and Waite, A. M. (2013). Seasonal variations in energy levels and metabolic processes of two dominant Acropora species (A. spicifera and A. digitifera) at Ningaloo Reef. Coral Reefs 32, 623–635.
Hoegh-Guldberg, O. (1999). Climate change, coral bleaching and the future of the world’s coral reefs. Mar. Freshw. Res. 50, 839–866.
Hoegh-Guldberg, O., and Smith, G. J. (1989). The effect of sudden changes in temperature, light and salinity on the population density and export of Zooxanthellae from the reef corals Stylophora-pistillata Esper and Seriatopora-hystrix Dana. J. Exp. Mar. Biol. Ecol. 129, 279–303. doi: 10.1016/0022-0981(89)90109-3
Hoogenboom, M. O., Campbell, D. A., Beraud, E., DeZeeuw, K., and Ferrier-Pagès, C. (2012). Effects of light, food availability and temperature stress on the function of Photosystem II and Photosystem I of coral symbionts. PLoS One 7:e30167. doi: 10.1371/journal.pone.0030167
Hughes, A., Grottoli, A., Pease, T., and Matsui, Y. (2010). Acquisition and assimilation of carbon in non-bleached and bleached corals. Mar. Ecol. Prog. Ser. 420, 91–101.
Hughes, A. D., and Grottoli, A. G. (2013). Heterotrophic compensation: a possible mechanism for resilience of coral reefs to global warming or a sign of prolonged stress? PLoS One 8:e81172. doi: 10.1371/journal.pone.0081172
Jeffrey, S. W., and Humphrey, G. F. (1975). New spectrophotometric equations for determining chlorophylls a, b, c1 and c2 in higher plants, algae and natural phytoplankton. Biochem. Phys. Pflanzen 167, 191–194. doi: 10.1016/s0015-3796(17)30778-3
Jokiel, P., Maragos, J. E., and Franzisket, L. (1978). “Coral growth: buoyant weight technique,” in Coral Reefs: Research Methods, eds D. R. Stoddart and R. E. Johannes (Paris: UNESCO), 529–541.
Jokiel, P. L. (2011). Ocean Acidification and control of reef coral calcification by boundary layer limitation of proton flux. BMS 87, 639–657. doi: 10.5343/bms.2010.1107
Kim, S.-T., and O’Neil, J. R. (1997). Equilibrium and nonequilibrium oxygen isotope effects in synthetic carbonates. Geochim. Cosmochim. Acta 61, 3461–3475. doi: 10.1038/s41467-019-08336-5
Leder, J. J., Swart, P. K., Szmant, A. M., and Dodge, R. E. (1996). The origin of variations in the isotopic record of scleractinian corals: I. Oxygen. Geochim. Cosmochim. Acta 60, 2857–2870. doi: 10.1016/0016-7037(96)00118-4
Leder, J. J., Szmant, A. M., and Swart, P. K. (1991). The effect of prolonged?bleaching on skeletal banding and stable isotopic composition in Montastrea annularis: preliminary observations. Coral Reefs 10, 19–27.
Levas, S., Grottoli, A. G., Schoepf, V., Aschaffenburg, M., Baumann, J., Bauer, J. E., et al. (2016). Can heterotrophic uptake of dissolved organic carbon and zooplankton mitigate carbon budget deficits in annually bleached corals? Coral Reefs 35, 495–506. doi: 10.1007/s00338-015-1390-z
Levas, S., Schoepf, V., Warner, M. E., Aschaffenburg, M., Baumann, J., and Grottoli, A. G. (2018). Long-term recovery of Caribbean corals from bleaching. J. Exp. Mar. Biol. Ecol. 506, 124–134. doi: 10.1016/j.jembe.2018.06.003
Levas, S. J., Grottoli, A. G., Hughes, A., Osburn, C. L., and Matsui, Y. (2013). Physiological and biogeochemical traits of bleaching and recovery in the mounding species of coral Porites lobata: implications for resilience in mounding corals. PLoS One 8:e63267. doi: 10.1371/journal.pone.0063267
Lough, J. M., and Barnes, D. J. (2000). Environmental controls on growth of the massive coral Porites. J. Exp. Mar. Biol. Ecol. 245, 225–243. doi: 10.1016/s0022-0981(99)00168-9
Loya, Y., Sakai, K., Yamazato, K., Nakano, Y., Sambali, H., and van Woesik, R. (2001). Coral bleaching: the winners and the losers. Ecol. Lett. 4, 122–131. doi: 10.1046/j.1461-0248.2001.00203.x
Marsh, J. A. (1970). Primary productivity of reef-building Calcareous Red Algae. Ecology 51, 255–263.
Marshall, A. T., and Clode, P. (2004). Calcification rate and the effect of temperature in a Zooxanthellate and an Azooxanthellate Scleractinian reef coral. Coral Reefs 23, 218–224.
Marshall, P. A., and Baird, A. H. (2000). Bleaching of corals on the Great Barrier Reef: differential susceptibilities among taxa. Coral Reefs 19, 155–163.
McConnaughey, T. (1989). 13C and 180 isotopic disequilibrium in biological carbonates: I. Patterns. Geochim. Cosmochim. Acta 53, 151–162.
McDermond, J. (2014). Reproduction and Population of Porites divaricata at Rodriguez Key: the Florida Keys, USA. Ph.D. thesis. Fort Lauderdale, FL: Nova Southeastern University.
McLachlan, R., Dobson, K., and Grottoli, A. G. (2020). Quantification of Total Biomass in Ground Coral Samples. Columbus, OH: Protocols.io.
Muscatine, L. (1989). The effect of external nutrient resources on the population dynamics of zooxanthellae in a reef coral. Proc. R. Soc. Lond. B 236, 311–324.
National Data Buoy Center (2021). Station 42056 (LLNR 80) - Yucatan Basin - 120 NM ESE of Cozumel, MX. Bay St. Louis, MS: National Data Buoy Center.
NOAA Coral Reef Watch (2019). NOAA Coral Reef Watch Version 3.1 Daily 5km Satellite Regional Virtual Station Time Series Data for Southeast Florida, Mar. 12, 2013-Mar. 11, 2014. College Park, MD: NOAA Coral Reef Watch.
Oku, H., Yamashiro, H., Onaga, K., Sakai, K., and Iwasaki, H. (2003). Seasonal changes in the content and composition of lipids in the coral Goniastrea aspera. Coral Reefs 22, 83–85.
Porter, J. W. (1976). Autotrophy, heterotrophy, and resource partitioning in Caribbean Reef-Building Corals. Am. Nat. 110, 731–742. doi: 10.1086/283100
Price, J. T., McLachlan, R. H., Jury, C. P., Toonen, R. J., and Grottoli, A. G. (2021). Isotopic approaches to estimating the contribution of heterotrophic sources to Hawaiian corals. Limnol. Oceanogr. 66, 2393–2407. doi: 10.1002/lno.11760
Reynaud, S., Leclercq, N., Romaine-Lioud, S., Ferrier-Pagés, C., Jaubert, J., and Gattuso, J.-P. (2003). Interacting effects of CO 2 partial pressure and temperature on photosynthesis and calcification in a scleractinian coral: effects of pCo2 and temperature on coral. Glob. Change Biol. 9, 1660–1668.
Rodrigues, L. J., and Grottoli, A. G. (2006). Calcification rate and the stable carbon, oxygen, and nitrogen isotopes in the skeleton, host tissue, and Zooxanthellae of bleached and recovering Hawaiian corals. Geochim. Cosmochim. Acta 70, 2781–2789.
Rodrigues, L. J., and Grottoli, A. G. (2007). Energy reserves and metabolism as indicators of coral recovery from bleaching. Limnol. Oceanogr. 52, 1874–1882. doi: 10.4319/lo.2007.52.5.1874
Ross, C., Falter, J., Schoepf, V., and McCulloch, M. (2015). Perennial growth of hermatypic corals at Rottnest Island, Western Australia (32°S). PeerJ 3:e781. doi: 10.7717/peerj.781
Ross, C., Schoepf, V., Decarlo, T., and McCulloch, M. (2018). Mechanisms and seasonal drivers of calcification in the temperate coral Turbinaria reniformis at its latitudinal limits. Proc. R. Soc. B Biol. Sci. 285:20180215. doi: 10.1098/rspb.2018.0215
Schoepf, V., Grottoli, A. G., Levas, S. J., Aschaffenburg, M. D., Baumann, J. H., Matsui, Y., et al. (2015). Annual coral bleaching and the long-term recovery capacity of coral. Proc. R. Soc. B 282:20151887. doi: 10.1098/rspb.2015.1887
Smith, P. K., Krohn, R. I., Hermanson, G. T., Mallia, A. K., Gartner, F. H., Provenzano, M. D., et al. (1985). Measurement of protein using bicinchoninic acid. Anal. Biochem. 150, 76–85. doi: 10.1016/0003-2697(85)90442-7
Spalding, M., Burke, L., Wood, S. A., Ashpole, J., Hutchison, J., and Zu, E. P. (2017). Mapping the global value and distribution of coral reef tourism. Mar. Policy 82, 104–113.
Stimson, J., Sakai, K., and Sembali, H. (2002). Interspecific comparison of the symbiotic relationship in corals with high and low rates of bleaching-induced mortality. Coral Reefs 21, 409–421. doi: 10.1007/s00338-002-0264-3
Stimson, J. S. (1987). Location, quantity and rate of change in quantity of lipids in Tissue of Hawaiian Hermatypic Corals. Bull. Mar. Sci. 41, 889–904.
Suggett, D. J., and Smith, D. J. (2020). Coral bleaching patterns are the outcome of complex biological and environmental networking. Glob. Change Biol. 26, 68–79. doi: 10.1111/gcb.14871
Szmant, A. M. (1986). Reproductive ecology of Caribbean reef corals. Coral Reefs 5, 43–54. doi: 10.1007/bf00302170
Teece, M. A., Estes, B., Gelsleichter, E., and Lirman, D. (2011). Heterotrophic and autotrophic assimilation of fatty acids by two scleractinian corals, Montastraea faveolata and Porites astreoides. Limnol. Oceanogr. 56, 1285–1296. doi: 10.4319/lo.2011.56.4.1285
Teneva, L., Karnauskas, M., Logan, C. A., Bianucci, L., Currie, J. C., and Kleypas, J. A. (2012). Predicting coral bleaching hotspots: the role of regional variability in thermal stress and potential adaptation rates. Coral Reefs 31, 1–12. doi: 10.1007/s00338-011-0812-9
Thornhill, D. J., Rotjan, R. D., Todd, B. D., Chilcoat, G. C., Iglesias-Prieto, R., Kemp, D. W., et al. (2011). A connection between colony biomass and death in Caribbean Reef-Building Corals. PLoS One 6:e29535. doi: 10.1371/journal.pone.0029535
Towle, E. K., Carlton, R., Langdon, C., and Manzello, D. P. (2015). In-situ measurement of metabolic status in three coral species from the Florida Reef Tract. Reg. Stud. Mar. Sci. 2, 145–153. doi: 10.1016/j.rsma.2015.09.007
Warner, M. E., LaJeunesse, T. C., Robison, J. D., and Thur, R. M. (2006). The ecological distribution and comparative photobiology of symbiotic dinoflagellates from reef corals in Belize: potential implications for coral bleaching. Limnol. Oceanogr. 51, 1887–1897. doi: 10.4319/lo.2006.51.4.1887
Keywords: Porites divaricata, Porites astreoides, Orbicella faveolata, coral physiology, natural variability, coral bleaching, energy reserves, heterotrophy
Citation: Chapron L, Schoepf V, Levas SJ, Aschaffenburg MD, Warner ME and Grottoli AG (2022) Natural Variability in Caribbean Coral Physiology and Implications for Coral Bleaching Resilience. Front. Mar. Sci. 8:811055. doi: 10.3389/fmars.2021.811055
Received: 08 November 2021; Accepted: 24 December 2021;
Published: 13 January 2022.
Edited by:
José D. Carriquiry, Universidad Autónoma de Baja California, MexicoReviewed by:
Amilcar Leví Cupul-Magaña, University of Guadalajara, MexicoWilliam Fitt, University of Georgia, United States
Copyright © 2022 Chapron, Schoepf, Levas, Aschaffenburg, Warner and Grottoli. This is an open-access article distributed under the terms of the Creative Commons Attribution License (CC BY). The use, distribution or reproduction in other forums is permitted, provided the original author(s) and the copyright owner(s) are credited and that the original publication in this journal is cited, in accordance with accepted academic practice. No use, distribution or reproduction is permitted which does not comply with these terms.
*Correspondence: Leila Chapron, Y2hhcHJvbkBvYnMtYmFueXVscy5mcg==; Andréa G. Grottoli, Z3JvdHRvbGkuMUBvc3UuZWR1