- 1Laboratory of Algal and Plant Physiology, Dipartimento di Scienze della Vita e dell’Ambiente, Università Politecnica delle Marche, Ancona, Italy
- 2BCCM/DCG Diatoms Collection, Department of Biology, Ghent University, Ghent, Belgium
- 3Faculty of Sciences, STU-UNIVPM Joint Algal Research Center, Shantou University, Shantou, China
Predation is one of the strongest selection pressures phytoplankton has evolved strategies to cope with. Concurrently, phytoplankton growth must deal with resource acquisition. Experiments on mono- and mixed cultures of morphologically different diatoms exposed to copepods were performed to assess if size and shape were primary drivers in avoiding predation. Additionally, frustule silicification was investigated as a potential factor affecting prey selection by copepods. Thalassiosira pseudonana, Conticribra weissflogii, Cylindrotheca closterium, and Phaeodactylum tricornutum were exposed to the presence of Temora longicornis, a calanoid copepod. The physiological response in terms of growth, elemental composition and morphology was determined. The power of Image Flow Cytometry allowed functional single-cell analyses of mixed cultures in the presence and absence of copepods. Results highlighted that T. pseudonana although the most eaten by copepods in monospecific cultures, was not the preferred prey when the bigger C. weissflogii was added to the culture. When pennates were co-cultured with centric diatoms, their growth was unaffected by predators. Our data suggested that the frustule morphology contributes to long-term prey-predator interaction since the elongated thinner frustule, which evolved more recently, benefited cells in escaping from predators also when facing competition for resources.
Introduction
Diatoms are important oceans’ primary producers, which strongly affect global food webs. From a biological perspective, the world of diatoms has been moulded by the competitive interactions with other phytoplankton groups, resulting in the spatial patterns of marine primary production observed today (Armbrust, 2009; Vallina et al., 2014; Vincent and Bowler, 2020), and by the co-evolution with copepods and other micro-predators that have influenced radiation trajectories from higher levels of food webs (Turner, 2004; Hamm and Smetacek, 2007; Giordano et al., 2018; Lürling, 2020).
Evolution led to an explosion of morphological varieties of diatom cells, from a radial to an elongated geometry, with a significant change in the frustule silicification (Finkel and Kotrc, 2010; Kotrc and Knoll, 2015). This change in silicification is consistent with a decreasing availability of silicic acid in oceans through geologic eras (Maliva et al., 1989; Lazarus et al., 2009, 2014; Finkel et al., 2010; Finkel and Kotrc, 2010; Conley et al., 2017), so the more recently evolved pennate diatoms developed thinner, thus less silicified frustules.
The morphological shift is still in progress and probably linked to a continuous balance between an effective response to different selective pressures including predation and the cost of shell thickening (Martin-Jezequel et al., 2000; Hildebrand et al., 2018; Grønning and Kiørboe, 2020). Phytoplankton has evolved multiple strategies to avoid predation (Pančić and Kiørboe, 2018; Lürling, 2020), those may involve not only morphological defences (e.g., colony formation, thick silica shell) but also behavioural defences (e.g., motility) or physiological defences (e.g., toxicity). In particular, pennate diatoms with a thinner frustule have adopted new chemical ways to avoid predation as compared to centric diatoms, i.e., they may produce apo-fucoxanthinoids or phycotoxins (i.e., domoic acid) acting as feeding deterrents and/or harmful compounds to predators (Shaw et al., 1995a,b, 1997; Tammilehto et al., 2015. Beside the macroevolutionary morphological changes, increasing silicification of diatoms has also been observed as a rapid response to a sudden exposure to predators (Pondaven et al., 2007; Pančić et al., 2019).
In fact, as already reported (Hamm et al., 2003; Friedrichs et al., 2013; Liu et al., 2016; Zhang et al., 2017; Xu et al., 2021), silicon deposition in the diatom cell wall is adopted as selective criterion by predators to choose suitable preys since higher silicification provides a stronger armour against predation, decreasing diatoms palatability.
However, cited studies only report exposure to predators of a single algal species at the time. So, for a deeper comprehension of the frustule role in shaping evolution, our purpose was to verify if lower silicified pennate diatoms were selected by copepods when strongly shielded centric diatoms where in the same culture.
Furthermore, the role of diatom morphology in predator-prey relation is not independent from the role played in the competition for resources: both cell size and shape are crucial in phytoplankton light harvesting, nutrient uptake, and buoyancy (Pahlow et al., 1997; Key et al., 2010; Naselli-Flores and Barone, 2011; Naselli-Flores et al., 2021). Culturing differently sized and shaped diatoms allowed us to introduce interspecies competition for growth-related resources next to the predation pressure, mimicking more natural dynamics.
Therefore, two centric diatoms Thalassiosira pseudonana and Conticribra weissflogii, and two pennate diatoms Cylindrotheca closterium and Phaeodactylum tricornutum were exposed to Temora longicornis for up to 7 days. Analysis of cellular morphology, Si content and elemental composition were carried out at different timepoints during the exposure to grazers and compared to the corresponding values obtained in cultures without grazing pressure.
Finally, it was possible to accurately study predator-prey interactions as well as interspecies competition on cultures of diatom species mixed together due to the single cell approach of Imaging Flow Cytometry, which allowed to analyse in detail cell populations of different diatom species in a mix.
Materials and Methods
Cultures
Two centric diatom species, Thalassiosira pseudonana (CCAP 1085/12)1 and Conticribra weissflogii (DCG 0320), and two raphid pennate diatoms characterised by their pronounced elongated shape and thin frustules, Cylindrotheca closterium (DCG 0685) and Phaeodactylum tricornutum (DCG 0981) (Supplementary Figure 1), were cultured using f/2 medium (Guillard, 1975) in a controlled-environment room at 18°C, illuminated with cool white fluorescent lamps at 60 μmol photons m–2 s–1 and 12:12 h light-dark cycles. These species were selected because of their contrasting shapes and difference in cell size, ranging from 2 to 45 μm. The DCG strains were acquired from the BCCM/DCG Diatoms Collection.2
Predators were caught offshore in the Belgian North Sea (51°18.088 Lat., 2°39.667 Long.) while on board of the Flanders Marine Institute vessel RV Simon Stevin. Sampling was performed using a WP2 vertical planktonic net (total length: 2.6 m, mesh width: 200 μm). Zooplankton was collected through light attraction and the copepod species Temora longicornis was isolated and identified using a stereomicroscope and subsequently caught with a pipette and put in a separate tank. This species was chosen as diatoms feeder due to its known capacity to break differently sized frustules (Miller et al., 1990; Jansen, 2008; Pančić et al., 2019). The copepods were maintained in natural seawater in climate chambers with aerators, illuminated with cool white fluorescent lamps at 60 μmol photons m–2 s–1 and 12:12 h light-dark cycles.
Monospecific diatom cultures were established in 175 mL plastic flasks (VWR Tissue Culture Flask, treated, vented cap, sterile. Manufactured for VWR International, LLC Radnor Corporate Center, 100 Matsonford Rd. Radnor, PA, United States) filled with 50 mL of f/2 medium. The density of the inoculated diatom cells was determined to obtain a ratio of 1 copepod to 0.2–0.7 mg algal C (Ratti et al., 2013): 8 × 105 cells/ml of T. pseudonana and P. tricornutum were exposed to 2 copepods, 4 × 105 cells/ml of C. closterium to 1 copepod and 8 × 104 cells/ml of C. weissflogii to 2 copepods. Copepods were washed and kept for 3 h in f/2 medium in a controlled-environment room at 18°C, illuminated with cool white fluorescent lamps at 60 μmol photons m–2 s–1 and 12:12 h light-dark cycles before being transferred to the diatom cultures. Cultures in three replicas were checked daily and each dead copepod was replaced with a new living individual; diatom cultures without copepods were maintained as controls under the same growth conditions. Diatoms were sampled and analysed before (day 0 in tables, T0 in text and graphs) and after 1, 3, and 7 days (day 1, 3, 7 in tables, T1, T3, T7 in text and graphs) of copepods exposure. Copepods and fecal pellets were removed with a 0.45 mm filter prior to each analysis.
Next to the monospecific tests, three mixed diatom cultures were established (Table 1), with and without copepods to evaluate grazing pressure during interspecies diatom competition, each of them in three replicas. The initial inoculum was calculated considering the C ratio of algae to copepods as described before but applying a dilution factor of 10 (1 Copepod – 0.02–0.07 algal C) to avoid dense algal cultures and hence to limit possible self-shading, transient O2 inhibition and nutrient shortage. The experimental sampling was conducted as described above for monospecific culture tests.
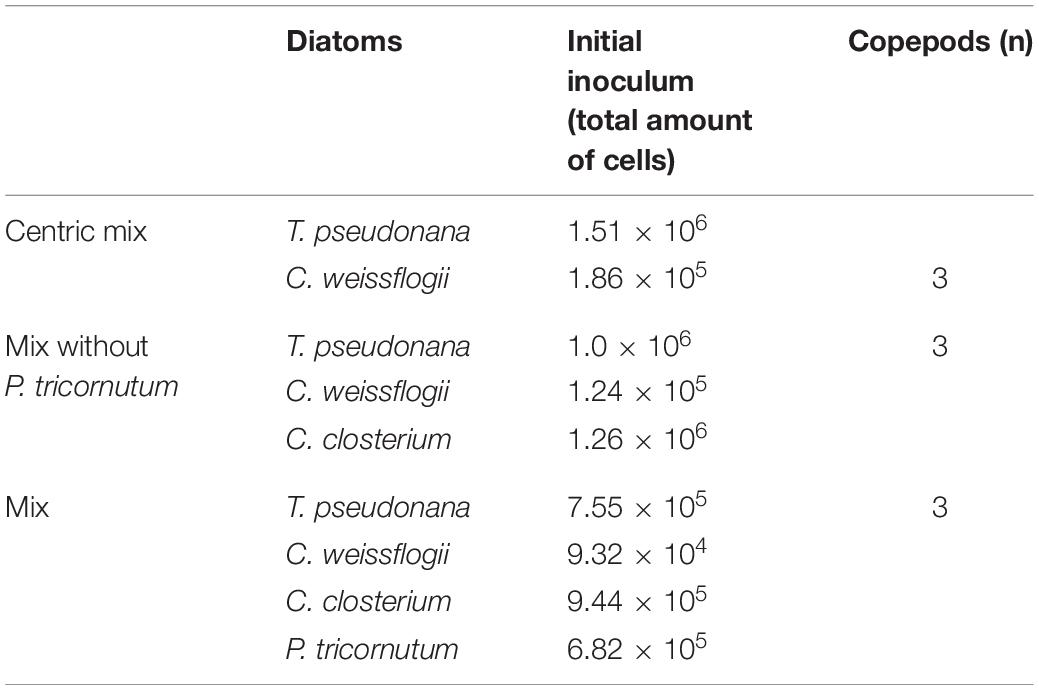
Table 1. Composition of the 3 mixed diatom cultures: algal species, number of algal cells and copepods added to 50 ml of cultures.
Morphological Features by Imaging Flow Cytometer Analysis
Diatoms were analysed using the Imaging Flow Cytometer (IFC) ImageStreamX® Mark II, (Amnis Corp., Seattle, WA, United States) using the INSPIRE software package (Amnis Corp.) to determine cell density and morphometric characteristics. For each analysis, a volume of 2.5 mL diatom culture was sampled and centrifuged (Centrifuge Sigma 4K15) for 5 min at 3,500 rpm. The supernatant fluid was removed, the cell pellet resuspended in 30 μl of f/2 medium and subsequently stored at 4°C. All IFC samples were analysed within 24 h after sampling. IFC data of all objects present in the 30 μl were saved. Details and settings of the IFC technique were as follows: 10 μm core size diameter, 66 mm/s speed, 40× magnification; bright field data were collected in channel 1 (LED intensity 34.63 mW) and chloroplast autofluorescence in channel 5 (642 nm, laser 0.5 mW). Post – acquisition data analysis was performed using the IDEAS software package. Specific masks, separating the pixels occupied by the diatoms from the background, for both brightfield and autofluorescence images were created for each diatom species. All objects lacking chloroplast autofluorescence, as determined by the Intensity feature of the IDEAS software, were considered debris or dead diatoms and, consequently, excluded from cell counting and all other metrics. Among the living cells, only single and well-focused diatoms were gated to obtain morphological parameters.
The morphological features considered for the analysis were Height, Width, Length, Elongatedness (height/width), Thickness max, Area, Circularity (IDEAS User Manual, version 6.0, March 2013). Given numbers refer to at least 1,000 cells for each biological replica.
Height and Width were used to calculate the biovolume of cells using the equation [V = π/4⋅d2⋅h] for C. weissflogii and T. pseudonana (cylinder, Hillebrand et al., 1999) and [V = π/6⋅d2⋅h] for C. closterium and P. tricornutum (prolate spheroid, Hillebrand et al., 1999).
Algal Growth
Cell density was determined as described before by IFC analysis or by Burker hemocytometer counts (depth 0.1 mm, MARIENFELD Superior, Germany) for the monospecific control cultures. Diatom growth rates, μ (1), were derived from cell samples taken at the abovementioned 4 time points (day 0, 1, 3, 7). To estimate grazer feeding rate for the monospecific culture tests, the rate of growth of the different diatom species cultured with and without copepods was compared. In mixed cultures plus copepods, the growth rate of each species was compared to the ones of other diatoms in the mix and to the one of the same species in the mix without copepods.
(1) (Monod, 1949)
Elemental Composition
Cellular C and N quotas were determined by elemental analyser (ECS 4010, Costech Italy) on 0.1 – 1 mg DW of cells which were previously washed twice in ammonium formate solution isosmotic to the culturing media (0.5 M) and dried at 80°C (Ratti et al., 2011). Elemental quantification used sulfanilamide (C:N:S = 6:2:1) for the standard curve. Data acquisition and analysis were performed using the software EAS- Clarity (Costech Analytical Technologies Inc., Milano, ItalyOrganic composition). All measurements were performed on 3 biological replicas of samples collected on day 0 and day 7 of monospecific cultures.
The amount of P, S, K, Ca, Fe, Si per cell was measured by Total Reflectance X – ray Fluorescence spectrometer (S2 Picofox, Bruker AXS Microanalysis GmbH, Berlin, Germany). Sampled diatoms were washed twice with an ammonium formate solution isosmotic to the culture media and resuspended in 250 μl of dH2O. A solution of 0.1 g L–1 Ga (Sigma Aldrich, St. Luis, MO, United States) in 5% HNO3 was added as an internal standard to a final concentration of 0.5 μL L–1. The suspension was carefully vortexed and an aliquot of 10 μl was deposed on a plastic sample holder, dried on a heating plate and measured for 1,000 s (Ratti et al., 2013). Spectral deconvolution and quantification of elemental abundances were performed by the SPECTRA 6.1 software (Bruker AXS Microanalysis GmbH, Berlin, Germany). All measurements were performed in both monospecific and mixed cultures on day 0, 1, 3, and 7.
Statistical Analysis
Significant differences among the means of Si content per volume (dependent variable) over time (independent variable) were tested with a one-way analysis of variance (ANOVA), followed by Tukey’s post-hoc test.
Comparison of growth dependent variables (growth rate and maximum cell density) between cells exposed or not to copepods (independent variable) was carried out with a two-tailed t-test.
Significant differences among the means of growth dependent variables (cell density and growth rate) in different species (independent variable) growing in the presence or in the absence of copepods (independent variable) were tested with a two-way analysis of variance (ANOVA), followed by Tukey’s post-hoc test. The level of significance was set at 0.05. GraphPad prism 8.0.2.263 was used to perform the aforementioned tests (GraphPad Software, San Diego, CA, United States).
Principal Component Analysis (PCA) was done using PAST 4.03 (Hammer et al., 2001. PAST: Paleontological statistics software package for education and data analysis). PCA was performed on different morphological features (e.g., Height, Width, Length, Elongatedness (height/width), Thickness max, Area, Circularity) obtained through Imaging Flow Cytometer analysis as dependent variables along the time course of the experiment (independent variable) and in different species (independent variable) exposed or not to copepods (independent variable). Data were normalised using z-values [(n-mean)/SD].
Results
Monospecific Cultures
The presence of copepods strongly affected the growth rate of both centric diatoms (Figures 1A,D). In particular, T. pseudonana showed a negative growth rate as well as a maximum cell density that was half when grazers were present. In C. weissflogii growth rate was significantly lower when cells were exposed to copepods (p = 0.04, Supplementary Table 11), but no difference in maximum cell density was observed. A statistically significant increase in Si content per cell volume was observed on day 1 compared to days 0, 3, and 7 in C. weissflogii [F(3, 7) = 40.59, p < 0.0001, Figure 1B and Supplementary Table 11]. Moreover, on day 1, C. weissflogii was the only species showing a decrease in cell density. In T. pseudonana, the highest Si concentration per volume on day 7 occurred when the cell number was lowest [F(3, 7) = 8.806, p = 0.0090, Supplementary Table 11]. It is worth mentioning that the peak in Si concentration was always present together with a higher concentration of C, N, P, S, K, Ca, Fe elemental quotas per volume (Supplementary Table 1).
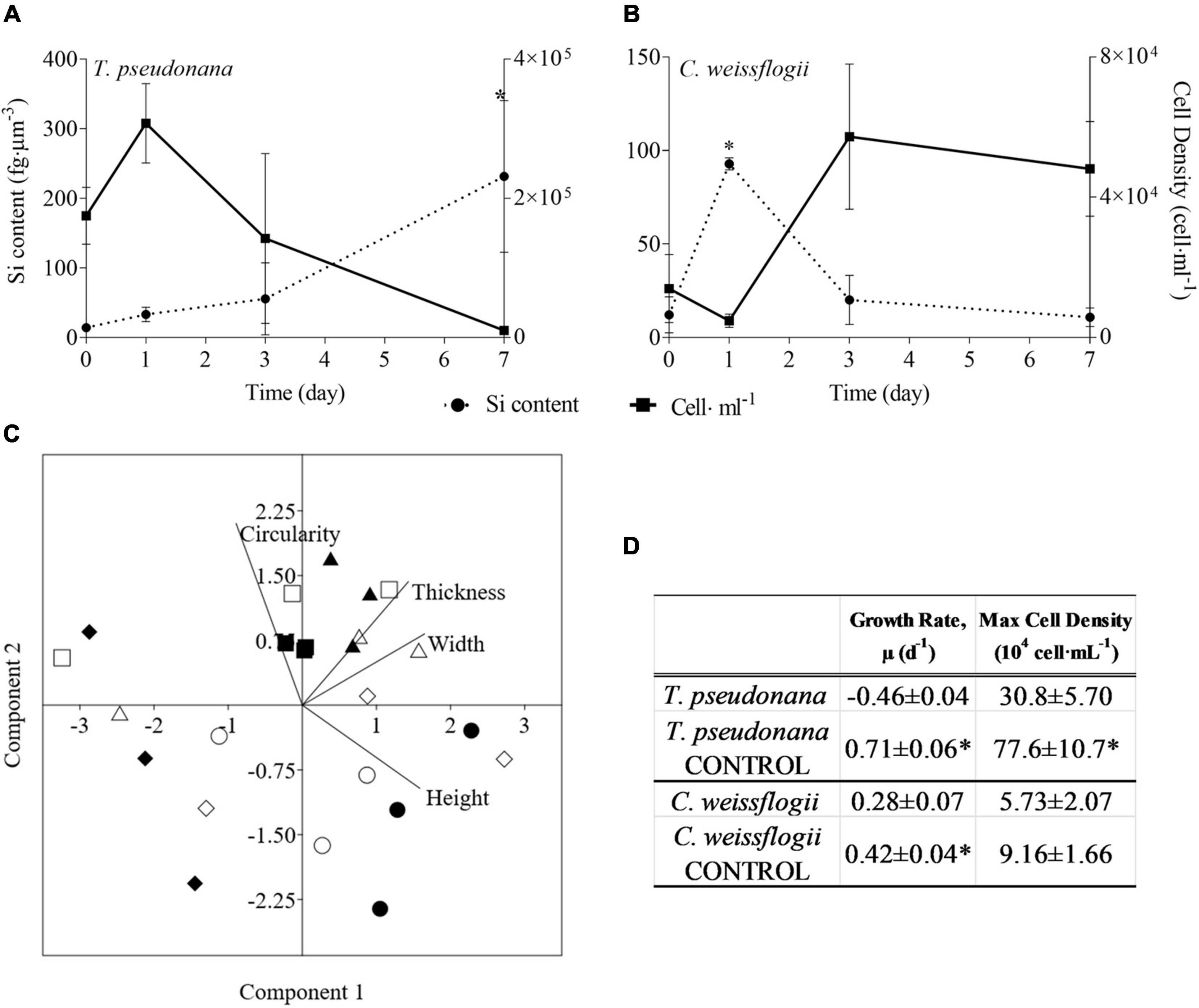
Figure 1. (A,B) Si content (fg μm–3; dashed line) and cell density (cell ml–1; solid line) in the two centric diatom species under copepods exposure. Data are means of 3 biological replicas. Error bars show SD while asterisks indicate significant differences in Si content of each species among days according to one-way ANOVA followed by Tukey’s post-hoc test, p < 0.05 (details in Supplementary Table 11). (C) PCA analysis on morphological characteristics of centric diatoms (T. pseudonana and C. weissflogii) in monospecifc cultures. Different symbols indicate different time points during the experiment: T0 ○●; T1 □■, T3△▲, T7 ◇◆, while different colours indicate different species: black for T. pseudonana white for C. weissflogii. (D) Comparison of growth rates (d–1) and maximum cell densities (cells⋅mL–1) for each of the two diatom species between cultures with and without (CONTROL) copepods. The values are means of 3 biological replicas ± SD. For each species, asterisks indicate when growth rate or max cell density were statistically different between diatoms exposed or not (CONTROL) to copepods, unpaired t test, p < 0.05 (Supplementary Table 11).
Morphological observations of centric diatoms (Circularity, Thickness, Width, Height, Figure 1C) were presented through PCA. PC1 and PC2 explained 97.94% of the total variation present in the data matrix with PC1 accounting for 65.06% and PC2 for 32.88%. Loading values (eigenvalues in Supplementary Table 3, means values Supplementary Table 4) differentiated days 0 and 7 of copepod exposure from days 1 and 3 according to PC2, indicating an increase in circularity when the stress was present. Furthermore, there is a separation between T. pseudonana T0 and T7 according to PC1, recording the reduction in size after grazing pressure (Supplementary Tables 2, 4).
Regarding the pennate species, neither growth rate nor maximum number of cells were significantly affected by the presence of copepods (Figures 2A,D). Except for the higher Si content per cell volume of C. closterium at day 1 as compared to the other time points [F(3, 8) 11.73, p = 0.0027, Supplementary Table 11], also observed in the response of C. weissflogii, in both the pennate diatoms there was not a statistically significant change in Si content (Figures 2A,B). The morphological characterisation of pennate diatoms through PCA (Elongatedness, Length, Thickness, Width, Figure 2C) explained 94.68% of the total variance (PC1 + PC2), where the weight of PC1 was 60.36% while PC2 34.32%. It showed a net division between T0 and T7 according to PC2, but the two species on day 7 were separated according to PC1. Indeed, both underwent a reduction in width after 7 days of copepods pressure, but P. tricornutum increased the elongatdness while C. closterium reduced its length.
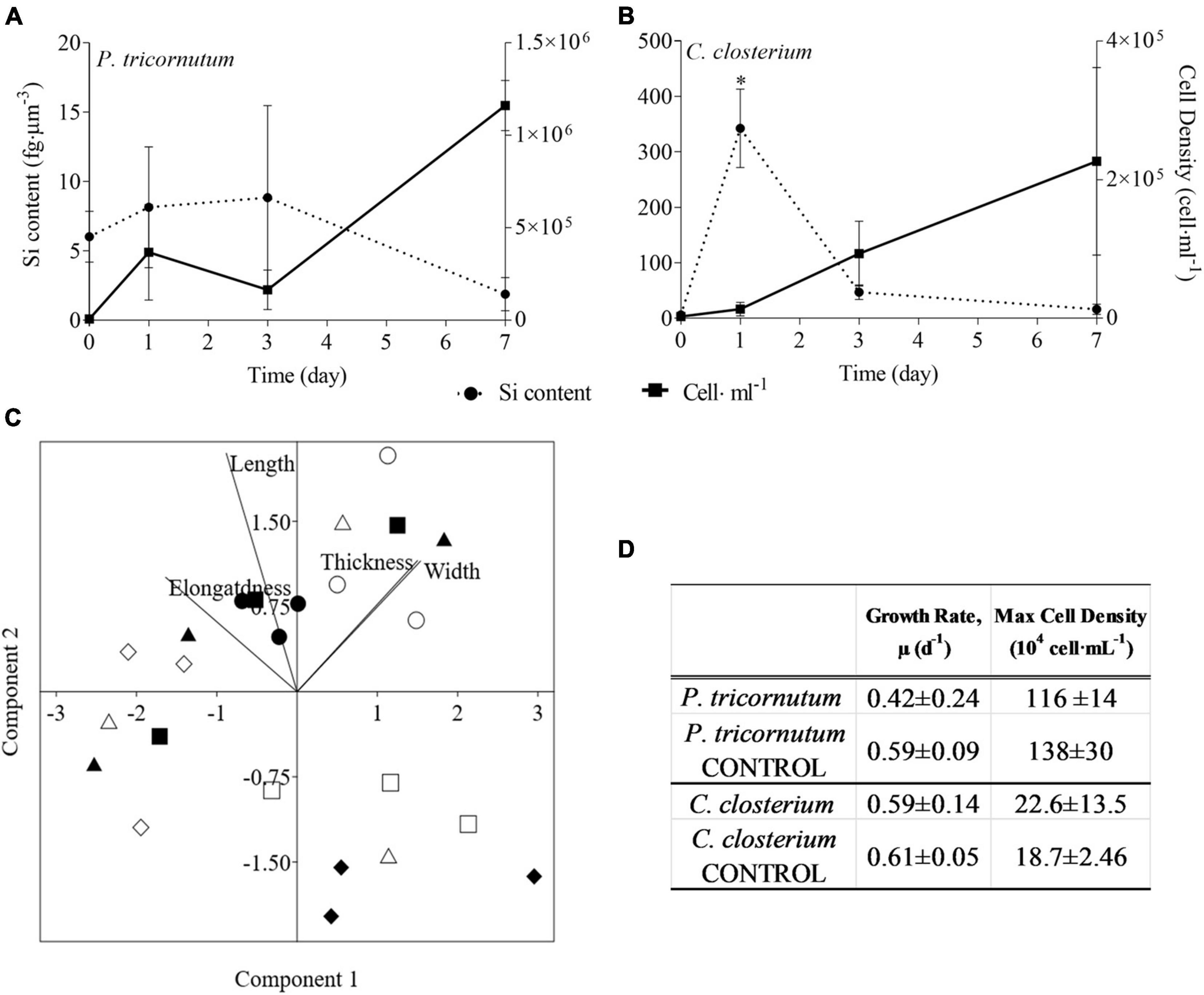
Figure 2. (A,B) Si content (fg μm–3; dashed line) and cell density (cell ml–1; solid line) in the two pennate diatom species under copepods exposure. Data are means of 3 biological replicas. Error bars show SD while asterisks indicate significant differences in Si content of each species among days according to one-way ANOVA followed by Tukey’s post-hoc test, p < 0.05 (details in Supplementary Table 11). (C) PCA analysis on morphological characteristics of pennate diatoms (C. closterium and P.tricornutum) in monospecifc cultures. Different symbols indicate different time points during the experiment: T0 ○●; T1 □■, T3△▲, T7 ◇◆, while different colours indicate different species: black for P. tricornutum, white for C. closterium. (D) Comparison of growth rates (d–1) and maximum cell densities (cells⋅mL–1) for each of the two diatom species between cultures with and without (CONTROL) copepods. The values are means of 3 biological replicas ± SD. For each species, asterisks indicate when growth rate or max cell density were statistically different between diatoms exposed or not (CONTROL) to copepods as determined by unpaired t test, p < 0.05 (Supplementary Table 11).
Mixed Cultures
For the centric mix, according to the growth curves (Figures 3A,B) and the growth rate values (Supplementary Figure 3A), T. pseudonana was growing significantly faster than C. weissflogii in both the presence (F = 27.04, p < 0.0001, Supplementary Table 11) and the absence of copepods (F = 89.36, p < 0.0001, Supplementary Table 11). Whereas C. weissflogii was negatively affected by grazers only on day 7 (Figure 3A).
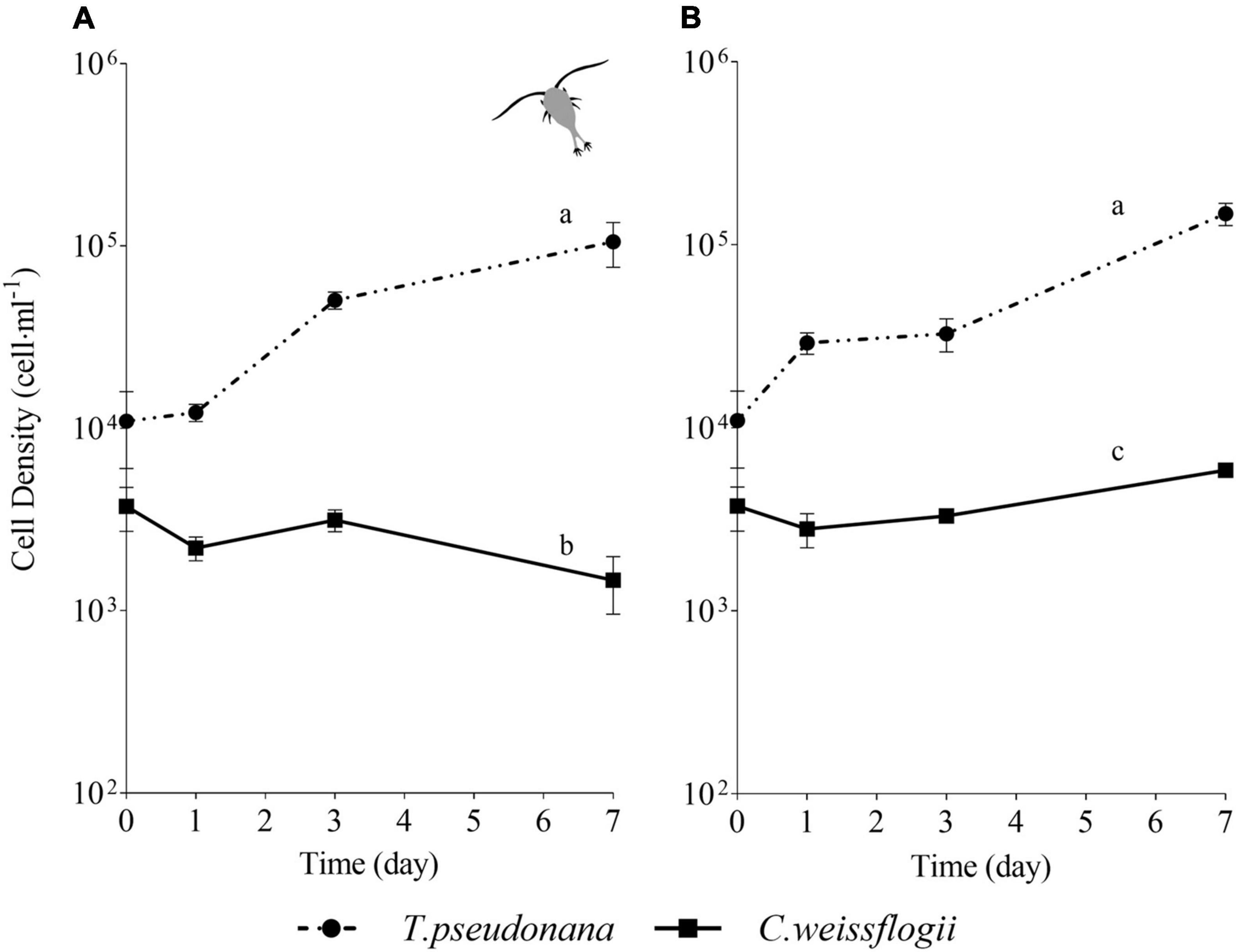
Figure 3. Comparison between growth curves of the centric diatom mix exposed (A) or not (B) to copepods. The data are means of 3 biological replicas. Error bars show SD while letters indicate significant differences according to two-way ANOVA followed by Tukey’s post-hoc test, p < 0.05 (Supplementary Table 11).
Morphological features for centric mixed diatoms (Circularity, Area, Width, Height) were represented with PCA in Figure 4. PC1 + PC2 accounted for 99.9% (91.3 + 8.6%) and 98.3% (60.3 + 38.0%) of the total variation present in T. pseudonana and C. weissflogii, respectively (Figures 4A,B, eigenvalues in Supplementary Table 4, means values Supplementary Table 6). In both centic diatoms, PCA differentiated day 0 from day 7 according to PC1, recording a change in cells’ circularity, in T. pseudonana also for PC2 adding a decrease in size during the experiment. Interestingly, C. weissflogii showed separation according to PC2 in the different condition analysed (exposure or not to copepods) at day 7, highlighting an increase in Area only when copepods were absent.
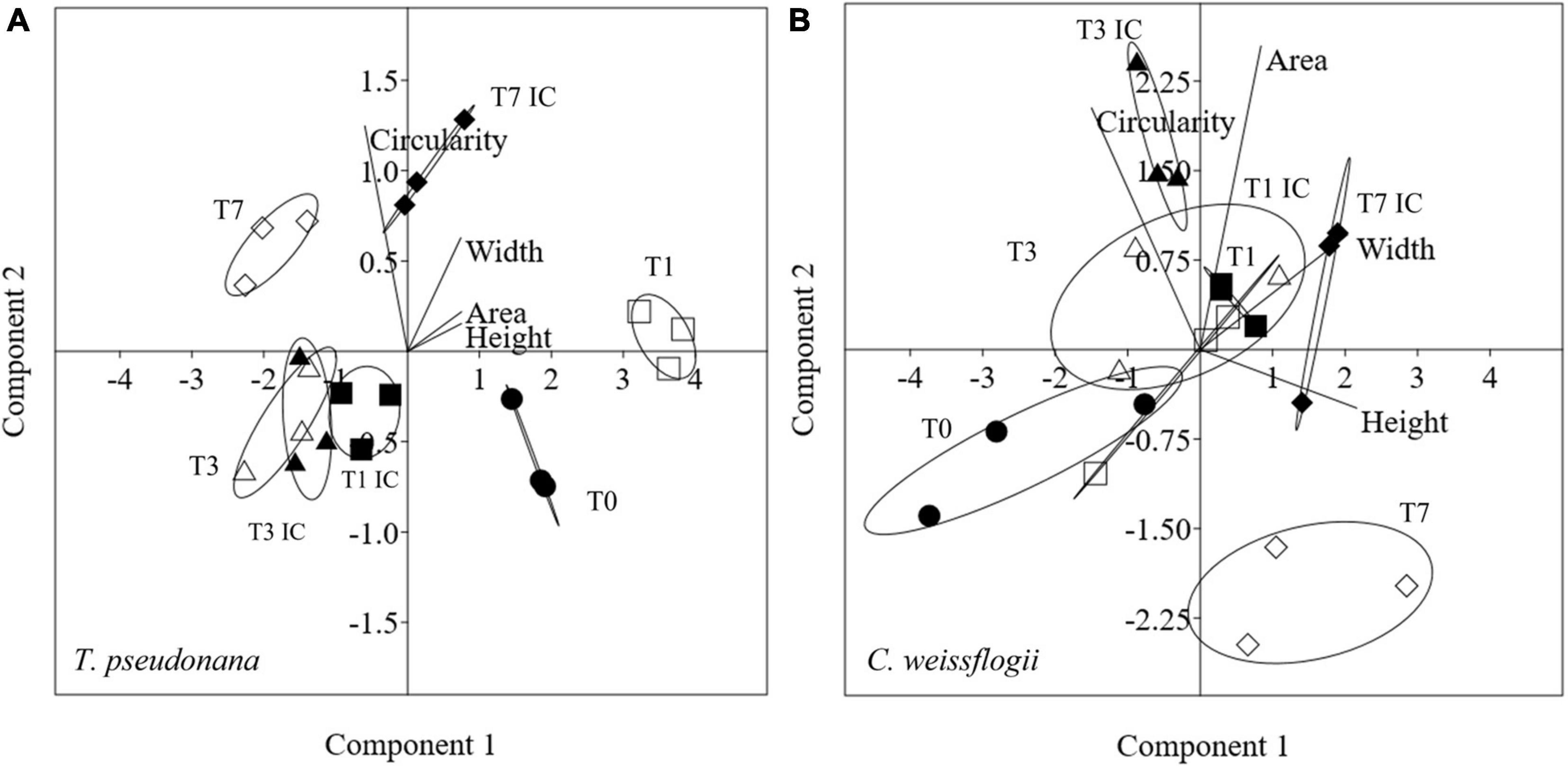
Figure 4. PCA analysis on morphological characteristics in T. pseudonana (A) and C. weissflogii (B) when mixed together. Different symbols indicate different time during the experiment: T0 ○●; T1 □■, T3△▲, T7 ◇◆; white symbols indicate copepods exposure while black symbols indicate absence of copepods and interspecies competition (IC) only.
In the mix with the two centric diatoms and the pennate C. closterium, growth of T. pseudonana had a positive trend under grazing pressure (Figure 5A) while in the absence of copepods cell density declined after reaching the maximum value on day 1, without further recovery (Figure 5B). Cell density of C. weissflogii showed a peak on day 1 and then decreased until day 7, independently from copepods pressure (Figures 5A,B). After initial cell decline (days 1 and 3), C. closterium grew on day 7, reaching a similar cell density of T. pseudonana (Figure 5A) when exposed to copepods. In the absence of grazers, C. closterium had the highest cell density among the species after 7 days (Figure 5B). Overall, data resumed in Figure 5 showed that in the presence of copepods T. pseudonana and C. closterium managed to grow even if not from the first day; in both cases, species grew better than in the absence of copepods (see also Supplementary Figure 3B). Conversely, C. weissflogii growth had the same negative behaviour in both the analysed conditions.
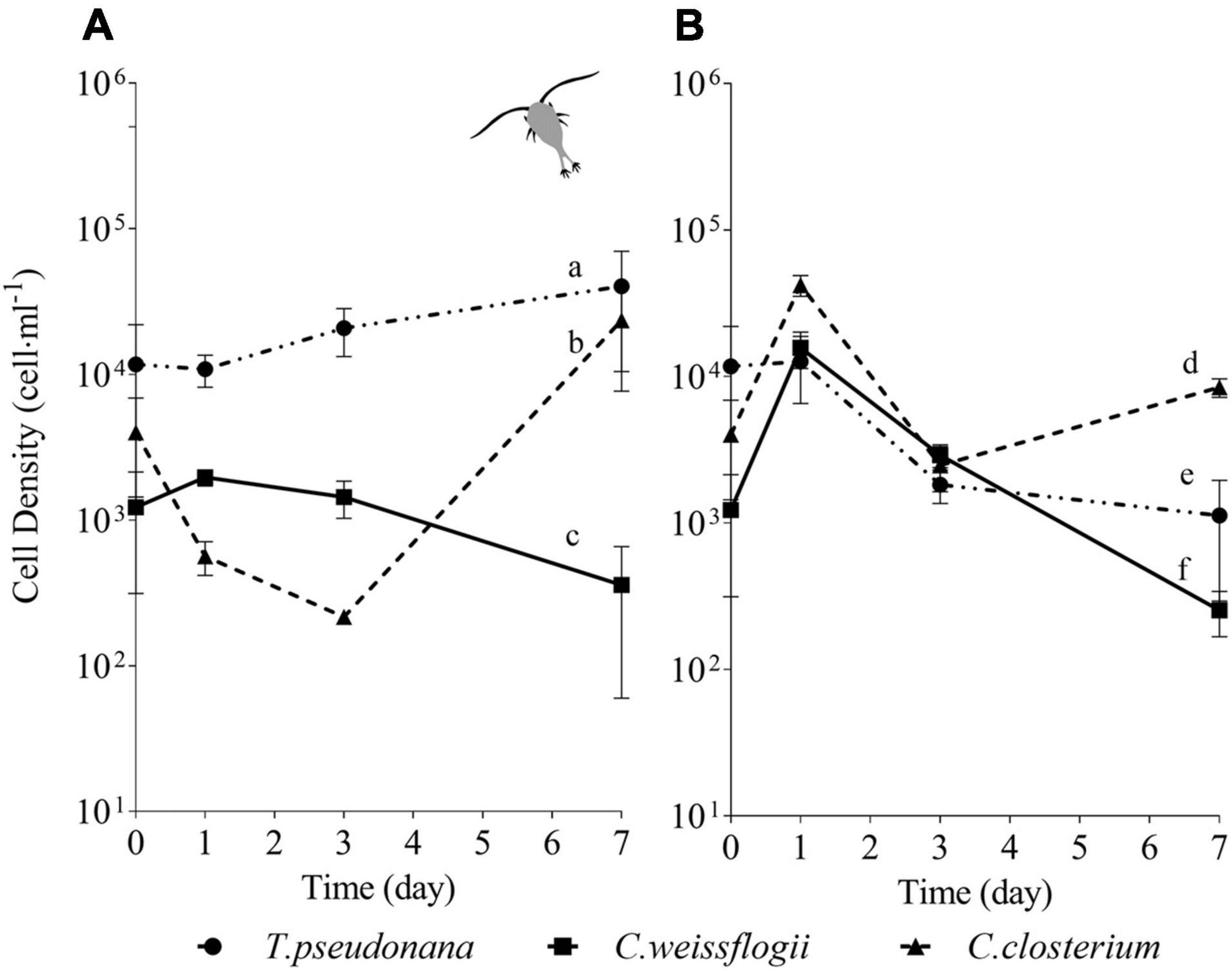
Figure 5. Comparison between growth curves of the diatom mix without P. tricornutum exposed (A) or not (B) to copepods. The data are means of 3 biological replicas. Error bars show SD while letters indicate significant differences according to two-way ANOVA followed by Tukey’s post-hoc test, p < 0.05 (Supplementary Table 11).
Morphological features for centric diatoms (Circularity, Area, Width, Height) and pennate diatoms (Elongatedness, Length, Height, Width) were represented with PCA in Figure 6. Considering the mixed culture of the two centric diatoms and C. closterium, in Figures 6A,B, PC1 + PC2 accounted for respectively 98.8% (86.2 + 12.6%) and 78.7% (48.6 +31.1%), of the total variation in T. pseudonana and C. weissflogii. Day 0 and 7 were again separated according to PC1 and PC2 (eigenvalues in Supplementary Table 7, means values Supplementary Table 8). T. pseudonana showed, independently from copepods pressure, a reduced area combined with an increased circularity. C. weissflogii had a different morphological behaviour depending on the presence of grazers: they reduced area and circularity when copepods were in the culture, contrarily they increased area and circularity when copepods were absent. PCA in C. closterium (Figure 6C, eigenvalues in Supplementary Table 7, means values Supplementary Table 8) explained 99.7% of the total variance (PC1 and PC2 being 53.4 and 46.3%). Here, PC1 differentiated all the experimental days, recording a significant fluctuation in cell length and height during the experiment in both conditions (copepods exposure and interspecies competition). Furthermore, in days 1 and 3, interspecies competition and grazing pressure data were separated according to PC2, indicating that copepods presence increased diatoms width (Supplementary Table 8).
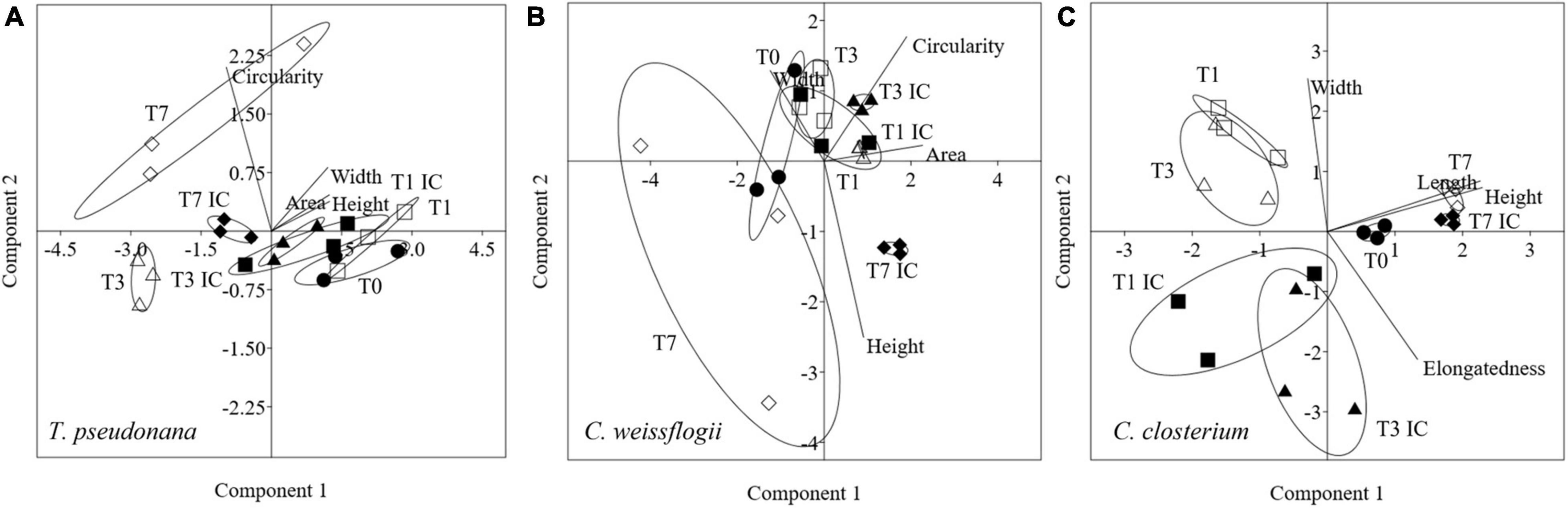
Figure 6. PCA analysis on morphological characteristics in T. pseudonana (A), C. weissflogii (B) and C. closterium (C) when mixed together. Different symbols indicate different time during the experiment: T0 ○●; T1 □■, T3△▲, T7 ◇◆; white symbols indicate copepods exposure while black symbols indicate absence of copepods and interspecies competition (IC) only.
In the mixed culture of the four diatom species exposed to copepods (Figure 7A), after initial growth, T. pseudonana showed no further variation in the next days, whereas in the absence of grazers (Figure 7B) showed a decline at day 7. C. weissflogii in both the situations (Figures 7A,B), never succeeded in growing at day 7, as occurred in the previously described mix (i.e., two centric diatoms and C. closterium, see also Figure 5). In the presence of grazers (Figure 7A), both pennate diatoms had a decline in growth at day 3, but they succeeded in increasing cell density at day 7, while in the absence of copepods (Figure 7B) C. closterium was the only one which managed to grow at day 7. In conclusion, C. closterium was the only diatom showing a positive trend of growth independently of the presence of grazers (Supplementary Figures 3B,C).
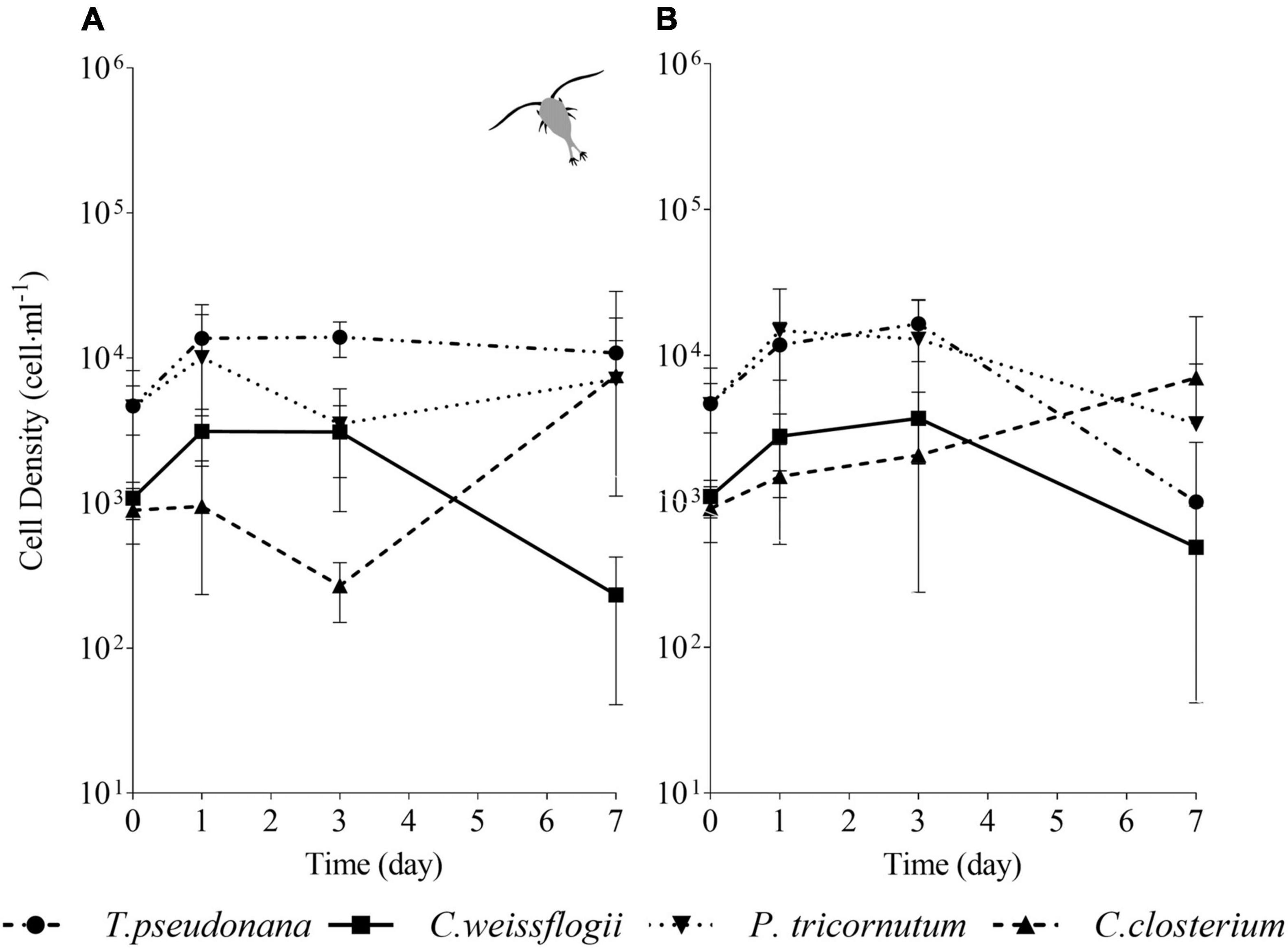
Figure 7. Comparison between growth curves of the diatom mix containing all the species exposed (A) or not (B) to copepods. The data are means of 3 biological replicas. Error bars show SD; no significant difference is observed (two-way ANOVA, p < 0.05).
In Figure 8, the PCA pictured increasing complexity due to 4 mixed diatom species in a single culture; it accounted for 99.5% (PC1 + PC2 being 78.8 + 20.7%, Figure 8A), 93.4% (PC1 + PC2 being 59.7 + 33.9%, Figure 8B), 97% (PC1 + PC2 being 92.1 + 4.9%. Figure 8C) and 99.4% (PC1 + PC2 being 86.4 + 13.0%, Figure 8D) of the overall variation in T. pseudonana, C. weissflogii, P. tricornutum, and C. closterium respectively (eigenvalues in Supplementary Table 9, means values Supplementary Table 10). Conversely from what observed in the other mixes (Figures 4, 6), the two centric diatoms T. pseudonana (8A) and C. weissflogii (8B) did not show a regular trend in morphological changes during the experiment. In C. closterium (8D), different days of exposure were separated according to PC1; as occurred in the previous mix (see also Figure 6C), length and height fluctuations during the experiment were recorded. P. tricornutum (8C) was not characterised by consistent shape evolution during the experiment.
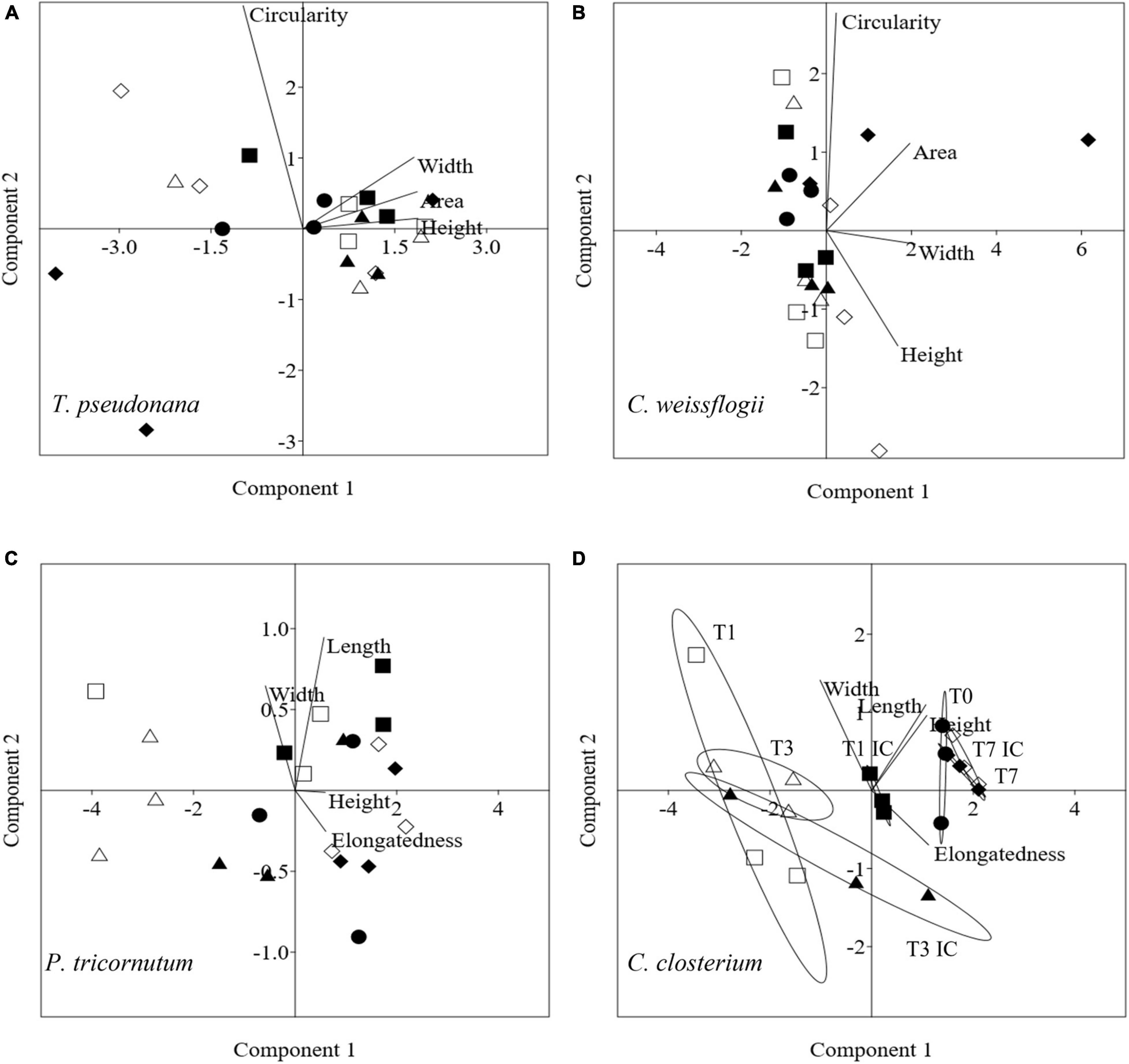
Figure 8. PCA analysis on morphological characteristics in T. pseudonana (A), C. weissflogii (B) P. tricornutum (C) and C. closterium (D) when mixed together. Different symbols indicate different time during the experiment: T0 ○●; T1 □■, T3△▲, T7 ◇◆; white symbols indicate copepods exposure while black symbols indicate absence of copepods and interspecies competition (IC) only.
Discussion
Monospecific Cultures
Of the four monospecific cultured diatoms, T. pseudonana was the most affected by predation and showed a population decline when grown together with copepods compared to cultures maintained without these predators (Figure 1A). The initial size (161 ± 3 μm3, Supplementary Table 2) and the shape characteristics of T. pseudonana were clearly fit to feed the adult copepods. Although cells of T. pseudonana exposed to copepods changed in morphology and elemental composition (Figures 1A,C and Supplementary Tables 1, 2, 4), these alterations were insufficient to counter the decrease of the predated populations. In diatoms, increasing Si content is a known strategy to rise mechanical strength and to reduce predation pressure (Pančić et al., 2019; Grønning and Kiørboe, 2020). However, a higher Si deposition corresponds to a higher cost of shell thickening (Friedrichs et al., 2013; Pančić et al., 2019; Grønning and Kiørboe, 2020) and thus, this extra cost may also have contributed to the lower growth rate shown in Figures 1A,D for T. pseudonana.
Unlike T. pseudonana, Si shell thickening occurred in C. weissflogii already after 24 h of exposure to the predators (Figure 1B), confirming a similar trend described in the literature (Pondaven et al., 2007; Pančić et al., 2019). A temporary growth decline was observed while this extra Si accumulation happened (Figure 1B), this could be explained by the extra cost associated with the shell thickening process as discussed above.
Considering the observed maximum cell density (Figures 1B,D), a higher mechanical resilience successfully acted as feeding deterrent against predators in C. weissflogii but not in T. pseudonana. Our data suggested size may play a major role, as the studied C. weissflogii is c. 30 times bigger than the examined T. pseudonana (Supplementary Table 2). Moreover, bigger size and higher silicification play an important role in sinking, driving vertical movements in the water column, steering prey away from certain predators (Raven and Waite, 2004; Gemmell et al., 2016). The response of C. weissflogii to the studied predators allowed to overcome predation by copepods as it represented the best compromise between minimizing the cost of adjusting to the new condition and growth rate maximisation (Giordano, 2013).
Copepods also fed on cultured pennate diatoms (as proved by the presence of fecal pellets, data not shown) but algal growth rates and maximum cell densities were unaltered compared to the values in unexposed cells (Figure 2D). Such response was already observed in Synechococcus sp. cultures under similar predation pressure and discussed in Ratti et al. (2011), where these authors stipulated that microalgal growth is being stimulated in presence of predators.
The P. tricornutum we studied did not present any change in Si content per biovolume (Figure 2A) suggesting that these small, spindle shaped and very poorly silicified cells do not rely on Si shell thickness for their defence against predators. On the other hand, becoming more elongated in shape (Figure 2C and Supplementary Table 4) likely provided a better buoyancy (Durante et al., 2019). Shape dependent predator-prey interaction likely caused the evolution of new defence strategies (i.e., vertical movements) in these elongated organisms (Pančić and Kiørboe, 2018). Among possible strategies P. tricornutum is known to produce apo-fucoxanthinoids as feeding deterrents (Shaw et al., 1995a,b, 1997; Tammilehto et al., 2015). The production of an anti-predation substance could be a valid explanation for the high number of dead copepods (and needed to be replaced) in cultures of P. tricornutum (Supplementary Figure 2A).
Mixed Cultures
When growing populations of different diatom species in one culture, the study of predator-prey interactions from an ecophysiological point of view also involves interspecies competition (e.g., for resources, algal interactions) (Armbrust, 2009; Vallina et al., 2014; Vincent and Bowler, 2020) and to the best of our knowledge there are no detailed studies available combining predation pressure and interspecies competition involving several species of diatoms exposed to copepods. For instance, competition among species was observed in our mixed cultures where diatoms showed lower growth rates (Supplementary Figure 3A) compared to the ones in control conditions (Figures 1D, 2D).
Moreover, the small diatom T. pseudonana had higher growth rates than the bigger C. weissflogii (Figure 3 and Supplementary Figure 3A), this most probably due to a larger surface-to-volume (S/V) ratio in T. pseudonana, which allowed a more efficient resource uptake, especially when algal growth reduced the availability of nutrients and light in the mixed culture (Reynolds, 2006; Ryabov et al., 2021).
The interspecies competition brought out a striking difference between the growth rates of the C. weissflogii populations in the co-cultured centrics with and without copepods (Figure 3 and Supplementary Figure 3A) suggesting that copepods preferred bigger diatoms. Although this behaviour did not support the selection of smaller preys to prevent C loss according to the “sloppy feeding” theory (Roy et al., 1989; Moller and Nielsen, 2001; Moller, 2005), it has already been observed that T. longicornis came up with extraordinary ways to avoid inefficient nutrient uptake (Jansen, 2008) optimizing feeding tools (e.g., mouth appendages) to capture and break even large diatom frustules without losing cell organic matter (Smetacek, 2001). The energetic convenience of feeding on larger cells is likely due to the lower number of bites performed to acquire a higher amount of nutrients per cell (Friedrichs et al., 2013) as shown by our results (Supplementary Tables 1, 2).
When culturing C. weissflogii and T. pseudonana populations together, from our findings morphological changes in the latter did not depend on predation pressure but rather on competition for resources since reducing surface area (Figure 4 and Supplementary Table 6) improves the S/V ratio. Contrary, cells of C. weissflogii increased their area and reduced circularity (Figure 4 and Supplementary Table 6) becoming bigger due to the growth limitation independently from predation pressure. This can be explained by the general trend in diatoms to accumulate fixed C in macromolecular storage pools (i.e., lipids, carbohydrates) and thus enlarging their cell volume (Palmucci et al., 2011; Giordano et al., 2017) when resource availability decreases. Thus, according to our data, interspecies competition disadvantaged bigger species (cells) when co-cultured with a smaller species (cells), even more so when copepods are added to the mix because the increasing size, due to resource limitation, made the larger diatoms even more susceptible to predation.
When the pennate diatom C. closterium was co-cultured with the centric diatoms, in our setup including copepods, T. pseudonana growth was still favoured (Figures 5, 7 and Supplementary Figures 3B,C) compared to the two bigger species (Supplementary Table 2), at least for the first 3 days (when resources were likely still plentiful), again proving the size dependent prey selection by predators. Conversely, in the setup without copepods, where big cells were not selectively eaten, cells of T. pseudonana stopped growing after the first day while the pennate diatom species showed the highest cell density after day 3 (Figure 5B). Overall, C. closterium seemed to be the best performing in terms of growth with or without copepods (Figures 5, 7 and Supplementary Figures 3B,C). A similar trend was observed when the 2 centric diatoms were cultured together with both pennate species (Figures 5A, 7A). The morphological response followed growth behaviour, this is particularly visible in cells of C. closterium where the elongated shape which characterised this diatom at the start (day 0) was attenuated at time 1 and 3, when a reduction in length and an increase in width were observed (Supplementary Tables 8, 10). Remarkably, the elongated shape observed on day 0 was then restored on day 7 (Figures 6C, 8D). In general, our data show that, in the presence of copepods, small diatoms increase growth performance compared to the bigger diatoms in the same culture, but when predation is absent and interspecies competition acts as the only stress factor, an elongated shaped diatom is more efficient in resource acquisition than a radial shaped one (Pahlow et al., 1997; Key et al., 2010; Naselli-Flores and Barone, 2011; Naselli-Flores et al., 2021; Ryabov et al., 2021).
Under predation pressure, although frustules of pennate diatoms are in general less thick (silicified) compared to centric ones (Finkel and Kotrc, 2010; Kotrc and Knoll, 2015) and copepods may theoretically spend less energy by processing them, the pennate shape per se benefits cells in escaping from predators. Indeed, elongated diatoms have lower sinking rates (Durante et al., 2019), therefore better buoyancy and stability in the water column allowing active substrate adhesion and gliding motion thanks to raphae (Cohn and Disparti, 1994) as a response to many external factors such as light (Cohn et al., 2015), monosaccharides (Cooksey and Cooksey, 1988), dissolved silicate (Bondoc et al., 2016a) and pheromone (Bondoc et al., 2016b, 2019a,b). Since the pennate diatoms used in this study were raphid, we could not rule out that adhesion and gliding motion were involved to actively migrate away from microcurrents generated by copepods appendix to convey algae near to their mouth (Sengupta et al., 2017). Moreover, as already discussed for monospecific cultures, the higher number of dead copepods replaced in mixed cultures where P. tricornutum was present (Supplementary Figure 2B) could mark the presence of toxic metabolites (Pančić and Kiørboe, 2018) which were most likely produced by P. tricornutum as a response to the predation pressure (Shaw et al., 1995a,b, 1997; Tammilehto et al., 2015). Cell size, robustness, shape and behaviour are therefore crucial in moulding phytoplanktonic communities, as the aforementioned characteristics are not only involved in actively or passively avoiding predators, but also in nutrient uptake, photosynthetic efficiency and buoyancy.
Data Availability Statement
The original contributions presented in the study are included in the article/Supplementary Material, further inquiries can be directed to the corresponding author.
Author Contributions
AP carried out all the experiments and analysed the data. PC was involved in diatom cultivation, Imaging Flow Cytometry measurements and analysis. AN conceived and designed the project and helped carrying out the experiments. AP, PC, and AN wrote the manuscript. All authors contributed to the article and approved the submitted version.
Funding
This work was carried at the University of Ghent and Flanders Marine Institute in the context of ASSEMBLE Plus Transnational Access, research infrastructure initiative being funded by the European Union’s Horizon 2020 Research and Innovation Programme. The project called DIGRAE (Diatoms’ grazing experiment) received the Grant No. 730984 in the 3rd call, September 2019. Research for AP was also funded by Cariverona Foundation, Italy.
Conflict of Interest
The authors declare that the research was conducted in the absence of any commercial or financial relationships that could be construed as a potential conflict of interest.
Publisher’s Note
All claims expressed in this article are solely those of the authors and do not necessarily represent those of their affiliated organizations, or those of the publisher, the editors and the reviewers. Any product that may be evaluated in this article, or claim that may be made by its manufacturer, is not guaranteed or endorsed by the publisher.
Acknowledgments
We wish to thank Andre Cattrijsse, who helped in sampling copepods from the Belgian North Sea. Especial gratitude goes to the late Mario Giordano who contributed with ideas on prey predator interaction.
Supplementary Material
The Supplementary Material for this article can be found online at: https://www.frontiersin.org/articles/10.3389/fmars.2021.804960/full#supplementary-material
Footnotes
References
Armbrust, E. V. (2009). The life of diatoms in the world’s oceans. Nature 459, 185–192. doi: 10.1038/nature08057
Bondoc, K. G. V., Heuschele, J., Gillard, J., Vyverman, W., and Pohnert, G. (2016a). Selective silicate-directed motility in diatoms. Nat. Commun. 7: 10540.
Bondoc, K. G. V., Lembke, C., Vyverman, W., and Pohnert, G. (2016b). Searching for a mate: pheromone-directed movement of the benthic diatom Seminavis robusta. Microb. Ecol. 72, 287–294. doi: 10.1007/s00248-016-0796-7
Bondoc, K. G. V., Lembke, C., Lang, S. N., Germerodt, S., Schuster, S., Vyverman, W., et al. (2019a). Decision-making of the benthic diatom Seminavis robusta searching for inorganic nutrients and pheromones. ISME J. 13, 537–546.
Bondoc, K. G. V., Lembke, C., Vyverman, W., and Pohnert, G. (2019b). Selective chemoattraction of the benthic diatom Seminavis robusta to phosphate but not to inorganic nitrogen sources contributes to biofilm structuring. Microbiologyopen 8:e694. doi: 10.1002/mbo3.694
Cohn, S. A., and Disparti, N. C. (1994). Environmental factors influencing diatom cell motility. J. Phycol. 30, 818–828. doi: 10.1111/j.0022-3646.1994.00818.x
Cohn, S. A., Halpin, D., Hawley, N., Ismail, A., Kaplan, Z., Kordes, T., et al. (2015). Comparative analysis of light-stimulated motility responses in three diatom species. Diatom Res. 30, 213–225. doi: 10.1080/0269249X.2015.1058295
Conley, D. J., Frings, P. J., Fontorbe, G., Clymans, W., Stadmark, J., Hendry, K. R., et al. (2017). Biosilicification drives a decline of dissolved si in the oceans through geologic time. Front. Mar. Sci. 4:397. doi: 10.3389/fmars.2017.00397
Cooksey, B., and Cooksey, K. E. (1988). Chemical signal-response in diatoms of the genus Amphora. J. Cell Sc. 91, 523–529.
Durante, G., Basset, A., Stanca, E., and Roselli, L. (2019). Allometric scaling and morphological variation in sinking rate of phytoplankton. J. Phycol. 55, 1386–1393. doi: 10.1111/jpy.12916
Finkel, Z. V., and Kotrc, B. (2010). Silica use through time: macroevolutionary change in the morphology of the diatom fustule. Geomicrobiol. J. 27, 596–608. doi: 10.1080/01490451003702941
Finkel, Z. V., Matheson, K. A., Regan, K. S., and Irwin, A. J. (2010). Genotypic and phenotypic variation in diatom silicification under paleo-oceanographic conditions: diatom silicification. Geobiology 8, 433–445. doi: 10.1111/j.1472-4669.2010.00250.x
Friedrichs, L., Hörnig, M., Schulze, L., Bertram, A., Jansen, S., and Hamm, C. (2013). Size and biomechanic properties of diatom frustules influence food uptake by copepods. Mar. Ecol. Prog. Ser. 481, 41–51. doi: 10.3354/meps10227
Gemmell, B. J., Oh, G., Buskey, E. J., and Villareal, T. A. (2016). Dynamic sinking behaviour in marine phytoplankton: rapid changes in buoyancy may aid in nutrient uptake. Proc. R. Soc. B 283:20161126. doi: 10.1098/rspb.2016.1126
Giordano, M. (2013). Homeostasis: an underestimated focal point of ecology and evolution. Plant Sci. 211, 92–101. doi: 10.1016/j.plantsci.2013.07.008
Giordano, M., Norici, A., and Beardall, J. (2017). Impact of inhibitors of amino acid, protein, and RNA synthesis on C allocation in the diatom Chaetoceros muellerii: a FTIR approach. Algae 32, 161–170. doi: 10.4490/algae.2017.32.6.6
Giordano, M., Olivieri, C., Ratti, S., Norici, A., Raven, J. A., and Knoll, A. H. (2018). A tale of two eras: phytoplankton composition influenced by oceanic paleochemistry. Geobiology 16, 498–506. doi: 10.1111/gbi.12290
Grønning, J., and Kiørboe, T. (2020). Diatom defence: grazer induction and cost of shell-thickening. Funct. Ecol. 34, 1790–1801. doi: 10.1111/1365-2435.13635
Guillard, R. R. L. (1975). “Culture of phytoplankton for feeding marine invertebrates,” in Culture of Marine Invertebrate Animals, eds W. L. Smith, and M. H. Chanley (Boston, MA: Springer). doi: 10.1007/978-1-4615-8714-9_3
Hamm, C., and Smetacek, V. (2007). “Armor: why, when, and how,” in Evolution of Primary Producers in the Sea, eds P. G. Falkowski and A. H. Knoll (London: Elsevier), 311–332. doi: 10.1016/B978-012370518-1/50015-1
Hamm, C. E., Merkel, R., Springer, O., Jurkojc, P., Maier, C., Prechtel, K., et al. (2003). Architecture and material properties of diatom shells provide effective mechanical protection. Nature 421, 841–843. doi: 10.1038/nature01416
Hammer, O., Harper, D. A. T., and Ryan, P. D. (2001). PAST: paleontological statistics software package for education and data analysis. Palaeontol. Electron. 4:9.
Hildebrand, M., Lerch, S. J. L., and Shrestha, R. P. (2018). Understanding diatom cell wall silicification—moving forward. Front. Mar. Sci. 5:125. doi: 10.3389/fmars.2018.00125
Hillebrand, H., Dürselen, C.-D., Kirschtel, D., Pollingher, U., and Zohary, T. (1999). Biovolume calculation for pelagic and benthic microalgae. J. Phycol. 35, 403–424. doi: 10.1046/j.1529-8817.1999.3520403.x
Jansen, S. (2008). Copepods grazing on Coscinodiscus wailesii: a question of size? Helgol. Mar. Res. 62, 251–255. doi: 10.1007/s10152-008-0113-z
Key, T., McCarthy, A., Campbell, D. A., Six, C., Roy, S., and Finkel, Z. V. (2010). Cell size trade-offs govern light exploitation strategies in marine phytoplankton. Environ. Microbiol. 12, 95–104. doi: 10.1111/j.1462-2920.2009.02046.x
Kotrc, B., and Knoll, A. H. (2015). A morphospace of planktonic marine diatoms. I. Two views of disparity through time. Paleobiology 41, 45–67. doi: 10.1017/pab.2014.4
Lazarus, D., Barron, J., Renaudie, J., Diver, P., and Türke, A. (2014). Cenozoic planktonic marine diatom diversity and correlation to climate change. PLoS One 9:e84857. doi: 10.1371/journal.pone.0084857
Lazarus, D. B., Kotrc, B., Wulf, G., and Schmidt, D. N. (2009). Radiolarians decreased silicification as an evolutionary response to reduced Cenozoic ocean silica availability. Proc. Natl. Acad. Sci. 106, 9333–9338. doi: 10.1073/pnas.0812979106
Liu, H., Chen, M., Zhu, F., and Harrison, P. J. (2016). Effect of diatom silica content on copepod grazing, growth and reproduction. Front. Mar. Sci. 3:89. doi: 10.3389/fmars.2016.00089
Lürling, M. (2020). Grazing resistance in phytoplankton. Hydrobiologia 848, 237–249. doi: 10.1007/s10750-020-04370-3
Maliva, R. G., Knoll, A. H., and Siever, R. (1989). Secular change in chert distribution: a reflection of evolving biological participation in the silica cycle. Palaios 4:519. doi: 10.2307/3514743
Martin-Jezequel, V., Hildebrand, M., and Brzezinski, M. A. (2000). Silicon metabolism in diatoms: implications for growth. J. Phycol. 36, 821–840. doi: 10.1046/j.1529-8817.2000.00019.x
Miller, C. B., Nelson, D. M., Weiss, C., and Soeldner, A. H. (1990). Morphogenesis of opal teeth in calanoid copepods. Mar. Biol. 106, 91–101. doi: 10.1007/BF02114678
Moller, E. F., and Nielsen, T. G. (2001). Production of bacterial substrate by marine copepods: effect of phytoplankton biomass and cell size. J. Plankton Res. 23, 527–536. doi: 10.1093/plankt/23.5.527
Moller, E. F. (2005). Sloppy feeding in marine copepods: prey-size-dependent production of dissolved organic carbon. J. Plankton Res. 27, 27–35. doi: 10.1093/plankt/fbh147
Naselli-Flores, L., and Barone, R. (2011). Invited review - fight on plankton! or, phytoplankton shape and size as adaptive tools to get ahead in the struggle for life. Cryptogam. Algol. 32, 157–204. doi: 10.7872/crya.v32.iss2.2011.157
Naselli-Flores, L., Zohary, T., and Padisák, J. (2021). Life in suspension and its impact on phytoplankton morphology: an homage to Colin S. Reynolds. Hydrobiologia 848, 7–30. doi: 10.1007/s10750-020-04217-x
Pahlow, M., Riebesell, U., and Wolf-Gladrow, D. A. (1997). Impact of cell shape and chain formation on nutrient acquisition by marine diatoms. Limnol. Oceanogr. 42, 1660–1672. doi: 10.4319/lo.1997.42.8.1660
Palmucci, M., Ratti, S., and Giordano, M. (2011). Ecological and evolutionary implications of carbon allocation in marine phytoplankton as function of nitrogen availability: a fourier transform infrared spectroscopy approach: C allocation as a function of N availability. J. Phycol. 47, 313–323. doi: 10.1111/j.1529-8817.2011.00963.x
Pančić, M., and Kiørboe, T. (2018). Phytoplankton defence mechanisms: traits and trade-offs: defensive traits and trade-offs. Biol. Rev. 93, 1269–1303. doi: 10.1111/brv.12395
Pančić, M., Torres, R. R., Almeda, R., and Kiørboe, T. (2019). Silicified cell walls as a defensive trait in diatoms. Proc. R. Soc. B 286:20190184. doi: 10.1098/rspb.2019.0184
Pondaven, P., Gallinari, M., Chollet, S., Bucciarelli, E., Sarthou, G., Schultes, S., et al. (2007). Grazing-induced changes in cell wall silicification in a marine diatom. Protist 158, 21–28. doi: 10.1016/j.protis.2006.09.002
Ratti, S., Knoll, A. H., and Giordano, M. (2011). Did sulfate availability facilitate the evolutionary expansion of chlorophyll a+c phytoplankton in the oceans: sulfate and evolution of chlorophyll a+c phytoplankton. Geobiology 9, 301–312. doi: 10.1111/j.1472-4669.2011.00284.x
Ratti, S., Knoll, A. H., and Giordano, M. (2013). Grazers and phytoplankton growth in the oceans: an experimental and evolutionary perspective. PLoS One 8:e77349. doi: 10.1371/journal.pone.0077349
Raven, J. A., and Waite, A. M. (2004). The evolution of silicification in diatoms: inescapable sinking and sinking as escape? New Phytol. 162, 45–61. doi: 10.1111/j.1469-8137.2004.01022.x
Reynolds, C. S. (2006). The Ecology of Phytoplankton. Ecology, Biodiversity and Conservation. Cambridge: Cambridge University Press.
Roy, S., Harris, R., and Poulet, S. (1989). Inefficient feeding by Calanus helgolandicus and Temora longicornis on Coscinodiscus wailesii: quantitative estimation using chlorophyll-type pigments and effects on dissolved free amino acids. Mar. Ecol. Prog. Ser. 52, 145–153. doi: 10.3354/meps052145
Ryabov, A., Kerimoglu, O., Litchman, E., Olenina, I., Roselli, L., Basset, A., et al. (2021). Shape matters: the relationship between cell geometry and diversity in phytoplankton. Ecol. Lett. 24, 847–861. doi: 10.1111/ele.13680
Sengupta, A., Carrara, F., and Stocker, R. (2017). Phytoplankton can actively diversify their migration strategy in response to turbulent cues. Nature 543, 555–558. doi: 10.1038/nature21415
Shaw, B. A., Andersen, R. J., and Harrison, P. J. (1995a). Feeding deterrence properties of apo-fucoxanthinoids from marine diatoms. I. Chemical structures of apo-fucoxanthinoids produced by Phaeodactylum tricornutum. Mar. Biol. 124, 467–472. doi: 10.1007/BF00363921
Shaw, B. A., Andersen, R. J., and Harrison, P. J. (1995b). Feeding deterrence properties of apo-fucoxanthinoids from marine diatoms. I1. Physiology of production of apo-fucoxanthinoids by the marine diatoms Phaeodactylum tricornutum and Thalassiosira pseudonana, and their feeding deterrent effects on the copepod Tigriopus californicus. Mar. Biol. 124, 473–481.
Shaw, B. A., Andersen, R. J., and Harrison, P. J. (1997). Feeding deterrent and toxicity effects of apo-fucoxanthinoids and phycotoxins on a marine copepod (Tigriopus californicus). Mar. Biol. 128, 273–280. doi: 10.1007/s002270050092
Tammilehto, A., Nielsen, T. G., Krock, B., Møller, E. F., and Lundholm, N. (2015). Induction of domoic acid production in the toxic diatom Pseudo-nitzschia seriata by calanoid copepods. Aquat. Toxicol. 159, 52–61.
Turner, J. T. (2004). The importance of small planktonic copepods and their roles in pelagic marine food webs. Zool. Stud. 43, 255–266.
Vallina, S. M., Follows, M. J., Dutkiewicz, S., Montoya, J. M., Cermeno, P., and Loreau, M. (2014). Global relationship between phytoplankton diversity and productivity in the ocean. Nat. Commun. 5:4299. doi: 10.1038/ncomms5299
Vincent, F., and Bowler, C. (2020). Diatoms are selective segregators in global ocean planktonic communities. mSystems 5:e00444-19. doi: 10.1128/mSystems.00444-19
Xu, H., Shi, Z., Zhang, X., Pang, M., Pan, K., and Liu, H. (2021). Diatom frustules with different silica contents affect copepod grazing due to differences in the nanoscale mechanical properties. Limnol. Oceanogr., lno. 66, 3408–3420. doi: 10.1002/lno.11887
Keywords: predation, diatom, morphology, interspecies competition, silicification, frustule
Citation: Petrucciani A, Chaerle P and Norici A (2022) Diatoms Versus Copepods: Could Frustule Traits Have a Role in Avoiding Predation? Front. Mar. Sci. 8:804960. doi: 10.3389/fmars.2021.804960
Received: 29 October 2021; Accepted: 30 December 2021;
Published: 03 February 2022.
Edited by:
Hongbin Liu, Hong Kong University of Science and Technology, Hong Kong SAR, ChinaReviewed by:
Jelena Godrijan, Rudjer Boskovic Institute, CroatiaYelena Likhoshway, Limnological Institute, Russian Academy of Sciences (RAS), Russia
Alessandra Rogato, Institute of Bioscience and Bioresources, Italian National Research Council, Italy
Copyright © 2022 Petrucciani, Chaerle and Norici. This is an open-access article distributed under the terms of the Creative Commons Attribution License (CC BY). The use, distribution or reproduction in other forums is permitted, provided the original author(s) and the copyright owner(s) are credited and that the original publication in this journal is cited, in accordance with accepted academic practice. No use, distribution or reproduction is permitted which does not comply with these terms.
*Correspondence: Alessandra Norici, YS5ub3JpY2lAdW5pdnBtLml0