- 1National Demonstration Center for Experimental Fisheries Science Education (Shanghai Ocean University), Shanghai, China
- 2Key Laboratory of Aquatic Genomics, Ministry of Agriculture and Rural Affairs, Yellow Sea Fisheries Research Institute, Chinese Academy of Fishery Sciences, Qingdao, China
- 3Laboratory for Marine Fisheries Science and Food Production Processes, Qingdao National Laboratory for Marine Science and Technology, Qingdao, China
- 4Nicholas School of the Environment, Duke University, Durham, NC, United States
- 5Key Laboratory of Sustainable Development of Marine Fisheries, Ministry of Agriculture and Rural Affairs, Yellow Sea Fisheries Research Institute, Chinese Academy of Fishery Sciences, Qingdao, China
Ammonia is a common environmental stressor encountered during aquaculture, and is a significant concern due to its adverse biological effects on vertebrate and invertebrate including crustaceans. However, little information is available on physiological and molecular responses in crustaceans under long-term ammonia exposure, which often occurs in aquaculture practices. Here, we investigated temporal physiological and molecular responses in the gills, the main ammonia excretion organ, of the swimming crab Portunus trituberculatus following long-term (4 weeks) exposure to three different ammonia nitrogen concentrations (2, 4, and 8 mg l–1), in comparison to seawater (ammonia nitrogen below 0.03 mg l–1). The results revealed that after ammonia stress, the ammonia excretion and detoxification pathways were initially up-regulated. These processes appear compromised as the exposure duration extended, leading to accumulation of hemolymph ammonia, which coincided with the reduction of adenosine 5′-triphosphate (ATP) and adenylate energy charge (AEC). Considering that ammonia excretion and detoxification are highly energy-consuming, the depression of these pathways are, at least partly, associated with disruption of energy homeostasis in gills after prolonged ammonia exposure. Furthermore, our results indicated that long-term ammonia exposure can impair the antioxidant defense and result in increased lipid peroxidation, as well as induce endoplasmic reticulum stress, which in turn lead to apoptosis through p53-bax pathway in gills of the swimming crab. The findings of the present study further our understanding of adverse effects and underlying mechanisms of long-term ammonia in decapods, and provide valuable information for aquaculture management of P. trituberculatus.
Introduction
Ammonia is a common environmental stressor in aquatic ecosystem, and is also of significant concern to the aquaculture industry. In intensive aquaculture systems, ammonia is mostly derived from the decomposition of uneaten feed and from the brachial excretion of the cultured animals (Romano and Zeng, 2007). As aquaculture is moving toward more intensive systems with high stocking density and feed supply, there has been rising concerns on ammonia levels in crustacean aquaculture industry. In recent years, an increasing number of studies have been performed to understand the adverse effects and defensive mechanisms in crustaceans (Zhao et al., 2020). However, most of these studies focused on acute ammonia exposure, and there has been limited information available on stress responses in crustaceans under long-term exposure (Liang et al., 2019; Liu F. et al., 2020). Previous studies in fish showed that the detrimental effects of ammonia is largely influenced by the duration of exposure (Brinkman et al., 2009). In addition, during aquaculture practice, ammonia may accumulate over time due to excessive feeding, and culture animals may be exposed at HEA for a long period, leading to high rates of mortality. Therefore, it is of great significance to study the stress responses of crustaceans under long-term ammonia stress.
Ammonia is present in water in two forms, unionized (NH3) and ionized (NH4+) (in this study, the term “ammonia” refers to the sum of NH3 and NH4+). The unionized NH3 is more toxic because it is diffusible through phospholipid bilayers of the gill epithelial cells (Benli et al., 2008). High environmental ammonia (HEA) may result in accumulation of internal ammonia, and in turn, cause a board range of adverse effects in aquatic animals. At the organismal level, ammonia can lead to reduction in growth (Lemarié et al., 2004; Foss et al., 2009), decreased resistance to diseases (Ackerman et al., 2006; Zhang et al., 2019), histopathological changes in tissues (Romano and Zeng, 2007; Liang et al., 2016), and even death (Romano and Zeng, 2010; Henry et al., 2017). At the physiological and molecular levels, ammonia can alter hormonal regulation (Cui et al., 2017; Zhang X. et al., 2020), influence ionic balance (Romano and Zeng, 2010; Henry et al., 2012), affect energy metabolism (Racotta and Herna′ndez-Herrera, 2000; Shan et al., 2019), induce oxidative stress and endoplasmic reticulum (ER) stress (Ching et al., 2009; Liang et al., 2016), and trigger apoptosis (Cheng et al., 2015; Zhang T. et al., 2020).
To protect against ammonia stress, various excretory and defense mechanisms have been reported in aquatic animals including in crustaceans (Ip et al., 2001; Weihrauch et al., 2004; Zhao et al., 2020). As ammonotelic animals, crustaceans can excrete ammonia actively against an inwardly directed gradient. Gills are considered to act as the predominant excretory organs for ammonia in aquatic crustaceans (Henry et al., 2012). Previous studies have shown that ammonia can be transported through the gills via several transport proteins, including Rhesus (Rh) glycoprotein, Na+-K+-ATPase (NKA), Na+/K+/2Cl–-cotransporter (NKCC), and Na+/H+-exchanger (NHE) (Lucu et al., 1989; Weihrauch et al., 2017; Weihrauch and Allen, 2018). Furthermore, ammonia can also be excreted from the gills with a microtubule dependent mechanism with vacuolar-type H+-ATPase (VAT) and vesicle associated membrane protein (VAMP) (Weihrauch et al., 2002). There are evidences that crustaceans possess ammonia detoxifying mechanisms involving the conversion of ammonia to glutamine through the combined action of glutamate dehydrogenase (GDH) and glutamine synthetase (GS) (Murray et al., 2003), and to less-toxic urea by the ornithine-urea cycle (OUC) (Liu et al., 2014).
The swimming crab Portunus trituberculatus (Crustacea: Decapoda: Brachyura) is widely distributed in the estuary and coastal areas of Korea, Japan, China, and Southeast Asia (Dai et al., 1986). This species dominates the swimming crab fishery around the world, and is an important aquaculture species in China with a production of 116,251 tons in 2018 (China Agriculture Press, 2020). In intensive aquaculture systems, the swimming crabs are often exposed to elevated ammonia in coastal ponds. Although several studies reported the physiological responses of this species to acute ammonia (Ren et al., 2015; Pan et al., 2018; Si et al., 2018), there has been no report describing the stress responses under prolonged ammonia exposure, a common occurrence in aquaculture operations. In this study, we investigated the temporal responses associated with ammonia excretion and detoxification, energy metabolism, antioxidant defense, unfolded protein responses (UPR), and apoptosis in the gills, the major excretory organs for ammonia, of P. trituberculatus during long-term ammonia stress. The results provide a better understanding of detrimental effects during long-term ammonia exposure and the underlying mechanisms in the swimming crab, and valuable information for improving aquaculture management.
Materials and Methods
Animals and Experimental Setup
Adult female P. trituberculatus with an average weight of 213.84 ± 21.55 g (means ± S.D.) were obtained from Haifeng company (Weifang, China). After transferring to the laboratory, crabs without visible damage were reared for 2 weeks at ambient temperature of 14.0 ± 0.8°C, that is considered the optimum temperatures for these organisms. Water was well-aerated and the pH was 7.6 ± 0.2, salinity was 30.3 ± 0.6, ammonia nitrogen concentration was below 0.03 mg l–1 and the photoperiod was set as 12 h of light: 12 h of dark. One half of water was exchanged in each tank daily, and the crabs were fed ad libitum everyday with fresh clams Ruditapes philippinarum.
After acclimation, eighty crabs were randomly allocated into four recirculating systems, each system contains twenty 10-L tanks. To avoid cannibalism, each tank only has one individual. Based on the results from our survey on seawater quality in culture ponds, the highest ammonia level in the seawater near sediments was ∼4 mg l–1. In this study, four concentrations of ammonia nitrogen were chosen for the different groups, below 0.03 mg l–1 (seawater) for the control group, 2.0 mg l–1 for the low-ammonia (LA) group, 4.0 mg l–1 for the medium-ammonia (MA) group, and 8.0 mg l–1 for the high-ammonia (HA) group. The ammonia-nitrogen concentrations for the treatment groups were achieved by infusion of calculated amount of ammonium chloride (NH4Cl) stock solutions that was prepared with filtered seawater (pH was 7.8, salinity was 30.8). Ammonia nitrogen concentration in these groups was monitored using salicylic acid method with spectrophotometer, and it was adjusted with freshly prepared stock solution daily to ensure consistency.
At the end of each week during the total experiment period (4 weeks), five individuals from each group were anesthetized for hemolymph and gill collection. The gills from each crab were collected, snap frozen in liquid nitrogen, and stored in −80°C. Hemolymph samples were obtained by puncturing the arthrodial membrane at the base of the swimming leg with a sterilized syringe containing equal volume of the anti-coagulant, and then centrifuged at 1,500 g at 4°C for 10 min. The supernatant was collected as the plasma sample and frozen at -80°C until analysis. The animal experiment was approved by the Institutional Animal Care and Use Committee of Yellow Sea Fisheries Research Institute.
Physiological Assay
The levels of adenosine 5′-triphosphate (ATP), adenosine 5′-diphosphate (ADP) and adenosine 5′-monophosphate (AMP) in the gill samples were determined by liquid chromatography as described in Lu et al. (2015). The gills from each sample were ground in liquid nitrogen and homogenized in 9 volumes of ice-cold 0.9 mol l–1 perchloric acid. After centrifugation at 4 °C and 7,000 g for 5 min, the supernatant was neutralized to pH 6.5–7.0 with 3.75 M potassium carbonate. Precipitated potassium perchlorate was removed with a second centrifugation, and the homogenate was filtered through a 0.45 μm HV-Millipore filter. The aliquots of 20 μl were injected into an Agilent 1100 high-performance liquid chromatography (HPLC, Agilent Corp., United States) system for analyzing ATP, ADP, and AMP contents on an Ultimate AQ-C18 column (4.6 × 250 mm) at 254 nm, using phosphate buffer (40 mmol l–1 KH2PO4 and 60 mmol l–1 K2HPO4, pH 6.50) as the mobile phases. Flow rate of 1.0 ml min–1, and column temperature was constantly at 35°C. The elution time was 24 min. The concentration of the adenylate was calculated from the measured peak areas and standard curves, which were made from standards of known concentrations (ATP, 0–0.8 mmol/L; ADP, 0–1.2 mmol/L; AMP, 0–1.5 mmol/L). The adenylate energy charge (AEC) was calculated as follow:
The levels of hemolymph ammonia and urea, and the activity of the enzymes involved in ammonia detoxification, including glutamate dehydrogenase (GDH), glutamine synthase (GS), arginase (ARG), were determined with assay kits from Nanjing Jiancheng Bioengineering Institute (Nanjing, China) (ammonia, A086-1-1; urea, C013-2-1; GDH, A125-1-1; GS, A047-1-1) and BioAssay Systems (Hayward, United States) (Arginase, DARG-100).
To study the effects of ammonia on energy metabolism in gills, the activities of hexokinase (HK), pyruvate kinase (PK), and succinate dehydrogenase (SDH) were measured using commercial diagnostic kits purchased from Nanjing Jiancheng Bioengineering Institute (Nanjing, China) (HK, A077-3-1; PK, A076-1-1; SDH, A022-1-1).
The activities of antioxidant enzymes, including superoxide dismutase (SOD), catalase (CAT), and glutathione peroxidase (GPX) and were analyzed with assay kits (Nanjing Jiancheng Bioengineering Institute, Nanjing, China) (SOD, A001-3-2; CAT, A007-1-1; GPX, A005-1-2). Malondialdehyde (MDA) was measured to assess oxidative damage to lipids. MDA concentrations were determined using assay kit (Solarbio Life Science, Beijing, China) (MDA, BC0025).
Gene Expression Analysis
Total RNA of the samples was isolated using TransZol UP Plus RNA Kit (TransGen Biotech, China). A sample of 0.8 μg of total RNA was used as the template for synthesis of the first strand of cDNA with PrimeScript RT reagent kit (Takara, China). The PCR reactions were run in ABI 7500 fast real-time PCR system (Applied Biosystems, United States) using SYBR Green Premix Pro Taq HS qPCR Kit (Accurate Biology, China). The PCR was performed in a total volume of 20 μl, containing 10 μl of 2X SYBR Green Pro Taq HS Premix II, 2 μl of diluted cDNA, 0.8 μl each of 10 mM each primer and 7.2 μl DNase-free water. The PCR program comprised a 95°C activation step for 30 min, followed by 40 cycles of 95°C for 5 s (denaturation) and 60°C for 30 s (annealing and extension). The β-actin was used as the reference gene to normalize expression levels of the target genes. All the specific primers used are listed in Table 1. The relative expression levels were calculated using the 2–△△Ct method (Livak and Schmittgen, 2001).
Statistical Analysis
All the data were analyzed with SPSS 20.0. The levels of physiological parameters and gene expression among different groups at each sample point were analyzed with one-way ANOVA after checking tests of normality and homogeneity of variance. When significant differences were found, Duncan’s-test was used to identify the differences between treatments. The correlation among physiological parameters and gene expression was analyzed using Spearman correlation analysis using the OmicShare tools. Significant differences were considered at P < 0.05.
Results
Energy Metabolism
The levels of adenylates (ATP, AMP, ADP), ADP/ATP, AMP/ATP, and AEC significantly changed during the long-term ammonia exposure (Table 2). For the 4 mg l–1 (MA group) and 8 mg l–1 (HA group) groups, the contents of AMP and ADP, as well as the ratio of ADP/ATP and AMP/ATP showed significant increase, whereas ATP and AEC decreased significantly from 2 week to the end of the experiment (P < 0.05). For the 2 mg l–1 (LA group), ATP content and AEC exhibited significant decrease at the end of the 4 weeks (P < 0.05). The expression of AMPKα and activity of HK, PK, and SDH in the MA and HA groups were significantly elevated at 1 week, after which their levels decreased as exposure time increased and become lower than the control at 4 week (Figure 1).
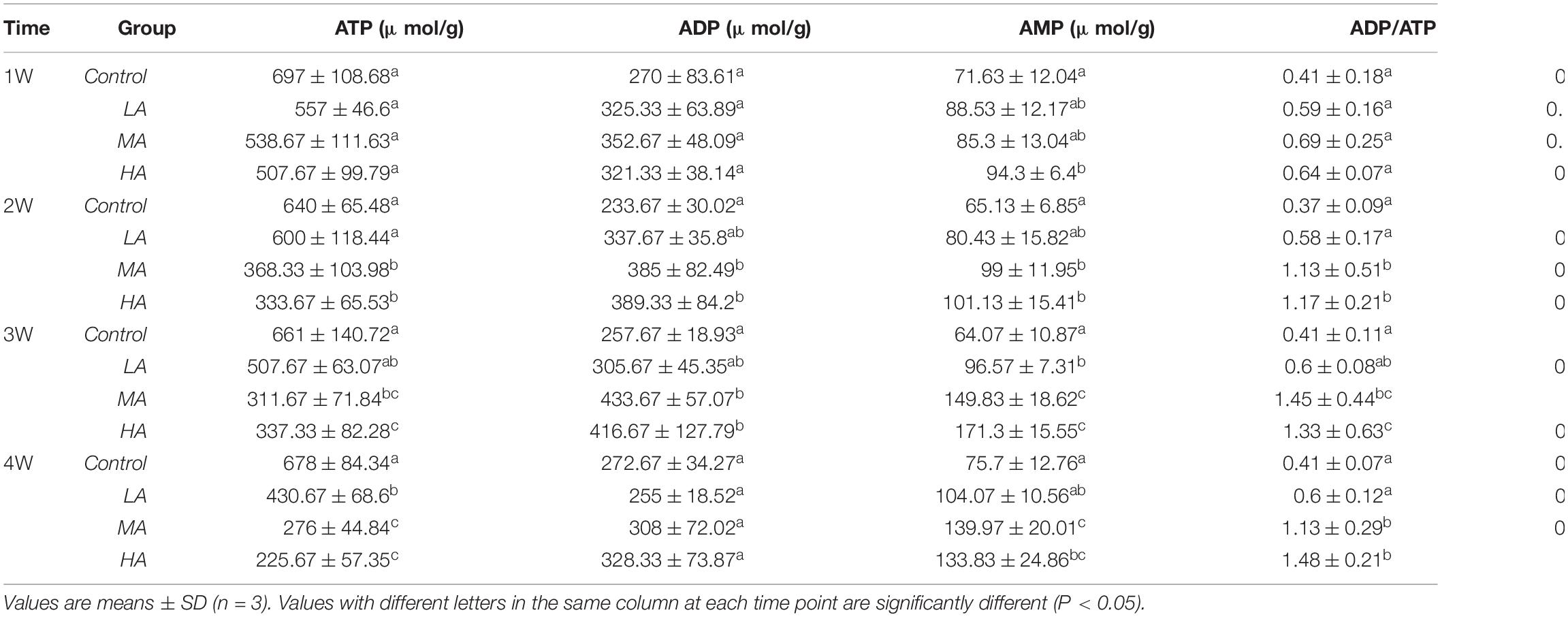
Table 2. Adenine nucleotides in the gills of P. trituberculatus in different groups during long-term ammonia stress.
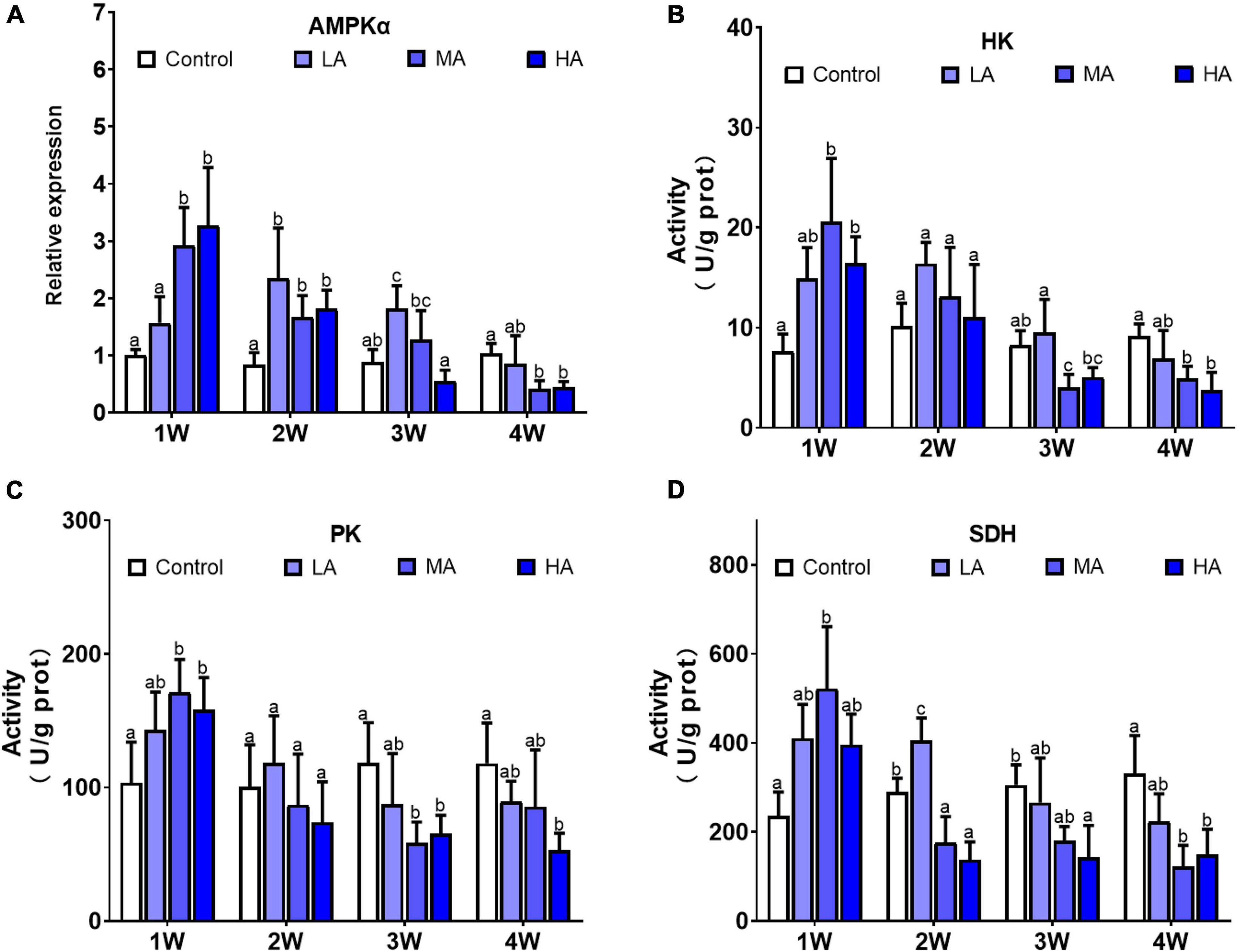
Figure 1. Expression of AMPKα (A) and activities of HK (B), PK (C), and SDH (D) in different treatments during long-term ammonia stress. Values are means ± S.D (n = 3). Different letters represent significant differences among different groups (P < 0.05).
Hemolymph Ammonia and Urea Contents
During the entire period of ammonia exposure, the levels of hemolymph ammonia of the three treatment groups remained lower than that of external medium, which indicated that the swimming crab has the capacity to excrete and detoxify ammonia under long-term ammonia (Table 3). However, the hemolymph ammonia contents in all the treatment groups showed an increasing trend as the exposure duration extended. Hemolymph ammonia concentrations in the MA and HA groups increased to 0.98 ± 0.28 mg l–1 and 1.96 ± 0.56 mg l–1 which were significantly higher than the control group (0.42 ± 0.14 mg l–1) at 1 week after exposure (P < 0.05), and further increased in a stepwise fashion during the remaining exposure period. Hemolymph ammonia in LA group increased gradually, and reached the level (P < 0.05) significantly higher than the control group at four week. Similarly, the hemolymph urea contents in MA and HA groups became significantly higher than that in the control at 1 week, and increased gradually afterward. Despite a slight increase at 4 week, no significant increase of urea in LA group was found.
Ammonia Excretion
Significant differences in expression of NKA, NHE, Rh, VAMP, and VAT were observed in the swimming crabs under the long-term ammonia exposure (P < 0.05), while no obvious change was found in NKCC expression (Figure 2). The expression of NKA, NHE, Rh, VAMP, and VAT showed a similar pattern during the exposure. For the MA and HA groups, their expression reached the highest levels at 1 or 2 week, and then decreased gradually to the levels comparable or lower than the control group. For the LA group, the expression of these genes rose with the exposure duration, and exhibited significant up-regulation at 2 or 3 week, and returned to the control level.
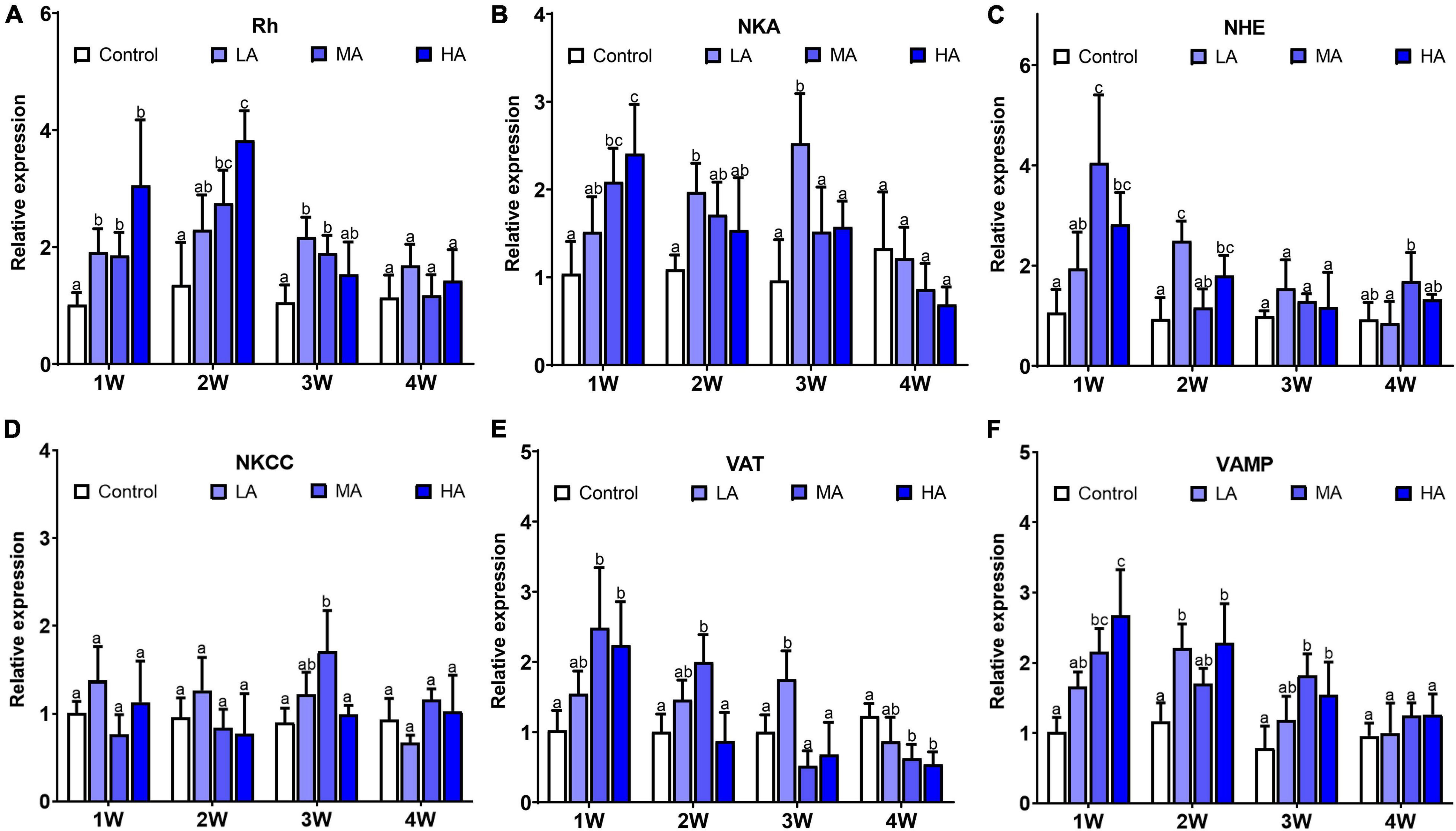
Figure 2. Expression of NKA (A), Rh (B), NHE (C), NKCC (D), VAT (E), and VA MP (F) in different treatments during long-term ammonia stress. Values are means ± S.D (n = 3). Different letters represent significant differences among different groups (P < 0.05).
Ammonia Detoxification
The activity of the ammonia detoxifying enzymes, including GDH, GS, and ARG, was significantly elevated after exposure in MA group and HA group for 1 week (P < 0.05), and then decreased to the control level at 2 (GS and ARG) and 3 week (GDH), respectively, and remained stable to the end of exposure (Figure 3). A similar pattern was also observed in the expression of urea transporter (UT). There was a significant up-regulation in UT expression in the MA and HA groups at 1 week (P < 0.05), followed by a sharp decrease at week 2. No significant difference in activity of GDH, GS, and ARG, and expression of UT was found in LA group during the exposure.
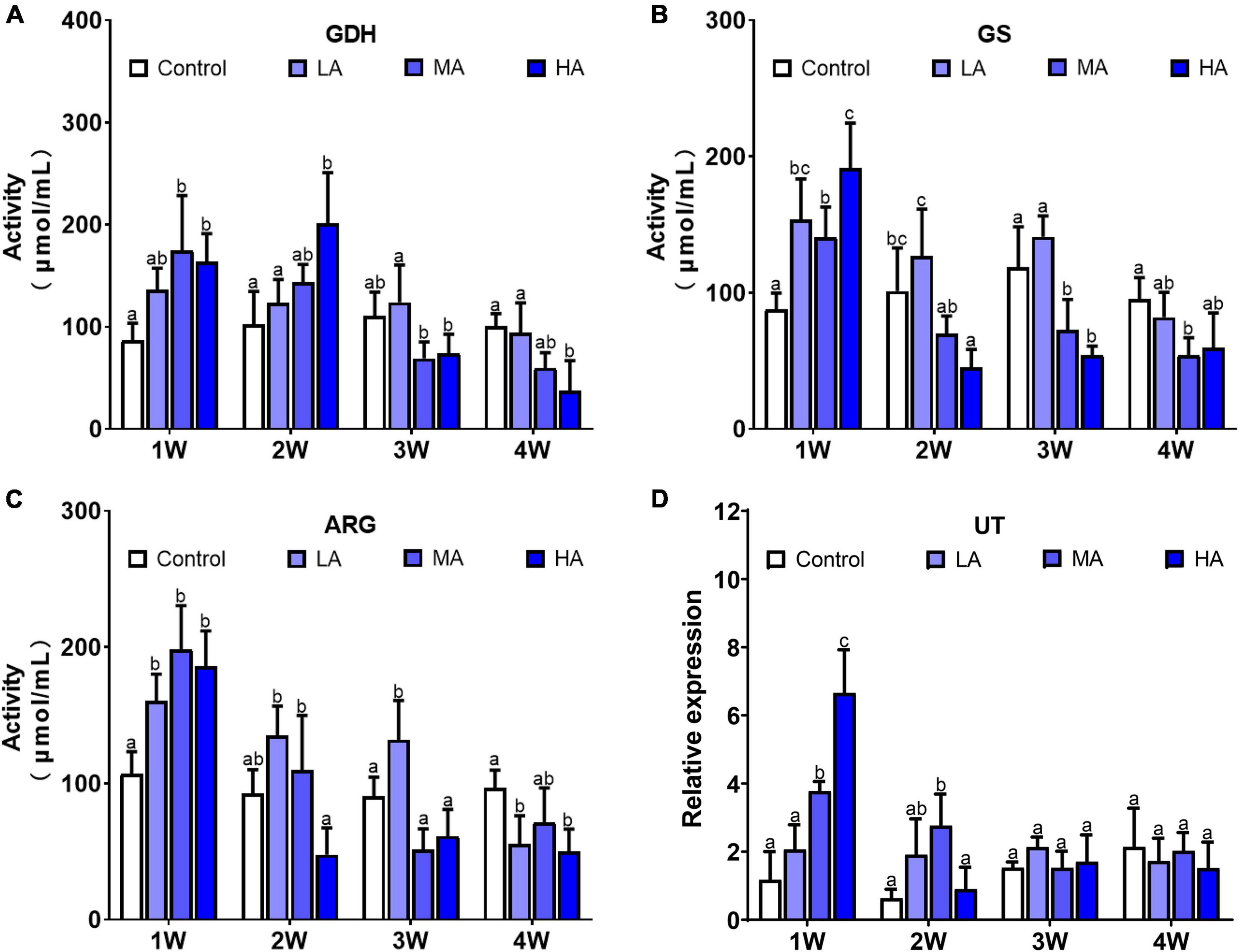
Figure 3. Activities of GDH (A), GS (B), ARG (C), and expression of UT (D) in different treatments during long-term ammonia stress. Values are means ± S.D (n = 3). Different letters represent significant differences among different groups (P < 0.05).
Antioxidant Defense and Oxidative Damage
The activity of the antioxidant enzymes were significantly altered during the long-term ammonia exposure (Figure 4). Compared with the control group, SOD activity in the MA and HA groups were constantly higher in the exposure period (P < 0.05), while CAT activity showed a gradual decrease after a slight increase at 1 week, and GPX activity decreased significantly at 4 week (P < 0.05). MDA levels in all the treatment groups exhibited an increasing trend as exposure time extended, and significantly elevated levels of MDA were observed in the MA and HA groups from 2 to 4 week (P < 0.05).
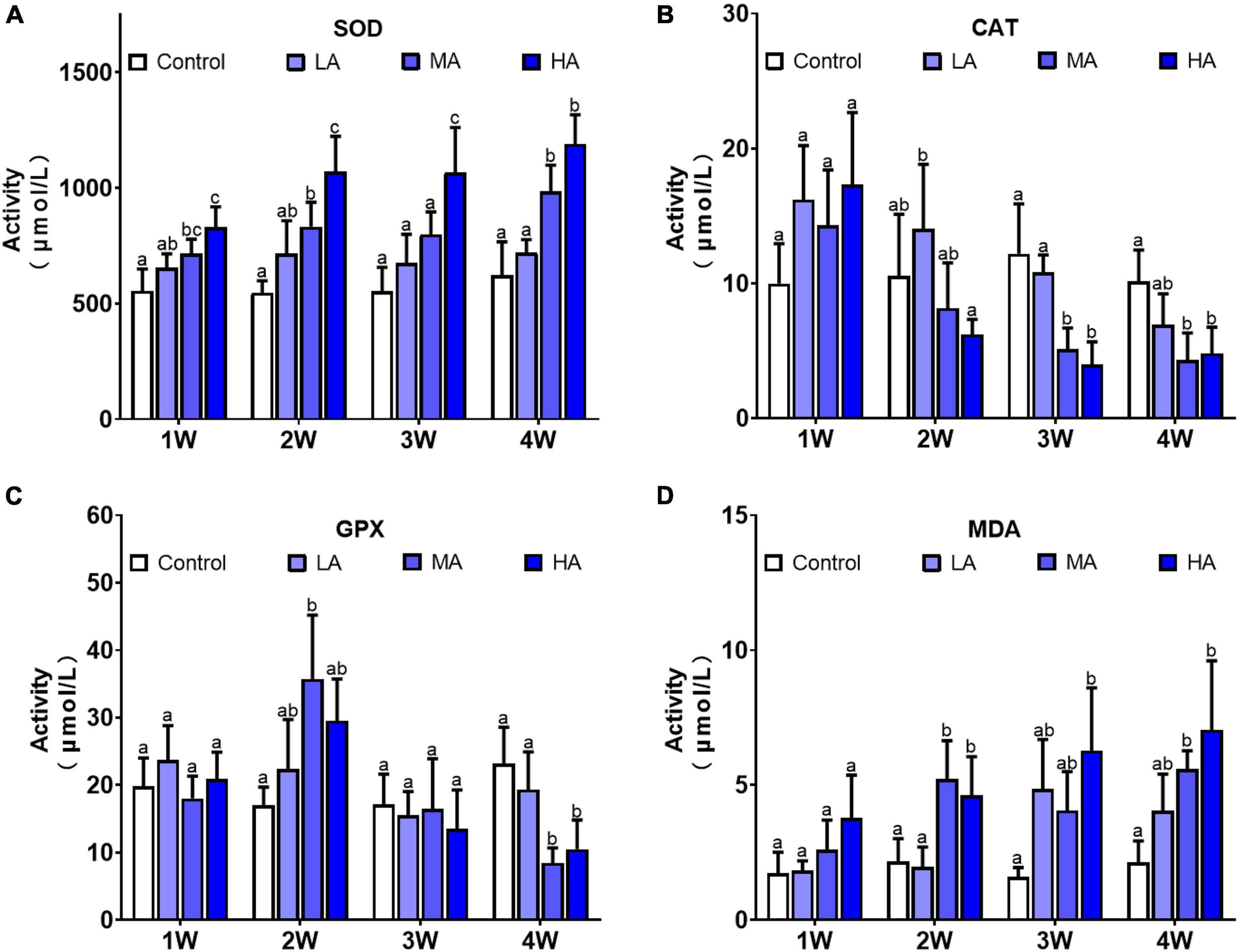
Figure 4. Activities of SOD (A), CAT (B), GPX (C), and levels of MDA (D) in different treatments during long-term ammonia stress. Values are means ± S.D (n = 3). Different letters represent significant differences among different groups (P < 0.05).
Endoplasmic Reticulum Stress and Unfolded Protein Response
Gene expression analysis showed that mRNA levels of Bip, IRE1, XBP1, ATF-4, and ATF-6 were significantly altered after ammonia exposure, while no significant difference was found in elF2α expression (Figure 5). Up-regulation of Bip, IRE1, XBP1, ATF-4, and ATF-6 was observed in the MA and HA groups from 1 to 3 week (P < 0.05). However, their expression decreased to the levels similar to (IRE1, XBP1, ATF-4, and ATF-6) or lower than (Bip) the control at 4 week post exposure.
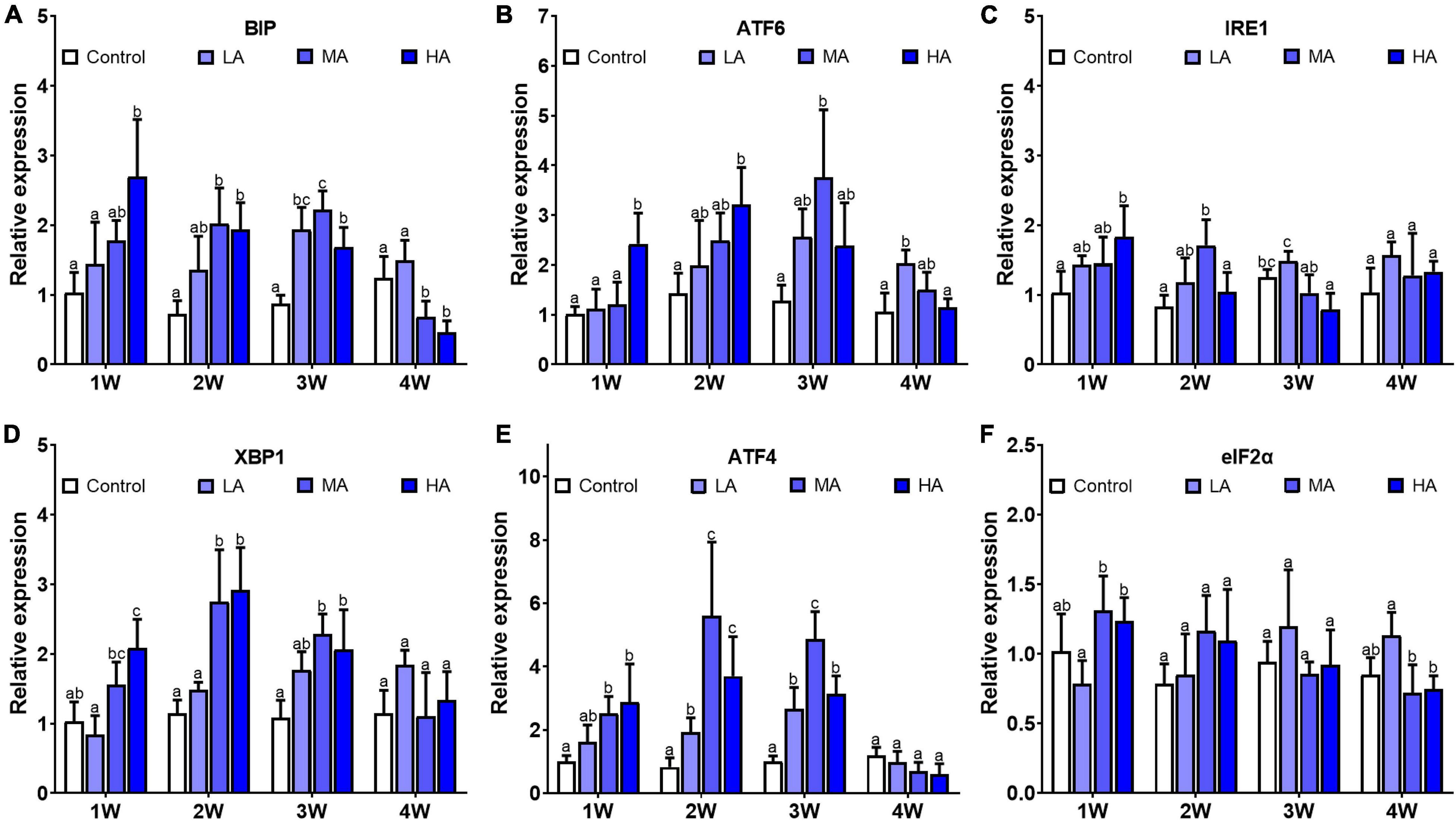
Figure 5. Expression of BIP (A), ATF-6 (B), IRE1 (C), XBP1 (D), ATF-4 (E), and eIF2α (F) in different treatments during long-term ammonia stress. Values are means ± S.D (n = 3). Different letters represent significant differences among different groups (P < 0.05).
Apoptosis
As shown in Figure 6, there was significant differences in expression of caspase-3, bax, and bcl-2 in all the treatment groups, while p53 only showed differential expression in MA group at week 2. Compared with the control group, caspase-3 and bax exhibited higher expression in the MA and HA groups from 1 week (P < 0.05). In contrast, expression of bcl-2 was lower in the MA and HA groups (P < 0.05). For the LA group, expression of caspase-3 increased significantly after 3 and 4 weeks of exposure (P < 0.05).
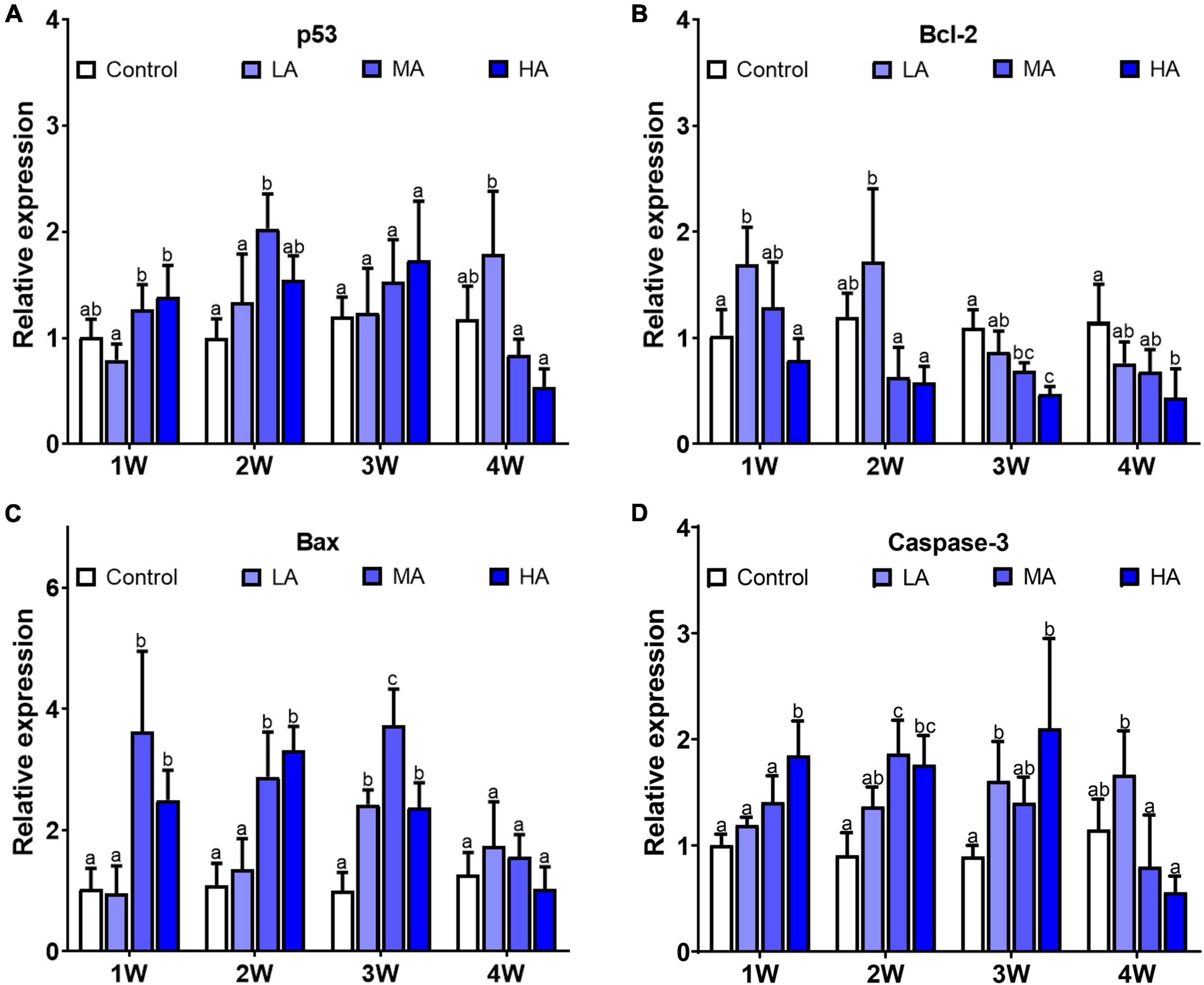
Figure 6. Expression of p53 (A), bcl-2 (B), bax (C), and caspase-3 (D) in different treatments during long-term ammonia stress. Values are means ± S.D (n = 3). Different letters represent significant differences among different groups (P < 0.05).
Discussion
Although many studies have been conducted on stress responses to acute ammonia stress in crustaceans, there has been limited information regarding their physiological and molecular responses under long-term exposure. In this study, we investigated alteration of energy metabolism, ammonia excretion and detoxification, antioxidant and UPR, and apoptosis in gills, the main excretory organ, of the swimming crab during prolonged ammonia exposure.
Energy Homeostasis
Energy metabolism plays a central role in restoring and maintaining cellular homeostasis under environmental stress (Sokolova et al., 2012), including ammonia stress (Sinha et al., 2012; Shan et al., 2019). Under ammonia exposure, energy demand associated with ammonia excretion and detoxification, and cellular stress responses may increase substantially. A recent study in Penaeus vannamei showed that acute ammonia exposure at 32.11 mg l–1 (∼half of LC50) resulted in significant reduction in ATP, the universal currency of free energy, and AEC, an indicator of cellular energy status, indicating that acute HEA perturbs energy homeostasis (Wang et al., 2021). In the present study, the levels of ATP and AEC in gills of all the treatment groups decreased significantly after 4-week exposure, indicating that long-term ammonia challenge, even at the concentration of 2 mg l–1 (LA group), can disrupt energy homeostasis in the gills of P. trituberculatus.
AMP-activated protein kinase (AMPK) is a key regulator of cellular energy balance (Hardie et al., 2012). Once activated by low energy status (AMP/ATP or ADP/ATP), AMPK initiates downstream signaling and switches on catabolic pathways such as glycolysis and oxidative metabolism, to enhance ATP production (Herzig and Shaw, 2018). It was reported that acute ammonia exposure stimulates AMPK signaling in gills of P. vannamei (Wang et al., 2021). Consistent with that result, significant upregulation of the expression of AMPKα, as well as activities of HK, PK, and SDH, were observed in MA and HA groups at 1 week post exposure, indicating that AMPK signaling was activated after a short-term ammonia exposure and thereby promotes glycolysis and oxidative phosphorylation, to meet increased energy requirement for defending against ammonia stress. However, as exposure time increase, there was a general trend of decline in AMPKα expression accompanied by decrease in activities of the metabolic enzymes in MA and HA groups, though the ATP levels in those groups remained lower than those in control group. These results suggest that long-term ammonia stress likely directly or indirectly inhibits AMPK signaling, that contributes to energy deprivation in gills of the swimming crab. Since most of the ammonia excretion and detoxification pathways, and cellular stress responses are energy-consuming, the energy imbalance is likely to have significant adverse consequences as outlined next.
Ammonia Excretion and Hemolymph Ammonia
Benthic species employ a variety of ammonia excretion pathways to survive the HEA (Weihrauch et al., 2009; Ip and Chew, 2010). In crustaceans, ammonia excretion is accomplished mainly at the gill epithelium through several transporters, such as Rh, NKA, VAT, and NHE, as well as a microtubule-dependent vesicular excretion mechanism (Weihrauch et al., 2002). Numerous investigations in decapods have shown that, under acute ammonia stress, these excretion pathways are activated to maintain hemolymph ammonia concentration (Si et al., 2018; Zhang X. et al., 2020). However, little is known about the alteration of ammonia excretion pathways and hemolymph ammonia during long-term ammonia stress. During the 4-week exposure period in this study, the levels of hemolymph ammonia in all the treatment groups were below those in ambient seawater, suggesting that active ammonia excretion continued to counteract ammonia influx. Nevertheless, hemolymph ammonia concentrations in all the exposure groups showed an increasing trend as exposure duration increased, and is potentially a result of gradually decreasing expression of ammonia excretion genes. Similar results were also observed in the Dungeness crab Metacarcinus magister (Martin et al., 2011). These results indicated that efficient branchial ammonia excretion can only last for a certain time period, and prolonged exposure depresses the excretion mechanisms, leading to accumulation of internal ammonia in the swimming crab.
It is noteworthy that the expression of NKA and VAT in MA and HA groups decreased to the control level earlier than the other genes involved in ammonia excretion. NKA and VAT are the major ATP-requiring participants in the ammonia excretion mechanisms (Weihrauch et al., 2002). The decrease of NKA and VAT expression coincided with the reduction in ATP contents. It is possible that the down-regulation of NKA and VAT is due to the disruption of energy homeostasis due to the prolonged ammonia exposure. Both NKA and VAT are key proteins in the transepithelial ammonia transport processes (Gonçalves et al., 2006; Henry et al., 2012). NKA, localized in basolateral membrane, transports ammonia from the hemolymph into the epithelial cell. VAT is distributed in cytoplasm and is critical in microtubule-dependent vesicular excretion through diffusion of NH3 into vesicles (Allen and Weihrauch, 2021). Considering their importance in the excretion, the depression expression of NKA and VAT may severely impair the ammonia excretion process in gills and is perhaps a result of declining overall cellular health.
Ammonia Detoxification and Hemolymph Urea
Aside from excreting ammonia directly, crustaceans have detoxification strategies via converting ammonia into other compounds to protect against HEA (Romano and Zeng, 2013). One of the detoxification strategies is conversion of ammonia to glutamate by GDH and then to glutamine by GS which can be stored in tissues and used as an oxidative substrate when returned to normal condition (Weihrauch et al., 1999; Hong et al., 2007). It is well-documented that this strategy is important for decapods to mitigate acute ammonia stress. In this study, both GDH and GS activities in MA and HA groups were significantly elevated at the initial stage, followed by a decrease to the levels comparable with or lower than the control group at later stages. This confirms that glutamine synthesis is important for detoxification under short-term ammonia, however, this detoxification pathway may be inhibited after long-term exposure. Although glutamine formation is considered to be an effective ammonia detoxification mechanism in crustaceans, it is a highly energy-consuming processes (Newsholme et al., 2003), where the conversion of glutamate and ammonia to glutamine, catalyzed by GS, is an ATP-dependent reaction. Results from the present study showed that GS activity decreased concurrently with the reduction of ATP. Moreover, correlation analysis revealed that GS activity was positively correlated with ATP level (Supplementary Figure 1). These results indicated that the depression of glutamine conversion after long-term exposure could be attributed to limited energy available.
It has been reported that converting ammonia into less toxic urea via ornithine-urea cycle (OUC) is an important pathway for ammonia detoxification in crustaceans (Liu et al., 2014; Geng et al., 2020). Similar to the detoxification via glutamate synthesis, urea production from ammonia is also energetically costly, and it requires at least 2 mol of ATP for synthesizing 1 mol urea (Wood, 1993). In the present study, the activity of ARG, a key enzyme in OUC, shared a similar temporal pattern with that of GS, and decreased paralleled with ATP content. This further indicate that the depressed energy-consuming detoxification pathways in gills after prolonged exposure is likely due to the lack of ATP. Despite the reduction of ARG activity in gill after long-term exposure, the level of hemolymph urea increased significantly. This might be related to increased urea production in hepatopancreas, and many studies have reported that hepatopancreas is another organ for converting ammonia into urea (Pan et al., 2018). Therefore, another possibility is that the swimming crabs were unable to excrete urea efficiently as evidenced by no increment in expression of UT, the specific transporter that facilitates urea transport across the gills (You et al., 1993). Urea accumulation without increased excretion capacity was also observed in largemouth bass, and it was speculated that it may be a strategy to avoid the loss of metabolic carbon fuel (Egnew et al., 2019). On the other hand, urea is toxic, though less than ammonia (Randall and Tsui, 2002). To date, its exact toxicity in crustacean is still unclear. Further work is required to understand the physiological consequences of urea accumulation in the swimming crab under ammonia stress.
Oxidative Stress, Endoplasmic Reticulum Stress, and Apoptosis
A number of studies in decapods have reported that acute ammonia stress can induce reactive oxygen species (ROS) generation, leading to oxidative damage to biomolecules including lipids (Zhang et al., 2015; Liang et al., 2016). Our data showed that MDA, an index of lipid peroxidation, accumulated in gills in a time- and dose-dependent manner following long term ammonia exposure. The build-up of MDA is probably associated with the alteration of antioxidant defense system. SOD, CAT, and GPx are key enzymes in antioxidant system, and represents the first line of defense against oxidative stress (McCord, 2000). For MA and HA group, a significant increase in SOD activity was observed after exposure, while CAT and GPx activities showed a gradual decrease during the exposure. SOD catalyzes the dismutation of superoxide ions (O2–) to hydrogen peroxide (H2O2), and CAT and GPx convert H2O2 to water (Xie et al., 2016). Increment of SOD activity can result in elevated levels of H2O2, and reduction of CAT and GPx activity can lead to accumulation of H2O2, and then this build-up of H2O2 can oxidatively damaged lipids and other biomolecules (Magdalan et al., 2011; Meng et al., 2014).
Oxidative stress is usually coupled with ER stress during which protein folding is overwhelmed, and leads to accumulation of unfold and misfold proteins in ER (Todd et al., 2008). To restore ER homeostasis, cells activate several signaling pathways, collectively called the unfolded protein response (UPR), aiding protein folding and eliminating terminally misfolded protein (Hetz, 2012). Results of this study showed that Bip, the master regulator of UPR, was significantly upregulated in all the treatment groups, indicating that long-term ammonia exposure can cause ER stress and induce UPR in the swimming crab. The activation of the UPR involves three signaling pathways, including PERK/ATF4, IRE1/XBP1 and ATF6 (Kim et al., 2006). Previous studies in Litopenaeus vannamei showed that short-term HEA (20∼42 mg l–1) can activate PERK/ATF4 and IRE1/XBP1 pathways in hepatopancreas tissue (Liang et al., 2016; Wang et al., 2020). In this study, upregulation of ATF4, ATF6, IRE1, and XBP1 indicated that all the three UPR signaling pathways were initiated to alleviate ER stress in gills after ammonia stress. Notably, the expression of ATF4, ATF6, IRE1, and XBP1 in MA and HA groups decreased, accompanied by Bip, to the levels similar to the control group at 4 week. This result suggested that prolonged ammonia exposure can compromise UPR signaling, which may lead to aggravation of ER stress and cell death (Sano and Reed, 2013).
It is well-documented that both oxidative stress and ER stress can interfere with cell function and activate pro-apoptotic signaling (Chandra et al., 2000; Tabas and Ron, 2011). Moreover, oxidative stress and ER stress could accentuate each other in a positive feedback loop, which further perturbs cellular homeostasis and elicit apoptotic cell death (Liu X. et al., 2020). Expression analysis revealed that caspase-3, the executioner of apoptosis, was significantly up-regulated in all the treatment groups, indicating that long-term ammonia stress, even at the concentration as low as 2 mg l–1 (LA group), can induce apoptosis in gills of the swimming crab. Recent studies in fish showed that ammonia induced apoptosis in hepatopancreas via p53-Bax signaling pathway (Cheng et al., 2015; Jia et al., 2015). In this pathway, activation of p53 down-regulates anti-apoptotic genes, such as bcl-2, and up-regulates pro-apoptotic genes including the bax, which causes activation of caspases and eventually apoptosis (Schuler and Green, 2001). In the present study, enhanced bax expression and reduced bcl-2 expression were observed after ammonia exposure. However, there was only slight increase in p53 expression, which is possibly because p53 is activated through post-translational modifications (Xu, 2003). Collectively, our results indicate that ammonia-induces apoptosis in gills of P. trituberculatus likely via the p53-Bax pathway.
Conclusion
In summary, this study investigated stress responses in the gills of the swimming crab P. trituberculatus following long-term ammonia stress over a temporal scale (Figure 7). The results revealed that prolonged ammonia impaired energy homeostasis possibly through inhibiting AMPK signaling, and depressed ammonia excretion and detoxification machinery. This likely leads to hemolymph ammonia accumulation, which was, at least in part, due to the mismatch in the increased energy demand and reduced ATP generation. Furthermore, the long-term exposure resulted in oxidative damage and ER stress, and elicited apoptosis in gills of the crabs, even for those at low ammonia concentration (2 mg l–1). The findings of the present study further our understanding of detrimental effects and mechanisms of long-term ammonia in swimming crabs, and provide valuable information for aquaculture management.
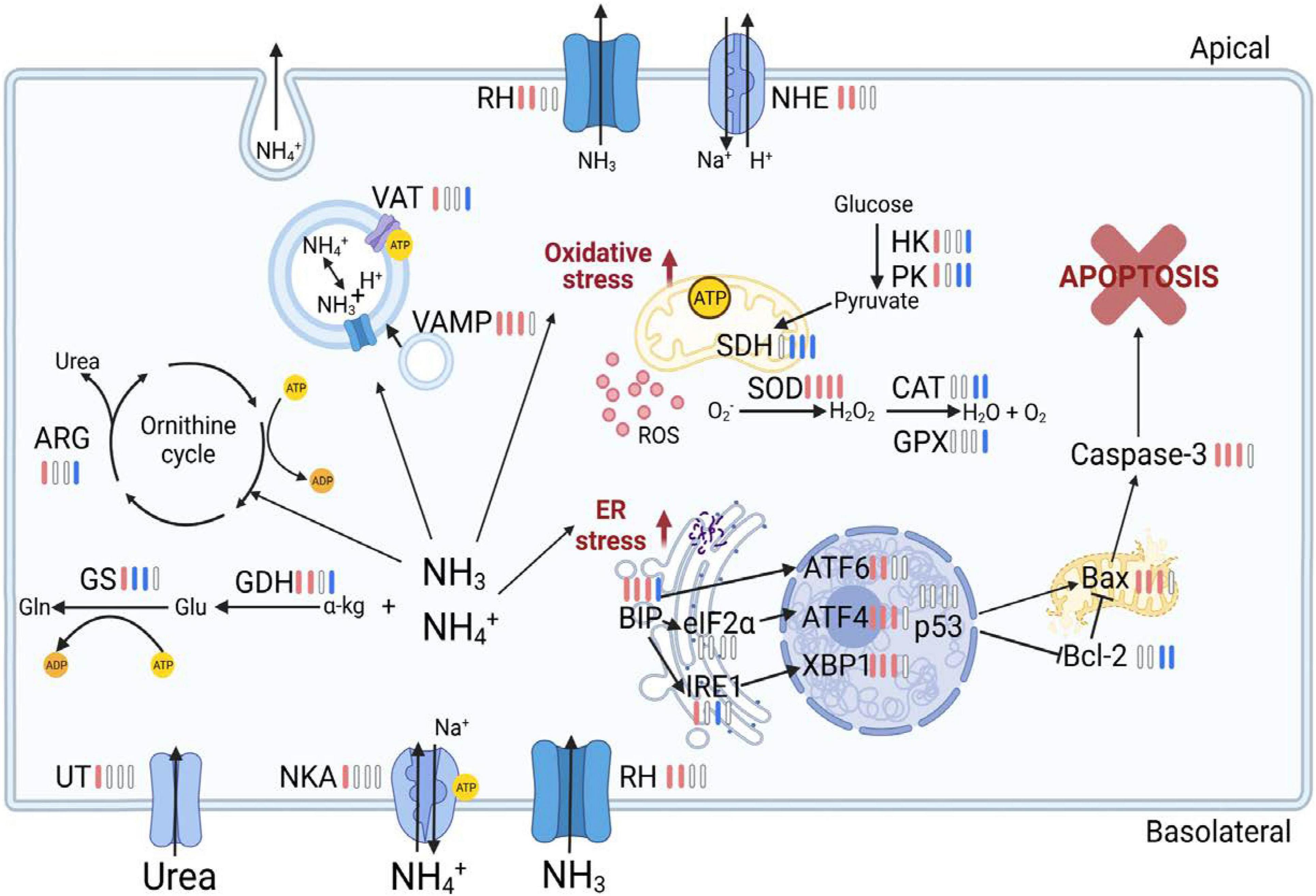
Figure 7. Overview of physiological and molecular responses in the gill of the swimming crab Portunus trituberculatus during long-term ammonia stress. The different colors of the bars indicate the changes of physiological parameters and gene expression in HA group (8 mg l–1). Red bars represent significant up-regulation, blue bars represent significant down-regulation and white bars represent no significant difference, compared with the control group. The figure is created in BioRender.
Data Availability Statement
The original contributions presented in the study are included in the article/Supplementary Material, further inquiries can be directed to the corresponding author/s.
Author Contributions
JZ and XM: conceptualization. JZ and MZ: methodology and investigation. XM, JZ, XR, and BG: software, validation, formal analysis, data curation, and original draft. NJ, JZ, and XM: writing, review, and editing. PL and JL: resources, supervision, and project administration. XM: funding acquisition. All authors contributed to the article and approved the submitted version.
Funding
This research was funded by the National Key Research and Development Plan (2019YFD0900402), the Qingdao Shinan District Science and Technology Program (2020-2-001-QT), the Central Public-interest Scientific Institution Basal Research Fund, CAFS (2020TD46), and the China Agriculture Research System of MOF and MARA.
Conflict of Interest
The authors declare that the research was conducted in the absence of any commercial or financial relationships that could be construed as a potential conflict of interest.
Publisher’s Note
All claims expressed in this article are solely those of the authors and do not necessarily represent those of their affiliated organizations, or those of the publisher, the editors and the reviewers. Any product that may be evaluated in this article, or claim that may be made by its manufacturer, is not guaranteed or endorsed by the publisher.
Supplementary Material
The Supplementary Material for this article can be found online at: https://www.frontiersin.org/articles/10.3389/fmars.2021.797241/full#supplementary-material
References
Ackerman, P. A., Wicks, B. J., Iwama, G. K., and Randall, D. J. (2006). Low levels of environmental ammonia increase susceptibility to disease in Chinook salmon smolts. Physiol. Biochem. Zool. 79, 695–707. doi: 10.1086/504615
Allen, G. J. P., and Weihrauch, D. (2021). Exploring the versatility of the perfused crustacean gill as a model for transbranchial transport processes. Comp. Biochem. Physiol. B. 254:110572. doi: 10.1016/j.cbpb.2021.110572
Benli, A. ÇK., Köksal, G., and Özkul, A. (2008). Sublethal ammonia exposure of Nile tilapia (Oreochromis niloticus L.): effects on gill, liver and kidney histology. Chemosphere 72, 1355–1358. doi: 10.1016/j.chemosphere.2008.04.037
Brinkman, S. F., Woodling, J. D., Vajda, A. M., and Norris, D. O. (2009). Chronic toxicity of ammonia to early life stage rainbow trout. Trans. Am. Fish. Soc. 138, 433–440. doi: 10.1577/T07-224.1
Chandra, J., Samali, A., and Orrenius, S. (2000). Triggering and modulation of apoptosis by oxidative stress. Free. Radical. Biol. Med. 29, 323–333. doi: 10.1016/S0891-5849(00)00302-6
Cheng, C. H., Yang, F. F., Ling, R. Z., Liao, S. A., Miao, Y. T., Ye, C. X., et al. (2015). Effects of ammonia exposure on apoptosis, oxidative stress and immune response in pufferfish (Takifugu obscurus). Aquat. Toxicol. 164, 61–71. doi: 10.1016/j.aquatox.2015.04.004
China Agriculture Press (2020). China Fishery Statistical Yearbook. Beijing: China Agriculture Press, 24–34.
Ching, B., Chew, S. F., Wong, W. P., and Ip, Y. K. (2009). Environmental ammonia exposure induces oxidative stress in gills and brain of Boleophthalmus boddarti (mudskipper). Aquat.Toxicol. 95, 203–212. doi: 10.1016/j.aquatox.2009.09.004
Cui, Y., Ren, X., Li, J., Zhai, Q., Feng, Y., Xu, Y., et al. (2017). Effects of ammonia-N stress on metabolic and immune function via the neuroendocrine system in Litopenaeus vannamei. Fish. Shellfish Immunol. 64, 270–275. doi: 10.1016/j.fsi.2017.03.028
Egnew, N., Renukdas, N., Ramena, Y., Yadav, A. K., Kelly, A. M., Lochmann, R. T., et al. (2019). Physiological insights into largemouth bass (Micropterus salmoides) survival during long-term exposure to high environmental ammonia. Aquat. Toxicol. 207, 72–82. doi: 10.1016/j.aquatox.2018.11.027
Foss, A., Imsland, A. K., Roth, B., Schram, E., and Stefansson, S. O. (2009). Effects of chronic and periodic exposure to ammonia on growth and blood physiology in juvenile turbot (Scophthalmus maximus). Aquaculture 296, 45–50. doi: 10.1016/j.aquaculture.2009.07.013
Geng, Z., Liu, Q., Wang, T., Ma, S., and Shan, H. (2020). Changes in physiological parameters involved in glutamine and urea synthesis in Pacific white shrimp, Litopenaeus vannamei, fed Ampithoe sp. meal and exposed to ammonia stress. Aquac. Res. 51, 2725–2734. doi: 10.1111/are.14611
Gonçalves, R. R., Masui, D. C., McNamara, J. C., Mantelatto, F. L., Garçon, D. P., Furriel, R. P., et al. (2006). A kinetic study of the gill (Na+, K+)-ATPase, and its role in ammonia excretion in the intertidal hermit crab, Clibanarius vittatus. Comp. Biochem. Physiol. A 145, 346–356. doi: 10.1016/j.cbpa.2006.07.007
Hardie, D. G., Ross, F. A., and Hawley, S. A. (2012). AMPK: a nutrient and energy sensor that maintains energy homeostasis. Nat. Rev. Mol. Cell. Biol. 13, 251–262. doi: 10.1038/nrm3311
Henry, R. P., Lucu, C., Onken, H., and Weihrauch, D. (2012). Multiple functions of the crustacean gill: osmotic/ionic regulation, acid-base balance, ammonia excretion, and bioaccumulation of toxic metals. Front. Physiol. 3:431. doi: 10.3389/fphys.2012.00431
Henry, Y., Piscart, C., Charles, S., and Colinet, H. (2017). Combined effect of temperature and ammonia on molecular response and survival of the freshwater crustacean Gammarus pulex. Ecotoxicol. Environ. Saf. 137, 42–48. doi: 10.1016/j.ecoenv.2016.11.011
Herzig, S., and Shaw, R. J. (2018). AMPK: guardian of metabolism and mitochondrial homeostasis. Nat. Rev. Mol. Cell. Biol. 19, 121–135. doi: 10.1038/nrm.2017.95
Hetz, C. (2012). The unfolded protein response: controlling cell fate decisions under ER stress and beyond. Nat. Rev. Mol. Cell. Biol. 13, 89–102. doi: 10.1038/nrm3270
Hong, M., Chen, L., Sun, X., Gu, S., Zhang, L., and Chen, Y. (2007). Metabolic and immune responses in Chinese mitten-handed crab (Eriocheir sinensis) juveniles exposed to elevated ambient ammonia. Comp. Biochem. Physiol. C 145, 363–369. doi: 10.1016/j.cbpc.2007.01.003
Ip, Y. K., and Chew, S. F. (2010). Ammonia production, excretion, toxicity, and defense in fish: a review. Front. Physiol. 1:134. doi: 10.3389/fphys.2010.00134
Ip, Y., Chew, S., and Randall, D. J. (2001). Ammonia toxicity, tolerance, and excretion. Fish. Physiol. 20, 109–148. doi: 10.1016/S1546-5098(01)20005-3
Jia, R., Han, C., Lei, J. L., Liu, B. L., Huang, B., Huo, H. H., et al. (2015). Effects of nitrite exposure on haematological parameters, oxidative stress and apoptosis in juvenile turbot (Scophthalmus maximus). Aquat.Toxicol. 169, 1–9. doi: 10.1016/j.aquatox.2015.09.016
Kim, R., Emi, M., Tanabe, K., and Murakami, S. (2006). Role of the unfolded protein response in cell death. Apoptosis 11, 5–13. doi: 10.1007/s10495-005-3088-0
Lemarié, G., Dosdat, A., Covès, D., Dutto, G., Gasset, E., and Person-Le Ruyet, J. (2004). Effect of chronic ammonia exposure on growth of European seabass (Dicentrarchus labrax) juveniles. Aquaculture 229, 479–491. doi: 10.1016/s0044-8486(03)00392-2
Liang, C., Liu, J., Cao, F., Li, Z., and Chen, T. (2019). Transcriptomic analyses of the acute ammonia stress response in the hepatopancreas of the kuruma shrimp (Marsupenaeus japonicus). Aquaculture 513:734328. doi: 10.1016/j.aquaculture.2019.734328
Liang, Z., Liu, R., Zhao, D., Wang, L., Sun, M., Wang, M., et al. (2016). Ammonia exposure induces oxidative stress, endoplasmic reticulum stress and apoptosis in hepatopancreas of pacific white shrimp (Litopenaeus vannamei). Fish. Shellfish Immunol. 54, 523–528. doi: 10.1016/j.fsi.2016.05.009
Liu, F., Li, S., Yu, Y., Sun, M., Xiang, J., and Li, F. (2020). Effects of ammonia stress on the hemocytes of the Pacific white shrimp Litopenaeus vannamei. Chemosphere 239:124759. doi: 10.1016/j.chemosphere.2019.124759
Liu, S., Pan, L., Liu, M., and Yang, L. (2014). Effects of ammonia exposure on nitrogen metabolism in gills and hemolymph of the swimming crab Portunus trituberculatus. Aquaculture 432, 351–359. doi: 10.1016/j.aquaculture.2014.05.029
Liu, X., Zhang, E., Yin, S., Zhao, C., Fan, L., and Hu, H. (2020). Activation of the IRE1α arm, but not the PERK arm, of the unfolded protein response contributes to fumonisin B1-induced hepatotoxicity. Toxins 12:55. doi: 10.3390/toxins12010055
Livak, K., and Schmittgen, T. (2001). Analysis of relative gene expression data using real-time quantitative PCR and the 2 (-MC (T)) Method. Methods 25, 402–408. doi: 10.1006/meth.2001.1262
Lu, Y., Wang, F., and Dong, S. (2015). Energy response of swimming crab Portunus trituberculatus to thermal variation: implication for crab transport method. Aquaculture 441, 64–71. doi: 10.1016/j.aquaculture.2015.02.022
Lucu, Č, Devescovi, M., and Siebers, D. J. (1989). Do amiloride and ouabain affect ammonia fluxes in perfused Carcinus gill epithelia? J. Exp. Zool. 249, 1–5. doi: 10.1002/jez.1402490102
Magdalan, J., Piotrowska, A., Gomułkiewicz, A., Sozański, T., Szeląg, A., and Dziegiêl, P. (2011). Influence of commonly used clinical antidotes on antioxidant systems in human hepatocyte culture intoxicated with α-amanitin. Hum. Exp. Toxicol. 30, 38–43. doi: 10.1177/0960327110368418
Martin, M., Fehsenfeld, S., Sourial, M. M., and Weihrauch, D. (2011). Effects of high environmental ammonia on branchial ammonia excretion rates and tissue Rh-protein mRNA expression levels in seawater acclimated Dungeness crab Metacarcinus magister. Comp. Biochem. Physiol. A 160, 267–277. doi: 10.1016/j.cbpa.2011.06.012
McCord, J. M. (2000). The evolution of free radicals and oxidative stress. Am. J. Med. 108, 652–659. doi: 10.1016/S0002-9343(00)00412-5
Meng, X. L., Liu, P., Li, J., Gao, B. Q., and Chen, P. (2014). Physiological responses of swimming crab Portunus trituberculatus under cold acclimation: antioxidant defense and heat shock proteins. Aquaculture 434, 11–17. doi: 10.1016/j.aquaculture.2014.07.021
Murray, B. W., Busby, E. R., Mommsen, T. P., and Wright, P. A. (2003). Evolution of glutamine synthetase in vertebrates: multiple glutamine synthetase genes expressed in rainbow trout (Oncorhynchus mykiss). J. Exp. Biol. 206, 1511–1521. doi: 10.1242/jeb.00283
Newsholme, P., Procopio, J., Lima, M. M. R., Pithon-Curi, T. C., and Curi, R. (2003). Glutamine and glutamate-their central role in cell metabolism and function. Cell. Biochem. Funct. 21, 1–9. doi: 10.1002/cbf.1003
Pan, L., Si, L., Liu, S., Liu, M., and Wang, G. (2018). Levels of metabolic enzymes and nitrogenous compounds in the swimming crab Portunus trituberculatus exposed to elevated ambient ammonia-N. J. Ocean. Univ. China 17, 957–966. doi: 10.1007/s11802-018-3574-y
Racotta, I. S., and Herna′ndez-Herrera, R. (2000). Metabolic responses of the white shrimp, Penaeus vannamei, to ambient ammonia. Comp. Biochem. Physiol. A 125, 437–443. doi: 10.1016/S1095-6433(00)00171-9
Ren, Q., Pan, L., Zhao, Q., and Si, L. (2015). Ammonia and urea excretion in the swimming crab Portunus trituberculatus exposed to elevated ambient ammonia-N. Comp. Biochem. Physiol. A 187, 48–54. doi: 10.1016/j.cbpa.2015.04.013
Romano, N., and Zeng, C. (2007). Ontogenetic changes in tolerance to acute ammonia exposure and associated gill histological alterations during early juvenile development of the blue swimmer crab, Portunus pelagicus. Aquaculture 266, 246–254. doi: 10.1016/j.aquaculture.2007.01.035
Romano, N., and Zeng, C. (2010). Survival, osmoregulation and ammonia-N excretion of blue swimmer crab, Portunus pelagicus, juveniles exposed to different ammonia-N and salinity combinations. Comp. Biochem. Physiol. C 151, 222–228. doi: 10.1016/j.cbpc.2009.10.011
Romano, N., and Zeng, C. (2013). Toxic effects of ammonia, nitrite, and nitrate to decapod crustaceans: a review on factors influencing their toxicity, physiological consequences, and coping mechanisms. Rev. Fish. Sci. 21, 1–21. doi: 10.1080/10641262.2012.753404
Sano, R., and Reed, J. C. (2013). ER stress-induced cell death mechanisms. Biochim. Biophys. Acta Mol. Cell. Res. 1833, 3460–3470. doi: 10.1016/j.bbamcr.2013.06.028
Schuler, M., and Green, D. (2001). Mechanisms of p53-dependent apoptosis. Biochem. Soc. Trans. 29, 684–688. doi: 10.1042/bst0290684
Shan, H., Geng, Z., Ma, S., and Wang, T. (2019). Comparative study of the key enzymes and biochemical substances involved in the energy metabolism of Pacific white shrimp, Litopenaeus vannamei, with different ammonia-N tolerances. Comp. Biochem. Physiol. C 221, 73–81. doi: 10.1016/j.cbpc.2019.04.001
Si, L., Pan, L., Wang, H., and Zhang, X. (2018). Identification of the role of Rh protein in ammonia excretion of the swimming crab Portunus trituberculatus. J. Exp. Biol. 221, jeb184655. doi: 10.1242/jeb.184655
Sinha, A. K., Liew, H. J., Diricx, M., Blust, R., and De Boeck, G. (2012). The interactive effects of ammonia exposure, nutritional status and exercise on metabolic and physiological responses in gold fish (Carassius auratus L.). Aquat. Toxicol. 109, 33–46. doi: 10.1016/j.aquatox.2011.11.002
Sokolova, I. M., Frederich, M., Bagwe, R., Lannig, G., and Sukhotin, A. A. (2012). Energy homeostasis as an integrative tool for assessing limits of environmental stress tolerance in aquatic invertebrates. Mar. Environ. Res. 79, 1–15. doi: 10.1016/j.marenvres.2012.04.003
Tabas, I., and Ron, D. (2011). Integrating the mechanisms of apoptosis induced by endoplasmic reticulum stress. Nat. Cell. Biol. 13, 184–190. doi: 10.1038/ncb0311-184
Todd, D. J., Lee, A. H., and Glimcher, L. H. (2008). The endoplasmic reticulum stress response in immunity and autoimmunity. Nat. Rev. Immunol. 8, 663–674. doi: 10.1038/nri2359
Wang, T., Li, W., Shan, H., and Ma, S. (2021). Responses of energy homeostasis and lipid metabolism in Penaeus vannamei exposed to ammonia stress. Aquaculture 544:737092. doi: 10.1016/j.aquaculture.2021.737092
Wang, T., Shan, H. W., Geng, Z. X., Yu, P., and Ma, S. (2020). Dietary supplementation with freeze-dried Ampithoe sp. enhances the ammonia-N tolerance of Litopenaeus vannamei by reducing oxidative stress and endoplasmic reticulum stress and regulating lipid metabolism. Aquacult. Rep. 16:100264. doi: 10.1016/j.aqrep.2019.100264
Weihrauch, D., and Allen, G. J. J. (2018). Ammonia excretion in aquatic invertebrates: new insights and questions. J. Exp. Biol. 221:jeb169219. doi: 10.1242/jeb.169219
Weihrauch, D., Becker, W., Postel, U., Luck-Kopp, S., and Siebers, D. (1999). Potential of active excretion of ammonia in three different haline species of crabs. J. Comp. Physiol. 169, 25–37. doi: 10.1007/s003600050190
Weihrauch, D., Fehsenfeld, S., and Quijada-Rodriguez, A. (2017). “Nitrogen excretion in aquatic crustaceans,” in Acid-Base Balance and Nitrogen Excretion in Invertebrates, eds D. Weihrauch and M. O’Donnell (Cham: Springer), 1–24. doi: 10.1007/978-3-319-39617-0_1
Weihrauch, D., Morris, S., and Towle, D. W. (2004). Ammonia excretion in aquatic and terrestrial crabs. J. Exp. Biol. 207, 4491–4504. doi: 10.1242/jeb.01308
Weihrauch, D., Wilkie, M. P., and Walsh, P. J. (2009). Ammonia and urea transporters in gills of fish and aquatic crustaceans. J. Exp. Biol. 212, 1716–1730. doi: 10.1242/jeb.024851
Weihrauch, D., Ziegler, A., Siebers, D., and Towle, D. W. (2002). Active ammonia excretion across the gills of the green shore crab Carcinus maenas: participation of Na+/K+-ATPase, V-type H+-ATPase and functional microtubules. J. Exp. Biol. 205, 2765–2775. doi: 10.1242/jeb.205.18.2765
Wood, C. M. (1993). “Ammonia and urea metabolism and excretion,” in The Physiology of Fishes, ed. D. H. Evans (Boca Raton: CRC Press), 379–425.
Xie, Z. Z., Liu, Y., and Bian, J. S. (2016). Hydrogen sulfide and cellular redox homeostasis. Oxid. Med. Cell. Longev. 2016:6043038. doi: 10.1155/2016/6043038
Xu, Y. (2003). Regulation of p53 responses by post-translational modifications. Cell. Death Differ. 10, 400–403. doi: 10.1038/sj.cdd.4401182
You, G., Smith, C. P., Kanai, Y., Lee, W. S., Stelzner, M., and Hediger, M. A. (1993). Cloning and characterization of the vasopressin-regulated urea transporter. Nature 365, 844–847. doi: 10.1038/365844a0
Zhang, T., Yan, Z., Zheng, X., Wang, S., Fan, J., and Liu, Z. (2020). Effects of acute ammonia toxicity on oxidative stress, DNA damage and apoptosis in digestive gland and gill of Asian clam (Corbicula fluminea). Fish Shellfish Immunol. 99, 514–525. doi: 10.1016/j.fsi.2020.02.046
Zhang, W., Jiang, Q., Liu, X., Pan, D., Yang, Y., and Yang, J. (2015). The effects of acute ammonia exposure on the immune response of juvenile freshwater prawn, Macrobrachium nipponense. J. Crustacean. Biol. 35, 76–80. doi: 10.1163/1937240X-00002292
Zhang, W., Li, J., Chen, Y., Si, Q., Tian, J., Jiang, Q., et al. (2019). Exposure time relevance of response to nitrite exposure: insight from transcriptional responses of immune and antioxidant defense in the crayfish, Procambarus clarkii. Aquat. Toxicol. 214:105262. doi: 10.1016/j.aquatox.2019.105262
Zhang, X., Pan, L., Wei, C., Tong, R., Li, Y., Ding, M., et al. (2020). Crustacean hyperglycemic hormone (CHH) regulates the ammonia excretion and metabolism in white shrimp, Litopenaeus vannamei under ammonia-N stress. Sci. Total Environ. 723:138128. doi: 10.1016/j.scitotenv.2020.138128
Keywords: long-term, ammonia, crab, gill, physiology
Citation: Zhang J, Zhang M, Jayasundara N, Ren X, Gao B, Liu P, Li J and Meng X (2021) Physiological and Molecular Responses in the Gill of the Swimming Crab Portunus trituberculatus During Long-Term Ammonia Stress. Front. Mar. Sci. 8:797241. doi: 10.3389/fmars.2021.797241
Received: 18 October 2021; Accepted: 18 November 2021;
Published: 09 December 2021.
Edited by:
Yangfang Ye, Ningbo University, ChinaReviewed by:
Hongbo Jiang, Shenyang Agricultural University, ChinaBin Xia, Qingdao Agricultural University, China
Copyright © 2021 Zhang, Zhang, Jayasundara, Ren, Gao, Liu, Li and Meng. This is an open-access article distributed under the terms of the Creative Commons Attribution License (CC BY). The use, distribution or reproduction in other forums is permitted, provided the original author(s) and the copyright owner(s) are credited and that the original publication in this journal is cited, in accordance with accepted academic practice. No use, distribution or reproduction is permitted which does not comply with these terms.
*Correspondence: Xianliang Meng, eGxtZW5nQHlzZnJpLmFjLmNu