- 1Ocean College, Hainan University, Haikou, China
- 2Tropical Aquaculture Research and Development Center, South China Sea Fisheries Research Institute, Chinese Academy of Fishery Sciences, Sanya, China
- 3Key Laboratory of South China Sea Fishery Resources Exploitation and Utilization, Ministry of Agriculture and Rural Affairs, Guangzhou, China
- 4Sanya Tropical Fisheries Research Institute, Sanya, China
This study was conducted to understand the changes of physiological and biochemical indexes of black and red shell Pinctada fucata under acute high and low salt stress. In this study, the salinity of 35‰ was used as the control, while the salinities of 20 and 50% salinity were used as the low and high salt treatment groups, respectively. The osmotic pressure (OSM) and ion concentration in the hemolymph, Na+-K+ -ATPase (NKA) activity and respiratory metabolism in gills, and antioxidant and immune (non) enzymes in the hepatopancreas of P. fucata with two shell colors were compared and analyzed at the time periods of 1.5 and 3 h post-salinity stress. The results showed that the OSM and inorganic ion (Na+, Ca2+, and Cl–) concentration in the hemolymph of the black and red P. fucata increased significantly with the increase of salinity after the time periods of 1.5 and 3 h. At 3 h, the black P. fucata NKA activity decreased significantly with the increase of salinity, while red P. fucata reached the highest value at high salinity. The succinate dehydrogenase (SDH) and lactate dehydrogenase (LDH) activities of red P. fucata showed U-shaped and inverted U-shaped distributions with the increase of salinity after 1.5 h, respectively. With the increase of salinity, the phenoloxidase (POX) activity of red and black P. fucata showed inverted U-shaped and U-shaped distributions, respectively. The contents of glutathione (GSH) and vitamin C (VC) in black P. fucata decreased significantly with the increase of salinity at 1.5 and 3 h. Red P. fucata GSH and VC reached their maximum value in the 1.5-h low salinity group and 3-h high salinity group. The vitamin E (VE) content in black P. fucata increased significantly with the increase of salinity at 1.5 h, and reached the maximum at 3 h in the control group. Red P. fucata VE reached the maximum at 1.5 and 3 h in the control group. The results obtained from the present study revealed that the sensitivity of P. fucata to salinity varied in shell color. Compared to black P. fucata, red P. fucata responds more quickly to sharp salinity changes, thereby reducing more likely damage. Compared with a high salt environment, P. fucata was more adaptable to the changes of acute low salt environment. The results obtained from the present study provide the physical references for subsequent selective breeding of this species.
Introduction
Pinctada fucata, belonging to mollusks, bivalves, Pinctada and Pinctada family mainly distributed in the tropical and subtropical area, is one of the main shellfish species for cultivating seawater pearls in China (Sun et al., 2021). Since 1949, P. fucata has been cultivated in Hainan and Liangguang (Guangdong and Guangxi) Provinces of China until pearl production peaked in the 1990s (Ai et al., 2003; Yang et al., 2017). P. fucata has greatly promoted the development of pearl industry in China and created considerable economic income for the nation. However, massive mortalities of P. fucata are frequently reported and have caused considerable economic losses in the past 10 years. To understand the causes of such mortalities, a series of studies have been conducted. Evidence has indicated that temperature, salinity, dissolved oxygen, and pH can change the physiological indexes of P. fucata, including osmotic pressure (OSM), oxygen consumption rate, ammonia excretion rate and cellular immune level, disturb balance basal metabolism, inhibit growth, and even lead to death (Yang, 2016; Liu, 2018; Sun et al., 2021). All the evidence reveal that massive mortality of P. fucata is closely related to these environmental changes.
Salinity is an important ecological factor in marine ecosystems, and its fluctuation can change the osmotic adjustment, metabolism, distribution, and biological rhythm of bivalves. Extreme salinity fluctuation will damage the physiological factors of bivalves, resulting in death and economic in severe cases (Kurihara, 2017; Sun et al., 2021). In addition to human activities, heavy rainfall, storm, and ebb will significantly change the salinity, which directly affects the OSM of marine animals and indirectly impairs the survival, growth, and reproduction of organisms (Cheng et al., 2002; Pourmozaffar et al., 2020). A few studies have shown that the filtration, respiration, growth rate, and feeding activity of marine bivalves decrease when salinity changes (Alagarswami and Victor, 1976; Widdows, 1985; Berger and Kharazova, 1997). Furthermore, evidence suggests that the maximum growth of organisms occurs in isotonic media as the energy consumption is the least and the energy conversion efficiency is the highest in OSM regulation (Chen and Lin, 1995; Selven and Philip, 2013). In mollusks, inorganic ions are firstly used as mobilization osmolytes for cell volume regulation (intracellular isosmotic regulation), and K+, Cl–, and Na+ ions are mainly involved in osmotic regulation (Lin et al., 2021). Natochin et al. (1979) found that besides the Na+ and K+ pump, both Mytilus edulis and Littorina littorea have a Cl–-related sodium ion exchange system, which could regulate OSM and cell volume. Under the condition of acute low permeability (hyposmotic), the osmotic adjustment is first carried out in response to salinity change (Zhao et al., 2018; Lin et al., 2021). In general, increasing salinity is more harmful to invertebrates compared to seawater dilution. Moreover, most studies on salinity challenges in mollusks involve seawater dilution rather than seawater concentration, and only a few studies have tested mollusks in high salt seawater (Willmer, 1978; Berger and Kharazova, 1997; Veiga et al., 2016).
Both high and low salt stress can change the physiological activities and states of marine shellfish. For example, snails remain active and the gill cover open in pre-55‰ high salinity seawater, either suddenly closing the gill cover or closing the wall (aquarium wall) for a longer time in diluted seawater (Veiga et al., 2016). In a short period of salinity reduction, the hemolymph volume of greenlip abalone (Haliotis laevigata) and blacklip abalone (Haliotis rubra) increased by 25‰ (Edwards, 2003). While the abalone hemolymph volume decreased, gill cilia movement, adhesion, and heartbeat were affected with an increase in salinity (Edwards, 2003). Salinity can significantly affect various physiological functions of marine bivalves, and its stress effects in different species are different. At the salinity of 27‰, the expression level of α-amylase in P. fucata was significantly higher than that in the high and low salinity treatment groups (Huang et al., 2016). At low salinity, mitochondria in the gills of Crassostrea virginica showed a higher ratio of glutamic acid oxidation (Ballantyne and Moyes, 1987). Rapid changes in salinity in American oysters (C. virginica) affected the hemocyte locomotion rate and prolonged their transmission time (Fisher and Newell, 1986).
It is reported that shell color may be potentially associated with beneficial characteristics such as salt resistance and metabolic response (Nie et al., 2017). Different color phenotypes of the same species may have different adaptation efficiencies and resistances to salinity stress (Berger and Kharazova, 1997; Nie et al., 2017). By comparing Littorina obtusata with different shell color phenotypes, it can be proven that the adaptation of snails to salinity changes is significantly different (Sergievskii and Berger, 1984). The shell color of directional breeding can be inherited stably to offspring. Previous studies have shown that the purification rates of black and red shell color in the F3 breeding line of P. fucata are the highest, which are 95.83 and 100‰, respectively (Sun, 2018). The ammonia excretion rates in black and red colors of the F6 breeding line P. fucata are significantly different from ordinary population, indicating its research value (Zhu et al., 2011). A comparison of SOD and CAT activities between zebra and white zebra strains of Ruditapes philippinarum under low salt stress showed that strains with higher salt tolerance could change their biochemical mechanisms under salinity stress (Nie et al., 2020). At the same time, there is also evidence that the physiology and biochemistry of P. fucata can be regulated by salinity changes. It was found that the ammonia excretion and oxygen consumption rate of P. fucata decreased with the increase of salinity in the range of 21–36‰ (Liu et al., 2011). The aquaporin expression level of P. fucata was restored to the control level (27‰) under low salinity (16‰) for 72 h and under high salinity (36‰) for 168 h (Pan et al., 2020). Low lysozyme (LZM) and catalase activities in P. fucata under short-term low salt stress may made it vulnerable to diseases (Arisman et al., 2018). In this study, the effects of salinity on OSM regulation, respiratory metabolism, immunity, and antioxidant capacity were measured to explore the response of P. fucata with black and red shells to acute salinity changes. The results from the present study will provide a physical reference for the selective breeding of P. fucata and will improve our understanding on the salt tolerance mechanism in this species.
Materials and Methods
Animals
A total of 360 P. fucata were bred and cultured by Tropical Aquaculture Research and Development Center, South China Sea Fisheries Research Institute, Chinese Academy of Fishery Sciences (Lingshui Town, Hainan, China). After removing the attached organisms on the shell surface, 270 healthy individuals with distinct shell color (black and red) characteristics and similar size (body mass: 34.19 ± 1.39 g, DVM: 50.75 ± 1.43 mm) were collected and transferred to a 5,000-L cement tank and acclimated for a week. During the acclimation, the environmental parameters were maintained at the salinity of 35‰, 30 ± 1°C, PH 8.0 ± 0.1, DO > 6.5 mg/L, and light intensity <500 Lx with a natural photoperiod. Platymonas subcordiformis (2 × 105 cells/ml) was fed once a day at regular intervals (09:00–09:30) in the morning, and 50‰ of the seawater was replaced daily. The feeding was stopped at the day before the experiment started.
Experimental Design
The experiment was carried out in nine 800-L cement tanks. The salinity of 35‰ (natural salinity in the rearing area) was used as the control, and the salinities of 20 and 50‰ were used as two testing groups. In the testing groups, the salinities of 20 and 50‰ were adjusted either by mixing natural seawater with 24 h aerated tap water or sea salt. In this study, three replicates were used in both testing groups and the control group, and each replicate contained 15 P. fucata with black and red shells, respectively. Six shellfish samples (3 red P. fucata and 3 black P. fucata) were randomly selected from each replicate at 1.5 and 3 h, respectively.
Hemolymph Sample Collection
The shell was opened with a shell opener, and the adductor muscle was cut off along the left shell of the shell with a dissector. The hemolymph was extracted from the pericardiac cavity of the shellfish with a 2-ml syringe. The hemolymph of each replicate of 6 P. fucata with black and red shells, respectively, was collected at each point in time (1.5 or 3 h) and mixed together in pairs. Then, they were quickly discharged into some 2 ml centrifuge tubes. PL2000Plus blood gas biochemical analyzer (NanjingPlang Medical Equipment Co., Ltd., Nanjing, China) was used to extract 0.3–0.4 ml hemolymph each time to determine the blood routine indicators: K+, Na+, Ca2+, Cl–, and OSM.
Tissue Sample Collection
After the hemolymph was collected, the gills and hepatopancreas tissues of each shellfish were quickly collected on the ice bag with a sterile dissector. After washing with pre-cooling 0.9‰ saline, the tissue surface water was gently dried with clean filter paper and quickly placed in a 2-ml centrifuge tube, and stored in -80°C refrigerator for further determination. The 0.9‰ normal saline or the corresponding “sample homogenizing medium” was added into tissue samples under ice bath conditions, then they were homogenized into or diluted to the optimal concentration required for different enzymes. Finally, the following relevant indicators were determined according to the experimental steps of the instructions.
The biochemical parameters of hepatopancreas and gills tissue were determined according to the instructions of the manufacturer using commercial kits (Nanjing Jiancheng Biological Co., Ltd., Nanjing, China and Abbkine Scientific Co., Ltd., Wuhan, China), i.e., lactate dehydrogenase (LDH) (Item No. KTB1110): 2,4-Dinitrophenylhydrazine method; Na+-K+ -ATPase (NKA) (Item No. KTB1800): Inorganic phosphorus method; succinate dehydrogenase (SDH) (Item No. A022-1-1): Phenazine methosulphate method; phenoloxidase (POX) (Item No. H247): competition method; LZM (Item No. A050-1-1): Micrococcus Lysodeik-ticus method; glutathione (GSH) (Item No. A006-2-1): Ellman reagent colorimetric method; vitamin E (VE) (Item No. A008-1-1): Phenanthroline colorimetry method; vitamin C (VC) (Item No. A009-1-1): Xylene dichloroindophenol colorimetry method; and total protein (Item No. A045-4-4): bicinchoninic acid method. The biochemical parameter analyses of all gills and hepatopancreas tissues were performed thrice.
Statistical Analysis
The data were expressed as mean ± SD and analyzed by IBM SPSS Statistics 26 statistical analysis software. A one-way ANOVA and the Duncan multiple comparison method were used to analyze the hemolymph biochemical indices and related indices of tissues (gill and liver) of P. fucata with the same shell color under different salinities. When analyzing the differences in the abovementioned related indicators of black and red P. fucata with different shell colors under the same salinity, the t-test was used. p < 0.05 was used as the significant difference level.
Results
Determination of Osmotic Pressure in Hemolymph
The responses of hemolymph OSM to salinity changes in P. fucata with different shell colors are shown in Figure 1. At 1.5 and 3 h, the OSM gradually increased with the increase of salinity within 20–50‰ (p < 0.05). After exposure to the salinities of 20 and 50‰ for 3 h and exposure to the salinity of 20‰ for 1.5 h, the OSM of black P. fucata was significantly higher than that of red P. fucata (p < 0.05). After exposure to the salinity of 50‰ for 1.5 h, the OSM of red P. fucata was significantly higher than that of black P. fucata (p < 0.05). At the salinity of 35‰, there was no significant difference in the OSM between red and black P. fucata (p > 0.05).
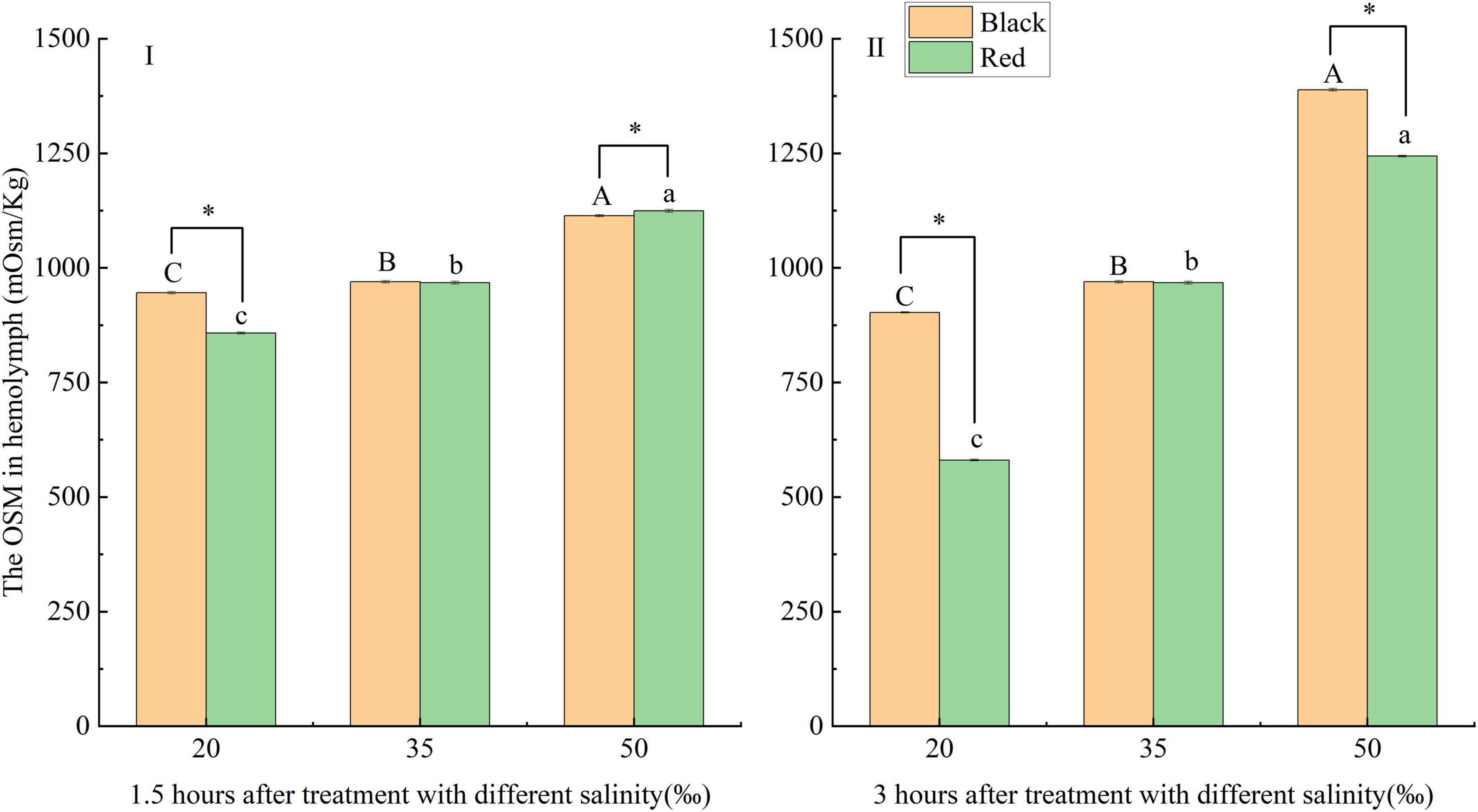
Figure 1. Effects of acute (I: 1.5 h; II: 3 h) salinity stress on hemolymph osmotic pressure (OSM) of black and red Pinctada fucata. Different capital letters (A, B, C) denotes that the hemolymph OSM of black P. fucata was significantly different at different salinities (p < 0.05), while the same showed no significant difference (p > 0.05). Different lowercase letters (a, b, c) denotes that the hemolymph OSM of P. fucata in red shell color varied significantly under different salinities (p < 0.05), while the same showed no significant difference (p > 0.05). The asterisk sign “*” indicates that there are significant differences between the different shell colors at the same salinity (p < 0.05).
Determination of Related Ion Concentration in Hemolymph
The responses of the concentration of related inorganic ions in the hemolymph of P. fucata with different shell colors to salinity changes are shown in Figure 2. The K+ concentration of black P. fucata at 1.5 and 3 h and of red P. fucata at 3 h gradually increased with the salinity (p < 0.05). Under the same salinity, the K+ concentration of black and red P. fucata is significantly different after 3 h treatment with the salinities of 20–50‰ and 1.5 h treatment with the salinities of 20 and 35‰ (p < 0.05). The K+ concentration of red P. fucata was significantly higher than that of black P. fucata (p < 0.05) except for the treatment group exposed to the salinity of 20‰ for 3 h (Figures 2I,II).
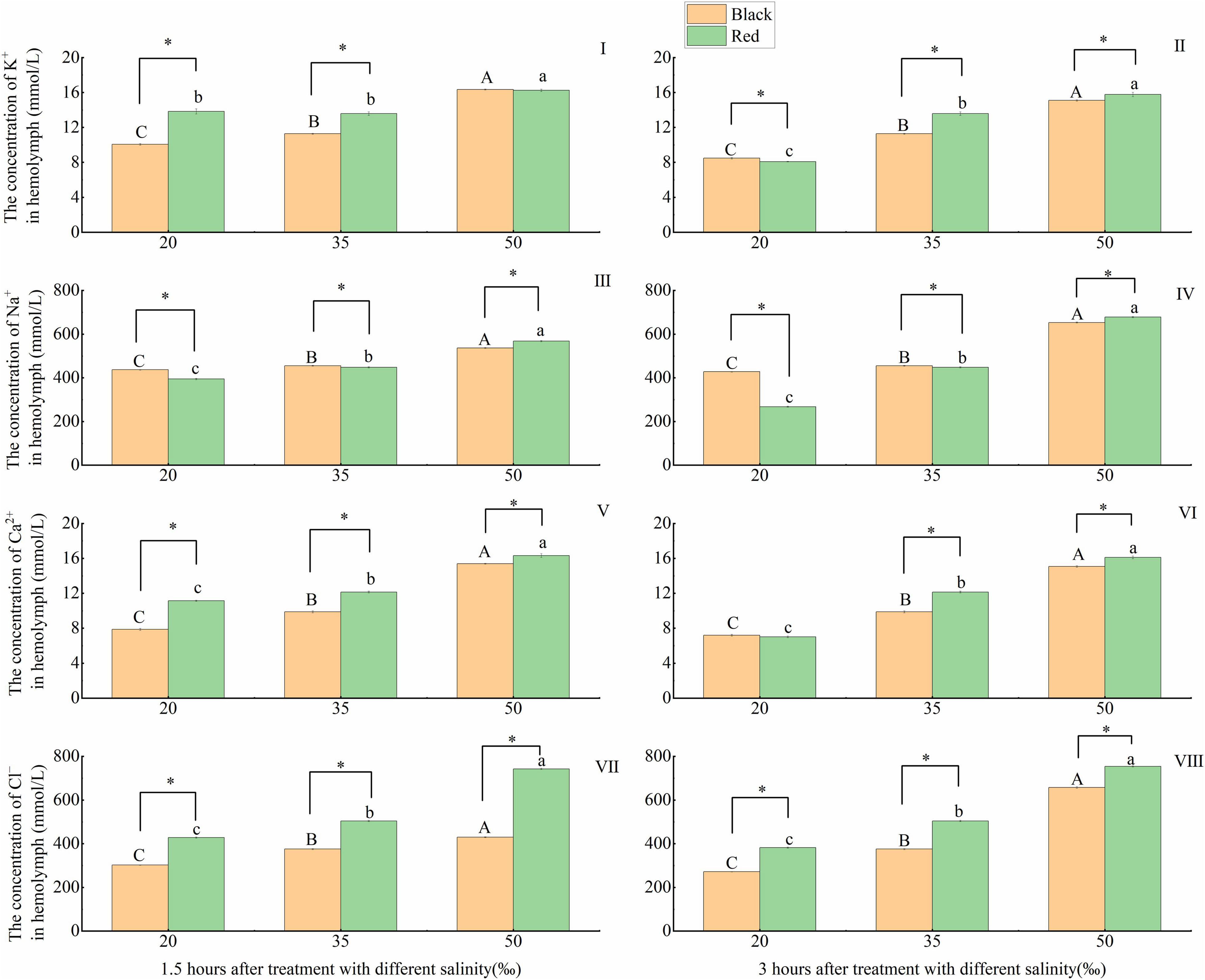
Figure 2. Effects of acute salinity stress on the concentration of inorganic ions in the hemolymph of black and red P. fucata (I: 1.5 h, K+ concentration. II: 3 h, K+ concentration. III: 1.5 h, Na+ concentration. IV: 3 h, Na+ concentration. V: 1.5 h, Ca2+ concentration. VI: 3 h, Ca2+ concentration. VII: 1.5 h, Cl– concentration. VIII: 3 h, Cl– concentration). The asterisk sign “*” indicates that there are significant differences between the different shell colors at the same salinity (p < 0.05).
At 1.5 and 3 h, with the increase of salinity, the concentration of Na+ increased significantly (p < 0.05). At the same salinity, the Na+ concentration of black P. fucata was significantly different from that of red P. fucata (p < 0.05). Under the salinities of 20 and 35‰, the Na+ concentration of black P. fucata was significantly higher than that of red P. fucata (p < 0.05). Under the salinity of 50‰, the Na+ concentration of red P. fucata was significantly higher than that of black P. fucata (p < 0.05, Figures 2III,IV).
At 1.5 and 3 h, with the increase of salinity, Ca2+ concentration in the hemolymph gradually increased (p < 0.05). At the same salinity after 1.5 h exposure, and at the salinities of 35‰ and 50 after 3 h exposure, the Ca2+ concentration in red P. fucata was significantly higher than that in black P. fucata (p < 0.05, Figures 2V,VI).
At 1.5 and 3 h, the concentration of Cl– gradually increased with the increase of salinity (p < 0.05). At the same salinity and time (1.5 or 3 h), the Cl– concentration in red P. fucata was significantly higher than that in black P. fucata (p < 0.05, Figures 2VII,VIII).
Effect of Salinity on the Activities of Enzymes Related to Respiratory Metabolism and Na+-K+ -ATPase in Gills
The responses of the activities of respiratory metabolism and osmoregulation-related enzymes in the gill tissues of P. fucata with different shell colors to salinity changes were shown in Figure 3. The SDH activity of red and black P. fucata was significantly higher than that in the 35‰ at the salinity of 50‰ after 1.5 h exposure and at the salinity of 20‰ after 3 h exposure (p < 0.05). Under the same salinity and time (1.5 or 3 h), there was a significant difference between red and black P. fucata SDH (p < 0.05). When exposed to the salinities of 35 and 50‰ for 1.5 h, the SDH of black P. fucata was significantly higher than that of red P. fucata (p < 0.05). When exposed to the salinity of 20‰ for 1.5 h, the SDH of red P. fucata was significantly higher than that of black P. fucata (p < 0.05, Figures 3I,II).
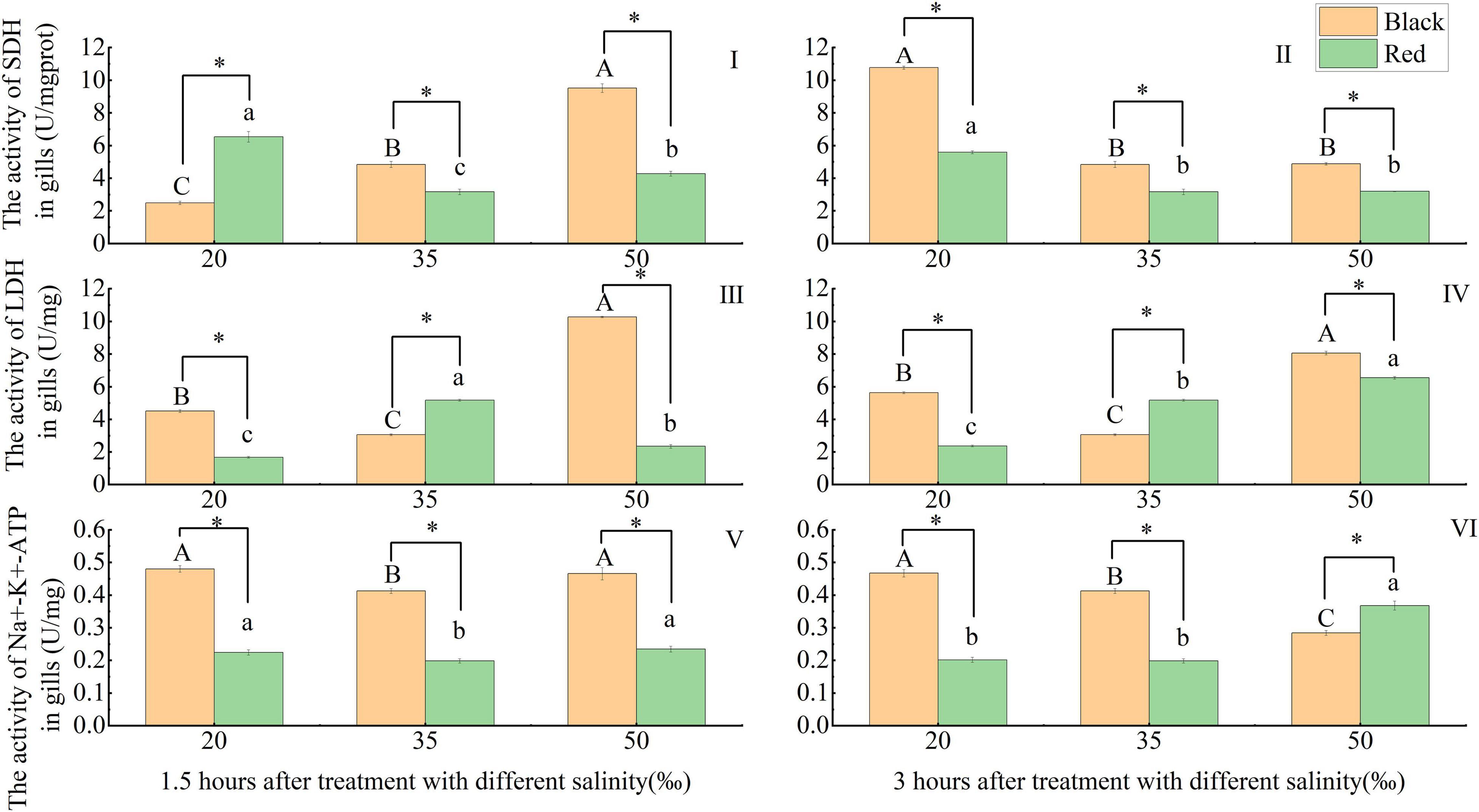
Figure 3. Effect of acute salinity stress on the activities of succinate dehydrogenase (SDH), lactate dehydrogenase (LDH), and Na+-K+ -ATPase (NKA) in the gill tissues of black and red shell-colored P. fucata (I: 1.5 h, SDH activity. II: 3 h, SDH activity. III: 1.5 h, LDH activity. IV: 3 h, LDH activity. V: 1.5 h, NKA activity. VI: 3 h, NKA activity). The asterisk sign “*” indicates that there are significant differences between the different shell colors at the same salinity (p < 0.05).
Compared with the 35‰ group, the LDH in black P. fucata increased significantly at the salinities of 20 and 50‰ at 1.5 and 3 h (p < 0.05), and red P. fucata LDH was significantly decreased at the salinities of 20 and 50‰ at 1.5 h (p < 0.05). At 3 h, red P. fucata LDH increased significantly with the increase of salinity (p < 0.05). Under the same salinity and time (1.5 or 3 h), there was a significant difference between red and black P. fucata LDH (p < 0.05). Under the salinity of the 35‰ group, red P. fucata LDH was significantly higher than that of black P. fucata (p < 0.05), while the opposite was observed at the salinities of 20 and 50‰ (p < 0.05, Figures 3III,IV).
Compared with the 35‰ group, the NKA activity of P. fucata with two shell colors increased significantly at the salinities of 20 and 50‰ at 1.5 h (p < 0.05). At the same salinity and time (1.5 or 3 h), the NKA activity of red P. fucata was significantly different from that of black P. fucata (p < 0.05). At different salinities after 1.5 h exposure, and at the salinities of 20 and 35‰ after 3 h exposure, black P. fucata was significantly higher than red P. fucata (p < 0.05), while the opposite was observed at the salinity of 50‰ after 3 h (p < 0.05, Figures 3V,VI).
Effect of Salinity on the Activities of Immune-Related Enzymes in Hepatopancreas
Phenoloxidase activity decreased significantly with increasing salinity at 1.5 h (p < 0.05). After 3 h, compared with the 35‰ group, the POX activity of black P. fucata was significantly increased (p < 0.05), and that of red P. fucata was significantly decreased (p < 0.05). Under the same salinity and time (1.5 or 3 h), the POX activity of red P. fucata was significantly different from that of black P. fucata (p < 0.05). At different salinities after 1.5 and at the salinities of 20 and 35‰ after 3 h, the POX activity of red P. fucata was significantly higher than black P. fucata (p < 0.05). At the salinity of 50‰ after 3 h, black P. fucata was significantly higher than red P. fucata (p < 0.05, Figures 4I,4II).
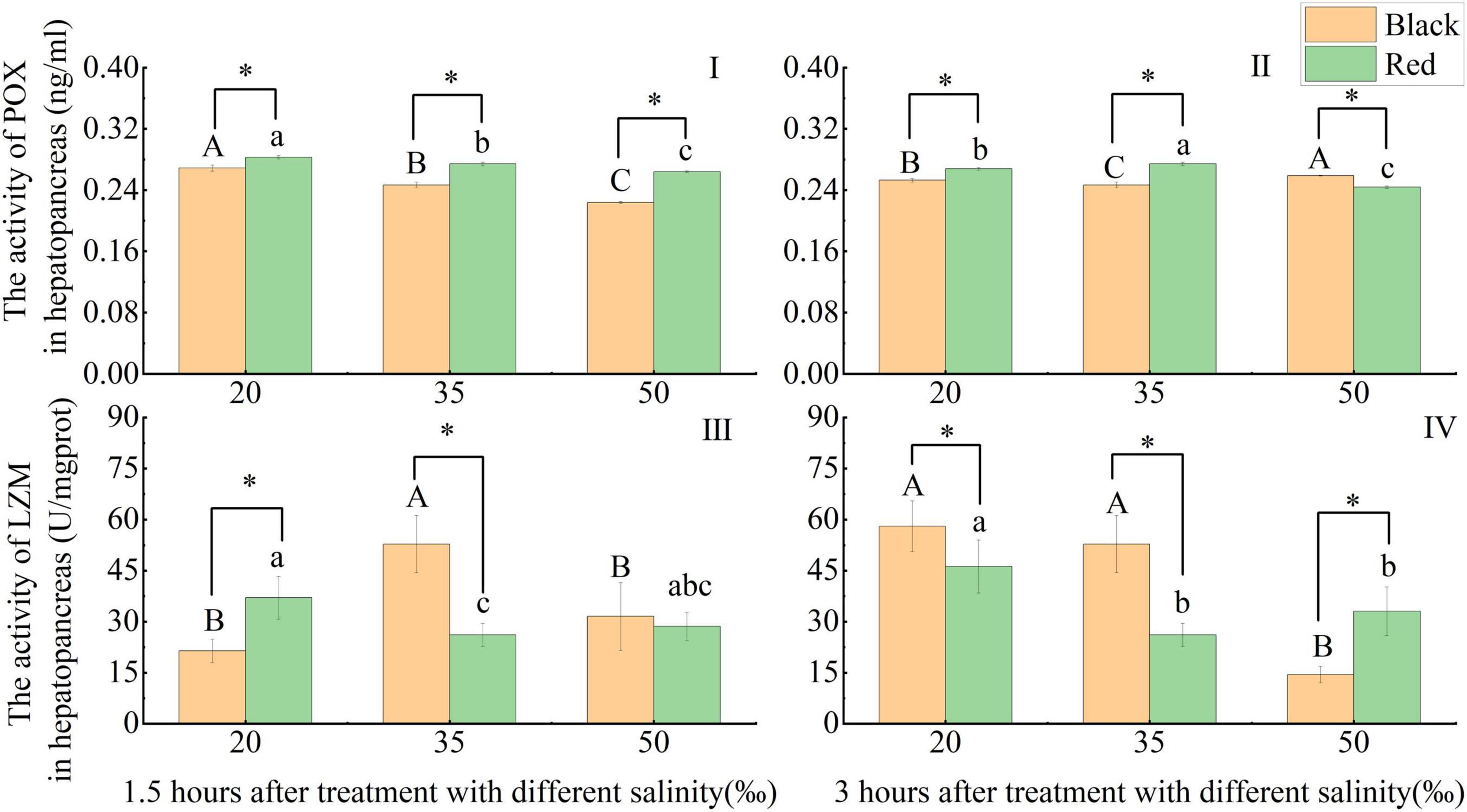
Figure 4. Effects of acute salinity stress on the activities of phenoloxidase (POX) and lysozyme (LZM) in the hepatopancreas of black and red P. fucata (I: 1.5 h, POX activity. II: 3 h, POX activity. III: 1.5 h, LZM activity. IV: 3 h, LZM activity). The asterisk sign “*” indicates that there are significant differences between the different shell colors at the same salinity (p < 0.05).
At 1.5 h, compared with the 35‰ group, the LZM activity of black P. fucata decreased significantly at the salinities of 20 and 50‰ (p < 0.05), red P. fucata LZM increased significantly at the salinity of 20‰ (p < 0.05). At 3 h, compared with the 35‰ group, black P. fucata decreased significantly at the salinity of 50‰ (p < 0.05) and showed no significant difference under the salinity of 20‰ (p > 0.05). In contrast, the LZM of red P. fucata increased significantly at the salinity of 20‰ (p < 0.05), and showed no significant difference at the salinity of 50‰ (p > 0.05). The LZM activity of red P. fucata was significantly different from that of black P. fucata after exposure at the salinities of 20 and 35‰ for 1.5 h and at different salinities for 3 h (p < 0.05). At 1.5 h, red P. fucata was significantly higher than black P. fucata at the salinity of 20‰ (p < 0.05), and the opposite was true at the salinity of 35‰ (p < 0.05), there was no significant difference in the shell color at the salinity of 50‰ (p > 0.05). At 3 h, black P. fucata was significantly higher than red P. fucata at the salinities of 20 and 35‰ (p < 0.05), and the opposite was observed at the salinity of 50‰ (p < 0.05, Figures 4III,IV).
Effect of Salinity on the Contents of Antioxidant-Related Non-enzymes in Hepatopancreas
The content of GSH in black and red P. fucata was significantly higher than that in the 35‰ at the salinity of 20‰ at 1.5 h (p < 0.05). At 1.5 h, the GSH content in black P. fucata was significantly higher than that in red P. fucata at the salinity of 35‰ (p < 0.05), there was no significant difference in the shell color at 20 and 50‰ (p > 0.05). At 3 h and the salinities of 20 and 35‰, the content of GSH in black P. fucata was significantly higher than that in red P. fucata (p < 0.05), and the opposite results were observed at the salinity of 50‰ (p < 0.05, Figures 5I,II).
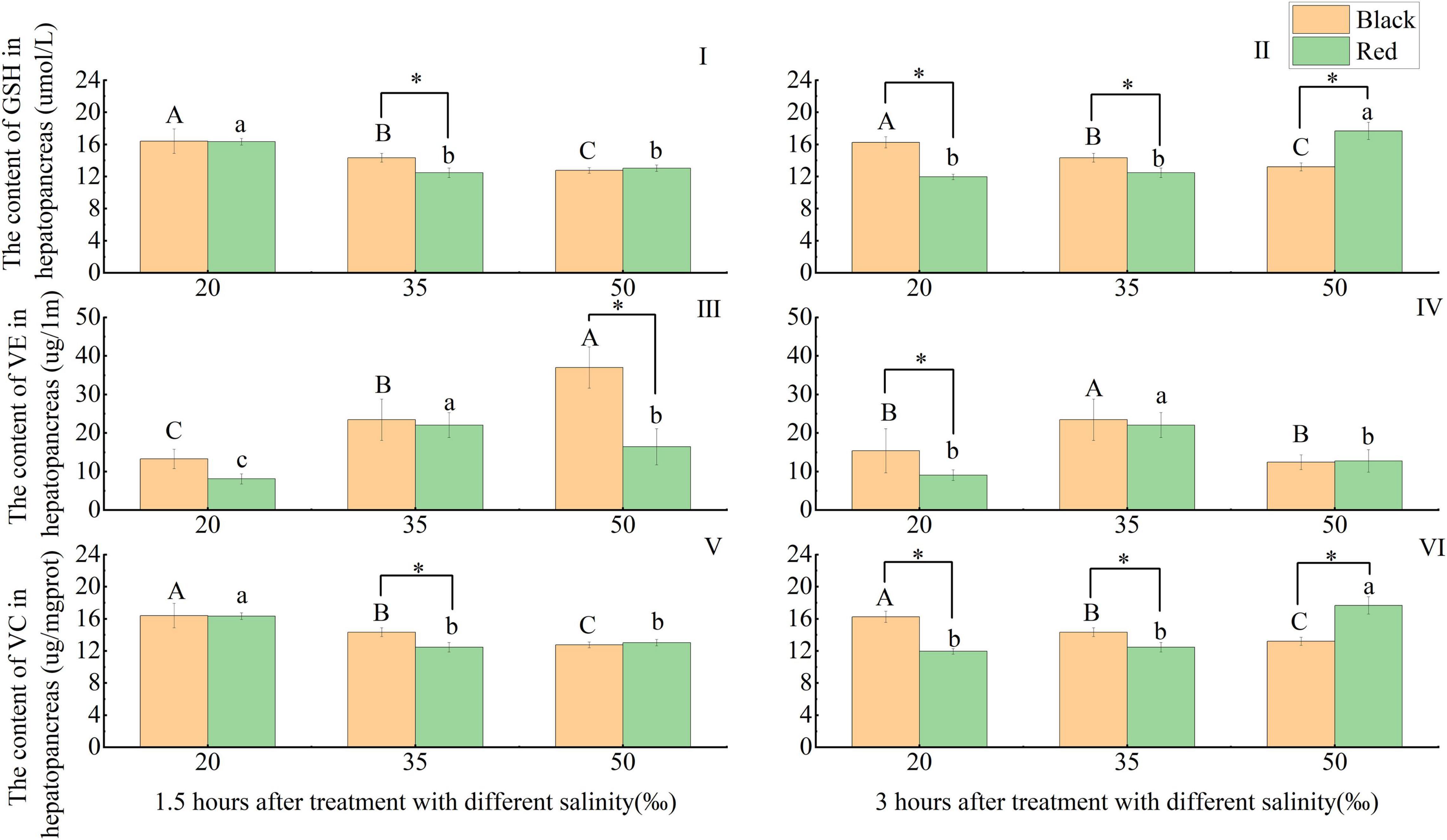
Figure 5. Effects of acute salinity stress on the contents of glutathione (GSH), vitamin E (VE), and vitamin C (VC) in the hepatopancreas of black and red P. fucata (I: 1.5 h, GSH content. II: 3 h, GSH content. III: 1.5 h, VE content. IV: 3 h, VE content. V: 1.5 h, VC content. VI: 3 h, VC content). The asterisk sign “*” indicates that there are significant differences between the different shell colors at the same salinity (p < 0.05).
At 1.5 h, black P. fucata VE content increased significantly with increasing salinity (p < 0.05), red P. fucata was significantly decreased at the salinities of 20 and 50‰ compared with the 35‰ group (p < 0.05). And the VE content of black P. fucata is significantly higher than that of red P. fucata at the salinity of 50‰ (p < 0.05), but there is no significant difference between black and red P. fucata at the salinities of 20 and 35‰ (p > 0.05). At 3 h, compared with the 35‰ group, the VE content of black and red P. fucata decreased significantly at the salinities of 20 and 50‰ (p < 0.05). And the VE content of black P. fucata was significantly higher than that of red P. fucata at the salinity of 20‰ (p < 0.05), there was no significant difference in the shell color between the salinities of 35 and 50‰ (p > 0.05, Figures 5III,IV).
The variation trend of VC content was completely consistent with that of GSH (Figures 5V,VI).
Discussion
Iono-Osmoregulation
The lower OSM resulted in the release of KCl through K+ and Cl– channels and K-Cl transporters. In addition, the NaCl and KCl concentration could be increased through Na-K-2Cl synergistic transporters and Na+/H+ and Cl–/HCO3–exchangers to respond to high osmotic conditions (Pourmozaffar et al., 2020). Therefore, the salinity adaptation of bivalves depends largely on their ability to regulate cell volume by collecting or reducing osmotic solutes.
The cell OSM of marine invertebrates such as bivalves is matched with the external environment. Therefore, any fluctuation of salinity level in the surrounding waters will cause the change of hemolymph OSM (Pourmozaffar et al., 2020). OSM allows the body to achieve homeostatic balance between the internal body and external environment, which is a basic physiological process for most aquatic animals (Sun et al., 2021). Most bivalve species exposed to reduced salinity were found to reduce OSM gradually under a low salinity environment (Bayne et al., 1976; Kurihara, 2017). Meanwhile, the osmoregulation has been reported in several marine bivalves species, such as horse mussels Modiolus sp. (Pierce, 1971), Pacific oyster Crassostrea gigas (Shumway, 1977), common mussel M. edulis (Willmer, 1978; Davenport, 1979), arcid clam Noetia ponderosa (Amende and Pierce, 1980), soft clam Mya arenaria (Deaton, 1992), Meretrix lusoria (Lin et al., 2021), and P. fucata (Sun et al., 2021). It was found that the hemolymph OSM directly altered with seawater density (seawater density altering OSM), and was either equal to the ambient OSM, or slightly hyperosmotic (5–50 mOsm/kg) to ambient media in a certain salinity atmosphere (non-lethal). In this study, the OSM of black and red P. fucata increased significantly with the increase of salinity at 1.5 and 3 h, which was consistent with previous findings. After exposure to the salinities of 20 and 50‰ for 1.5 and 3 h, the OSM of black and red P. fucata was significantly different. And, there was no significant difference in OSM between red and black P. fucata in the control group, indicating that black and red P. fucata may have different tolerance to high and low acute salinity stress. Compared with the results reported by Sun et al. (2021), the OSM of P. fucata at 3 h tended to be consistent with that at 12 and 24 h, indicating that the OSM might begin to stabilize after acute salinity stress for 3 h. In this study, at 1.5 and 3 h, there were significant differences in the OSM of P. fucata with two shell colors between the high and low salt groups and the control group. This may be due to a large variation of salinity in the test group compared with the control group, resulting in a wide range of OSM changes, which significantly increased or decreased in the test group.
After exposure to new environmental conditions, bivalves maintain OSM and cell volume by regulating free amino acids (FAAP) and ion exchange (Pourmozaffar et al., 2020). Inorganic ions are the main OSM effectors in the hemolymph of aquatic animals. In the bivalves, inorganic ions (Na+, K+, Cl–) are responsible for protecting them from a rapid change in salinity. Na+ and Cl– are the most important contributors and are also the most abundant ions in seawater (accounting for 85‰) (Cheng et al., 2002; Jiang, 2017; Pourmozaffar et al., 2020; Yamaguchi and Soga, 2020). The change of intracellular Na+ concentration affects the OSM response of organisms (Berger and Kharazova, 1997). K+ mainly acts on the maintenance of neuronal OSM and also on the maintenance of normal nervous system function (Cooper and Morris, 1997). According to the discovery of Sun et al. (2021), K+, Cl–, and Na+ are not regulated by hormones and the nervous system, and directly change with the salinity of external environment in bivalves. In this study, the ion (Na+, Ca2+, and Cl–) concentration of P. fucata with two shell colors and K+ concentration of black P. fucata in the hemolymph increased significantly with the increase of salinity. The K+ concentration in red P. fucata hemolymph reached the maximum at high salinity of 1.5 h, and increased significantly with the increase of salinity at 3 h, which was basically consistent with the change trend of OSM. Other species have proved this trend: the concentration of Cl–, Na+, and K+ in the hemolymph of invasive golden apple snail Pomacea canaliculata is proportional to salinity (Yang et al., 2018). OSM, Na+, and Cl– concentration in the hemolymph of soft clam decreased significantly with a decrease in salinity (Lin et al., 2021). The OSM and ion (Cl– and Mg2+) concentration of the intertidal gastropod Stramonita brasiliensis (Muricidae) in the hemolymph under sudden extreme salinity (70‰) was higher than the OSM and ion concentration of the control (35‰). However, compared with the control (35‰), the OSM, Cl–, and Mg2+ of S. brasiliensis did not decrease when exposed to freshwater (Veiga et al., 2016). In this study, the OSM and inorganic ions of P. fucata in the low salt group were significantly lower than those in the control group. This may be due to the different types of tolerance of different species to salinity. At the same time or at the same salinity, there was a significant difference between black and red P. fucata, indicating that the salt tolerance sensitivity of the two shell colors is different.
Na+-K+ -ATPase is a P-type ATPase, which can actively transport Na+ out and import K+ into cells, and is essential for maintaining cell OSM (Chikashi et al., 2011; Morth et al., 2011; Li and Langhans, 2015). The activity of NKA provides a major driving force for activating other ion transport systems involved in osmotic regulation (Lin et al., 2021). In this study, the activity of NKA also showed a U-shaped distribution with the increase of salinity at 1.5 h. At 3 h, the NKA activity of black P. fucata decreased significantly with increasing salinity. Sun et al. (2021) found that NKA in black P. fucata had an inverted U-shaped distribution with salinity at 12 and 24 h, and that in red P. fucata had an U-shaped distribution with salinity after 12 h. It indicated that black P. fucata NKA might gradually become stable after 3–12 h salinity stress. The inverted U-shaped NKA distribution of black P. fucata might be related to its weak adaptability to salinity changes and the temporary inhibition of NKA activity. Lin et al. (2021) found that the specific activity of NKA was not affected during adaptation to low salinity when soft clam was transferred from natural salinity to low salinity. It was consistent with the results of low salinity and control groups of red P. fucata at 3 h, while the trend was consistent with OSM at high salinity. NKA provided a driving force for secondary ion transport related to cell osmotic regulation in gills and gill caps (Lin et al., 2021). Yang et al. (2018) suggest that NKA activity in the mantle cavity of invasive golden apple snail P. canaliculata was negatively correlated with salinity. Similar results were also observed in this study, indicating that the osmotic regulation of P. fucata is not completely realized by NKA in the gill tissue.
Fitness Performance
In the process of life activities of shellfish, respiratory metabolism is the basic physiological activity of energy metabolism, which reflects the physiological status, metabolic characteristics, and adaptability to external environmental stress of shellfish (Marqueze et al., 2006; Li and He, 2016; Li, 2018). Aerobic respiration occupies the main position, but under low salt stress, anaerobic respiration can also provide energy for the body. SDH is an important part of mitochondrial inner membrane and plays a key role as the only enzyme in tricarboxylic acid cycle (TCA) (Jia et al., 2018). SDH activity can affect oxidative phosphorylation, so it reflects the level of aerobic metabolism to some extent (Wang et al., 2017; Jia et al., 2018). The results of this study showed that at 1.5 h, the SDH of red P. fucata under high and low salinity stress was significantly higher than that of the control group, which was contrary to the results of Nie et al.’s (2018) study on Dosinia corrugate. Because SDH reflected the level of aerobic metabolism, which was reflected under near isotonic conditions, indicating that the level of aerobic metabolism is the highest. Salinity stress enhanced the aerobic metabolism of P. fucata, which may be related to the need for large amounts of energy for the transmembrane transport of inorganic ions (Sun et al., 2021). The SDH activity of black P. fucata increased significantly with the increase of salinity at 1.5 h, which was consistent with the abovementioned research results. According to the study of Sun et al. (2021), after salinity stress for 24 h, red P. fucata may gradually adapt to salinity stress, and the aerobic metabolism level of black P. fucata was still affected. LDH is an important glycolytic enzyme required for cell energy metabolism (Nie et al., 2018).
Lactate dehydrogenase plays a key role in maintaining aerobic metabolism, and the activity of LDH is closely related to cell metabolism and can reflect the level of anaerobic respiration to some extent (Wei and Ma, 2001; Nie et al., 2018; Sun et al., 2021). In this study, the LDH in black P. fucata was significantly higher than that in the control group after 1.5 and 3 h at low salinity. Similar results have been reported by Sun et al. (2021) at 24 h and Nie et al. (2018) at low salinity. From the low salinity group to the control group, the LDH activity of black P. fucata gradually decreased with the increase of OSM, and anaerobic metabolism was hindered to some extent. More pyruvate entered and accelerated the TCA cycle, thus providing more energy for the body to adapt to environmental changes. Compared with the control group, the LDH of black P. fucata increased in the 1.5-h high salinity group. Combined with the change of SDH activity at 1.5 h, it indicated that anaerobic metabolism and aerobic respiration may jointly provide energy for resisting environmental stress, which was consistent with the research results of Shi et al. (2017). Our results indicated that the respiratory metabolism of black P. fucata increases under high salinity stress to meet the energy consumption of the body to adapt to environmental changes. After 1.5 h, the SDH and LDH of red P. fucata showed U-shaped and inverted U-shaped distributions with the increase of salinity, respectively. In other words, under 1.5-h salinity stress, the SDH of red P. fucata was higher than that of the control group, while the LDH of red P. fucata was lower than that of the control group, which means that aerobic respiration was done prior to anaerobic respiration. It was speculated that anaerobic respiration played a major auxiliary role near the isotonic point, and aerobic respiration decreased, while under salinity stress, aerobic respiration was mainly used to provide energy to resist environmental stress. Therefore, this mechanism provides more energy for the body to adapt to environmental changes, suggesting that red P. fucata may be better adapted to salinity changes.
The immune system and antioxidant system of shellfish also reflect their adaptability to environmental stress.
For invertebrates, the enzymes POX and LZM are essential in their innate defense mechanisms (Ruangsri et al., 2018). The innate immune system of invertebrates consists of many subsystems, and the POX (proPO) system has attracted much attention due to its important role in resisting pathogens and environmental stresses. It has been reported in a series of mollusks, including Sydney rock oysters, Pacific oyster, common mussel, Argentine mussels, pearl mussels, and Perna viridis (Coles and Pipe, 1994; Asokan et al., 1997; Aladaileh et al., 2007; Hellio et al., 2007; Jiang et al., 2020). POX is a copper-containing enzyme with oxidase activity and is an important immune-related enzyme in invertebrates. As a part of the proPO system, POX plays a vital role in the defense mechanism of mollusks (Jing et al., 2006; Pilar et al., 2006; Jiang et al., 2020). In this study, the POX activity of P. fucata decreased significantly with the increase of salinity at 1.5 h, which was completely opposite to the results reported in Haliotis discus (Jwa et al., 2009), Exopalaemon carinicauda, and Litopenaeus vannamei (Ge et al., 2017). This may be due to the inhibition of the immune system caused by high salinity in a short period of time, resulting in the decrease of immunity of P. fucata at high salinity. However, in E. carinicauda, it was found that the POX activity of larvae at low salinity was higher than that at high salinity, while the POX activity of adults was not significantly different (Ge et al., 2017). It was speculated that POX activity is related to species difference, age stage, and species specificity of the POX kit. In this study, the POX activity of black P. fucata showed an U-shaped distribution with the increase of salinity, which was basically consistent with the change trend of the SDH and LDH activity at 3 h. It was speculated that the activation of immune ability under low and high salinity stress may be related to the energy consumption of P. fucata. Compared with the control group, the black P. fucata POX activity at high salinity decreased significantly at first and then increased significantly from 1.5 to 3 h, while the POX activity increased significantly at 1.5 and 3 h under low salinity stress. It may suggest that under high salt stress, the immune system of black P. fucata improved over time, and low salt stress could more activate the immune system of P. fucata.
Lysozyme is a basic protein widely present in various tissues and body fluids, and is also a major component of the non-specific immune system, which plays an important role in the immunity of mollusks (Zhan et al., 2021). Environmental stress produces excessive oxygen free radicals in organisms, resulting in oxidative stress that can cause adverse physiological activities such as decline in growth, immunity and disease resistance, and even cause death. The rise and fall of salinity can cause changes in non-specific immune factors such as LZM, which is of great significance for disease prevention and resistance (Tian, 2019). In this study, the LZM activity of black P. fucata showed an inverted U-shaped distribution with the increase of salinity at 1.5 h. This results was consistent with the results reported in H. discus discus (Jwa et al., 2009) and Epinephelus moara (Liao, 2016), suggesting that high and low salinity stress caused the inhibition of immune system in black P. fucata. At 1.5 and 3 h, the LZM activity of red P. fucata was the highest in the low salinity group, and there was no significant difference between the control group and the high salinity group. It was speculated that red P. fucata had a greater immune response at low salinity as black P. fucata, and had a faster and better immune response than black P. fucata.
Environmental stress causes excessive reactive oxygen species (ROS) in organisms, resulting in the generation of products such as superoxide anion and hydrogen peroxide, which are highly cytotoxic in nature and have a negative impact on biological molecules (Huang et al., 2020). As an important biochemical strategy, cellular antioxidant defense systems can reduce oxidative stress damage by maintaining low intracellular ROS levels. The systems include non-enzymatic small antioxidant molecules and a series of enzymes (Halliwell and Gutteridge, 2015). Considering GSH as the substrate of GPX, it neutralizes hydrogen peroxide produced by SOD and has the effects of antioxidant and integrated detoxification, some studies have suggested that it plays an important role in osmotic and oxidative stress (Figueira et al., 2005; Manduzio et al., 2005; Paital and Chainy, 2010). In this study, the GSH of black P. fucata decreased significantly with the increase of salinity at 1.5 and 3 h. The result was consistent with the findings reported in three Veneridae clams (Venerupis decussata, Venerupis corrugate, and Venerupis philippinarum) (Carregosa et al., 2014), Pacific abalone (H. discus hannai) (Yang and Min, 2019), and arcid blood clam (Anadara granosa) (Anthony and Patel, 2000). Our results may suggest that black P. fucata can enhance the antioxidant defense ability to cope with the low salinity environment, while the antioxidant defense ability was inhibited under high salinity conditions. Compared with the control group, the content of GSH in red P. fucata was higher in the low salinity group at 1.5 h, and reached the maximum in the high salinity group at 3 h. There was no significant difference between the other salinity treatment group and the control group at different time periods. This may indicate that red P. fucata increases its antioxidant capacity in response to low salinity stress under the initial salinity stress.
Up to present, there are a few comparative studies on the effects of oxidative stress on the levels of VE and VC in aquatic organisms. In freshwater crayfish Astacus leptodactylus, the levels of VE in the hepatopancreas and muscle tissues are significantly lower than those in the control group (Barim and Karatepe, 2010; Barim-Oz, 2018). Similar trends were also observed in this study suggesting that (1) under salinity stress, the VE of red P. fucata consumed as a non-enzymatic antioxidant with low molecular weight to cope with the continuous generation of ROS produced by oxidative stress, resulting in a lower range than normal (control) (Barim-Oz, 2018) and (2) the decrease of VE level in tissues can be attributed to the inhibition of free radical formation on an antioxidant system (Barim and Karatepe, 2010). The VE content of black P. fucata increased significantly with the increase of salinity at 1.5 h, and the control group was significantly higher than the other salinity treatments at 3 h, indicating that black P. fucata may respond faster to low salinity environments, consistent with the previous findings.
Vitamin C is an important component of antioxidant defense system, which can reduce the damage of oxidative stress induced by salinity to the greatest extent (Mahmood et al., 2020). In this study, the VC content of black P. fucata decreased significantly with the increase of salinity at 1.5 and 3 h, while its content of red P. fucata reached the maximum at 1.5 h low salinity group and 3 h high salinity group. There was no significant difference between the other salinity treatment group and the control group at different times, which was completely consistent with the change trend of GSH content mentioned in this paper. This indicated that black P. fucata antioxidant defense has a better response to low salinity, which is consistent with the speculation of POX, LZM activity and GSH content mentioned in this paper. It was suggested that the antioxidant defense system and innate immune system were jointly involved in the resistance of P. fucata to salinity stress, and there was a certain correlation between its response to environment. It also suggested that red P. fucata may have stronger antioxidant defense ability than black P. fucata to cope with high and low salinity environmental changes.
Conclusion
In conclusion, this study compared and analyzed OSM and ion concentration in the hemolymph, NAK activity and respiratory metabolism in gills, and antioxidant and immune (non-) enzymes in the hepatopancreas of P. fucata with two shell colors under salinity stress. Our results indicate that the physiological and biochemical indexes of the two shell-colored P. fucata have different responses to salinity changes. Red P. fucata was more active and effective than black P. fucata in response to salinity changes in the external environment, reducing the damage caused by sharp environmental salinity changes. The results from the present study improve our understanding on the physical response of P. fucata to salinity stress, and provide a reference for further study on the salt tolerance mechanism of shell color-related P. fucata.
Data Availability Statement
The original contributions presented in the study are included in the article/Supplementary Material, further inquiries can be directed to the corresponding authors.
Ethics Statement
The animal study was reviewed and approved by the Animal Care and Use Committee of South China Sea Fisheries Research Institute, Chinese Academy of Fishery Sciences.
Author Contributions
GY and AW: conceptualization. JrY and MC: experimental operation. JrY, MC, ZF, and JS: field sampling. JlY and JS: sample determination. JrY: writing-original draft preparation. ZM, JlY, ZF, and ZG: writing-review and editing. All authors read and approved the final manuscript.
Funding
This study was funded by Central Public-Interest Scientific Institution Basal Research Fund, CAFS (No. 2020TD55), Guangxi Key Research and Development Program (Guike AB18221090), Natural Science Foundation of Hainan Province (319QN338), and Beihai Science and Technology Planning Project (Beikehe 201995002).
Conflict of Interest
The authors declare that the research was conducted in the absence of any commercial or financial relationships that could be construed as a potential conflict of interest.
Publisher’s Note
All claims expressed in this article are solely those of the authors and do not necessarily represent those of their affiliated organizations, or those of the publisher, the editors and the reviewers. Any product that may be evaluated in this article, or claim that may be made by its manufacturer, is not guaranteed or endorsed by the publisher.
Supplementary Material
The Supplementary Material for this article can be found online at: https://www.frontiersin.org/articles/10.3389/fmars.2021.792179/full#supplementary-material
References
Ai, H., Li, Y., and Ding, Y. (2003). A review of study on diseases in pearl oyster. J. Shanghai Ocean Univ. 12, 61–64.
Aladaileh, S., Rodney, P., Nair, S. V., and Raftos, D. A. (2007). Characterization of phenoloxidase activity in Sydney rock oysters (Saccostrea glomerata). Comp. biochem. physiol. B Biochem. Mol. Biol. 148, 470–480. doi: 10.1016/j.cbpb.2007.07.089
Alagarswami, K., and Victor, A. (1976). Salinity tolerance and rate of filtration of the pearl oyster Pinctada fucata. J. Mar. Biol. Assoc. India 18, 149–158.
Amende, L. M., and Pierce, S. K. (1980). Cellular volume regulation in salinity stressed molluscs: the response of Noetia ponderosa (Arcidae) red blood cells to osmotic variation. J. Comp. Physiol. 138, 283–289. doi: 10.1007/BF00691562
Anthony, K., and Patel, B. (2000). Combined effect of salinity and cadmium in the presence of other metals(Zn & Cu) and GSH on lysosomal responses in an arcid blood clam Anadara granosa. Indian J. Fish. 47, 311–319.
Arisman, N., Istiqomad, N., and Yoshimatsu, T. (2018). Impact of short-term hyposalinity stress on Akoya pearl oyster, Pinctada fucata (Gould 1850). Asian Fish Sci. 31, 265–275.
Asokan, R., Arumugam, M., and Mullainadhan, P. (1997). Activation of prophenoloxidase in the plasma and haemocytes of the marine mussel Perna viridis Linnaeus. Dev. Comp. Immunol. 21, 1–12. doi: 10.1016/s0145-305x(97)00004-9
Ballantyne, J. S., and Moyes, C. D. (1987). Osmotic effects on fatty acid, pyruvate, and ketone body oxidation in oyster gill mitochondria. Physiol. Zool. 60, 713–721. doi: 10.1086/physzool.60.6.30159987
Barim, O., and Karatepe, M. (2010). The effects of pollution on the vitamins A, E, C, β-carotene contents and oxidative stress of the freshwater crayfish, Astacus leptodactylus. Ecotoxicol. Environ. Saf. 73, 138–142. doi: 10.1016/j.ecoenv.2009.08.002
Barim-Oz, O. (2018). The effects on some non-enzymatic antioxidants and oxidative stress of Astacus leptodactylus (Esch., 1823) of starvation periods. Aquac. Nutr. 24, 492–503. doi: 10.1111/anu.12582
Bayne, B. L., Bayne, C. J., Carefoot, T. C., and Thompson, R. J. (1976). The physiological ecology of Mytilus californianus Conrad : 1. Metabolism and energy balance. Oecologia 22, 211–228. doi: 10.1007/bf00344793
Berger, V. J., and Kharazova, A. D. (1997). “Mechanisms of salinity adaptations in marine molluscs,” in Interactions and Adaptation Strategies of Marine Organisms, eds A. D. Naumov, H. Hummel, A. A. Sukhotin, and J. S. Ryland (Dordrecht: Springer Netherlands), 115–126.
Carregosa, V., Velez, C., Soares, A. M. V. M., Figueira, E., and Freitas, R. (2014). Physiological and biochemical responses of three Veneridae clams exposed to salinity changes. Comp. Biochem. Physiol. B Biochem. Mol. Biol. 177, 1–9. doi: 10.1016/j.cbpb.2014.08.001
Chen, J.-C., and Lin, C.-Y. (1995). Responses of oxygen consumption, Ammonia-N excretion and Urea-N excretion of Penaeus chinensis exposed to ambient ammonia at different salinity and pH levels. Aquaculture 136, 243–255. doi: 10.1016/0044-8486(95)01060-2
Cheng, W., Yeh, S.-P., Wang, C.-S., and Chen, J.-C. (2002). Osmotic and ionic changes in Taiwan abalone Haliotis diversicolor supertexta at different salinity levels. Aquaculture 203, 349–357. doi: 10.1016/S0044-8486(01)00606-8
Chikashi, T., Ryuta, K., and Flemming, C. (2011). First crystal structures of Na+,K+-ATPase: new light on the oldest ion pump. Structure 19, 1732–1738. doi: 10.1016/j.str.2011.10.016
Coles, J. A., and Pipe, R. K. (1994). Phenoloxidase activity in the haemolymph and haemocytes of the marine mussel Mytilus edulis. Fish Shellfish Immunol. 4, 337–352. doi: 10.1006/fsim.1994.1030
Cooper, A. R., and Morris, S. (1997). Osmotic and ionic regulation by Leptograpsus variegatus during hyposaline exposure and in response to emersion. J. Exp. Mar. Biol. Ecol. 214, 263–282. doi: 10.1016/S0022-0981(96)02778-5
Davenport, J. (1979). Is Mytilus edulis a short term osmoregulator? Comp. Biochem. Physiol. A Physiol. 64, 91–95.
Deaton, L. E. (1992). Osmoregulation and epithelial permeability in two euryhaline bivalve molluscs: Mya arenaria and Geukensia demissa. J. Exp. Mar. Biol. Ecol. 158, 167–177. doi: 10.1016/0022-0981(92)90224-X
Edwards, S. (2003). Assessment of the physiological effect of altered salinity on greenlip (Haliotis laevigata) and blacklip (Haliotis rubra) abalone using respirometry. Aquac. Res. 34, 1361–1365. doi: 10.1046/j.1365-2109.2003.00943.x
Figueira, S., Beeby, S., and Wu, A. S. (2005). “Topology-based meshes for local communication in data-parallel applications,” in Proceedings of the 2005 IEEE International Conference on Cluster Computing, Burlington, MA, 1–9.
Fisher, W. S., and Newell, R. I. E. (1986). Salinity effects on the activity of granular hemocytes of American Oysters. Crassostrea Virginica. 170, 122–134. doi: 10.2307/1541385
Ge, Q., Yu, G., Sun, M., Li, J., and Li, J. (2017). Comparison of white spot syndrome virus infection resistance between Exopalaemon carinicauda and Litopenaeus vannamei under different salinity stresses. J. Ocean Univ. China 16, 1195–1205. doi: 10.1007/s11802-017-3349-x
Halliwell, B., and Gutteridge, J. M. (2015). Free Radicals in Biology and Medicine. Oxford, MS: Oxford university press.
Hellio, C., Bado-Nilles, A., Gagnaire, B., Renault, T., and Thomas-Guyon, H. (2007). Demonstration of a true phenoloxidase activity and activation of a ProPO cascade in Pacific oyster, Crassostrea gigas (Thunberg) in vitro. Fish Shellfish Immunol. 22, 433–440. doi: 10.1016/j.fsi.2006.06.014
Huang, G., Guo, Y., Li, L., Fan, S., Yu, Z., and Yu, D. (2016). Genomic structure of the α-amylase gene in the pearl oyster Pinctada fucata and its expression in response to salinity and food concentration. Gene 587, 98–105. doi: 10.1016/j.gene.2016.04.044
Huang, Y., Wu, D., Li, Y., Chen, Q., and Zhao, Y. (2020). Characterization and expression of arginine kinase 2 from Macrobrachium nipponense in response to salinity stress. Dev. Comp. Immunol. 113:103804. doi: 10.1016/j.dci.2020.103804
Jia, X.-Y., Zhong, D.-S., Zhang, D., Wang, F., and Zhou, W.-L. (2018). Energy metabolic enzyme responses of Litopenaeus vannamei to thermal stress: a comparative study in freshwater and seawater conditions. Aquac. Int. 26, 1067–1081. doi: 10.1007/s10499-018-0268-9
Jiang, A. (2017). The Transcriptomic Analysis on Trachemys Scripta Elegans Under Chronic Salinity Stress. Haikou: Hainan Normal University.
Jiang, J., Zhao, Z., Yang, A., Gao, S., Dong, Y., Pan, Y., et al. (2020). Roles of phenoloxidases in the sea cucumber Apostichopus japonicus under environmental stresses. Aquac. Res. 51, 3664–3673. doi: 10.1111/are.14716
Jing, G., Li, Y., Xie, L., and Zhang, R. (2006). Metal accumulation and enzyme activities in gills and digestive gland of pearl oyster (Pinctada fucata) exposed to copper. Comp. Biochem. Physiol. Toxicol. Pharmacol. 144, 184–190. doi: 10.1016/j.cbpc.2006.08.005
Jwa, M.-S., Kang, K.-P., Choe, M. K., and Yeo, I.-K. (2009). Effects of low salinity stresses on the physiology of disc abalone, Haliotis discus discus. J. Fish Pathol. 22, 293–303.
Kurihara, T. (2017). Tolerance of the bivalve Trapezium liratum (Reeve, 1843) to decrease in salinity. Plankton Benthos Res. 12, 44–52. doi: 10.3800/pbr.12.44
Li, L., and He, L. (2016). Effects of water temperature and salinity on oxygen consumption rate of Cuneopsis pisciculus with different specifications. Hebei fisheries 2, 13–15.
Li, Y. (2018). Physiological Response of Ark Shell Scapharca broughtonii to Cu2+ and Ocean Acidification Stress. Shanghai: Shanghai Ocean University.
Li, Z., and Langhans, S. A. (2015). Transcriptional regulators of Na,K-ATPase subunits. Front. Cell. Dev. Biol. 3:66. doi: 10.3389/fcell.2015.00066
Liao, Y. (2016). Effects of Abrupt Salinity on Physiological Factors of Epinephelus moara. Master thesis. Shanghai: Shanghai Ocean University.
Lin, C. H., Yeh, P. L., and Lee, T. H. (2021). Time-course changes in the regulation of ions and amino acids in the hard clam Meretrix lusoria upon lower salinity challenge. J. Exp. Zool. A Ecol. Integr. Physiol. 335, 602–613. doi: 10.1002/jez.2503
Liu, J., Yu, D., and Li, J. (2011). Effects of salinity and pH on oxygen consumption and ammonia excretion rates in Pinctada fucata. Oceanol. Limnol. Sin. 42, 603–607.
Liu, Y. (2018). Preliminary Study on the Molecular Mechanism of Low Temperature Adaptability of Pinctada fucata martensii. Zhanjiang: Guangdong Ocean University.
Mahmood, N., Hameed, A., and Hussain, T. (2020). Vitamin E and selenium treatment alleviates saline environment-induced oxidative stress through enhanced antioxidants and growth performance in suckling kids of beetal goats. Oxid. Med. Cell. Longev. 2020:4960507. doi: 10.1155/2020/4960507
Manduzio, H., Rocher, B., Durand, F., Galap, C., and Leboulenger, F. (2005). The point about oxidative stress in molluscs. Invertebr. Surviv. J. 2, 91–104.
Marqueze, A., Carlos Kucharski, L., and Da Silva, R. S. M. (2006). Effects of anoxia and post-anoxia recovery on carbohydrate metabolism in the jaw muscle of the crab Chasmagnathus granulatus maintained on carbohydrate-rich or high-protein diets. J. Exp. Mar. Biol. Ecol. 332, 198–205. doi: 10.1016/j.jembe.2005.11.009
Morth, J. P., Pedersen, B. P., Buch-Pedersen, M. J., Andersen, J. P., Vilsen, B., Palmgren, M. G., et al. (2011). A structural overview of the plasma membrane Na+,K+-ATPase and H+-ATPase ion pumps. Nat. Rev. Mol. Cell Biol. 12, 60–70. doi: 10.1038/nrm3031
Natochin, Y. V., Berger, V. Y., Khlebovich, V. V., Lavrova, E. A., and Michailova, O. Y. (1979). The participation of electrolytes in adaptation mechanisms of intertidal molluscs’ cells to altered salinity. Comp. Biochem. Physiol. A Physiol. 63, 115–119. doi: 10.1016/0300-9629(79)90636-4
Nie, H., Chen, P., Huo, Z., Chen, Y., Hou, X., Yang, F., et al. (2017). Effects of temperature and salinity on oxygen consumption and ammonia excretion in different colour strains of the Manila clam, Ruditapes philippinarum. Aquaculture Research 48, 2778–2786. doi: 10.1111/are.13111
Nie, H., Jahan, K., Zhang, W., Huo, Z., and Yan, X. (2020). Physiological and biochemical responses of different shell color strains of Manila clam to low salinity challenges. Aquac. Rep. 16:100260. doi: 10.1016/j.aqrep.2019.100260
Nie, H., Zuo, S., Li, L., Tian, C., Cao, C., and Yan, X. (2018). Physiological and biochemical responses of Dosinia corrugata to different thermal and salinity stressors. J. Exp. Zool. A Ecol. Integr. Physiol. 329, 15–22. doi: 10.1002/jez.2152
Paital, B., and Chainy, G. B. N. (2010). Antioxidant defenses and oxidative stress parameters in tissues of mud crab (Scylla serrata) with reference to changing salinity. Comp. Biochem. Physiol. C Toxicol. Pharmacol. 151, 142–151. doi: 10.1016/j.cbpc.2009.09.007
Pan, X., Liu, H., Xu, M., Xu, H., Zhang, H., and He, M. (2020). Cloning and expression analysis of aquaporin gene AQP4 cDNA from Pinctada fucata martensii. Trop. Oceanogr 39, 66–75.
Pierce, S. K. (1971). Volume regulation and valve movements by marine mussels. Comp. Biochem. Physiol. A Physiol. 39, 103–117. doi: 10.1016/0300-9629(71)90350-1
Pilar, M., José, M., and Angeles, E. M. (2006). Phenoloxidase activity in three commercial bivalve species. Changes due to natural infestation with Perkinsus atlanticus. Fish Shellfish Immunol. 20, 12–19. doi: 10.1016/j.fsi.2005.02.002
Pourmozaffar, S., Jahromi, S. T., Rameshi, H., Sadeghi, A., Bagheri, T., Behzadi, S., et al. (2020). The role of salinity in physiological responses of bivalves. Rev. Aquac. 12, 1548–1566. doi: 10.1111/raq.12397
Ruangsri, J., Thawonsuwan, J., Wanlem, S., and Withyachumnarnkul, B. (2018). Effect of body size and sub-optimal water quality on some hemato-immunological parameters of spotted babylon snail Babylonia areolata. Fish. Sci. 84, 513–522. doi: 10.1007/s12562-018-1191-8
Selven, S., and Philip, R. (2013). Salinity a significant environmental factor for Vibrio harveyi virulence in Fenneropenaeus indicus. Aquac. Res. 44, 747–759. doi: 10.1111/j.1365-2109.2011.03080.x
Sergievskii, S., and Berger, V. J. (1984). Physiological differences of principal shell-colour phenotypes of the gastropod mollusc Littorina obtusata. Biol. Moria 2, 36–44.
Shi, Z., Liao, Y., Wang, X., Zhang, C., Peng, S., and Gao, Q. (2017). Impact of the abrupt salinity decrease on ion-regulation enzyme activity in the gill and serum osmolality from Epinehelus moara. J. Saf. Environ. 17, 1210–1214. doi: 10.13637/j.issn.1009-6094.2017.03.074
Shumway, S. E. (1977). Effect of salinity fluctuation on the osmotic pressure and Na+, Ca2+ and Mg2+ ion concentrations in the hemolymph of bivalve molluscs. Mar. Biol. 41, 153–177. doi: 10.1007/BF00394023
Sun, C. (2018). Study on the Compound Breeding of the Shell Color and Growth Traits of Hyriopsis cumingii “Shenzi No.1”. Master thesis. Shanghai: Shanghai Ocean University.
Sun, J., Chen, M., Fu, Z., Yang, J., Zhou, S., Yu, G., et al. (2021). A comparative study on low and high salinity tolerance of two strains of Pinctada fucata. Front. Mar. Sci. 8:704907. doi: 10.3389/fmars.2021.704907
Tian, L. (2019). Effects of Salinity on Growth, Nonspecific Immunity and Micro-Organism of Nibea albiflora. Master thesis. Zhoushan: Zhejiang Ocean University.
Veiga, M. P. T., Gutierre, S. M. M., Castellano, G. C., and Freire, C. A. (2016). Tolerance of high and low salinity in the intertidal gastropod Stramonita brasiliensis (Muricidae): behaviour and maintenance of tissue water content. J. Molluscan Stud. 82, 154–160. doi: 10.1093/mollus/eyv044
Wang, Q.-F., Shen, W.-L., Liu, C., Mu, D.-L., Wu, X.-F., Guo, N.-G., et al. (2017). Effects of multi-environmental factors on physiological and biochemical responses of large yellow croaker, Larimichthys crocea. Chemosphere 184, 907–915. doi: 10.1016/j.chemosphere.2017.06.043
Wei, D., and Ma, J. (2001). The investigation of comparison between contents of myoglobin and activity of lactate dehydrogenase in heart musule and skeletal Musule of plateau zokor and mouse. J. Qinghai Univ. 2, 20–21.
Widdows, J. (1985). “The effects of fluctuating and abrupt changes in salinity on the performance of Mytilus edulis,” in Marine Biology of Polar Regions and Effects of Stress on Marine Organisms, eds J. S. Gray and M. E. Christiansen (Chichester: John Wiley & Sons Ltd.).
Willmer, P. G. (1978). Volume regulation and solute balance in the nervous tissue of an osmoconforming bivalve (Mytilus edulis). J. Exp. Biol. 77, 157–179. doi: 10.1242/jeb.77.1.157
Yamaguchi, M., and Soga, K. (2020). Hemolymph composition, gene expressions in the gills, and thus the survival of euryhaline crabs are controlled by ambient minor cations according to osmotic condition-dependent manner. Ecol. Evol. 10, 12183–12199. doi: 10.1002/ece3.6846
Yang, C., Hao, R., Deng, Y., Liao, Y., Wang, Q., Sun, R., et al. (2017). Effects of protein sources on growth, immunity and antioxidant capacity of juvenile pearl oyster Pinctada fucata martensii. Fish Shellfish Immunol. 67, 411–418. doi: 10.1016/j.fsi.2017.06.037
Yang, J. (2016). Effects of Salinity and pH on Energy Metabolism and Gene Expression of Meretrix meretrix. Shanghai: Shanghai Ocean University.
Yang, S., Zhong, J. R., Zhao, L. L., Wu, H., Du, Z. J., Liu, Q., et al. (2018). The salinity tolerance of the invasive golden apple snail (Pomacea canaliculata). Molluscan Res. 38, 90–98. doi: 10.1080/13235818.2017.1386260
Yang, S. J., and Min, B. H. (2019). Sub-optimal or reduction in temperature and salinity decrease antioxidant activity and cellularity in the hemolymph of the Pacific abalone (Haliotis discus hannai). Fish Shellfish Immunol. 84, 485–490. doi: 10.1016/j.fsi.2018.10.041
Zhan, W., Zhou, S., Yuan, C., and Cui, Q. (2021). Effect of salinity on lymphocytes phagocytosis and expression of immune-related factor genes in Pacific white leg shrimp Litopenaeus vannamei. Chin. J. Fish. 34, 18–22.
Zhao, C., Guo, H., Zhang, J., Zhu, K., Guo, L., Zhang, N., et al. (2018). Molecular characteristics and expression analysis of AQP1a from Trachinotus ovatus under acute salinity stress. South China Fish. Sci. 14, 56–65.
Keywords: Pinctada fucata, salinity stress, osmotic regulation, respiratory metabolism, immune, antioxidant
Citation: Yang J, Yang J, Chen M, Fu Z, Sun J, Yu G, Wang A, Ma Z and Gu Z (2022) Physical Responses of Pinctada fucata to Salinity Stress. Front. Mar. Sci. 8:792179. doi: 10.3389/fmars.2021.792179
Received: 09 October 2021; Accepted: 17 November 2021;
Published: 03 January 2022.
Edited by:
Pengzhi Qi, Zhejiang Ocean University, ChinaReviewed by:
Sajjad Pourmozaffar, Iranian Fisheries Research Organization, IranHon Jung Liew, Universiti Malaysia Terengganu, Malaysia
You-Ting Zhu, Shanghai Ocean University, China
Copyright © 2022 Yang, Yang, Chen, Fu, Sun, Yu, Wang, Ma and Gu. This is an open-access article distributed under the terms of the Creative Commons Attribution License (CC BY). The use, distribution or reproduction in other forums is permitted, provided the original author(s) and the copyright owner(s) are credited and that the original publication in this journal is cited, in accordance with accepted academic practice. No use, distribution or reproduction is permitted which does not comply with these terms.
*Correspondence: Zhenhua Ma, emhlbmh1YS5tYUBzY3NmcmkuYWMuY24=; Zhifeng Gu, aG51Z3VAMTYzLmNvbQ==
†These authors have contributed equally to this work