- CCMAR – Center of Marine Sciences, University of Algarve, Faro, Portugal
Recently, increased attention is being paid to the importance of environmental history in species’ responses to climate-change related stressors, as more variable and heterogeneous environments are expected to select for higher levels of plasticity in species tolerance traits, compared to stable conditions. For example, organisms inhabiting environments with highly fluctuating thermal regimes might be less susceptible to the increasing frequency and intensity of marine heatwaves (MHWs). In this study, we assessed the metabolic and calcification responses of the rhodolith-bed forming Phymatolithon lusitanicum, from a coastal region that is strongly influenced by frequent changes between upwelling and downwelling conditions, to a simulated MHW scenario, with and without prior exposure to a moderate thermal stress. This allowed determining not only the influence of the species’ long-term thermal history on its resilience against MHWs, but also the rhodoliths capacity for short-term thermal stress memory and its importance during posterior MHW-exposure. Our findings indicate that the rhodoliths experienced negative impacts on daily net primary production (DNP) and calcification (DNC) during the MHW. The effect on the former was only temporary at the beginning of the MHW, while DNC was highly impacted, but exhibited a quick recovery after the event, suggesting a high resilience of the species. Furthermore, prior exposure to a moderate temperature increase, such as those occurring frequently in the natural habitat of the species, mitigated the effects of a subsequent MHW on DNP, while promoting a faster recovery of DNC after the event. Thus, our findings (1) support the hypothesis that benthic organisms living in nearshore habitats may benefit from the natural short-term temperature fluctuations in these environments with an increased resistance to MHW impacts and (2) provide first-time evidence for thermally induced stress memory in coralline algae.
Introduction
Benthic organisms that are sessile in nature cannot escape, but must respond to or endure fluctuating surroundings and diverse environmental challenges. These natural environmental regimes can shape the organisms’ stress tolerance through processes like acclimation and adaptation. Therefore, to understand whether particular marine ecosystems could be more resilient or resistant to stressors, namely those related to the ongoing climate change, a better working knowledge of the role played by local environmental variability in shaping the responses of benthic organisms is needed (Helmuth et al., 2006; Boyd et al., 2016; Rivest et al., 2017; Vargas et al., 2017; Kroeker et al., 2019; Bernhardt et al., 2020).
Natural environmental variability can essentially influence the responses of marine organisms to changing conditions, such as related to the ongoing climate change (Boyd et al., 2016), as (1) marine provinces differ strongly in their environmental heterogeneity (Zhao et al., 2020), (2) organisms from more heterogeneous environments may have an increased phenotypic plasticity (Vargas et al., 2017; Miller et al., 2020), and (3) the inclusion of natural environmental fluctuations in experimental climate-change simulations has been shown to yield different outcomes from those that do not (Oliver and Palumbi, 2011; Cornwall et al., 2013, 2018; Roleda et al., 2015; Schoepf et al., 2015; Britton et al., 2016; Johnson et al., 2021). In view of the latter, a common expectation for studies, examining the effects of climate change-related stressors, is that environmental heterogeneity and variability will select for higher levels of plasticity in specific tolerance traits compared to stable environments. Significant progress has been made to include naturally high pH variability in experimental designs studying ocean acidification effects on marine organisms, such as corals, coralline, and other macroalgae (Cornwall et al., 2013, 2018; Johnson et al., 2014, 2021; Roleda et al., 2015; Britton et al., 2016). Similarly, the effects of highly fluctuating thermal regimes on organisms’ thermotolerance received increased attention in recent years (Putnam et al., 2010; Oliver and Palumbi, 2011; Schoepf et al., 2015; Safaie et al., 2018; Hughes et al., 2019; McCoy and Widdicombe, 2019).
Studying the thermal responses of organisms and the influence that natural variability may have on those has become increasingly important in marine research, as current climate change projections predict an increase in seawater temperature, occurring through both gradual warming and also by the increase in events of anomalous high seawater temperature, such as marine heatwaves (MHWs). The latter represent events of changes in temperature within days and are predicted to become more frequent and intense in the future (Meehl and Tebaldi, 2004; Frölicher and Laufkötter, 2018; Oliver et al., 2018), driving alterations at the ecosystem scale, due to likely changes in the abundance, distribution and function of foundation species (e.g., Pearce and Feng, 2013; Wernberg et al., 2013). The plasticity in thermotolerance traits that may be promoted by naturally fluctuating thermal regimes will be crucial for organisms’ resilience and survival in the absence of time for evolutionary responses (Safaie et al., 2018; Hughes et al., 2019). Environments that exhibit strong and fast (daily, weekly) temperature fluctuations might not only promote thermotolerance through adaptation, but also through the induction of thermal stress memory (thermal priming).
Thermal priming or acquired thermotolerance, already well-studied in terrestrial plants, allows organisms to cope better under conditions of recurrent heat stress through exposure to prior stress events (Hilker et al., 2016). It is distinguished from the basal thermotolerance, i.e., an organism’s innate capacity to cope with heat stress, as it is induced either by exposure to a gradual temperature increase up to stressfully high temperatures (e.g., seasonal temperature fluctuations) or via a short pre-exposure to a mild heat stress. In the latter case, upon returning to non-stress temperatures, the primed state (referred to as heat stress memory) is maintained over several days or months (Iqbal and Ashraf, 2007; Rendina González et al., 2018). This phenomenon of thermal priming has very recently been adopted as a potential strategy for ecosystem restoration and macroalgal farming (Jueterbock et al., 2021). It is also a currently emerging field in marine climate change research, due to evidence of lower impacts and higher resilience of communities living in fluctuating thermal environments and/or experiencing recurrent stress events, compared to those from stable environments. So far, only a few marine studies on this topic were conducted in macroalgae, seagrasses or corals (Kishimoto et al., 2019; Nguyen et al., 2020; Yu et al., 2020).
Studies regarding the influence of thermally fluctuating environments on the response to temperature rise in coralline algae, a group of benthic organisms that has been shown to experience often negative impacts related to seawater temperature increase (Martin and Hall-Spencer, 2017; Cornwall et al., 2019), and their potential capacity for thermal priming are currently missing. These algae fulfill crucial ecological roles throughout the oceans, such as building extensive, worldwide distributed coastal ecosystems, the rhodolith beds (Foster, 2001; Riosmena-Rodríguez et al., 2017), or cementing and providing substrate for invertebrate larval settlement in coral reefs (e.g., Adey, 1978; Steneck, 1986; Littler and Littler, 2013). Though, in contrast to other ecosystems (e.g., coral reefs, kelp forests) still little information is available regarding their response to increased seawater temperature. Most studies have focused on determining the effects of gradual increases in mean temperature and report a broad range of responses (Martin and Hall-Spencer, 2017; Cornwall et al., 2019). The tolerance or susceptibility of coralline algae to MHWs is so far largely unknown and the scarce ecological information available indicates highly variable responses. For example, increased coralline algal mortality was reported after a thermal stress event in Western Australia (Short et al., 2015), while other studies reported their increased abundance during extreme heat stress associated to El Nino events (Murray and Horn, 1989; López-Pérez et al., 2016). Furthermore, as pointed out in the review of Cornwall et al. (2019), besides lacking information regarding the influence of MHWs on coralline algal physiology, also the role that naturally variable environments and hence, species’ past thermal history, could play in harboring more thermotolerant populations of coralline algae is largely unknown. Recent evidence showed none or little physiological responses of articulated coralline algae to simulated MHWs (Rendina et al., 2019; Ragazzola et al., 2021), while subtropical rhodolith species suffered strong negative effects (Schubert et al., 2019), and McCoy and Widdicombe (2019) reported that environmental history did not define the thermal response of another articulated alga, the intertidal Ellisolandia elongata.
In the present study the impacts of MHWs on a free-living coralline alga were investigated. Free-living coralline algae or rhodoliths are common foundation species (also named bioengineer, for their ecological role in structuring ecosystems) in the intertidal/shallow subtidal regions of temperate, subtropical and tropical coasts around the world (Foster, 2001; Riosmena-Rodríguez et al., 2017). The ecosystems built by these organisms, the rhodolith (or maërl) beds, are recognized biodiversity hot-spots and considered as critical marine habitats for conservation in several areas of the world (Riosmena-Rodríguez et al., 2017). Also, they are acknowledged as major carbonate factories that may contribute more prominently to the global CaCO3 budget than currently acknowledged (Nelson, 2009; Amado-Filho et al., 2012; van der Heijden and Kamenos, 2015; Smith and Mackenzie, 2016). Thus, in the face of climate change, the resilience and persistence of these organisms and the habitats they build are of particular interest and importance.
The newly described rhodolith species Phymatolithon lusitanicum (Pardo et al., 2014; Peña et al., 2015) studied here has a wide latitudinal distribution, from Scotland and Ireland to the Iberian Peninsula and the Western Mediterranean Sea, and dominate the rhodolith beds in the Iberian Peninsula, specifically in Galicia (NW Spain) and Algarve (South Portugal) (Carro et al., 2014; Peña et al., 2015; Bunker et al., 2018). The latter location is defined by fast inversions between upwelling and downwelling conditions, exposing the rhodolith community to a highly fluctuating thermal regime and allowing the assessment of (1) the influence of the long-term thermal history of P. lusitanicum on the species’ physiological response to MHWs, and (2) the species’ capacity for short-term thermal stress memory and its importance during posterior MHW-exposure.
Materials and Methods
Study Site and Historic Environmental Conditions
The studied rhodolith bed, dominated almost exclusively by Phymatolithon lusitanicum (Carro et al., 2014; Pardo et al., 2014; Peña et al., 2015), has an estimated area of 3 km2 and extends from 13 to 25 m depth. It is located at 4.7 nautical miles offshore of Armação de Pêra, in the Algarve, Southern Portugal (37°01′N, 8°19′W; Figure 1A). The region is influenced by coastal counter currents due to opposite alongshore winds that cause sharp current inversions in short time scales (<2–15 days), resulting in changes from upwelling- to downwelling-favorable conditions, especially during May-Oct (Sánchez et al., 2006; Garel et al., 2016). As shown by in situ data, these quick changes in coastal currents cause strong daily and weekly fluctuations in temperature and light availability at the location of the rhodolith bed (Figures 1B–D), but also strong temperature fluctuations along the day, with recorded variations of up to 3–4°C (Supplementary Figures 1A–C). The temperature variation is accompanied by a large variability of maximum in situ daily light intensities that range between no detectable light to maximum recorded values of 240 μmol quanta m–2 s–1 (Supplementary Figure 1D).
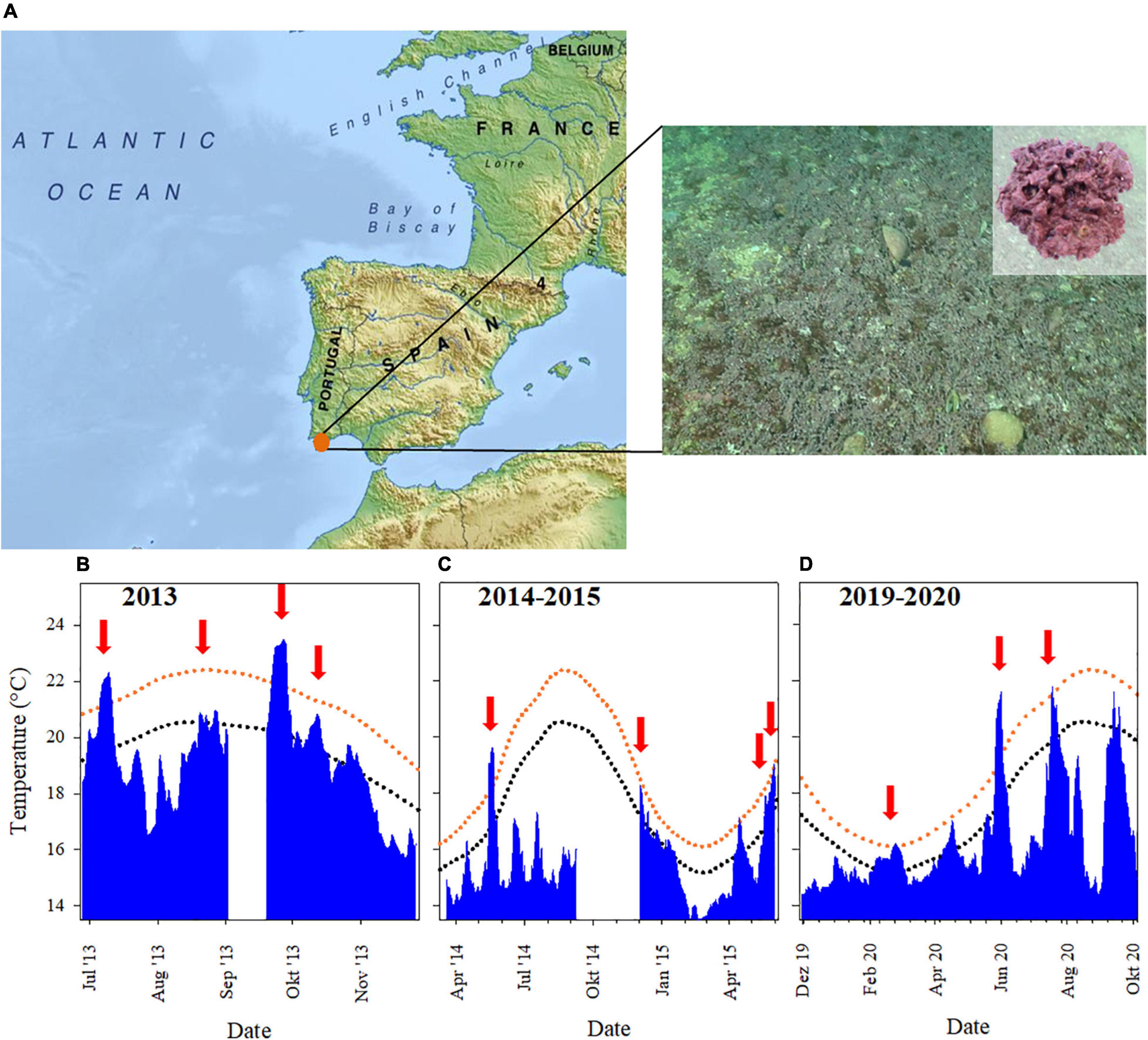
Figure 1. (A) Rhodolith bed off the coast of Armação de Pera, Portugal (orange dot; https://mapswire.com/), dominated by Phymatolithon lusitanicum (inlet photo). (B–D) Daily in situ maximum seawater temperature between 2013 and 2020 (blue area), recorded by HOBO temperature data loggers (Onset, United States) positioned at the rhodolith bed at 22 m depth. Climatological daily mean (dotted dark blue line) and threshold SST temperature (dotted orange line) for the location are shown and MHW events, as defined by the Marine Heatwave Tracker (Schlegel, 2018), are indicated by red arrows.
Climatological data from 1982 to 2020, based on satellite data of sea surface temperature (SST) retrieved from the Marine Heatwave Tracker (Schlegel, 2018) show that the southern Portuguese region experiences the influence of marine heatwaves (MHWs). Our analysis of the MHWs detected since 1982 indicate that not only the frequency, but also the severity of these events exhibits an increasing trend (Supplementary Figure 2A). In the 2010’s decade, 31 MHW events were recorded, 7 of which are considered strong events, with maximum temperature rises above the climatological threshold ranging between 1.1 and 4.3°C. The occurrence of MHWs shows a seasonal variability, with the majority of the events occurring during summer and autumn (Supplementary Figure 2B). Most of the MHWs are of short duration (<2 weeks; Supplementary Figure 2C), though there is a large variability regarding the time passed between events. Often 1–4 weeks pass between events, while longer time intervals (>11 weeks) are also quite frequent (Supplementary Figure 2D). These occurrences of thermally anomalous events, which are identified based on SST data, are consistent with strong in situ temperature increases (Figures 1B–D).
Sampling and Experimental Design
Individuals of P. lusitanicum were collected by SCUBA at 22 m depth in October 2020 (recorded in situ temperature: 17°C) and transported in coolers to the Center for Marine Sciences marine station Ramalhete (∼50 km from Armação de Pêra). Here, the rhodolith were maintained in seawater flow-through indoor tanks at 17°C and 50 μmol quanta m–2 s–1 (11 h light: 13 h dark; LEDVANCE Flood LED IP65, 50 W, 6500 K, Ledvance, Germany) during 2 weeks for pre-acclimation to the tank conditions. The chosen light intensity represents an intermediate maximum light level, based on previous records of natural light conditions at the location of the rhodolith bed (Supplementary Figure 1D).
To test for potential impacts of MHWs (sensu Hobday et al., 2016) on rhodolith physiology and the influence of recurrent thermal stress on those impacts, three different treatments were designed, (1) Control- kept at 17°C during the whole experiment (Figure 2A), (2) HT- simulation of a high-temperature event for 12 days, with a gradual temperature increase/decrease of 1.5°C day–1 and Tmax = 24.5°C for 4 days (Figure 2B). With 3.5°C above the climatological threshold of the sampling location and month (Oct- 21.2°C), this treatment simulated a MHW lasting 8 days. And (3) 2HT- simulation of two high-temperature events: the 1st event represented a moderately naturally occurring temperature increase during 12 days (max. 21°C for 6 days, gradual temperature increase/decrease: 1°C day–1), while the 2nd event simulated the same high-temperature event as in HT (Figure 2C).
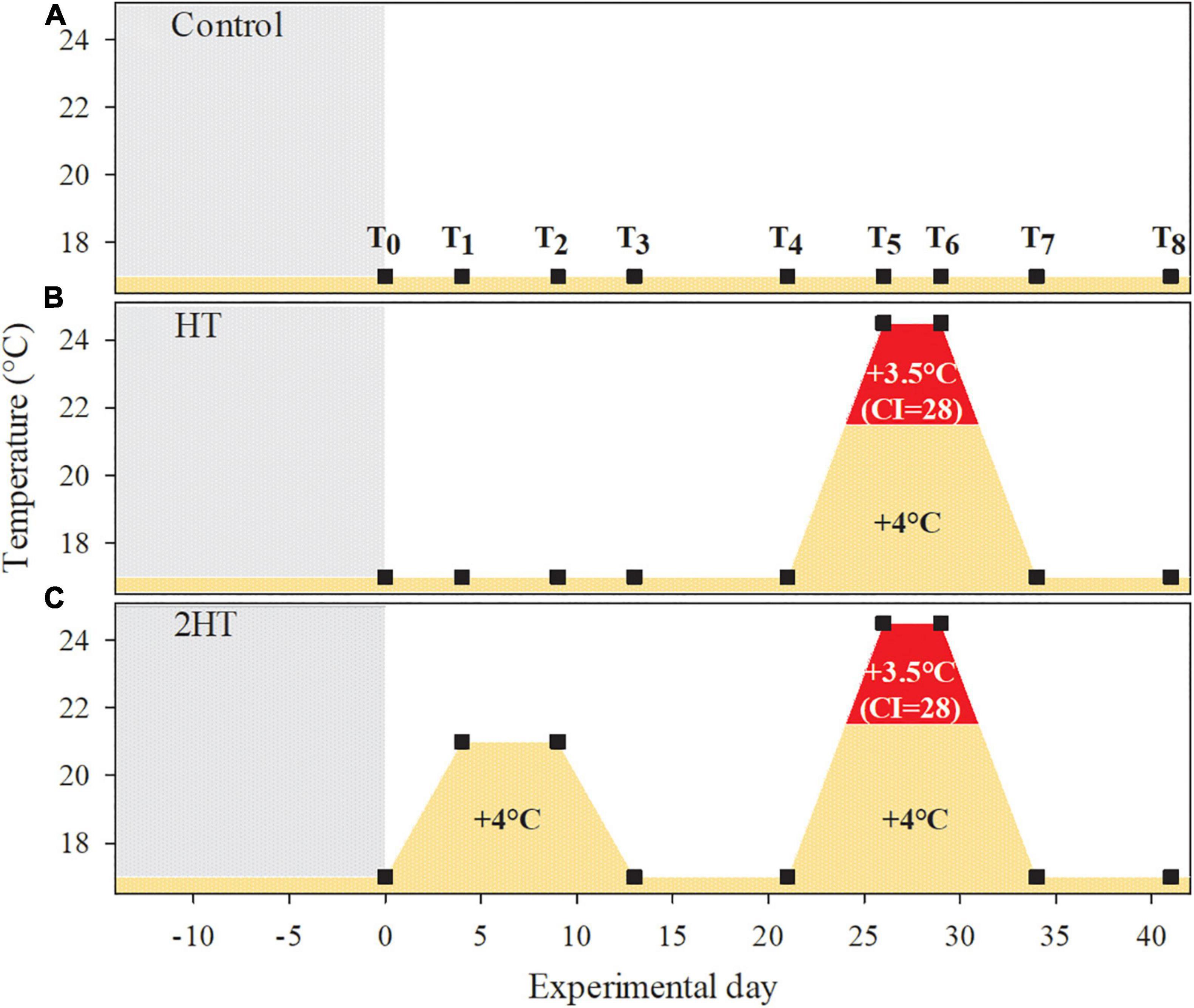
Figure 2. Schematic overview of the temperature profiles and daily average temperature in the different experimental treatments: (A) control treatment at 17°C, (B) HT – exposure to one high-temperature event, simulating a realistic MHW scenario, and (C) 2HT – exposure to two consecutive high-temperature events of different intensities, the 1st moderate event simulating a natural temperature fluctuation and the 2nd event simulating a realistic MHW scenario. The pre-acclimation period is indicated by the shaded area and the maximum temperature increase in the treatments and the cumulative intensity (CI = Duration*Mean Intensity) of the simulated MHW are given. The red area indicates the treatment conditions that correspond to a MHW event, with exposure to temperatures above the climatological threshold for the sampling location and period (>21.2°C) for >5 days (sensu Hobday et al., 2016). The time points at which physiological measurements were performed (T0–8) are indicated by black squares.
During the experiment, the rhodoliths were kept in a set-up consisting of 150–L treatment tanks (n = 5 per temperature treatment), connected to a seawater flow-through system. Before entering the experimental tanks, the seawater was pumped into a header tank (2000 L), in which the temperature was kept at 17°C by a heat pump (i-Komfort, Kripsol, Hayward), after passing through a preliminary mechanical filtration (sand filters), two in-line cartridge filters (10–20 μm and 5 μm) and two UV filters (16 and 8 W). From there, the water entered the experimental tanks, each equipped with a submersible air pump to ensure water circulation and an aquarium heater that was used to maintain and manipulate the temperature in the different treatments, which was monitored by a temperature data logger (HOBO, Onset, United States) positioned in each tank (Supplementary Figure 3).
To determine the treatment effects on rhodolith physiology, the responses in photosynthetic, respiratory and calcification rates of the same rhodoliths (n = 5 per treatment, n = 1 per tank) were monitored over the entire experimental period. For this purpose, short-term incubations of the individuals were performed at nine different time points, including measurements at the beginning of the experiment, during and in-between the different high-temperature event simulations and after a recovery period of 7 days (Figure 2).
Physiological Measurements
Individual rhodoliths were incubated (n = 5 per treatment and time point) at their respective treatment temperature with filtered seawater (0.45 μm) in sealed custom-made chambers (V = 70 mL) with internal circulation, provided by a magnetic stirrer. Firstly, the rhodoliths were exposed for 1 h to 50 μmol quanta m–2 s–1 (Lexman LED, 20 W, 6500 K) to determine photosynthetic and light calcification rates and subsequently, they were incubated in darkness for another hour to determine respiratory and dark calcification rates. Previous tests assured that 1 h-incubation time provides a high enough signal to noise ratio to allow detection of changes in both oxygen concentration and alkalinity, while ensuring that pH changes during incubations were <0.1 units. At the beginning and end of the incubation, the oxygen concentration was measured with an oxygen meter (Fibox 4, PreSens, Germany). Control incubations (without rhodolith samples) were also performed, showing that oxygen changes in the incubations water, e.g., due to microbial activity, were neglectable. Seawater samples were taken at the beginning and end of every incubation, poisoned with HgCl2 and stored in borosilicate tubes (two tubes per incubation chamber, V = 25 mL each) for later estimation of calcification rates. At the end of the experiment the rhodoliths were oven-dried for 48 h at 60°C and the estimated dry weight was used to normalize metabolic and calcification rates.
The calcification rates of the rhodoliths were determined from alkalinity measurements of seawater samples before and after the different incubations, using the alkalinity anomaly principle based on the ratio of two equivalents of total alkalinity per each mole of CaCO3 precipitation (Smith and Kinsey, 1978). For alkalinity (TA) measurements, duplicate analyses of each sample were performed, using the Gran titration method (Hansson and Jagner, 1973; Bradshaw et al., 1981). The samples were titrated with HCl 0.1 M, using an automated titration system (Titroline 7000, SI Analytics, Mainz, Germany), coupled to an autosampler (TW alpha plus, SI Analytics, Mainz, Germany). Data were captured and processed with a computer, using Titrisoft 3.2 software (SI Analytics, Mainz, Germany). For quality control, a certified reference material of known total alkalinity was used to calibrate the method (CRMs, Batch No. 160; supplied by the Marine Physical Laboratory, Scripps Institution of Oceanography, United States).
Statistical Analysis
During the experiment, the physiological responses of the same rhodolith individuals were tracked over time and thus, the data of every treatment were analyzed using one-way repeated measures ANOVA, with time as the within-subjects factor. In cases where significant differences were found, a Tukey’s HSD post hoc test was conducted to determine significant groupings. A priori, data were tested for normality and heteroscedasticity, using the Shapiro-Wilk and Levene’s tests, respectively. All statistical analyses were performed using STATISTICA 7.
Results
All measured physiological parameters of P. lusitanicum showed significant differences among time points in the treatments that either simulated one (HT) or two (2HT) transient high-temperature events (Table 1 and Supplementary Table 1). Unexpectedly, the rhodoliths kept under control conditions also showed significant differences for gross photosynthesis and consequently for daily net primary production (Table 1 and Figure 3A). This was coincident with a slight temporary increase in the treatment temperature (Supplementary Figure 3).
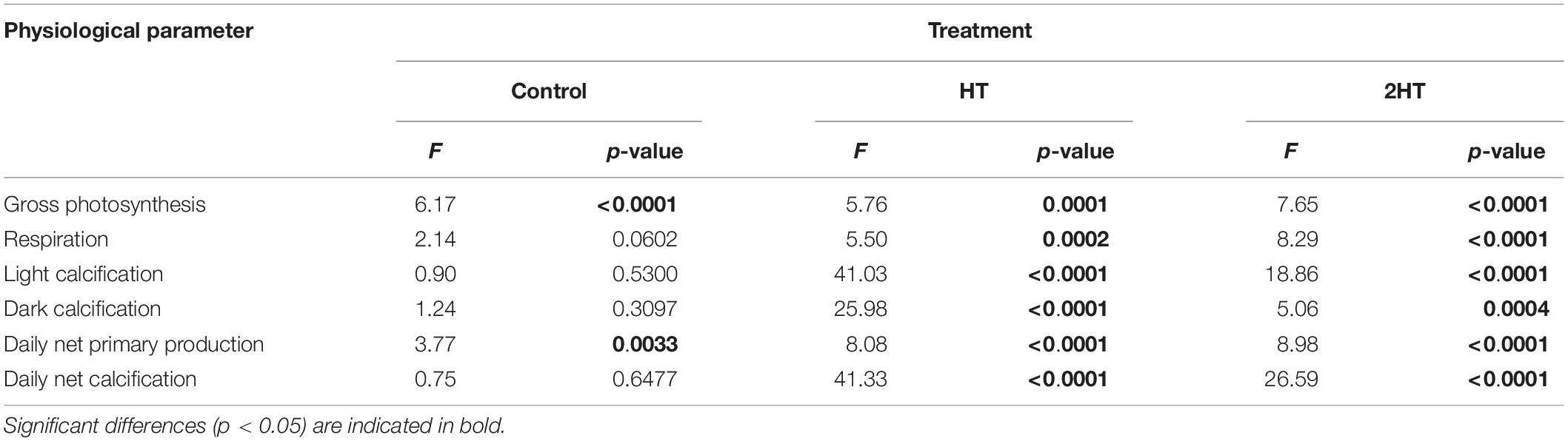
Table 1. Summary of the results of the one-way repeated measures ANOVA, indicating the effect of time (and corresponding temperature condition) on the measured parameters in each treatment.
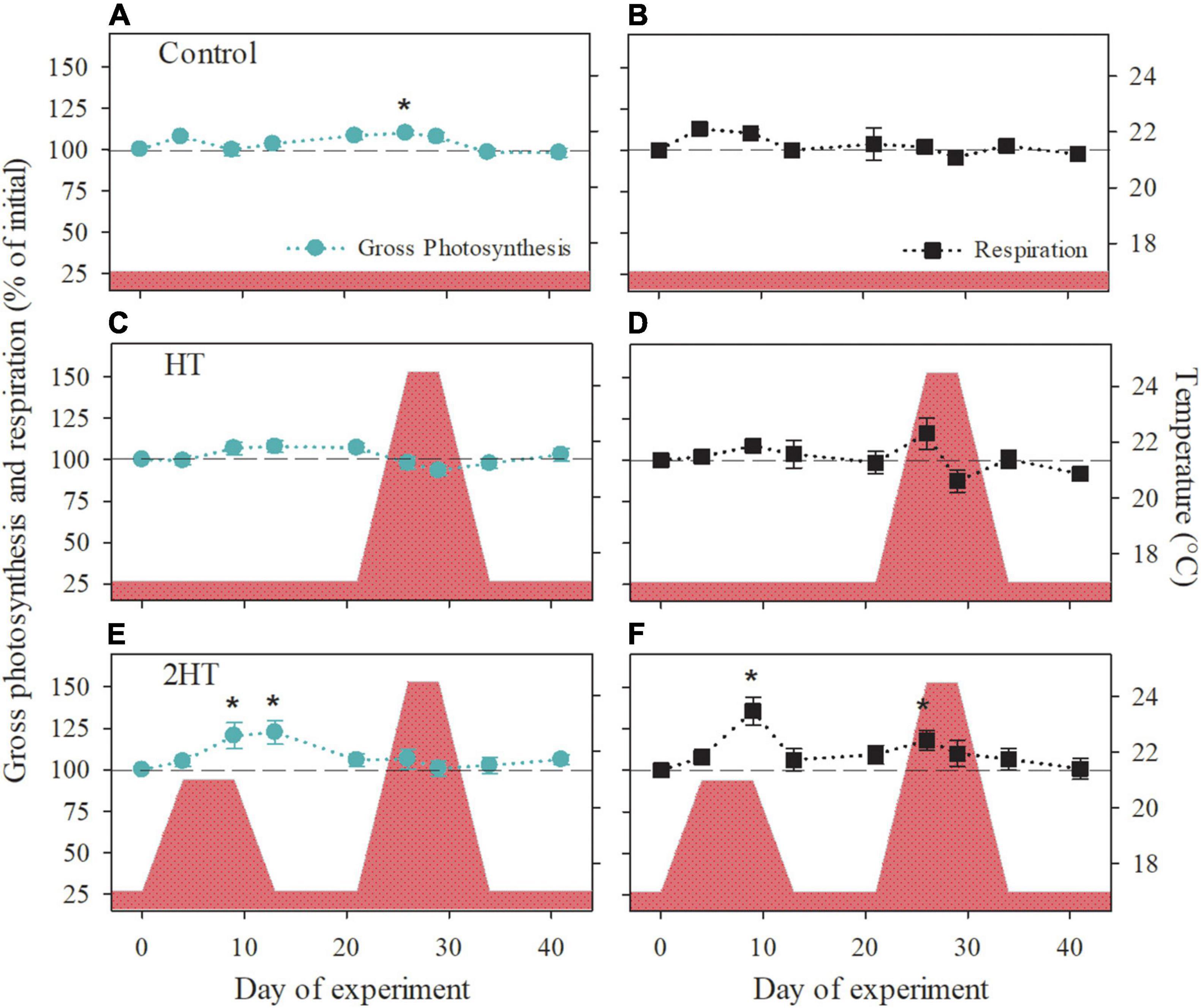
Figure 3. Metabolic rates of Phymatolithon lusitanicum in response to the experimental temperature treatments (indicated by the red area). (A,B) Control treatment, (C,D) HT-simulation of a high-temperature event, and (E,F) 2HT- simulation of two high-temperature events. Data are shown as percentage of the initial values and represent mean ± SE (n = 5 per treatment and time point). Significant changes with respect to the initial value are indicated by asterisks (one-way repeated measures ANOVA, Tukey’s HSD post hoc).
Rhodolith exposure to a temperature increase that simulated a MHW event (HT-treatment) did not induce a significant response in P. lusitanicum’s gross photosynthesis or respiratory demand, when compared to the respective initial values (Figures 3C,D). On the other hand, the individuals in the 2HT-treatment showed a significant increment in both gross photosynthetic and respiratory rates in response to the first moderate temperature increase, while the exposure to the subsequent MHW induced a significant increase only in respiration at T5, when maximum temperature was reached, an effect that had dissipated after 4 days at this temperature (T6; Figures 3E,F).
Rhodolith calcification showed positive, as well as negative responses to increasing temperatures, depending on the treatment (Figure 4). In the HT-treatment, rhodoliths experienced a strong significant decrease in both light and dark calcification after exposure for 4 days to the maximum temperature (T6), but recovered to 90% of their initial calcification after a week at pre-MHW temperature (T8; Figures 4C,D). In contrast, when exposed to a moderate temperature increase in the 2HT-treatment, rhodolith light and dark calcification was stimulated, which resulted in a significant increase in light calcification after 6 days at 21°C (T2; Figure 4E). This increase in light calcification was also observed initially at the subsequent MHW event (T5), but was here followed by a significant decrease after 4 days at the maximum temperature (T6). Dark calcification, on the other hand, declined as temperature increased during the subsequent MHW (T5), reaching values of 48% of T0 after 4 days at the maximum temperature (T6; Figure 4F). Afterward, in the 2HT-treatment both light and dark calcification showed complete recovery to initial light calcification rates after 4 days at pre-MHW temperature (T7; Figures 4E,F).
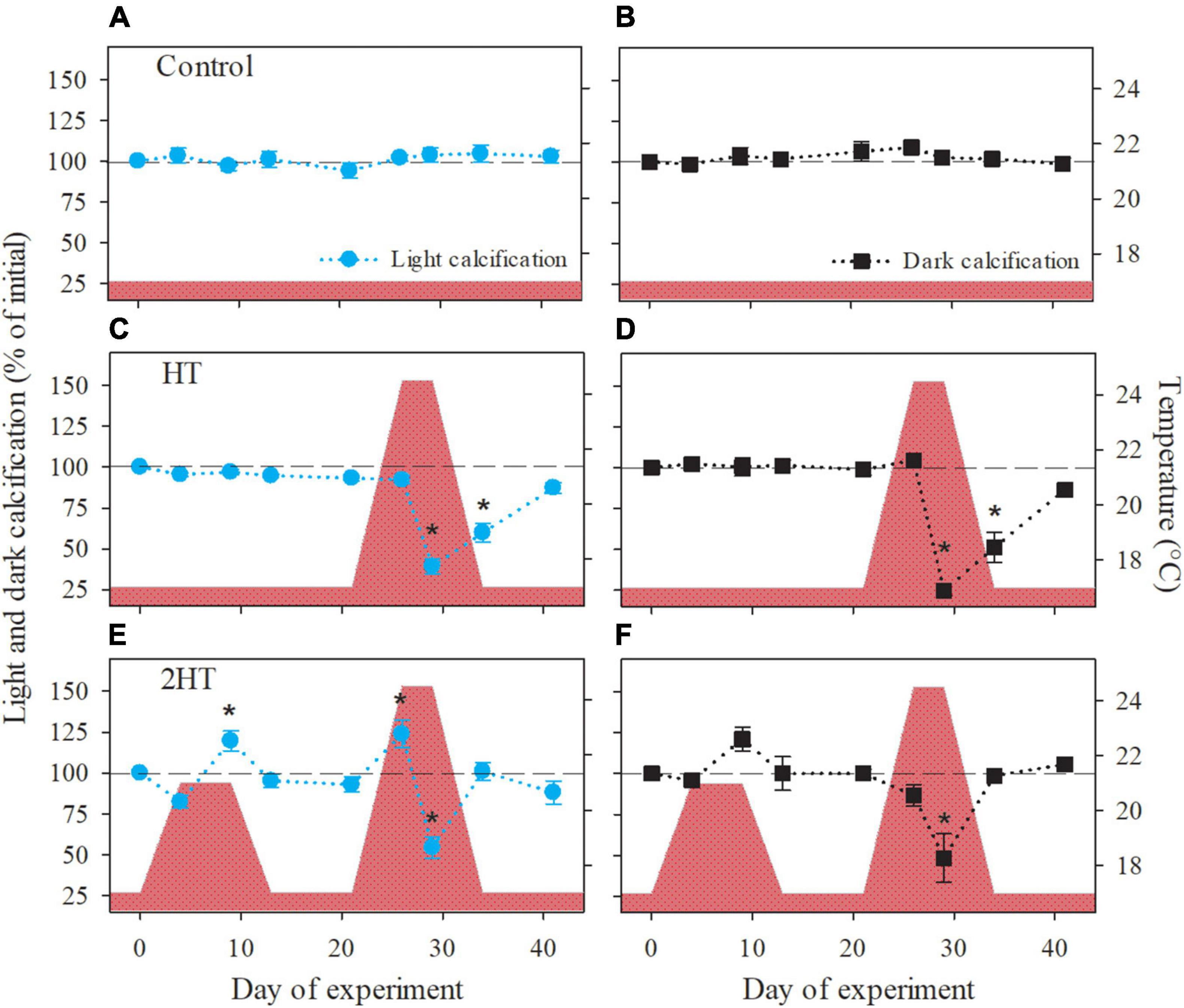
Figure 4. Light and dark calcification rates of Phymatolithon lusitanicum in response to the experimental temperature treatments (indicated by the red area). (A,B) Control treatment, (C,D) HT-simulation of a high-temperature event, and (E,F) 2HT- simulation of two high-temperature events. Data are shown as percentage of the initial values and represent mean ± SE (n = 5 per treatment and time point). Significant changes with respect to the initial value are indicated by asterisks (one-way repeated measures ANOVA, Tukey’s HSD post hoc).
The integration of the measured physiological responses, considering the daylight hours for the location at the time of the year in which the rhodoliths were collected and which were maintained during the experiment (11 h light: 13 h dark), indicated significant impacts of the exposure to a MHW event on daily net primary production (DNP) and daily net calcification (DNC). In the HT-treatment DNP decreased significantly at reaching the maximum temperature of the MHW (T5), with a subsequent increase to initial values after 4 days at this temperature (T6), following the pattern of the rhodoliths’ respiratory response to the temperature increase (Figure 5C). On the other hand, DNC in this treatment was initially unaffected by the temperature increase (T5), though after 4 days at 24.5°C (T6) it declined strongly (−60%, Figure 5D), matching the responses in both, light and dark calcification (Figures 4C,D). After the MHW, DNC showed a strong and fast recovery, reaching ∼90% of the initial values after 1 week at pre-MHW temperature (T8; Figure 5D). In contrast, rhodoliths in the 2HT-treatment that were firstly exposed to a moderate increment in temperature experienced a significant increase in DNP after the temperature was back to the control level (T3; Figure 5E), related to increased photosynthetic rates (Figure 4E). A similar, though not significant positive response to the moderate temperature increase was found also for DNC (T2; Figure 5F), related to the positive responses in both light and dark calcification (Figures 4E,F). Subsequently, at the beginning of the second, MHW-simulating temperature increase (T5), a significant increase in DNC was found, which declined significantly after longer exposure (4 days) to the maximum temperature (T6), but showed a quick and complete recovery by the end of the MHW event (T7; Figure 5F).
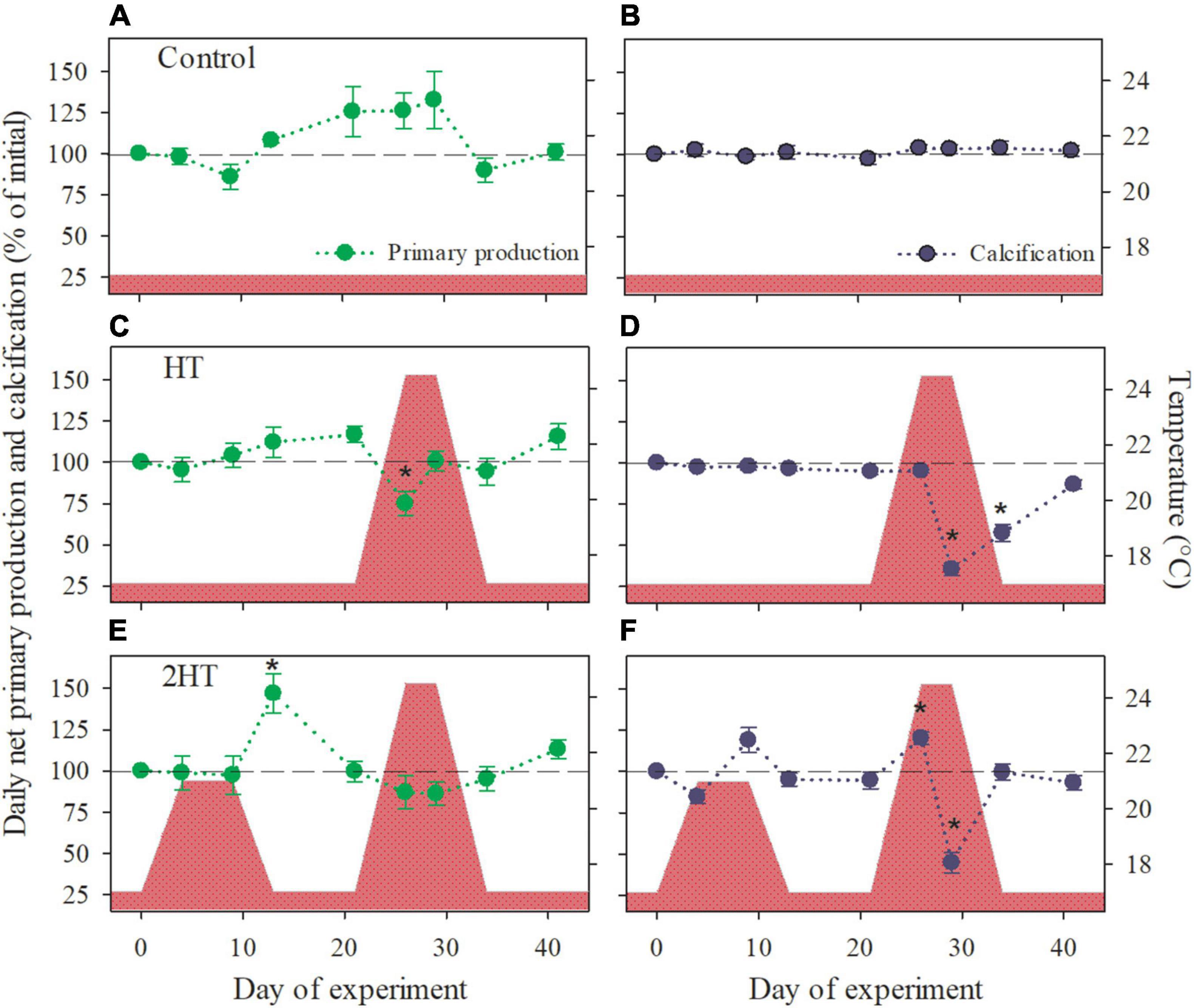
Figure 5. Daily integrated net primary production and calcification of Phymatolithon lusitanicum in response to the different thermal treatments (indicated by the red area). (A,B) Control treatment, (C,D) HT-simulation of a high-temperature event, and (E,F) 2HT- simulation of two high-temperature events. Data are shown as percentage of the initial values and represent mean ± SE (n = 5 per treatment and time point). Significant changes with respect to the initial value are indicated by asterisks (one-way repeated measures ANOVA, Tukey’s HSD post hoc).
Discussion
Our results demonstrate that a fluctuating environment pre-conditions the free-living coralline species P. lusitanicum to cope better with thermal stress, such as that experienced during MHWs. While the rhodoliths may experience some negative impacts on daily net primary production and calcification during a MHW event, the effect on DNP are rather short-lived and the larger impact on DNC is compensated by a high rate of recovery, suggesting a high resilience of the species. Moreover, events of prior exposure to moderate temperature increase, such as occurring frequently in the natural habitat of this species, due to fast changes between upwelling and downwelling conditions, mitigated the effects of a subsequent more intense MHW on DNP and promoted an even faster recovery of DNC. Thus, this study also provides the first insight into thermal stress-induced memory in coralline algae.
Marine Heatwave Impact on Rhodolith Physiology
Most studies on the physiological responses to temperature increase in coralline algae have been performed either by comparing their seasonal performance (i.e., winter vs. summer) or by assessing their responses to experimentally-induced temperature increases above a mean control or ambient temperature (Martin and Hall-Spencer, 2017; Cornwall et al., 2019). In contrast, information on coralline algal tolerance/susceptibility to MHW events is almost non-existent (but see Rendina et al., 2019; Ragazzola et al., 2021). Regardless of the approach, the available evidence suggests that coralline algae express a large variability in their responses to thermal stress, which may be related to species-specific differences, but also, as pointed out by Cornwall et al. (2019), to temperature variability within experimental set-ups and/or the past thermal history of the organisms.
In the present study, the acute thermal stress treatment, simulating a realistic MHW event (HT-treatment), showed little and only short-term negative effects on rhodolith net primary production, but a strong decrease in net calcification. The former was related to an initial, though not significant, increase in respiration, a response often found in coralline algae during exposure to higher temperatures (Steller et al., 2007; Vásquez-Elizondo and Enríquez, 2016). However, within a few days at the high temperature P. lusitanicum was able to adjust its metabolic demand, allowing the species to revert to the prior daily net primary production rates (Figure 5C). On the other hand, rhodolith calcification was strongly impacted by the MHW simulation, due to a decline in both light and dark calcification and consequently, daily net calcification (Figure 5D). The stronger impact on calcification, compared to the metabolic processes, has also been reported in other coralline algae (Vásquez-Elizondo and Enríquez, 2016; Schubert et al., 2019) and indicates an uncoupling of physiological processes, such as photosynthesis and light calcification under thermal stress conditions. On the other hand, the ∼40% recovery of daily net calcification at the time when temperature was back to the pre-MHW level suggests that recovery started already during the gradual temperature decrease at the end of the MHW. This, together with the 90% recovery 1 week after the event, indicates a high recovery capacity of P. lusitanicum and hence, species’ resilience in the face of MHW-associated heat stress, most likely related to an adaptive tolerance to the strong and fast temperature fluctuations of its natural habitat.
The influence of an organism’s thermal history on its response to acute thermal stress has recently been studied in coralline algae through prior pre-acclimation to higher or varying temperatures (naturally or experimentally) before exposure to severe heat stress. The experimental evidence indicates contradictory responses, either demonstrating reduced physiological performance (Rendina et al., 2019; Page et al., 2021) or no substantial effects on the studied species in response to acute heat stress (McCoy and Widdicombe, 2019), probably related to differences in the experimental design or the thermal history of the species. Experiments involving long-term acclimation of tropical and temperate species to an increase in mean temperature prior to acute thermal stress exposure reported negative effects (Rendina et al., 2019; Page et al., 2021), while an acute thermal stress did not exert any negative effects on individuals of the temperate species Ellisolandia elongata, collected from a strong intertidal gradient (McCoy and Widdicombe, 2019).
Direct comparison among studies regarding the responses of coralline algae to MHWs is very difficult due to (1) the currently extremely scarce number of studies, (2) the often large differences in the experimental design, e.g., MHW intensity and duration, and (3) the differences in the specific time points during the MHW (and after a recovery period), when physiological assessments were performed. To date, there are only two studies on temperate articulated coralline algae that have assessed the physiological responses to realistic scenarios of MHWs, based on climatological data and thresholds for the respective study location (Rendina et al., 2019; Ragazzola et al., 2021). In general terms, the lack of significant responses of P. lusitanicum’s metabolic rates to the MHW-associated temperature increase found here agrees with those observed in the temperate articulated coralline alga Ellisolandia elongata (Ragazzola et al., 2021), while a significant response has been reported in the articulated Corallina officinalis (Rendina et al., 2019). The latter species exhibited a decrease in gross photosynthesis, as well as respiratory demand immediately after a simulated MHW event, without signs of recovery after 2 weeks. Also, the calcification response of these two articulated coralline species differed greatly from that of the here studied rhodolith, as the former did not show any responses in either, light or dark calcification in response to a simulated MHW event or responded with a significant decline in dark calcification only (Rendina et al., 2019; Ragazzola et al., 2021). These contradictory findings are not surprising, considering the large variability in magnitude and direction of thermal stress responses in this group that in large part may be related to inherent species-specific differences (Martin and Hall-Spencer, 2017; Cornwall et al., 2019), also supported by a recent study showing that even different rhodolith species from the same location express differences in their responses to seasonal temperature variations (Qui-Minet et al., 2021).
Thermal Stress Memory
The comparison of the rhodolith responses upon exposure to a realistic MHW scenario, with and without prior moderate thermal stress, clearly showed that (1) a temperature increase consistent with naturally occurring rises in temperature boosted the rhodolith’s net primary production, and (2) this prior exposure mitigated MHW impacts on rhodolith calcification, DNP and DNC, and allowed for a quicker recovery of calcification and derived DNC, indicating the species’ capacity to acquire thermal stress memory that lasted for at least 1 week.
Regarding the first point, our results were mostly consistent with P. lusitanicum’s physiological responses to temperature levels within the species’ natural range (16–22°C) after a month-long acclimation, which showed that similarly to our study gross photosynthesis and light calcification were significantly increased at 22°C (Sordo et al., 2019). These findings are also supported by evidence from previous field and experimental studies, showing that the response of coralline algae to rising temperature is dependent on the magnitude of increase. A temperature rise within the range experienced in natural habitats, can increase growth, photosynthesis and calcification in both temperate and tropical species (Adey, 1970; Adey and McKibbin, 1970; Martin et al., 2006; Steller et al., 2007; Martin and Gattuso, 2009), while further increasing temperature results in a decline (Adey, 1970; Adey and McKibbin, 1970) and can have severe detrimental effects (Martin and Hall-Spencer, 2017).
The lower MHW impact on rhodolith physiology due to prior exposure to moderate temperature increase was consistent with the well-studied concept in terrestrial plants that appropriate responses to recurrent environmental fluctuations rely on the ability of an organism to keep “a memory” of prior exposure to certain conditions for a certain length of time (Bruce et al., 2007; Trewavas, 2009). Prior exposure to moderate heat stress led to acquired thermotolerance (or thermal priming) that enhanced the tolerance of P. lusitanicum to the subsequent challenge of a more severe heat stress event. The study of this phenomenon in marine science is a very recently emerging field, though ample ecological evidence exists from field observations that suggest weaker negative heat stress impacts on marine organisms exposed to recurrent thermal stress events (Oliver and Palumbi, 2011; Hughes et al., 2019) or high-frequency temperature variability, such as daily temperature range (Safaie et al., 2018). So far only a few studies on benthic primary producers have experimentally assessed the effect of thermal priming on acute (or MHW) stress responses (Kishimoto et al., 2019; Nguyen et al., 2020; Yu et al., 2020). The red alga Bangia fuscopurpurea acquired short-term thermal stress memory, which enhanced the species’ thermotolerance against subsequent lethal high-temperature stress (Kishimoto et al., 2019). In two seagrass species, Posidonia australis and Zostera muelleri, a similar experimental design as used in our study showed that non-preheated plants suffered a significant reduction in photosynthetic capacity, leaf growth and chlorophyll a content, while plants exposed to a prior mild thermal stress were able to cope better with the recurrent stressful event (Nguyen et al., 2020). Similar findings of increased thermal tolerance upon pre-exposure to moderate stress were also reported for the coral Acropora pruinosa (Yu et al., 2020). This increased thermotolerance through thermal stress memory has been associated to molecular modifications via activating, enhancing or speeding up responses to coping with environmental stressors (Crisp et al., 2016), e.g., higher expression of heat stress transcription factors regulating the expression of heat-shock proteins and antioxidant genes (Kotak et al., 2007; Song et al., 2012; Wang et al., 2014). Indeed, in the aforementioned studies regarding thermal priming in marine organisms, Nguyen et al. (2020) demonstrated that methylation-related genes were significantly regulated in seagrasses in response to thermal stress, while in B. fuscopurpurea modification of membrane fluidity via changes in membrane fatty acid composition was seemingly involved in the establishment and maintenance of heat-stress memory (Kishimoto et al., 2019).
Ecological Implications – Benefits of Living in a Naturally Fluctuating Thermal Regime
Phymatolithon lusitanicum exhibits a wide latitudinal distribution, spanning from Scotland and Ireland to the Iberian Peninsula and the Western Mediterranean Sea, but also a large vertical distribution from the intertidal zone down to 64 m (Peña et al., 2015; Bunker et al., 2018). This suggests that the species has a wide environmental tolerance, which is supported by our study that shows that it is well adapted to the highly fluctuating environment at the southern coast of Portugal. In fact, it seemingly benefits from the often strong temperature increases, due to inversions from upwelling to downwelling conditions, as these conditions not only boost the species productivity, but also allow it to cope better with more intense temperature rises, such as MHW events, ensuring lower impacts on net primary production and calcification, as well as a quicker recovery. The capacity of P. lusitanicum to remember past thermal incidents and store this knowledge to adapt to new challenges represents a great advantage for this species in its natural habitat, where environmental conditions, i.e., temperature and light availability, fluctuate fast and repeatedly. Thus, the P. lusitanicum population in the Algarve can be considered as resilient (sensu Hodgson et al., 2015), combining both, resistance to immediate physiological impacts and recovery after the stress exposure. The species’ resilience is also evidenced by the maintenance of relatively constant seasonal growth rates, despite the large environmental fluctuations and MHWs (Sordo et al., 2020). However, future research is needed to assess if other populations of P. lusitanicum along the species’ latitudinal distribution show a similar thermotolerance and resilience or if the naturally variable environment of this specific population is the major driver for its resistance to thermal stress.
Conclusion
Temperature can exert strong effects on coralline algal physiology, as evidenced by the present and previous studies (Martin and Hall-Spencer, 2017; Cornwall et al., 2019), though the direction and magnitude of these effects is strongly determined by the thermal history of the species, as temperature increases within the range experienced in their natural habitat can increase species’ physiological and growth performance, while increases above the local thermal maximum can cause adverse effects (Ichiki et al., 2001; Blake and Maggs, 2003; Steller et al., 2007; Vásquez-Elizondo and Enríquez, 2016; Tanaka et al., 2017; Graba-Landry et al., 2018).
As shown here and in other recent studies, MHW impacts in environments where thermal stress is recurrent are attenuated through the induction of thermal stress memory. Our findings highlight (1) the importance of considering local environmental conditions for designing realistic experimental MHW simulations and for the interpretation of the organisms’ responses to temperature increase, (2) the need for more detailed studies to test for the duration of the stress memory, which in P. lusitanicum lasts at least 1 week, but has been reported to last from several days to months in terrestrial plants (Iqbal and Ashraf, 2007; Rendina González et al., 2018), and (3) the usefulness of increased time-resolution for physiological determinations during experimental thermal stress induction and release for studying associated up- and/or down-regulation of physiological mechanisms.
Data Availability Statement
The original contributions presented in the study are included in the article/Supplementary Material, further inquiries can be directed to the corresponding author.
Author Contributions
NS and JS developed the concept and including the method and approach to be used and conducted the field work. NS conducted the experiment and the subsequent sample and data analysis. NS wrote the original draft of the article, supported by RS and JS who contributed to the writing, reviewing, and editing of the manuscript. All authors have read and agreed to the published version of the manuscript.
Funding
This research was funded by the European Union’s Horizon 2020 Research and Innovation Programme under the Marie Skłodowska-Curie (Grant agreement No. 844703), Portuguese national funds from FCT – Fundação para a Ciência e a Tecnologia through project (UIDB/04326/2020), and from the operational programmes CRESC Algarve 2020 and COMPETE 2020 through project EMBRC.PT ALG-01-0145-FEDER-022121.
Conflict of Interest
The authors declare that the research was conducted in the absence of any commercial or financial relationships that could be construed as a potential conflict of interest.
Publisher’s Note
All claims expressed in this article are solely those of the authors and do not necessarily represent those of their affiliated organizations, or those of the publisher, the editors and the reviewers. Any product that may be evaluated in this article, or claim that may be made by its manufacturer, is not guaranteed or endorsed by the publisher.
Acknowledgments
The authors thank João Reis and Miguel Patusco from the CCMAR’s Experimental Marine Station Ramalhete for their technical support with the mesocosm system and Miguel Rodrigues and the DiveSpot team for their support with the fieldwork.
Supplementary Material
The Supplementary Material for this article can be found online at: https://www.frontiersin.org/articles/10.3389/fmars.2021.791422/full#supplementary-material
References
Adey, W. H. (1970). The effects of light and temperature on growth rates in boreal-subarctic crustose corallines. J. Phycol. 6, 269–276. doi: 10.1111/j.1529-8817.1970.tb02392.x
Adey, W. H. (1978). Algal ridges of the Caribbean sea and West Indies. Phycologia 17, 361–367. doi: 10.2216/i0031-8884-17-4-361.1
Adey, W. H., and McKibbin, D. L. (1970). Studies on the maerl species Phymatolithon calcareum (Pallas) nov. comb. and Lithothamnium coralloides Crouan in the Ria de Vigo. Bot. Mar. 13, 100–106. doi: 10.1515/botm.1970.13.2.100
Amado-Filho, G. M., Moura, R. L., Bastos, A. C., Salgado, L. T., Sumida, P. Y., Guth, A. Z., et al. (2012). Rhodolith beds are major CaCO3 bio-factories in the tropical South West Atlantic. PLoS One 7:e35171. doi: 10.1371/journal.pone.0035171
Bernhardt, J. R., O’Connor, M. I., Sunday, J. M., and Gonzalez, A. (2020). Life in fluctuating environments. Phil. Trans. Roy. Soc. B Biol. Sci. 375:20190454. doi: 10.1098/rstb.2019.0454
Blake, C., and Maggs, C. A. (2003). Comparative growth rates and internal banding periodicity of maerl species (Corallinales, Rhodophyta) from northern Europe. Phycologia 42, 606–612. doi: 10.2216/i0031-8884-42-6-606.1
Boyd, P. W., Cornwall, C. E., Davison, A., Doney, S. C., Fourquez, M., Hurd, C. L., et al. (2016). Biological responses to environmental heterogeneity under future ocean conditions. Global Change Biol. 22, 2633–2650. doi: 10.1111/gcb.13287
Bradshaw, A. L., Brewer, P. G., Sharer, D. K., and Williams, R. T. (1981). Measurements of total carbon dioxide and alkalinity by potentiometric titration in the GEOSECS program. Earth Planet. Sci. Lett. 55, 99–115. doi: 10.1016/0012-821X(81)90090-X
Britton, D., Cornwall, C. E., Revill, A. T., Hurd, C. L., and Johnson, C. R. (2016). Ocean acidification reverses the positive effects of seawater pH fluctuations on growth and photosynthesis of the habitat-forming kelp, Ecklonia radiata. Sci. Rep. 6:26036. doi: 10.1038/srep26036
Bruce, T. J., Matthes, M. C., Napier, J. A., and Pickett, J. A. (2007). Stressful “memories” of plants: evidence and possible mechanisms. Plant Sci. 173, 603–608. doi: 10.1016/j.plantsci.2007.09.002
Bunker, F.StP.D., Mercer, T. M., Howson, C. M., Moore, J. M., Diaz, P., Maggs, C. A., et al. (2018). Site Condition Monitoring of Maerl Beds and Seagrass Beds in the Sound of Barra SAC 2015 – Diving Survey. Scottish Natural Heritage Research Report No. 924. Available online at: https://www.nature.scot/sites/default/files/2018-12/Publication%202018%20-%20SNH%20Research%20Report%20924%20-%20Site%20Condition%20monitoring%20of%20maerl%20beds%20and%20seagrass%20beds%20in%20the%20Sound%20of%20Barra%20SAC%202015%20-%20diving%20survey.pdf
Carro, B., Lopez, L., Peña, V., Bárbara, I., and Barreiro, R. (2014). DNA barcoding allows the accurate assessment of European maerl diversity: a Proof-of-Concept study. Phytotaxa 190, 176–189. doi: 10.11646/phytotaxa.190.1.12
Cornwall, C. E., Comeau, S., DeCarlo, T. M., Moore, B., D’Alexis, Q., and McCulloch, M. T. (2018). Resistance of corals and coralline algae to ocean acidification: physiological control of calcification under natural pH variability. Proc. Roy. Soc. B Biol. Sci. 285:20181168. doi: 10.1098/rspb.2018.1168
Cornwall, C. E., Diaz-Pulido, G., and Comeau, S. (2019). Impacts of ocean warming on coralline algal calcification: meta-analysis, knowledge gaps, and key recommendations for future research. Front. Mar. Sci. 6:186. doi: 10.3389/fmars.2019.00186
Cornwall, C. E., Hepburn, C. D., McGraw, C. M., Currie, K. I., Pilditch, C. A., Hunter, K. A., et al. (2013). Diurnal fluctuations in seawater pH influence the response of a calcifying macroalga to ocean acidification. Proc. Roy. Soc. B Biol. Sci. 280:20132201. doi: 10.1098/rspb.2013.2201
Crisp, P. A., Ganguly, D., Eichten, S. R., Borevitz, J. O., and Pogson, B. J. (2016). Reconsidering plant memory: intersections between stress recovery, RNA turnover, and epigenetics. Sci. Adv. 2:e1501340. doi: 10.1126/sciadv.1501340
Foster, M. S. (2001). Rhodoliths: between rocks and soft places. J. Phycol. 37, 659–667. doi: 10.1046/j.1529-8817.2001.00195.x
Frölicher, T. L., and Laufkötter, C. (2018). Emerging risks from marine heat waves. Nat. Commun. 9:650. doi: 10.1038/s41467-018-03163-6
Garel, E., Laiz, I., Drago, T., and Relvas, P. (2016). Characterisation of coastal counter-currents on the inner shelf of the Gulf of Cadiz. J. Mar. Syst. 155, 19–34. doi: 10.1016/j.jmarsys.2015.11.001
Graba-Landry, A., Hoey, A. S., Matley, J. K., Sheppard Brennand, H., Poore, A. G. B., Byrne, M., et al. (2018). Ocean warming has greater and more consistent negative effects than ocean acidification on the growth and health of subtropical macroalgae. Mar. Ecol. Prog. Ser. 595, 55–69. doi: 10.3354/meps12552
Hansson, I., and Jagner, D. (1973). Evaluation of the accuracy of Gran plots by means of computer calculations: application to the potentiometric titration of the total alkalinity and carbonate content in sea water. Anal. Chim. Acta 75, 363–373. doi: 10.1016/S0003-2670(01)82503-4
Helmuth, B., Mieszkowska, N., Moore, P., and Hawkins, S. J. (2006). Living on the edge of two changing worlds: forecasting the responses of rocky intertidal ecosystems to climate change. Annu. Rev. Ecol. Evol. Syst. 37, 373–404. doi: 10.1146/annurev.ecolsys.37.091305.110149
Hilker, M., Schwachtje, J., Baier, M., Balazadeh, S., Bäurle, I., Geiselhardt, S., et al. (2016). Priming and memory of stress responses in organisms lacking a nervous system. Biol. Rev. 91, 1118–1133. doi: 10.1111/brv.12215
Hobday, A. J., Alexander, L. V., Perkins, S. E., Smale, D. A., Straub, S. C., Oliver, E. C., et al. (2016). A hierarchical approach to defining marine heatwaves. Progr. Oceanogr. 141, 227–238. doi: 10.1016/j.pocean.2015.12.014
Hodgson, D., McDonald, J. L., and Hosken, D. J. (2015). What do you mean,‘resilient’? Trends Ecol. Evol. 30, 503–506. doi: 10.1016/j.tree.2015.06.010
Hughes, T. P., Kerry, J. T., Connolly, S. R., Baird, A. H., Eakin, C. M., Heron, S. F., et al. (2019). Ecological memory modifies the cumulative impact of recurrent climate extremes. Nat. Clim. Change 9, 40–43. doi: 10.1038/s41558-018-0351-2
Ichiki, S., Izuta, H., Yasui, H., and Yamamoto, H. (2001). Effects of irradiance and water temperature on the photosynthesis and growth of the crustose coralline alga Lithophyllum yessoense Foslie (Corallinales, Rhodophyceae). Bull. Fish. Sci. Hokkaido Univ. 52, 103–109.
Iqbal, M., and Ashraf, M. (2007). Seed preconditioning modulates growth, ionic relations, and photosynthetic capacity in adult plants of hexaploid wheat under salt stress. J. Plant Nutr. 30, 381–396. doi: 10.1080/01904160601171330
Johnson, M. D., Moriarty, V. W., and Carpenter, R. C. (2014). Acclimatization of the crustose coralline alga Porolithon onkodes to variable pCO2. PLoS One 9:e87678. doi: 10.1371/journal.pone.0087678
Johnson, M. D., Rodriguez Bravo, L. M., Lucey, N., and Altieri, A. H. (2021). Environmental legacy effects and acclimatization of a crustose coralline alga to ocean acidification. Clim. Change Ecol. 2:100016. doi: 10.1016/j.ecochg.2021.100016
Jueterbock, A., Minne, A. J., Cock, J. M., Coleman, M. A., Wernberg, T., Scheschonk, L., et al. (2021). Priming of marine macrophytes for enhanced restoration success and food security in future oceans. Front. Mar. Sci. 8:658485. doi: 10.3389/fmars.2021.658485
Kishimoto, I., Ariga, I., Itabashi, Y., and Mikami, K. (2019). Heat-stress memory is responsible for acquired thermotolerance in Bangia fuscopurpurea. J. Phycol. 55, 971–975. doi: 10.1111/jpy.12895
Kotak, S., Larkindale, J., Lee, U., von Koskull-Döring, P., Vierling, E., and Scharf, K. D. (2007). Complexity of the heat stress response in plants. Curr. Opin. Plant Biol. 10, 310–316. doi: 10.1016/j.pbi.2007.04.011
Kroeker, K. J., Bell, J. D., Donham, E. M., Hoshijima, U., Lummis, S., Toy, J. A., et al. (2019). Ecological change in dynamic environments: accounting for temporal environmental variability in studies of ocean change biology. Glob. Change Biol. 26, 54–67. doi: 10.1111/gcb.14868
Littler, M. M., and Littler, D. S. (2013). “The nature of crustose coralline algae and their interactions on reefs,” in Research and Discoveries: The Revolution of Science through Scuba; Smithsonian Contributions to the Marine Sciences eds M. A. Lang, R. L. Marinelli, S. J. Roberts, and P. R. Taylor (Washington, DC: Smithsonian Institution Scholarly Press) 199–212.
López-Pérez, A., Guendulain-García, S., Granja-Fernández, R., Hernández-Urraca, V., Galván-Rowland, L., Zepeta-Vilchis, R., et al. (2016). Reef community changes associated with the 2009–2010 El Niño in the Southern Mexican Pacific. Pac. Sci. 70, 175–190. doi: 10.2984/70.2.4
Martin, S., Castets, M. D., and Clavier, J. (2006). Primary production, respiration and calcification of the temperate free-living coralline alga Lithothamnion corallioides. Aquat. Bot. 85, 121–128. doi: 10.1016/j.aquabot.2006.02.005
Martin, S., and Gattuso, J. P. (2009). Response of Mediterranean coralline algae to ocean acidification and elevated temperature. Global Change Biol. 15, 2089–2100. doi: 10.1111/j.1365-2486.2009.01874.x
Martin, S., and Hall-Spencer, J. M. (2017). “Effects of ocean warming and acidification on rhodolith/maërl beds,” in Rhodolith/maërl Beds: A Global Perspective, eds R. Riosmena-Rodríguez, W. Nelson, and J. Aguirre (Berlin: Springer), 55–85.
McCoy, S. J., and Widdicombe, S. (2019). Thermal plasticity is independent of environmental history in an intertidal seaweed. Ecol. Evol. 9, 13402–13412. doi: 10.1002/ece3.5796
Meehl, G. A., and Tebaldi, C. (2004). More intense, more frequent, and longer lasting heat waves in the 21st century. Science 305, 994–997. doi: 10.1126/science.1098704
Miller, A. D., Coleman, M. A., Clark, J., Cook, R., Naga, Z., Doblin, M. A., et al. (2020). Local thermal adaptation and limited gene flow constrain future climate responses of a marine ecosystem engineer. Evol. Appl. 13, 918–934. doi: 10.1111/eva.12909
Murray, S. N., and Horn, M. H. (1989). Variations in standing stocks of central California macrophytes from a rocky intertidal habitat before and during the 1982-1983 El Nino. Mar. Ecol. Progr. Ser. 58, 113–122. doi: 10.3354/meps058113
Nelson, W. A. (2009). Calcified macroalgae–critical to coastal ecosystems and vulnerable to change: a review. Mar. Freshw. Res. 60, 787–801. doi: 10.1071/MF08335
Nguyen, H. M., Kim, M., Ralph, P. J., Marín-Guirao, L., Pernice, M., and Procaccini, G. (2020). Stress memory in seagrasses: first insight into the effects of thermal priming and the role of epigenetic modifications. Front. Plant Sci. 11:494. doi: 10.3389/fpls.2020.00494
Oliver, E. C., Donat, M. G., Burrows, M. T., Moore, P. J., Smale, D. A., Alexander, L. V., et al. (2018). Longer and more frequent marine heatwaves over the past century. Nat. Commun. 9:1324. doi: 10.1038/s41467-018-03732-9
Oliver, T. A., and Palumbi, S. R. (2011). Do fluctuating temperature environments elevate coral thermal tolerance? Coral Reefs 30, 429–440. doi: 10.1007/s00338-011-0721-y
Page, T. M., Bergstrom, E., and Diaz-Pulido, G. (2021). Acclimation history of elevated temperature reduces the tolerance of coralline algae to additional acute thermal stress. Front. Mar. Sci. 8:511. doi: 10.3389/fmars.2021.660196
Pardo, C., Lopez, L., Peña, V., Hernández-Kantún, J., Le Gall, L., Bárbara, I., et al. (2014). A multilocus species delimitation reveals a striking number of species of coralline algae forming maerl in the OSPAR maritime area. PLoS One 9:e104073. doi: 10.1371/journal.pone.0104073
Pearce, A. F., and Feng, M. (2013). The rise and fall of the “marine heat wave” off Western Australia during the summer of 2010/2011. J. Mar. Syst. 111, 139–156. doi: 10.1016/j.jmarsys.2012.10.009
Peña, V., Pardo, C., López, L., Carro, B., Hernandez-Kantun, J., Adey, W. H., et al. (2015). Phymatolithon lusitanicum sp. nov. (Hapalidiales, Rhodophyta): the third most abundant maerl-forming species in the Atlantic Iberian Peninsula. Cryptogam. Algol. 36, 429–459. doi: 10.7872/crya/v36.iss4.2015.429
Putnam, H. M., Edmunds, P. J., and Fan, T. Y. (2010). Effect of a fluctuating thermal regime on adult and larval reef corals. Invertebr. Biol. 129, 199–209. doi: 10.1111/j.1744-7410.2010.00199.x
Qui-Minet, Z. N., Davoult, D., Grall, J., Delaunay, C., Six, C., Cariou, T., et al. (2021). Physiology of maerl algae: comparison of inter- and intraspecies variations. J. Phycol. 57, 831–848. doi: 10.1111/jpy.13119
Ragazzola, F., Marchini, A., Adani, M., Bordone, A., Castelli, A., Cerrati, G., et al. (2021). An intertidal life: combined effects of acidification and winter heatwaves on a coralline alga (Ellisolandia elongata) and its associated invertebrate community. Mar. Environ. Res. 169:105342. doi: 10.1016/j.marenvres.2021.105342
Rendina, F., Bouchet, P. J., Appolloni, L., Russo, G. F., Sandulli, R., Kolzenburg, R., et al. (2019). Physiological response of the coralline alga Corallina officinalis L. to both predicted long-term increases in temperature and short-term heatwave events. Mar. Environ. Res. 150:104764. doi: 10.1016/j.marenvres.2019.104764
Rendina González, A. P., Preite, V., Verhoeven, K. J. F., and Latzel, V. (2018). Transgenerational effects and epigenetic memory in the clonal plant Trifolium repens. Front. Plant Sci. 9:1677. doi: 10.3389/fpls.2018.01677
Riosmena-Rodríguez, R., Nelson, W., and Aguirre, J. (2017). Rhodolith/Maërl Beds: A Global Perspective. Cham: Springer International Publishing.
Rivest, E. B., Comeau, S., and Cornwall, C. E. (2017). The role of natural variability in shaping the response of coral reef organisms to climate change. Curr. Clim. Change Rep. 3, 271–281. doi: 10.1007/s40641-017-0082-x
Roleda, M. Y., Cornwall, C. E., Feng, Y., McGraw, C. M., Smith, A. M., and Hurd, C. L. (2015). Effect of ocean acidification and pH fluctuations on the growth and development of coralline algal recruits, and an associated benthic algal assemblage. PLoS One 10:e0140394. doi: 10.1371/journal.pone.0140394
Safaie, A., Silbiger, N. J., McClanahan, T. R., Pawlak, G., Barshis, D. J., Hench, J. L., et al. (2018). High frequency temperature variability reduces the risk of coral bleaching. Nat. Commun. 9:1671. doi: 10.1038/s41467-018-04074-2
Sánchez, R. F., Mason, E., Relvas, P., Da Silva, A. J., and Peliz, A. (2006). On the inner-shelf circulation in the northern Gulf of Cádiz, southern Portuguese shelf. Deep Sea Res. Part II Top. Stud. Oceanogr. 53, 1198–1218. doi: 10.1016/j.dsr2.2006.04.002
Schlegel, R. W. (2018). Marine Heatwave Tracker: The App to See When and Where Marine Heatwaves are Happening Around the World. Available online at: http://www.marineheatwaves.org/tracker (accessed June 15, 2021).
Schoepf, V., Stat, M., Falter, J. L., and McCulloch, M. T. (2015). Limits to the thermal tolerance of corals adapted to a highly fluctuating, naturally extreme temperature environment. Sci. Rep. 5:17639. doi: 10.1038/srep17639
Schubert, N., Salazar, V. W., Rich, W. A., Vivanco Bercovich, M., Almeida Saá, A. C., Fadigas, S. D., et al. (2019). Rhodolith primary and carbonate production in a changing ocean: the interplay of warming and nutrients. Sci. Total Environ. 676, 455–468. doi: 10.1016/j.scitotenv.2019.04.280
Short, J., Foster, T., Falter, J., Kendrick, G. A., and McCulloch, M. T. (2015). Crustose coralline algal growth, calcification and mortality following a marine heatwave in Western Australia. Cont. Shelf Res. 106, 38–44. doi: 10.1016/j.csr.2015.07.003
Smith, S. V., and Kinsey, D. W. (1978). “Calcification and organic carbon metabolism as indicated by carbon dioxide,” in Coral Reefs: Research Methods, Monographs on Oceanographic Methodology, Vol. 5, eds D. R. Stoddart and R. E. Johannes (Paris: UNESCO), 469–484.
Smith, S. V., and Mackenzie, F. T. (2016). The role of CaCO3 reactions in the contemporary oceanic CO2 cycle. Aquat. Geochem. 22, 153–175. doi: 10.1007/s10498-015-9282-y
Song, L., Jiang, Y., Zhao, H., and Hou, M. (2012). Acquired thermotolerance in plants. Plant Cell Tissue Organ Cult. 111, 265–276. doi: 10.1007/s11240-012-0198-6
Sordo, L., Santos, R., Barrote, I., Freitas, C., and Silva, J. (2020). Seasonal photosynthesis, respiration and calcification of a temperate maërl bed in Southern Portugal. Front. Mar. Sci. 7:136. doi: 10.3389/fmars.2020.00136
Sordo, L., Santos, R., Barrote, I., and Silva, J. (2019). Temperature amplifies the effect of high CO2 on the photosynthesis, respiration, and calcification of the coralline algae Phymatolithon lusitanicum. Ecol. Evol. 9, 11000–11009. doi: 10.1002/ece3.5560
Steller, D. L., Hernández-Ayón, J. M., Riosmena-Rodríguez, R., and Cabello-Pasini, A. (2007). Effect of temperature on photosynthesis, growth and calcification rates of the free-living coralline alga Lithophyllum margaritae. Cienc. Mar. 33, 441–456. doi: 10.7773/cm.v33i4.1255
Steneck, R. S. (1986). The ecology of coralline algal crusts: convergent patterns and adaptive strategies. Ann. Rev. Ecol. Syst. 17, 273–303. doi: 10.1146/annurev.es.17.110186.001421
Tanaka, Y., Suzuki, A., and Sakai, K. (2017). Effects of elevated seawater temperature and phosphate enrichment on the crustose coralline alga Porolithon onkodes (Rhodophyta). Phycol. Res. 65, 51–57. doi: 10.1111/pre.12152
Trewavas, A. (2009). What is plant behaviour? Plant Cell Environ. 32, 606–616. doi: 10.1111/j.1365-3040.2009.01929.x
van der Heijden, L. H., and Kamenos, N. A. (2015). Reviews and syntheses: calculating the global contribution of coralline algae to total carbon burial. Biogeosciences 12, 6429–6441. doi: 10.5194/bg-12-6429-2015
Vargas, C. A., Lagos, N. A., Lardies, M. A., Duarte, C., Manríquez, P. H., Aguilera, V. M., et al. (2017). Species-specific responses to ocean acidification should account for local adaptation and adaptive plasticity. Nat. Ecol. Evol. 1:0084. doi: 10.1038/s41559-017-0084
Vásquez-Elizondo, R. M., and Enríquez, S. (2016). Coralline algal physiology is more adversely affected by elevated temperature than reduced pH. Sci. Rep. 6:19030. doi: 10.1038/srep19030
Wang, X., Cai, J., Liu, F., Dai, T., Cao, W., Wollenweber, B., et al. (2014). Multiple heat priming enhances thermo-tolerance to a later high temperature stress via improving subcellular antioxidant activities in wheat seedlings. Plant Physiol. Biochem. 74, 185–192. doi: 10.1016/j.plaphy.2013.11.014
Wernberg, T., Smale, D. A., Tuya, F., Thomsen, M. S., Langlois, T. J., De Bettignies, T., et al. (2013). An extreme climatic event alters marine ecosystem structure in a global biodiversity hotspot. Nat. Clim. Change 3, 78–82. doi: 10.1038/nclimate1627
Yu, X., Yu, K., Huang, W., Liang, J., Qin, Z., Chen, B., et al. (2020). Thermal acclimation increases heat tolerance of the scleractinian coral Acropora pruinosa. Sci. Total Environ. 733:139319. doi: 10.1016/j.scitotenv.2020.139319
Keywords: calcification, coralline algae, environmental variability, heatwave, net productivity, thermal stress memory
Citation: Schubert N, Santos R and Silva J (2021) Living in a Fluctuating Environment Increases Tolerance to Marine Heatwaves in the Free-Living Coralline Alga Phymatolithon lusitanicum. Front. Mar. Sci. 8:791422. doi: 10.3389/fmars.2021.791422
Received: 08 October 2021; Accepted: 10 December 2021;
Published: 23 December 2021.
Edited by:
Anne Chenuil, Centre National de la Recherche Scientifique (CNRS), FranceReviewed by:
Emily Rivest, William & Mary’s Virginia Institute of Marine Science, College of William & Mary, United StatesAurélien De Jode, University of Gothenburg, Sweden
Copyright © 2021 Schubert, Santos and Silva. This is an open-access article distributed under the terms of the Creative Commons Attribution License (CC BY). The use, distribution or reproduction in other forums is permitted, provided the original author(s) and the copyright owner(s) are credited and that the original publication in this journal is cited, in accordance with accepted academic practice. No use, distribution or reproduction is permitted which does not comply with these terms.
*Correspondence: Nadine Schubert, bmFkaW5lX3NjaHViZXJ0QGhvdG1haWwuY29t