- 1Duke University Marine Lab, Beaufort, NC, United States
- 2Department of Biology, Hopkins Marine Station, Stanford University, Pacific Grove, CA, United States
- 3Institute of Marine Sciences, Department of Marine Sciences, University of North Carolina at Chapel Hill, Morehead City, NC, United States
- 4Department of Environmental Engineering Sciences, University of Florida, Gainesville, FL, United States
- 5Department of Biology, Coastal Carolina University, Conway, SC, United States
- 6Independent Researcher, Madison, CT, United States
In coastal wetlands and tropical reefs, snails can regulate foundation species by feeding on marsh grasses and hard corals. In many cases, their impacts are amplified because they facilitate microbial infection in grazer-induced wounds. Whether snails commonly graze live plants and facilitate microbial growth on plants in tropical seagrass systems is less explored. On a Belizean Caye, we examined patterns in snail-generated grazer scars on the abundant turtlegrass (Thalassia testudinum). Our initial survey showed the occurrence of snail-induced scarring on live turtlegrass blades was common, with 57% of live leaves scarred. Feeding trials demonstrated that two of five common snails (Tegula fasciata–smooth tegula and Smaragdia viridis–emerald nerite) grazed unepiphytized turtlegrass blades and that smooth tegula abundance had a positive relationship with scarring intensity. Subsequent surveys at three Caribbean sites (separated by >150 km) also showed a high occurrence of snail-induced scars on turtlegrass blades. Finally, simulated herbivory experiments and field observations of a turtlegrass bed in Florida, United States suggests that herbivore damage could facilitate fungal growth in live seagrass tissue through mechanical opening of tissue. Combined, these findings reveal that snail grazing on live turtlegrass blades in the Caribbean can be common. Based on these results, we hypothesize that small grazers could be exerting top-down control over turtlegrass growth directly via grazing and/or indirectly by facilitating microbial infection in live seagrass tissue. Further studies are needed to determine the generality and relative importance of direct and indirect effects of gastropod grazing on turtlegrass health.
Introduction
In terrestrial systems, grazers can have large and diverse effects on plant communities. Studies across multiple habitats have demonstrated grazers’ ability to influence primary production (Vickery, 1972; Hik and Jefferies, 1990; Bruno et al., 2008; He and Silliman, 2016), leaf abscission rates (Mingo and Oesterheld, 2009), nutrient content in leaves (Zieman et al., 1984), flowering rates (Maschinski and Whitham, 1989; Brys et al., 2011), colonial propagation (Maschinski and Whitham, 1989), as well as plant diversity and succession (Huntly, 1991; Olff and Ritchie, 1998). Mechanisms of control can be direct, through the consumption of plant tissue, and/or through a myriad of indirect pathways. A powerful indirect pathway of grazer control on plant growth is facilitation of microbial infection in live plant tissues via grazer wounding (Taylor and Bardner, 1968; Smith and Odum, 1981; Turner, 1989; Furbish and Albano, 1994; Silliman and Newell, 2003; Daleo et al., 2009). For example, plant grazers, such as insects, can carry and facilitate invasion by microbial pathogens in agricultural crops, trees, and other plants (Linit, 1988; Pimentel, 1991; Eigenbrode et al., 2018). This grazer-pathogen-plant interaction often leads to suppressed plant growth and can make plants more susceptible to environmental stressors such as drought (Silliman, 2005; He et al., 2013).
In marine systems, grazers can similarly control many aspects of plant community structure and ecosystem function. Marine grazers have been shown to alter plant growth, reproduction, diversity, and plant-regulated nutrient cycling across virtually every marine ecosystem (Poore et al., 2012). Many of these studies recognize that grazer control of plant growth can occur through direct consumption of live plant tissue. For example, parrot fish consume macroalgae thus reducing competition between plants and algae (Valentine and Duffy, 2006; Mumby, 2009), crabs consume mangrove propagules leading to forest zonation patterns (Smith, 1987; Sousa and Mitchell, 1999), and snails and chitons rasp intertidal algae (Hawkins and Hartnoll, 1983). Additionally, in seagrass systems, it has been shown that urchins grazing seagrasses can lead to local extinction of seagrass beds (Camp et al., 1973; Valentine and Heck, 1991; Heck and Valentine, 1995), and dugongs and sea turtles grazing seagrasses can reduce seagrass shoot density and above and belowground biomass (Heinsohn and Birch, 1972; Ogden et al., 1983; Lanyon et al., 1989; Heck and Valentine, 2006; Larkum et al., 2006). Furthermore, other studies have shown that grazers can also control plant growth via indirect interactions, such as mesograzer facilitation of seagrass growth by ingesting epiphytic algae that would otherwise smother seagrasses (Orth et al., 1984).
However, only a few studies in marine systems have shown that grazer facilitation of disease in plants is an important mechanism of top-down control. In one example, research in salt marshes has shown that grazer facilitation of microbial infection in live plant tissue has negative consequences for plants (Silliman and Newell, 2003). Specifically, in southeastern U.S. salt marshes, the marsh periwinkle (Littoraria irrorata) has been shown to facilitate fungal infection in live smooth cordgrass (Spartina alterniflora). A series of experiments revealed L. irrorata, previously thought to be a detritivore specialist, grazes live S. alterniflora and generates open wounds that leaves the plant susceptible to fungal infection (Silliman and Zieman, 2001; Silliman and Newell, 2003). L. irrorata returns to grazed plants to consume fungi growing in wounds, exhibiting low-level fungal farming behavior. The result of this grazer-fungus interaction is decreased S. alterniflora growth and, at high snail densities, plant death. Fungal removal studies have demonstrated that microbial infection, not the direct consumption of live plant tissue, is the primary mechanism by which snails control plant growth (Silliman and Newell, 2003). This top-down fungal mechanism also occurs in Argentinian marshes, but with crabs as the primary facilitator (Daleo et al., 2009). Given these findings, and the abundance of grazers in marine systems, it is possible grazer control through microbial infection in marine plants is more common than currently thought.
In seagrass systems, small invertebrate grazers (e.g., amphipods, isopods, and gastropods, collectively called mesograzers) that eat plants and algae are of widespread importance (Brearley and Walker, 1995; Nakaoka et al., 2002; Fredriksen et al., 2004; Valentine and Duffy, 2006; Lewis and Boyer, 2014). However, most research testing for their top-down effects has focused on those grazer species that consume epiphytes growing on seagrass, rather than those that consume live seagrass directly. These studies have shown that epiphyte grazers often improve seagrass productivity by controlling the growth of competitively dominant algae, especially in eutrophic environments (Heck et al., 2000; Hughes et al., 2004; Reynolds et al., 2014; Campbell et al., 2018). In general, research on direct grazing (i.e., consumption of live plant tissue) of seagrass has typically highlighted the ability of vertebrates, such as fish, birds, sea turtles, and dugongs, to exert top-down control over seagrass (Valentine and Duffy, 2006; Kollars et al., 2017). Additionally, while there has been extensive research on certain invertebrate grazers, notably sea urchins, less work has explored the influence of small invertebrate grazers, such as snails, that feed directly on live seagrass and their cascading impacts on seagrass (Valentine and Heck, 1991; Alcoverro and Mariani, 2004; Eklöf et al., 2008). For example, a microcosm study found that snail grazing on live seagrass significantly reduced foliar biomass and chlorophyll (Zimmerman et al., 2001; Fredriksen et al., 2004; Holzer et al., 2011; Fong et al., 2018), while another study found that isopods could strongly suppress seagrass growth in a mesocosm (Nienhuis and Groenendijk, 1986; Duffy et al., 2001). In both cases, these grazers consumed live seagrass by making vertical scars, similar to those made by L. irrorata in marshes (B.R. Silliman personal observations and Silliman and Newell, 2003). This work, combined with observed scars on turtlegrass (Thalassia testudinum) in the Caribbean (B.R. Silliman personal observation) suggests that direct grazing of live seagrass by mesoinvertebrates could be common but understudied in tropical seagrasses. Furthermore, few studies have examined if this grazing by invertebrates can facilitate fungal growth on seagrasses, as has been shown in other coastal plant systems (Silliman and Zieman, 2001; Silliman and Newell, 2003).
Given the importance of seagrass as a foundation species in the Caribbean and evidence that invertebrate grazers can have cryptic but strong top-down control in other marine systems, we investigated if commonly abundant snails graze live turtlegrass (Thalassia testudinum) at multiple, dispersed Caribbean sites and if so, whether resulting scars promote fungal infection in live seagrass blades. We conducted field surveys to identify and quantify common snails in Belize’s seagrass beds and tested which of these snails would graze live seagrass. For those that did, we examined if their distribution in the field was positively correlated with abundance of grazing scars on live seagrass blades. To investigate the prevalence of snail grazing in other locations in the Caribbean, we conducted surveys in three other tropical seagrass beds (Florida, Bahamas, and USVI). Finally, we experimentally tested the effect scaring has on fungal presence on green seagrass blades.
Materials and Methods
Surveys (South Water Caye, Belize)
Initial work was conducted in South Water Caye, Belize. We surveyed turtlegrass (Thalassia testudinum) beds for grazing scars and snail density in January 2004. Seagrass beds were located approximately 20 m from shore at 1–2 m depth. Surveys were performed at two sites, approximately 200 m apart, one west and one south of the island. At each site, 15 random quadrats (25 × 25 cm) were surveyed during the day (12:00–16:00 h) and again at night (19:00–20:00 h). Within each quadrat, all aboveground seagrass biomass was collected using scissors. All snails on turtlegrass blades, the sediment surface, and within the top 2.5 cm of sediment in each quadrat were collected, enumerated, identified, and categorized by taxonomic group. In the lab, seagrass samples were sorted between live and dead plant material. Blades were considered alive if more than 90% of leaf tissue was green, and dead blades were discarded. Scar length (mm) and the total number of scars per turtlegrass blade was recorded. Leaf scars were only counted in our survey if they occurred on the green portion of the leaf to ensure they were not the product of detritivore activity. Snail grazer scars were highly consistent in morphology and characterized by longitudinal scrapes that did not completely penetrate the tissue. Individual scars were typically 2 mm wide and 1–30 cm in length (Figures 1A,B; Silliman et al., 2004). To compare relative densities of each snail species between the daytime and nighttime quadrat surveys, we ran a generalized linear model with a Poisson distribution. We then performed a post hoc test by computing the estimated marginal means and running pairwise comparisons of each group’s day and night densities using the “emmeans” package in R.
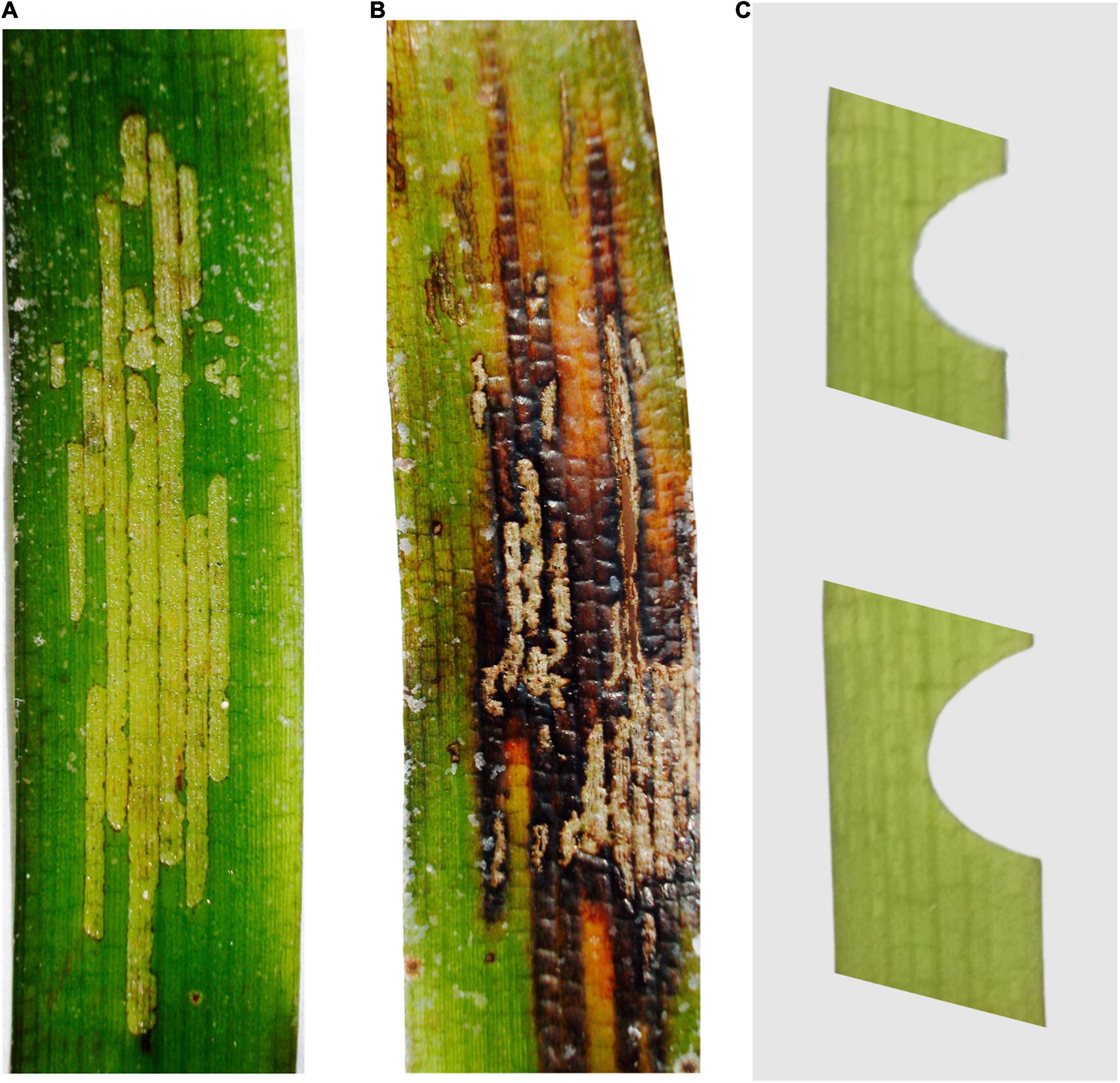
Figure 1. (A) Fresh grazing scars found on turtlegrass (Thalassia testudinum). (B) The onset of fungal infection as a result of grazer-induced scars on turtlegrass (Thalassia testudinum). (C) Turtlegrass blades after being wounded with a hole-punch for the simulated herbivory experiment. Images are courtesy of BS, Duke University.
Feeding Trials (South Water Caye, Belize)
To test if the five most common snails (Cerithium litteratum -stocky cerith, Tegula fasciata -smooth tegula, Smaragdia viridis -emerald nerite, Cerithium eburneum -ivory cerith, and Lithopoma phoebium -long-spined star snail) in South Water Caye, Belize (as determined by the field surveys) would consume live turtlegrass, we conducted mesocosm feeding trials. For feeding trials (n = 8 per snail species), three individuals of each snail species were starved for a 24-h period and placed in a container (20 cm high × 15 cm wide × 15 cm long) with mesh screening on five sides to eliminate snail escape and allow for continuous flow of sea water. Each container included two inches of sand substrate and freshly cut live turtlegrass blades that had no visible epiphytes on them (therefore likely a leaf that was 3–5 days old). Containers were placed in a shaded, well-flushed lagoon basin at 0.5 m depth. Snails were left inside these containers for 24 h, after which the number and length (mm) of scars on each turtlegrass blade were documented. The two species that grazed turtlegrass blades during feeding trials were emerald nerite and smooth tegula. Therefore, we performed one-way ANOVAs to compare the mean length and total number of scars, in order to investigate whether the scars were comparable between two species that differ in size and other natural history traits – e.g., the emerald nerite is much smaller than smooth tegula (Parr et al., 2014). Additional feeding trials were conducted to determine if tegula showed a preference for grazing non-epiphytized or epiphytized turtlegrass blades. The feeding trails consisted of placing one tegula snail in the mesocosm set-up previously described this time with two turtlegrass blades (one non-epiphytized and epiphytized) placed 1 cm apart to ensure the snail could detect both turtlegrass blades. The experiment was conducted over a 12-h period at night (19:00–7:00 h), with each feeding trial (n = 14) running until the first scar was made by tegula on one of the two turtlegrass blades. After the first scar occurred, the feeding trial ended and the turtlegrass blade (i.e., non-epiphytized or epiphytized) that was scarred was recorded.
Density-Mediated Effects (South Water Caye, Belize)
An additional field survey was conducted to assess if there was a relationship between snail density and scarring on green turtlegrass blades for smooth tegula and stocky cerith, the two most abundant snails. Emerald nerites were not included in these surveys as they were extremely rare during the daytime surveys (Figure 2). Although stocky ceriths did not scar green turtlegrass blades during the lab experiment, they were included in the field survey because they were found to be abundant during daytime surveys. Thus, we hypothesized there could be a density-dependent effect where stocky ceriths would only graze green turtlegrass blades under high densities, as has been seen in other marine snail-plant-interactions (Silliman and Zieman, 2001; Renzi and Silliman, 2020). Ten random plots (25 × 25 cm) were assessed for smooth tegula and stocky cerith densities. Additionally, within these plots, 10 random turtlegrass blades were selected and examined for wound occurrence as described above. We employed a non-parametric Spearman’s rank correlation test to evaluate whether there was a positive relationship between snail density and the number of grazing scars within each quadrat.
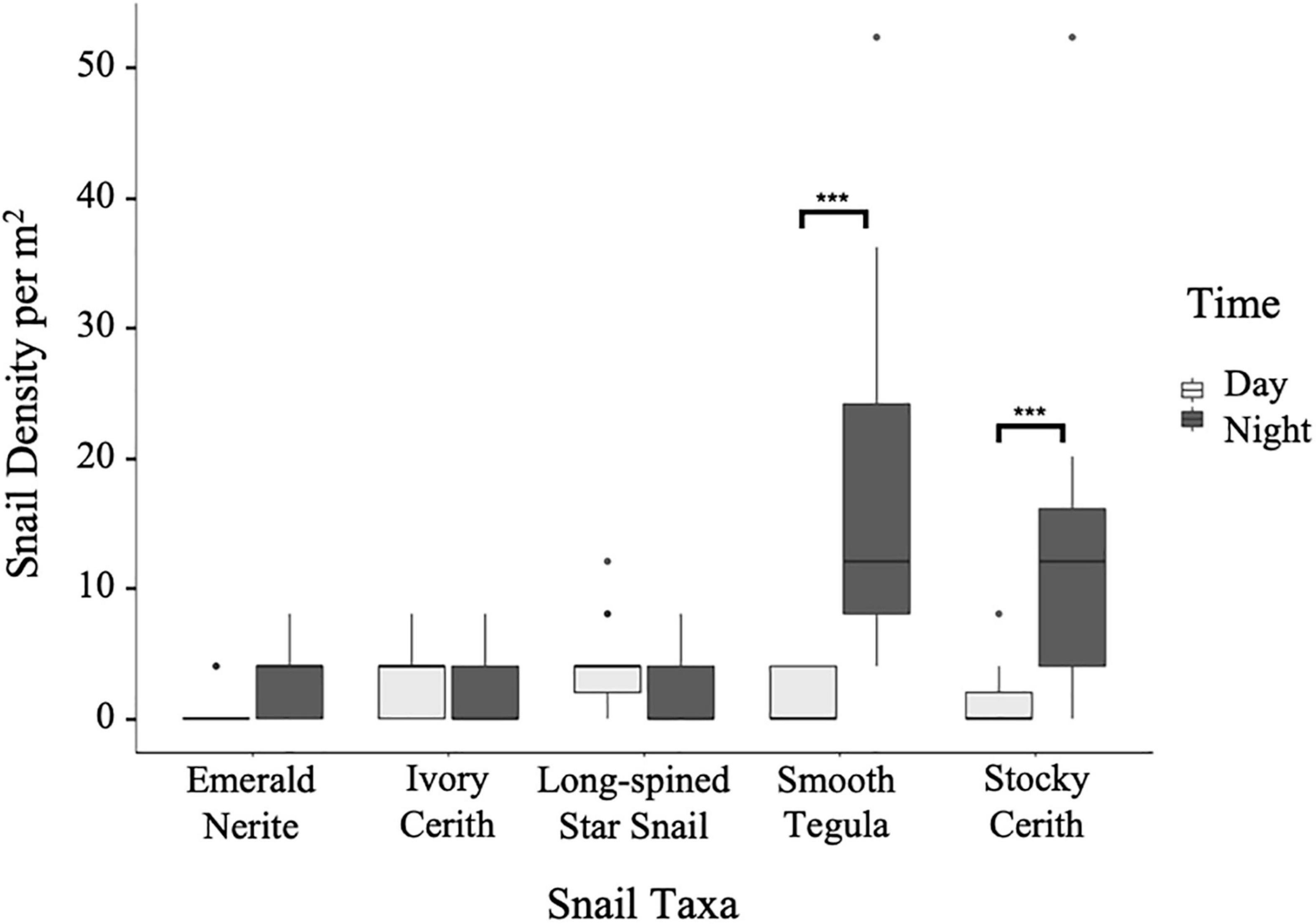
Figure 2. Comparison between day and night densities of five common Belize snails (Cerithium litteratum–stocky cerith, Tegula fasciata–smooth tegula, Smaragdia viridis–emerald nerite, Cerithium eburneum–ivory cerith, and Lithopoma phoebium–long-spined star snail). Three of the five common Belize snails (i.e., smooth tegula, stocky cerith, and emerald nerite) were found at higher densities during the night, while two (i.e., ivory cerith and long-spined star snail) were found in higher densities during the day. Significant p-values within each species comparing daytime and nighttime counts were calculated through a generalized linear model for Poisson and a post hoc estimated marginal means pairwise analysis ***p ≤ 0.0001.
Scarring Surveys (St. Croix, USVI, San Salvador, Bahamas, and Tampa Bay, Florida)
To test the generality of the relationship between grazer abundance and scar occurrence on live turtlegrass blades, follow-up surveys were conducted in seagrass meadows at 1–2 m depth on St. Croix, USVI (May 2004), San Salvador, Bahamas (May 2004), and Tampa Bay, Florida (May 2006). Scarring surveys consisted of ten random quadrats (25 × 25 cm), spaced at least three meters apart. From each plot, three random turtlegrass blades touching the east side of the quadrat were collected. All turtlegrass blades with parrot fish scarring were discarded. Parrot fish scarring was differentiated from invertebrate scarring by shape (half-moon cutouts vs. vertical scrapes, B.R. Silliman personal observation). The collected turtlegrass blades were examined and snail grazing scars were enumerated. We sought to test for commonness of grazing scars across variable locations. Therefore, we performed a one-way ANOVA to compare grazing wound occurrence between the different field survey sites (i.e., USVI, Bahamas and Florida), asking whether the average number of individual grazing scars per seagrass blade differed between locations.
Simulated Herbivory Experiments (Tampa Bay, Florida)
To explore the facilitation of fungal infection by herbivory-generated turtlegrass wounds, we used a hole punch to remove a semicircle portion from live turtlegrass blades in an experimental seagrass bed where surveys had been previously conducted (Figure 1C). Although this type of simulated damage does not replicate the type of scars snails generate, it did allow us to test whether simple mechanical damage of turtlegrass blades facilitates fungal infection in a standardized and repeatable way. We initially attempted to scrape the seagrass with razor blades (Silliman and Newell, 2003), which more similarly mimicked snail grazers, but this caused an unrealistic amount of damage to turtlegrass blades. Within the study meadow, 30 blades (3–4 cm length) with no epiphytes or scars were identified and wounded using the hole punch over a 2-day period. An additional 30 blades, located within 2 cm of each experimentally wounded blade, were designated as controls. The experiment ran for 2 weeks in June-July 2006. After 2-weeks, 16 of the 30 wounded turtlegrass and control blade pairs were found and collected. Blades were analyzed for fungal biomass using ergosterol-proxy techniques (Newell, 2002; Gulis and Bärlocher, 2017). We then performed a one-way ANOVA to compare fungal presence between wounded and non-wounded turtlegrass blades. For one randomly selected wounded turtlegrass blade, Lactophenol cotton blue (3 drops) was used to stain the fungal hyphae and examine whether hyphae had invaded live turtlegrass tissue near the hole punch wound. All statistical analyses were conducted using R (version: 4.0.5) and RStudio (Version: 1.4).
Results
Surveys (South Water Caye, Belize)
Of the five most common snails, smooth tegula was the most abundant with a mean of 9.2 ± 12.7 SD individuals/m2 (Figure 2), followed by stocky cerith (7.2 ± 10.6), long-spined star snail (2.9 ± 3.3), ivory cerith (2.4 ± 3.1), and emerald nerite (2.0 ± 2.7, Figure 2). Smooth tegula was significantly more abundant than all other species (Supplementary Table 1, Poisson GLM and post hoc Estimated Marginal Means analysis, <0.0001) apart from stocky cerith; stocky cerith was also significantly more abundant than the other species (Supplementary Table 1, Poisson GLM and post hoc EMMs, ≤0.0005). Of the five common snail grazers, only two species (smooth tegula and stocky cerith) showed a significant difference in abundances between day and night surveys (Figure 2, Poisson GLM and post hoc EMMs, p ≤ 0.0001), with both species being more abundant at night. During night hours, smooth tegula (17.1 ± 14.1/m2) was the most abundant, followed by stocky cerith (13.1 ± 12.3/m2), emerald nerite (3.2 ± 3.1/m2), and then long-spined star snail and ivory cerith (each were 1.9 ± 3.0/m2).
We found that 56.8% of turtlegrass blades in West Belize had grazing scars and an average of four scars per blade among grazed blades (Figure 3A). Similarly, 57.0% of surveyed turtlegrass blades in South Belize sites had scars, of which the average number of scars was six per blade (Figure 3A). The average scar length on turtlegrass blades in West Belize was 3.26 ± 4.79 mm and 4.25 ± 6.53 mm in South Belize.
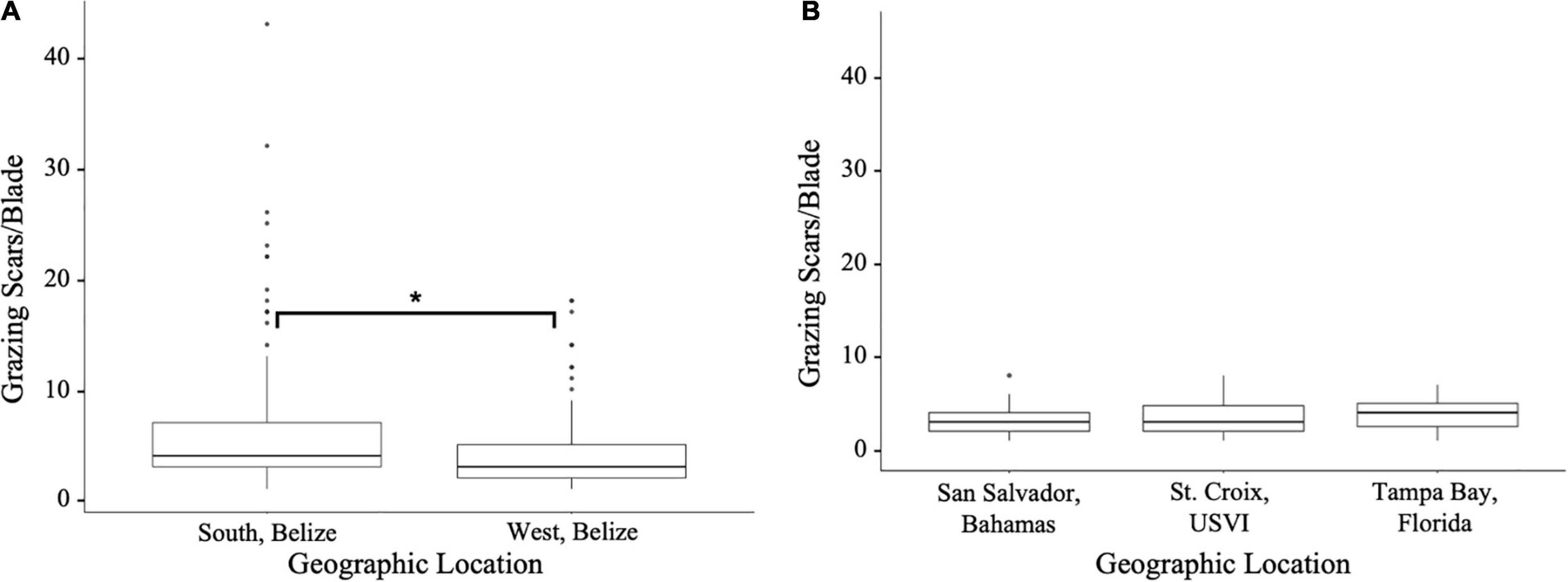
Figure 3. Abundance (per turtlegrass blade) of seagrass scarring detected during field surveys in (A) South and West, Belize, and (B) St. Croix, USVI, San Salvador, Bahamas, and Tampa Bay, Florida. Significant p-values between field sites were calculated through a one-way ANOVA and a Tukey test, *p ≤ 0.01.
Feeding Trials and Density-Mediated Effects (South Water Caye, Belize)
During the 24-h grazing trials, only smooth tegula and emerald nerite exhibited herbivory on the turtlegrass blade. Although the difference was not significant, there was a trend toward emerald nerites producing fewer wounds (4.34 ± 1.75) of a longer length (4.63 ± 2.26 mm) per blade, and smooth tegula producing a greater number of wounds (6.13 ± 2.97) that were shorter in length (3.88 ± 2.53 mm) (one-way ANOVA, p = 0.17 and p = 0.54, for scar length and number of scars, respectively). Based on these findings, there was no clear relationship between snail size and scar abundance or length.
A follow-up feeding trial examining grazing preferences of smooth tegula, showed a six-fold increase in grazing wounds produced on non-epiphytized turtlegrass blades (n = 12 of n = 14), compared to that of epiphytized blades (n = 2 of n = 14). Additionally, the density of smooth tegula exhibited a strong positive correlation with the number of wounds found on seagrass blades in our field surveys (Figure 4, Spearman’s rank correlation test, >0.0001, rho = 0.945122).
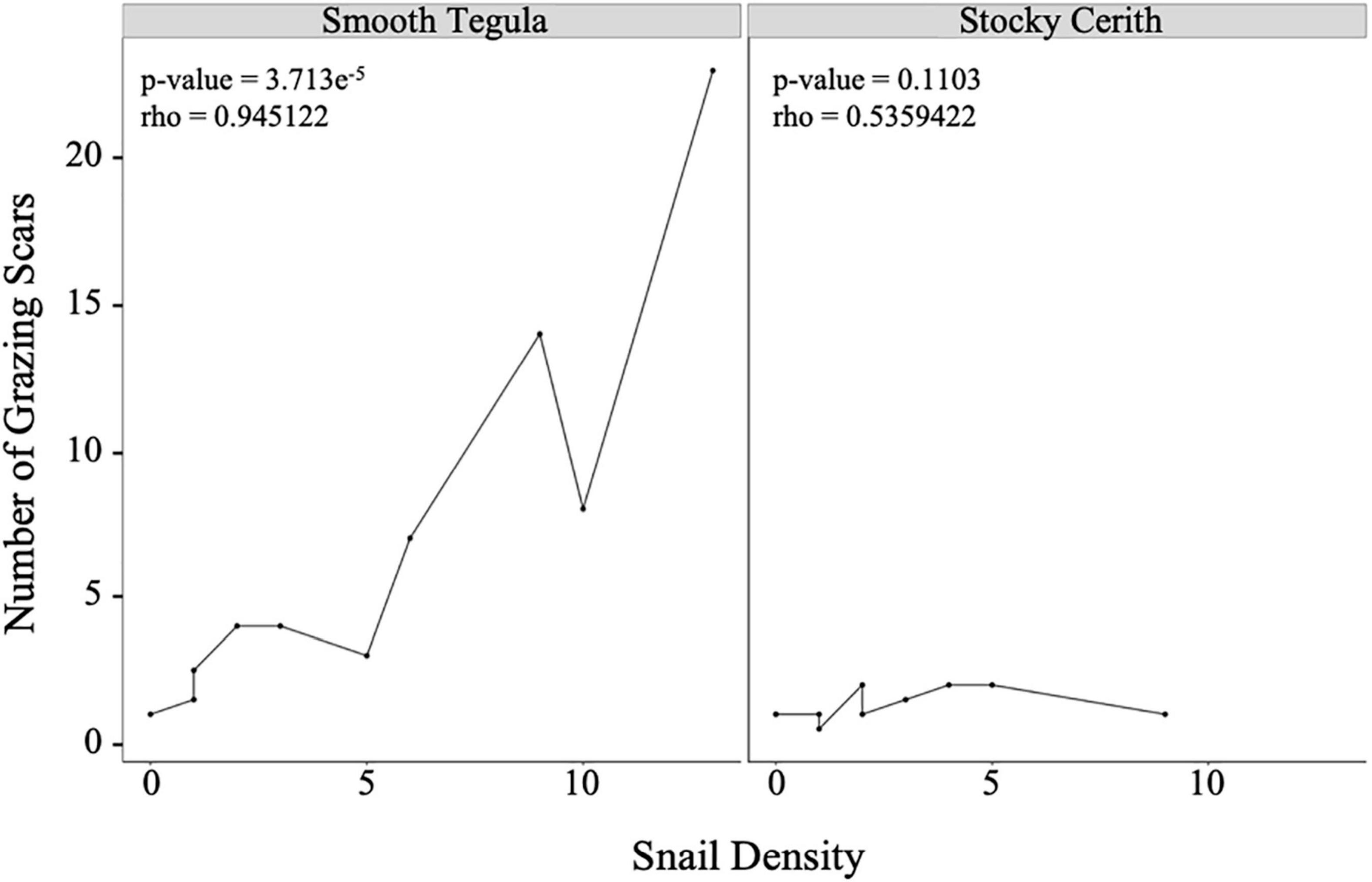
Figure 4. Left: The correlation between number of grazing wounds per turtlegrass blade and smooth tegula (Tegula fasciata) snail density. Right: The correlation between the number of grazing wounds and stocky cerith (Cerithium litteratum) snail density. P-values and adjusted R2 values are reported for the linear model.
Scarring Surveys (St. Croix, USVI, San Salvador, Bahamas, and Tampa Bay, Florida)
Field surveys in St. Croix, San Salvador, and Tampa Bay, revealed 86.7, 96.7, and 90.0%, respectively, of turtlegrass blades surveyed had at least one wound. Grazer wounds per blade averaged 2.9 ± 2.1 SD in St. Croix, 2.8 ± 1.7 in San Salvador, and 3.7 ± 1.9 in Tampa Bay. Wound density (i.e., the number of scars per turtlegrass blade) was not significantly different among these sites (Figure 3B, one-way ANOVA, F = 1.84, p = 0.157).
Simulated Herbivory Experiments (Tampa Bay, Florida)
In Tampa Bay, FL turtlegrass that was manually wounded had significantly higher fungal biomass (0.61 ± 0.24 μg erg/cm2) than those not scarred (0.26 ± 0.11 μg erg/cm2, Figure 5, F = 13.57, p = 0.0025). Staining techniques also revealed that the hyphae of invading fungi could move beyond the scar borders (1–2 cm) into unscarred live tissue. However, the frequency at which fungi invade living turtlegrass tissue is beyond the scope of this analysis.
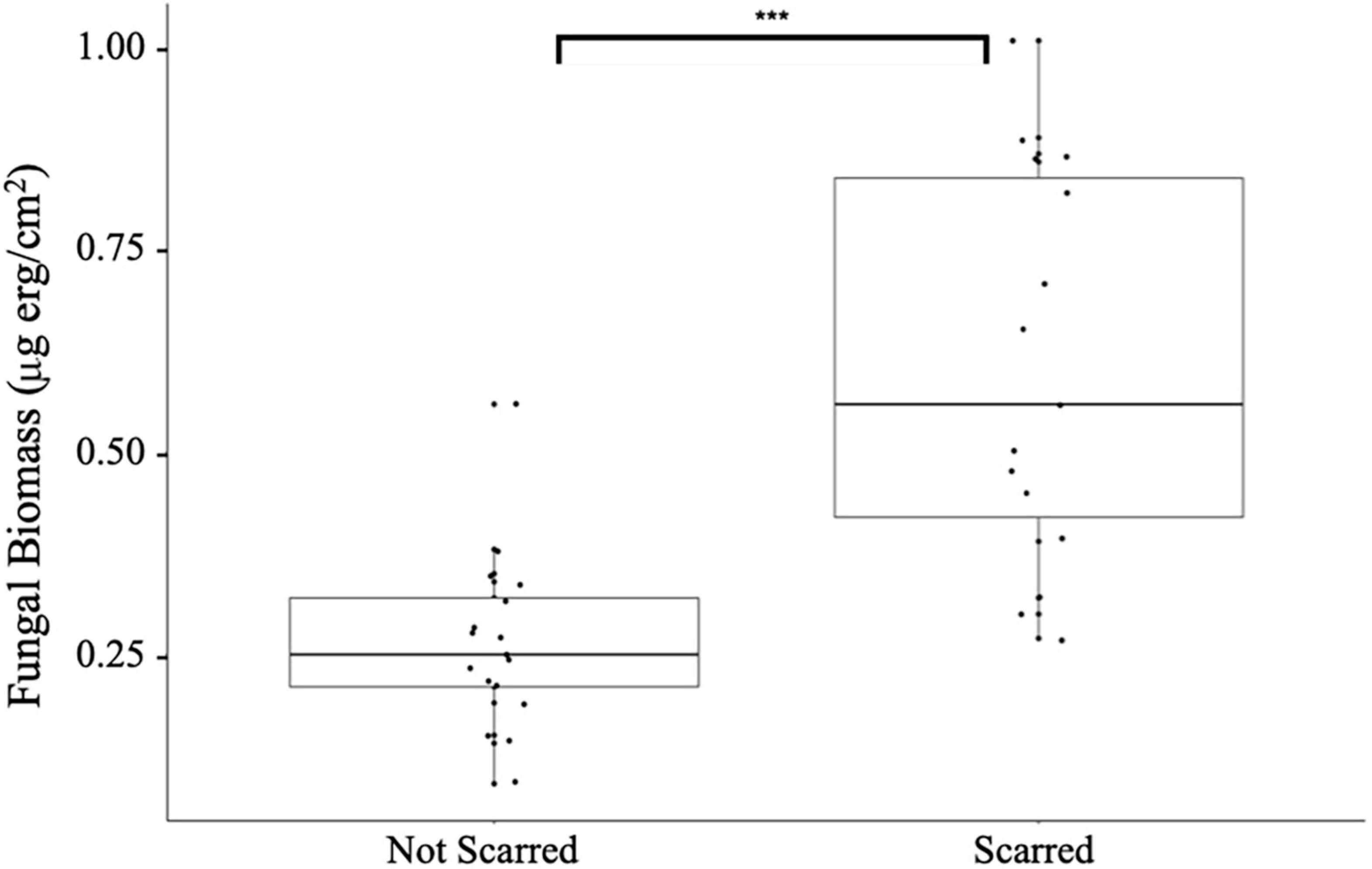
Figure 5. Fungal biomass (μg erg/cm2) on turtlegrass blades that were not manually scared compared to turtlegrass blades that were manually scared. Significant p-values comparing groups were calculated through a one-way ANOVA and a Tukey test, ***p ≤ 0.0001.
Discussion
Our results demonstrate that scarring by snails on live turtlegrass (Thalassia testudinum) is common at the five sites we surveyed that span four regions in the Caribbean (Belize, Florida, San Salvador, and USVI). Observational and experimental evidence show that two commonly occurring snail species (Tegula fasciata – smooth tegula and Smaragdia viridis – emerald nerite) generate scars on turtlegrass blades and that scarring on turtlegrass increases with increasing density of Tegula fasciata. Through scarring experiments, we demonstrated that simulated grazing wounds that damage live turtlegrass blades can facilitate fungal infection with the potential to amplify direct grazing effects. Future studies should investigate context-dependency and generality of this grazer-plant-fungal interaction in seagrasses and whether it can affect direct grazing effects on seagrass growth.
By highlighting the prevalence of snail grazing on live turtlegrass, our results contribute to a growing body of evidence demonstrating that consumption of seagrass by invertebrates is ubiquitous (Lewis and Hollingworth, 1982; Brearley and Walker, 1995; Heck and Valentine, 1995; Nakaoka et al., 2002; Fredriksen et al., 2004; Eklöf et al., 2008; Holzer et al., 2011; Unabia, 2011). Small invertebrates such as snails, chitons, and amphipods have been studied extensively in seagrass systems, however, those studies emphasize how these animal feed on the epiphytic algae that grow on seagrasses or on detritus, rather than directly on the seagrass itself (Orth et al., 1984). Results from our mesocosm feeding trial and surveys support this general trend, given that only a subset of the prevalent snail species were found to directly graze on turtlegrass. However, our results also expand this idea to show that some commonly abundant snails do graze live turtlegrass. Moreover, we found that the most common turtlegrass-grazing snail, smooth tegula, showed a positive, density-dependent relationship and that there was a high prevalence of grazing on turtlegrass at the five sites we surveyed (South Water Caye, Belize: 56.8–57% scarred, St Croix, USVI: 87% scarred, San Salvador, Bahamas: 97% scarred, Tampa Bay, Florida, United States: 90% scarred). This suggests that smooth tegula could be a key link in the direct grazing food-web in seagrass systems. Furthermore, since smooth tegula (and other grazing snails) are common prey items of predators in seagrass beds such as puffers, octopus, grunts, and hogfish (Fawcett, 1984), it is possible that theses predators/consumers control densities of fungal facilitating snails, similar to those in salt marshes (Silliman and Bertness, 2002). Further studies are needed to examine the effect of predator density on snail abundance. Smooth tegula also showed a strong preference for grazing unepiphytized leaves, which suggests that epiphytes could protect turtlegrass blades from direct grazing. Consequently, this also suggests that snails that graze epiphytes could potentially facilitate direct grazing on turtlegrass blades by removing epiphytes from the blade surface. Lastly, grazing snails (i.e., smooth tegula and green nerite) also seemed to be most active and abundant at night, which suggests many wounds are produced nocturnally and could explain why this phenomenon has been overlooked (i.e., most seagrass research takes place during the day). This also suggests that nocturnal predators, such as grunts and octopus, may have the ability to control grazing snail populations in seagrass systems, similar to what has been shown for snails on coral reefs (Shaver et al., 2020).
Our results question the notion in turtlegrass ecology that direct grazing by invertebrates is uncommon (Orth et al., 1984). Our survey results revealed that more than half of all turtlegrass blades (∼56–97%) surveyed in four different regions of the Caribbean were directly grazed by snails. However, our results also highlight the importance of species composition of snails in seagrass, with only two of the five common snail species consuming live seagrass tissue, of which only smooth tegula was particularly abundant during field surveys. This highlights how one species may have disproportionate scarring effects and could thereby have a larger and unique impact on seagrass systems through direct grazing. For example, Duffy et al. (2001) demonstrated amphipod grazer composition had a strong influence on Zostera marina biomass accumulation through grazing on epiphytes. Similarly, we found that direct grazing pressure by snails on seagrass is likely dependent on species composition, providing further evidence that biodiversity of the mesograzer functional group is an important factor in seagrass productivity.
Plant scarring has been shown to facilitate fungal infection in other marine systems. For example, snail scarring on Spartina alterniflora can cause fungal infections that lead to die-off of marsh plants (Silliman, 2005). Our simulated herbivory experiment suggests that herbivore damage to live seagrass blades may facilitate fungal growth in live turtlegrass tissue, similar to what has been shown in marsh systems. We hypothesize that snail grazer-generated scars damage the tissue of turtlegrass, thus creating an entry point for infection and resources for fungal growth within grazer wounds, possibly leading to a harmful infection. However, this hypothesis and the implication for ecosystem health has yet to be tested in seagrass systems, as the wounds produced during our herbivory experiments more closely mimics wounds produced by grazing fish than snails. If grazers do indeed facilitate fungal growth and fungal presence is shown to be detrimental to turtlegrass health, we predict that under physical stressors (i.e., water quality degradation and pollution), the impacts of grazer-facilitated microbial infection on seagrass health could be exacerbated, as has been shown in marshes (Silliman, 2005).
Despite evidence in terrestrial and other marine systems of the strong top-down control invertebrates can exhibit over plant communities, studies of herbivory in seagrass systems have primarily focused on direct consumption by megafauna (turtles, manatees, dugongs, and parrotfish) and indirect grazing effects of mesograzers. While some studies have investigated the role of direct grazing by small invertebrates, such as gastropods and sea urchins (Heinsohn and Birch, 1972; Ogden et al., 1983; Lanyon et al., 1989; Alcoverro and Mariani, 2004; Larkum et al., 2006; Eklöf et al., 2008; Mumby, 2009), relatively few studies have examined the negative impacts of invertebrate grazing on seagrass health (most of those studies have been with urchins). One explanation for the lack of studies investigating how small scars (from invertebrate grazers) on live seagrass tissue affects seagrass health, could be that these abundant scars may have been overlooked because apparent tolerance to such sublethal damage suggests they are not harmful to turtlegrass or their impact may be offset by epiphyte grazing snail species. Additionally, while the scars are commonplace, they may also be overlooked due to the marks of discoloration being difficult to distinguish relative to cropped blades while conducting field surveys (e.g., swimming above seagrass beds) and are only easily seen when looking carefully at collected seagrass blades. Alternatively, invertebrate grazers may have only recently become more important in seagrass beds as a result of overfishing of their predators such as blue crabs and smaller fish (Eriksson et al., 2009). While invertebrate grazers (i.e., mesograzers) have historically been presented as key species that generate positive impacts on seagrass growth through the consumption of epiphytes (Orth and Van Montfrans, 1984), emerging research is demonstrating their ability to have negative cascading impacts on community structure through the consumption of seagrass tissue, especially as their predator populations decline (Alcoverro and Mariani, 2004; Heck and Valentine, 2006).
Our study found pervasive evidence that snails commonly graze on living turtlegrass blades. However, only a few snail species are likely responsible for this grazing pattern that is density-dependent and may result in fungal infection in seagrasses. Further studies are needed to test whether gastropod grazing facilitates fungal infection and the resulting impact on seagrass health, and studies should also test the generality and relative importance of direct and indirect effects of gastropod grazing on tissue senescence, blade turnover, growth, and overall health. Studies should also examine how shifting food webs, disturbance regimes, and environmental stressors may alter the strength of gastropod-seagrass interactions.
Data Availability Statement
The datasets presented in this study can be found in online repositories. The names of the repository/repositories and accession number(s) can be found below: The datasets analyzed for this study can be found on Dataverse in the Experimental and survey data for “Invertebrate grazing on live turtlegrass (Thalassia testudinum): a common but overlooked interaction that can facilitate fungal infection.” repository (https://dataverse.harvard.edu/dataset.xhtml?persistentId=doi:10.7910/DVN/7HWCDF).
Author Contributions
ADB and BRS wrote the original manuscript and interpreted the results. BRS designed all experiments and field surveys. AHA, CC, and BRS collected the data. NSW analyzed the data and produced the manuscript figures. VG processed fungal samples. All authors revised the manuscript and gave final approval for publication.
Funding
This work was supported by the Brown University, National Science Foundation Career grant (#1439504), and the NSF for a CAREER grant to BRS 1056980.
Conflict of Interest
The authors declare that the research was conducted in the absence of any commercial or financial relationships that could be construed as a potential conflict of interest.
Publisher’s Note
All claims expressed in this article are solely those of the authors and do not necessarily represent those of their affiliated organizations, or those of the publisher, the editors and the reviewers. Any product that may be evaluated in this article, or claim that may be made by its manufacturer, is not guaranteed or endorsed by the publisher.
Acknowledgments
The authors would like to thank Brown University for support for a graduate level Tropical Marine Ecology course.
Supplementary Material
The Supplementary Material for this article can be found online at: https://www.frontiersin.org/articles/10.3389/fmars.2021.789380/full#supplementary-material
Supplementary Table 1 | Detailed summary of statistics results, consisting of all one-way ANOVAs, post hoc Tukey tests, Spearman’s rank correlation tests, generalized linear model with a Poisson distribution, and post hoc estimated marginal means pairwise analysis.
References
Alcoverro, T., and Mariani, S. (2004). Patterns of fish and sea urchin grazing on tropical Indo-Pacific seagrass beds. Ecography 27, 361–365. doi: 10.1111/j.0906-7590.2004.03736.x
Brearley, A., and Walker, D. I. (1995). Isopod miners in the leaves of two Western Australian Posidonia species. Aquat. Bot. 52, 163–181. doi: 10.1016/0304-3770(95)00493-9
Bruno, J. F., Boyer, K. E., Duffy, J. E., and Lee, S. C. (2008). Relative and interactive effects of plant and grazer richness in a benthic marine community. Ecology 89, 2518–2528. doi: 10.1890/07-1345.1
Brys, R., Shefferson, R. P., and Jacquemyn, H. (2011). Impact of herbivory on flowering behaviour and life history trade-offs in a polycarpic herb: a 10-year experiment. Oecologia 166, 293–303. doi: 10.1007/s00442-010-1842-7
Camp, D. K., Cobb, S. P., and van Breedveld, J. F. (1973). Overgrazing of seagrasses by a regular urchin, “Lytechinus variegatus.”. BioScience 23, 37–38. doi: 10.2307/1296366
Campbell, J. E., Altieri, A. H., Johnston, L. N., Kuempel, C. D., Paperno, R., Paul, V. J., et al. (2018). Herbivore community determines the magnitude and mechanism of nutrient effects on subtropical and tropical seagrasses. J. Ecol. 106, 401–412. doi: 10.1111/1365-2745.12862
Daleo, P., Silliman, B., Alberti, J., Escapa, M., Canepuccia, A., Peña, N., et al. (2009). Grazer facilitation of fungal infection and the control of plant growth in South-Western Atlantic salt marshes. J. Ecol. 97, 781–787.
Duffy, J. E., Macdonald, K. S., Rhode, J. M., and Parker, J. D. (2001). Grazer diversity, functional redundancy, and productivity in seagrass beds: an experimental test. Ecology 82, 2417–2434.
Eigenbrode, S. D., Bosque-Pérez, N. A., and Davis, T. S. (2018). Insect-borne plant pathogens and their vectors: ecology, evolution, and complex interactions. Annu. Rev. Entomol. 63, 169–191. doi: 10.1146/annurev-ento-020117-043119
Eklöf, J. S., de la Torre-Castro, M., Gullström, M., Uku, J., Muthiga, N., Lyimo, T., et al. (2008). Sea urchin overgrazing of seagrasses: a review of current knowledge on causes, consequences, and management. Estuar. Coast. Shelf Sci. 79, 569–580. doi: 10.1016/j.ecss.2008.05.005
Eriksson, B. K., Ljunggren, L., Sandström, A., Johansson, G., Mattila, J., Rubach, A., et al. (2009). Declines in predatory fish promote bloom-forming macroalgae. Ecol. Appl. 19, 1975–1988. doi: 10.1890/08-0964.1
Fawcett, M. H. (1984). Local and latitudinal variation in predation on an herbivorous marine snail. Ecology 65, 1214–1230. doi: 10.2307/1938329
Fong, J. M., Lai, S., Yaakub, S. M., Ow, Y. X., and Todd, P. A. (2018). The diet and feeding rates of gastropod grazers in Singapore’s seagrass meadows. Botanica Marina 61, 181–192. doi: 10.1515/bot-2017-0091
Fredriksen, S., Christie, H., and Boström, C. (2004). Deterioration of eelgrass (Zostera marina L.) through destructive grazing by the gastropod Rissoa membranacea (J. Adams). Sarsia 89, 218–222. doi: 10.1080/00364820410005593
Furbish, C. E., and Albano, M. (1994). Selective herbivory and plant community structure in a Mid-Atlantic salt marsh. Ecology 75, 1015–1022. doi: 10.2307/1939425
Gulis, V., and Bärlocher, F. (2017). “Fungi: biomass, production, and community structure,” Methods in Stream Ecology Vol. 1, eds F. R. Hauer and G. A. Lamberti (San Diego, CA: Academic Press) 177–192. doi: 10.1016/B978-0-12-416558-8.00010-X
Hawkins, S. J., and Hartnoll, R. G. (1983). Grazing of intertidal algae by marine invertebrates. Oceanogr. Mar. Biol. 21, 195–282.
He, Q., and Silliman, B. R. (2016). Consumer control as a common driver of coastal vegetation worldwide. Ecol. Monogr. 86, 278–294. doi: 10.1002/ecm.1221
He, Q., Bertness, M. D., and Altieri, A. H. (2013). Global shifts towards positive species interactions with increasing environmental stress. Ecol. Lett. 16, 695–706. doi: 10.1111/ele.12080
Heck, K. L., and Valentine, J. F. (1995). Sea urchin herbivory: evidence for long-lasting effects in subtropical seagrass meadows. J. Exp. Mar. Biol. Ecol. 189, 205–217. doi: 10.1016/0022-0981(95)00012-G
Heck, K. L., and Valentine, J. F. (2006). Plant–herbivore interactions in seagrass meadows. J. Exp. Mar. Biol. Ecol. 330, 420–436. doi: 10.1016/j.jembe.2005.12.044
Heck, K. L., Pennock, J. R., Valentine, J. F., Coen, L. D., and Sklenar, S. A. (2000). Effects of nutrient enrichment and small predator density on seagrass ecosystems: an experimental assessment. Limnol. Oceanogr. 45, 1041–1057. doi: 10.4319/lo.2000.45.5.1041
Heinsohn, G. E., and Birch, W. R. (1972). Foods and feeding habits of the dugong, Dugong dugong (Erxleben), in northern Queensland, Australia. Mammalia 36, 414–422. doi: 10.1515/mamm.1972.36.3.414
Hik, D. S., and Jefferies, R. L. (1990). Increases in the net above-ground primary production of a salt-marsh forage grass: a test of the predictions of the herbivore-optimization model. J. Ecol. 78, 180–195. doi: 10.2307/2261044
Holzer, K. K., Rueda, J. L., and McGlathery, K. J. (2011). Differences in the feeding ecology of two seagrass-associated snails. Estuar. Coasts 34, 1140–1149. doi: 10.1007/s12237-011-9406-6
Hughes, A., Bando, K., Rodriguez, L., and Williams, S. (2004). Relative effects of grazers and nutrients on seagrasses: a meta-analysis approach. Mar. Ecol. Progr. Ser. 282, 87–99. doi: 10.3354/meps282087
Huntly, N. (1991). Herbivores and the dynamics of communities and ecosystems. Annu. Rev. Ecol. Syst. 22, 477–503. doi: 10.1146/annurev.es.22.110191.002401
Kollars, N. M., Henry, A. K., Whalen, M. A., Boyer, K. E., Cusson, M., Eklöf, J. S., et al. (2017). Meta-analysis of reciprocal linkages between temperate seagrasses and waterfowl with implications for conservation. Front. Plant Sci. 8:2119. doi: 10.3389/fpls.2017.02119
Lanyon, J. M., Limpus, C. J., and Marsh, H. (1989). “Dugongs and turtles: grazers in the seagrass system,” in Biology of Seagrasses: A Treatise on the Biology of Seagrasses with Special Reference to the Australian Region, eds A. W. D. Larkum, McComb AJ, and S. A. Shepherd (Amsterdam: Elsevier), 610–634.
Larkum, A. W. D., Orth, R. J., and Duarte, C. M. (eds) (2006). Seagrasses: Biology, Ecology, and Conservation. Dordrecht: Springer.
Lewis, J. B., and Hollingworth, C. E. (1982). Leaf epifauna of the seagrass Thalassia testudinum. Mar. Biol. 71, 41–49. doi: 10.1007/BF00396991
Lewis, J. T., and Boyer, K. E. (2014). Grazer functional roles, induced defenses, and indirect interactions: implications for eelgrass restoration in San Francisco Bay. Diversity 6, 751–770. doi: 10.3390/d6040751
Linit, M. J. (1988). Nematode-vector relationships in the pine wilt disease system. J. Nematol. 20, 227–235.
Maschinski, J., and Whitham, T. G. (1989). The continuum of plant responses to herbivory: the influence of plant association, nutrient availability, and timing. Am. Nat. 134, 1–19.
Mingo, A., and Oesterheld, M. (2009). Retention of dead leaves by grasses as a defense against herbivores. A test on the palatable grass Paspalum dilatatum. Oikos 118, 753–757. doi: 10.1111/j.1600-0706.2008.17293.x
Mumby, P. J. (2009). Herbivory versus corallivory: are parrotfish good or bad for Caribbean coral reefs? Coral Reefs 28, 683–690. doi: 10.1007/s00338-009-0501-0
Nakaoka, M., Mukai, H., and Chunhabundit, S. (2002). Impacts of dugong foraging on benthic animal communities in a Thailand seagrass bed: impacts of dugongs on benthic communities. Ecol. Res. 17, 625–638. doi: 10.1046/j.1440-1703.2002.00520.x
Newell, S. Y. (2002). “Fungi in marine/estuarine waters,” in Encyclopedia of Environmental Microbiology, ed. G. Bitton (New York, NY: Wiley), 1394–1400.
Nienhuis, P., and Groenendijk, A. (1986). Consumption of eelgrass (Zostera marina) by birds and invertebrates: an annual budget. Mar. Ecol. Progr. Ser. 29, 29–35. doi: 10.3354/meps029029
Ogden, J., Robinson, L., Whitlock, K., Daganhardt, H., and Cebula, R. (1983). Diel foraging patterns in juvenile green turtles (Chelonia mydas) in St Croix United States Virgin Islands. J. Exp. Mar. Biol. Ecol. 66, 199–205. doi: 10.1016/0022-0981(83)90160-0
Olff, H., and Ritchie, M. E. (1998). Effects of herbivores on grassland plant diversity. Trends Ecol. Evol. 13, 261–265. doi: 10.1016/S0169-5347(98)01364-0
Orth, R. J., and Van Montfrans, J. (1984). Epiphyte-seagrass relationships with an emphasis on the role of micrograzing: a review. Aquat. Bot. 18, 43–69. doi: 10.1016/0304-3770(84)90080-9
Orth, R. J., Heck, K. L., and van Montfrans, J. (1984). Faunal communities in seagrass beds: a review of the influence of plant structure and prey characteristics on predator-prey relationships. Estuaries 7:339. doi: 10.2307/1351618
Parr, C. S., Wilson, N., Leary, P., Schulz, K. S., Lans, K., Walley, L., et al. (2014). The encyclopedia of Life v2: providing global access to knowledge about life on earth. Biodiv. Data J. e1079. doi: 10.3897/BDJ.2.e1079
Pimentel, D. (1991). Diversification of biological control strategies in agriculture. Crop Protect. 10, 243–253. doi: 10.1016/0261-2194(91)90001-8
Poore, A. G., Campbell, A. H., Coleman, R. A., Edgar, G. J., Jormalainen, V., Reynolds, P. L., et al. (2012). Global patterns in the impact of marine herbivores on benthic primary producers. Ecol. Lett. 15, 912–922. doi: 10.1111/j.1461-0248.2012.01804.x
Renzi, J., and Silliman, B. (2020). Increasing grazer density leads to linear decreases in Spartina alterniflora biomass and exponential increases in grazing pressure across a barrier island. Mar. Ecol. Progr. Ser. 659, 49–58. doi: 10.3354/meps13569
Reynolds, P. L., Richardson, J. P., and Duffy, J. E. (2014). Field experimental evidence that grazers mediate transition between microalgal and seagrass dominance. Limnol. Oceanogr. 59, 1053–1064. doi: 10.4319/lo.2014.59.3.1053
Shaver, E. C., Renzi, J. J., Bucher, M. G., and Silliman, B. R. (2020). Relationships between a common Caribbean corallivorous snail and protected area status, coral cover, and predator abundance. Sci. Rep. 10:16463. doi: 10.1038/s41598-020-73568-1
Silliman, B. R. (2005). Drought, snails, and large-scale die-off of southern U.S. salt marshes. Science 310, 1803–1806. doi: 10.1126/science.1118229
Silliman, B. R., and Bertness, M. D. (2002). A trophic cascade regulates salt marsh primary production. Proc. Natl. Acad. Sci. U.S.A. 99, 10500–10505. doi: 10.1073/pnas.162366599
Silliman, B. R., and Newell, S. Y. (2003). Fungal farming in a snail. Proc. Natl. Acad. Sci. U.S.A. 100, 15643–15648. doi: 10.1073/pnas.2535227100
Silliman, B. R., and Zieman, J. C. (2001). Top-down control of Spartina alterniflora production by periwinkle grazing in a Virginia salt marsh. Ecology 82, 2830–2845.
Silliman, B. R., Layman, C. A., Geyer, K., and Zieman, J. C. (2004). Predation by the black-clawed mud crab, Panopeus herbstii, in Mid-Atlantic salt marshes: further evidence for top-down control of marsh grass production. Estuaries 27, 188–196. doi: 10.1007/BF02803375
Smith, T. J. (1987). Seed predation in relation to tree dominance and distribution in mangrove forests. Ecology 68, 266–273. doi: 10.2307/1939257
Smith, T. J., and Odum, W. E. (1981). The effects of grazing by snow geese on coastal salt marshes. Ecology 62, 98–106. doi: 10.2307/1936673
Sousa, W. P., and Mitchell, B. J. (1999). The effect of seed predators on plant distributions: is there a general pattern in mangroves? Oikos 86, 55–66. doi: 10.2307/3546569
Taylor, W. E., and Bardner, R. (1968). Leaf injury and food consumption by larvae of Phaedon cochleariae (Coleoptera; Chrysomelidae) and Plutella maculipennis (Lepidoptera; Plutellidae) feeding on turnip and radish. Entomol. Exp. Appl. 11, 177–184. doi: 10.1111/j.1570-7458.1968.tb02043.x
Turner, M. G. (1989). Landscape ecology: the effect of pattern on process. Annu. Rev. Ecol. Syst. 20, 171–197. doi: 10.1146/annurev.es.20.110189.001131
Unabia, C. R. C. (2011). The snail Smaragdia bryanae (Neritopsina, Neritidae) is a specialist herbivore of the seagrass Halophila hawaiiana (Alismatidae, Hydrocharitaceae): gastropod grazing on a seagrass. Invertebr. Biol. 130, 100–114. doi: 10.1111/j.1744-7410.2011.00225.x
Valentine, J. F., and Duffy, J. E. (2006). “The central role of grazing in seagrass ecology,” in Seagrasses: Biology, Ecology, and Conservation, eds A. W. D. Larkum, R. J. Orth, and C. M. Duarte (Dordrecht: Springer Netherlands), 463–501. doi: 10.1007/978-1-4020-2983-7_20
Valentine, J., and Heck, K. (1991). The role of sea urchin grazing in regulating subtropical seagrass meadows: evidence from field manipulations in the Northern Gulf of Mexico. J. Exp. Mar. Biol. Ecol. 154, 215–230. doi: 10.1016/0022-0981(91)90165-S
Vickery, P. J. (1972). Grazing and net primary production of a temperate grassland. J. Appl. Ecol. 9, 307–314. doi: 10.2307/2402064
Zieman, J., Iverson, R., and Ogden, J. (1984). Herbivory effects on Thalassia testudinum leaf growth and nitrogen content. Mar. Ecol. Progr. Ser. 15, 151–158. doi: 10.3354/meps015151
Keywords: seagrass, fungal infection, snails, herbivory, mesograzers
Citation: Boyd AD, Walker NS, Valdez SR, Zhang YS, Altieri AH, Gulis V, Crain C and Silliman B (2022) Invertebrate Grazing on Live Turtlegrass (Thalassia testudinum): A Common Interaction That May Facilitate Fungal Growth. Front. Mar. Sci. 8:789380. doi: 10.3389/fmars.2021.789380
Received: 04 October 2021; Accepted: 03 December 2021;
Published: 12 January 2022.
Edited by:
Thanos Dailianis, Hellenic Centre for Marine Research, GreeceReviewed by:
Katharyn Boyer, San Francisco State University, United StatesJohan Sven Eklöf, Stockholm University, Sweden
Copyright © 2022 Boyd, Walker, Valdez, Zhang, Altieri, Gulis, Crain and Silliman. This is an open-access article distributed under the terms of the Creative Commons Attribution License (CC BY). The use, distribution or reproduction in other forums is permitted, provided the original author(s) and the copyright owner(s) are credited and that the original publication in this journal is cited, in accordance with accepted academic practice. No use, distribution or reproduction is permitted which does not comply with these terms.
*Correspondence: Anjali D. Boyd, YW5qYWxpLmJveWRAZHVrZS5lZHU=