- 1ESSO-National Centre for Polar and Ocean Research, Ministry of Earth Sciences, Vasco-da-Gama, India
- 2School of Earth, Ocean and Atmospheric Sciences (SEOAS), Goa University, Taleigao, India
- 3Shirshov Institute of Oceanology, Russian Academy of Sciences, Moscow, Russia
The subpolar North Atlantic (SPNA) Ocean has complex hydrography, and moderates the global climate through the Atlantic Meridional Overturning Circulation (AMOC). The surface water mass dynamics in SPNA and the upper limb of AMOC, govern the plankton distribution. Specifically, the habitat of modern planktic foraminifera is strongly affected by the SPNA hydrography. In the present study, 25 surface sediment samples from the Labrador Sea to the Iceland-Faroe-Shetland Channel (IFSC) were examined for planktic foraminifera distribution along a latitudinal transect at 59.50°N. The planktic foraminifera distribution followed the transition in water mass structure in the study area from the Sub-Arctic water in the west to the warm North Atlantic water in the east. Temperature and salinity are two dominant ecological factors controlling planktic foraminifera assemblages in the region. This hydrographic contrast was also reflected in the ratio of Neogloboquadrina pachyderma/Neogloboquadrina incompta along the transect. Based on the cluster analysis, the planktic foraminifera assemblages could be assigned to three groups. A cold/polar group in the Labrador Sea, a mixed (both cold and warm) group in the Irminger Sea and IFSC, and a warmer temperate group in the eastern part of the transect were represented by different planktic foraminifera assemblages. Additionally, a decrease in Globorotalia inflata in the eastern transect and an increase in Turborotalita quinqueloba in the Iceland basin and Irminger Sea was observed in our study when compared with the published dataset. From this, we suggest a shift in planktic assemblages in the SPNA. The present study on the distribution of modern planktic foraminifera can help paleoceanographic reconstructions in the SPNA ocean.
Introduction
Planktic foraminifera are unicellular protists widely distributed in the modern oceans. They thrive in the upper ocean water column and are constrained by various environmental parameters, especially water mass characteristics (temperature, salinity, nutrients, etc.) and food availability (Parker, 1960; Bé and Tolderlund, 1971; CLIMAP Project Members, 1976; Ortiz and Mix, 1992; Schiebel and Hemleben, 2005, 2017; Rebotim et al., 2017). The calcium carbonate shells of planktic foraminifera are one of the major biogenic components of marine sediments. Planktic foraminifera species and assemblages are excellent indicators of ambient environmental factors and have been widely used to infer paleoenvironments (Kucera, 2007; Schiebel et al., 2017). The depth stratification and ecological preferences of planktic foraminifera play a vital role in reconstructing paleoceanographic changes. However, the paleoenvironmental reconstructions rely on a better understanding of the modern foraminiferal ecology and factors governing their abundance in different water masses. The distribution and ecology of planktic foraminifera have been studied in the plankton tows, sediment traps, and surface sediments from the world oceans (Berger, 1969; Thunell and Reynolds, 1984; Schiebel and Hemleben, 2000; Schiebel et al., 2001; Jonkers et al., 2010; Meilland et al., 2020). The studies referred are either confined to different basins or show regional heterogeneity. A complete ecological and spatial distribution of planktic foraminifera is understudied in the subpolar North Atlantic (SPNA) ocean, an area influenced by the large-scale changes in the surface hydrography on both short and large timescale (such as seasonal, annual, interannual, and decadal).
The SPNA exhibits significant west to east oceanographic gradients from the subarctic to temperate regions (McCartney and Talley, 1982; Read, 2000; Brambilla and Talley, 2008). In particular, the SPNA hydrography is characterized by the presence and interaction of different warm and cold surface water currents (Read, 2000; García-Ibáñez et al., 2015). The strength and extent of the Subpolar Gyre (SPG) influence the hydrography in the SPNA (Hátún et al., 2005; Staines-Urías et al., 2013). The hydrographic diversity strongly affects the distribution of modern planktic foraminifera in the SPNA, resulting in the occurrence of several cold-, warm- and mixed-water planktic foraminifera associations (Bé and Tolderlund, 1971; Ottens, 1991; Schiebel et al., 2001).
The enhanced warming of the high latitude regions in recent decades has led to major oceanographic shifts and resulted in the changing ecology of various planktic faunas (Fossheim et al., 2015; Oziel et al., 2017; Neukermans et al., 2018; Jonkers et al., 2019). Especially, planktic foraminiferal assemblages in high latitude oceans have shown a change in diversity, with an influx of temperate species (Stangeew, 2001; Schiebel et al., 2017; Jonkers et al., 2019; Meilland et al., 2020). As opposed to the single dominant species found in polar regions (Bé and Tolderlund, 1971), several plankton tow experiments reported a rise in warmer-water foraminiferal species in polar regions (Stangeew, 2001; Jonkers et al., 2019; Meilland et al., 2020). The distribution of modern planktic foraminifera reported from the SPNA ocean is mainly confined to either the western (Stangeew, 2001; Jonkers et al., 2010) or the eastern SPNA ocean (Schiebel and Hemleben, 2000). These studies are based mainly upon plankton tows and infer the planktic foraminifera populations with respect to seasonality. Several studies discuss the variations in planktic foraminifera assemblage structure in a latitudinal as well as a longitudinal transect, pertaining to a change in water mass structure in Nordic seas as well as in the Subarctic Pacific (Stangeew, 2001; Kuroyanagi and Kawahata, 2004; Chapman, 2010; Pados and Spielhagen, 2014; Meilland et al., 2020); but not in the SPNA ocean. Thus, the main objective of the present study is to assess the spatial distribution of planktic foraminiferal assemblages and their ecological preferences in the SPNA ocean. Moreover, this becomes interesting as SPNA has complex hydrography, showing an east-west gradient in its physiographic properties, which significantly modulates the planktic foraminiferal distribution. This study, covering the entire SPNA from west to east, would improve our understanding of planktic foraminifera distribution related to various environmental parameters of different water masses and significant shifts in high-latitude regions.
Oceanographic Context
The SPNA Ocean has a very complex hydrographic system owing to the interaction of different types of surface currents, such as the warm North Atlantic Current (NAC) and its derivatives- East Reykjanes Ridge Current (ERRC), Irminger Current (IC), and cold fresh East Greenland Current (EGC), West Greenland Current (WGC), and Labrador Current (LC) affecting the diverse regional environments (Pollard et al., 1996; Read, 2000; Brambilla and Talley, 2008; Daniault et al., 2016). The NAC, an extension of the Gulf stream, brings warm and saline water to the region through various branches (Daniault et al., 2016; Figure 1A). EGC brings cold and less saline water to the SPNA ocean along the eastern coast of Greenland. These currents and the associated water masses are controlled by the dynamics of cyclonic Subpolar Gyre (SPG). A strengthened and expanded SPG brings more subpolar water from the western SPNA to the eastern SPNA through the eastern limb of SPG. A weakened and contracted gyre brings subtropical warm water to the east SPNA through the eastern limb of SPG (Staines-Urías et al., 2013). The upper water mass at the east SPNA gets colder and denser as it travels downstream toward the Labrador Sea through mixing with the surrounding subpolar water mass and air-sea interaction (Read, 2000).
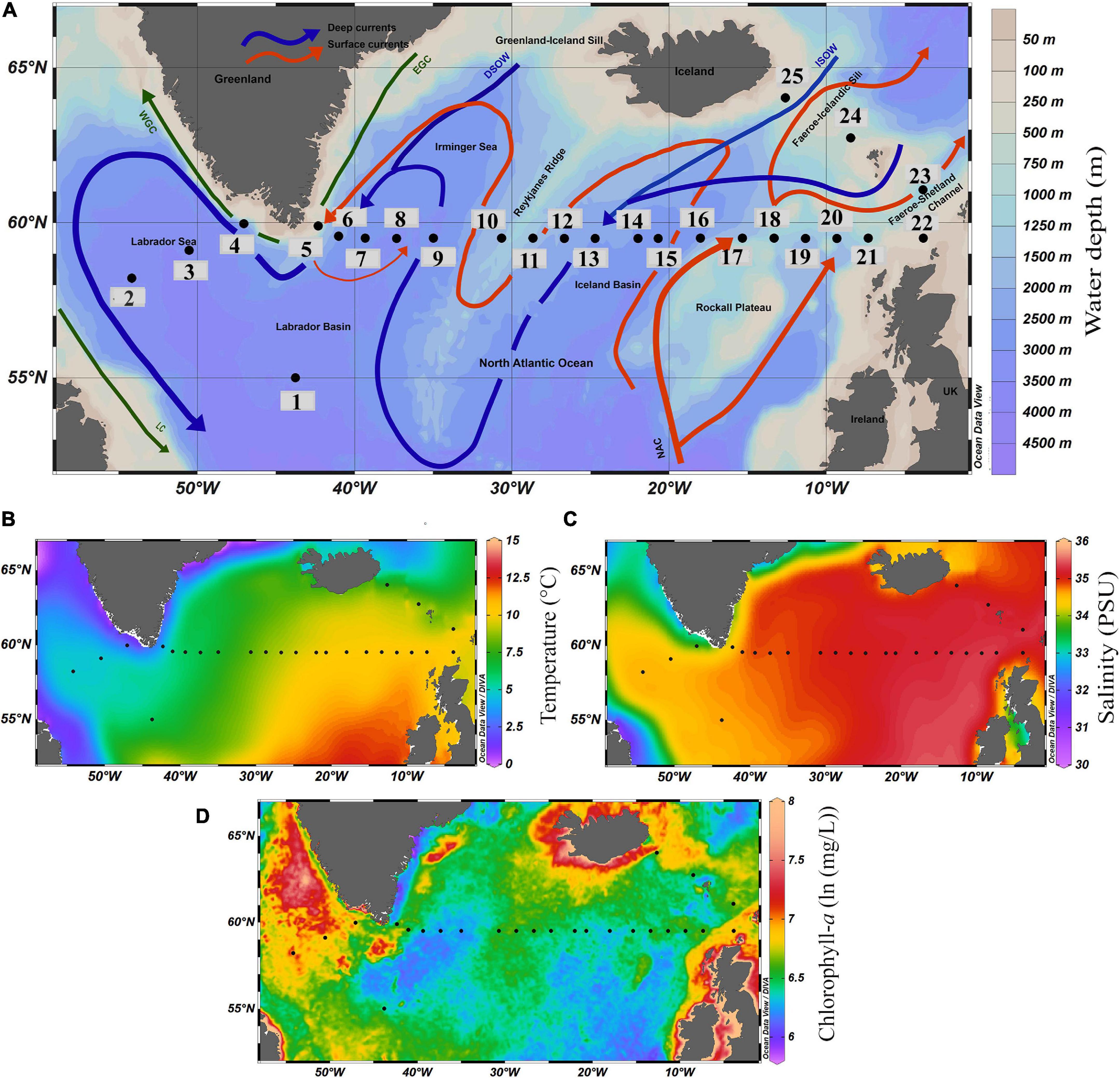
Figure 1. (A) Map showing locations of surface sediment samples and major ocean currents in subpolar North Atlantic Ocean (Schlitzer, 2015). Total 25 samples along 59.50°N latitude were retrieved during Akademik Ioffe 51 cruise; the black dots represent the locations of the surface sediment samples and the numbers denote the name of the stations. Warm and cold surface currents are in red and green, respectively, and cold deep currents are shown in blue color. NAC, North Atlantic Current; EGC, East Greenland Current; WGC, West Greenland Current; ISOW, Iceland-Scotland Overflow water; DSOW, Denmark Strait Overflow Water; LC, Labrador Current; Map showing surficial distribution of, (B) Annual average Sea Surface Temperature (SST,°C). (C) Annual average Sea Surface Salinity (SSS, PSU), and (D) Annual average Chlorophyll-a [ln (mg/L)] of the modern ocean.
In general, alternating warm and cold water currents create a prominent hydrological contrast along the studied transect at 59.50°N (Figures 1B–D). The annual average temperature and salinity of the surface water (averaged for a water depth of 0–100 m) vary from 10.33°C and 35.28 PSU on the eastern side, through 8.12°C and 35.00 PSU on the central part near Reykjanes Ridge, to 4.60°C and 34.57 PSU in the Labrador Sea (Locarnini et al., 2018; Zweng et al., 2018). The primary productivity in the region (chlorophyll-a concentration) ranges from 5.80 [ln (mg/L)] to 8.00 [ln (mg/L)] (Figure 1D).
The hydrographic dynamics in the SPNA are associated with different-scale eddies and hydrological fronts, bringing more nutrient-rich water to the region. The warm subtropical water from the NAC and cold subpolar water are separated by the subpolar front, creating a highly productive region (Taylor and Ferrari, 2011a). The eddies associated with Iceland Basin and Irminger Sea bring more nutrient-rich water to the surface (Mahadevan et al., 2012). This makes the SPNA a highly productive region (Taylor and Ferrari, 2011a; Mahadevan et al., 2012). Also, the extent and strength of the SPG circulation directly impact productivity changes in SPNA (Hátún et al., 2009). The increased freshening of the SPNA and decreased Atlantic Meridional Overturning Circulation (AMOC) strength have a negative effect on primary productivity (Osman et al., 2019). The productivity of plankton communities in SPNA exhibits clear seasonal character peaking from spring to autumn after the shutdown of winter convection and the formation of stable surface stratification (Taylor and Ferrari, 2011b). Primary productivity, along with the other physical parameters, controls the distribution of planktic foraminifera assemblages (Bé and Tolderlund, 1971; Schiebel and Hemleben, 2017). The abundance of foraminifera species is also linked with chlorophyll-a (Chl-a) concentration (Schiebel et al., 2001; Kuroyanagi and Kawahata, 2004; Retailleau et al., 2011).
Materials and Methods
Sediment Samples
A total of 25 surface sediment samples were collected using Van Veen grab sampler on board the R/V Akademik Ioffe 51st cruise during the summer (June–July) of 2016. The top sediment (0–1 cm) section was carefully scooped from the collected samples for this study. The samples were collected along a latitudinal transect from the Labrador Sea in the west, to Iceland-Faroe-Shetland Ridge (IFSR) in the east of the SPNA ocean, with a depth varying between 158 m at station 22 and 3,477 m at station 3 (Figure 1A). Of these 25 stations, 21 were from the west-east transect along latitude ∼59.50°N; one sample was from 55.00°N in the Labrador Basin, and three were from a southeast-northwest transect in IFSR (Figure 1A; for more information, see Supplementary Table 1).
Foraminiferal Counts and Calcium Carbonate Content
Around ∼5 g sediment was freeze-dried per sample and soaked in de-ionized water for 6 h, followed by wet sieving through 63 and 500 μm sieves. The >63 μm residue was then dry sieved over >100 μm mesh. The size fraction of >100 μm was chosen for faunal study to avoid any underestimation of small-sized species, e.g., Turborotalita quinqueloba, Globigerinita uvula following previous studies (Bauch, 1994; Kandiano and Bauch, 2002; Husum and Hald, 2012). The sieved fraction of >100 μm was split using a micro-splitter until a minimum of 300 planktic foraminifera specimens were picked from each sample (CLIMAP Project Members, 1976). The planktic foraminifera species were counted and identified to species level using a stereo-zoom microscope (Nikon SMZ1500 with the magnification of 112X) following the taxonomic nomenclature by Kennett and Srinivasan (1983) and Hemleben et al. (1989). The planktic foraminifera specimens were well preserved in all the samples, and no significant fragmentation of the planktic foraminifera was found. The total planktic foraminifera abundances (TPF) per one gram of dry sediment (ind. × g–1), the individual species abundances per one gram of dry sediment (ind. × g–1), and their relative abundances (%) were calculated.
For the estimation of the CaCO3 content, we measured the Total Inorganic Carbon (TIC, weight%) of all the samples using a Coulometer (UIC, Inc. CM5017). We followed the method of Espitalié et al. (1977) to estimate the CaCO3%,
In Eqs. 1, 2, TC is Total Carbon and TOC is Total Organic Carbon.
Environmental Parameters
The annual average temperature and salinity data were obtained from the World Ocean Atlas 2018 (WOA18)1 from the National Oceanic and Atmospheric Administration (NOAA) (Locarnini et al., 2018; Zweng et al., 2018). Both temperature and salinity data were averaged over 0–100 m of water depth. The depth range (0–100 m) was preferred due to the occurrence of the maximum abundance of planktic foraminifera in the upper 100 m water column in the northeast Atlantic (Schiebel and Hemleben, 2000). Annual average Chl-a (mg m–3) data was taken from the Moderate Resolution Imaging Spectroradiometer (MODIS) (NASA Goddard Space Flight Center, 2018) Chl-a concentration Level 3 data2 from the year 2001 to 2018 (Figure 1D). These data were used to analyze the relationship between planktic foraminifera distribution and main environmental variables along the transect.
Statistical Analyses
Statistical analyses were carried out to facilitate the understanding between various environmental parameters and the relative species abundance. A strict criterion was followed wherein species showing relative abundances greater than 2% in at least three stations were considered for statistical analyses.
Principal Component Analysis
Principal Component Analysis (PCA) was performed on the environmental parameters, such as temperature, salinity, water depth of sampling point, Chl-a with total planktic foraminifera abundances, and species percentages using the software STATISTICA v. 10 (StatSoft). This method of statistical correlation has been used to understand the major environmental factors responsible for variation in the distribution of the planktic foraminifera assemblages (Mallo et al., 2017). The marked correlations are significant at a p < 0.05.
Cluster Analysis
A hierarchical cluster analysis was performed on the planktic foraminifera assemblages to delineate different groups of stations using the Bray Curtis similarity index. Each group was represented by its characteristic foraminiferal assemblage. This analysis has been used previously to identify the relationship between the group of stations and different water masses based on their specific planktic foraminifera assemblages (Parker and Berger, 1971; Thunell, 1978; Ottens, 1991). We also performed the similarity percentages (SIMPER) analysis (Clarke, 1993) to determine the individual species contribution to each cluster group.
Results
Total Planktic Foraminifera and Calcium Carbonate
The TPF varied between 1,212 and 183,557 ind. g–1. The highest abundance occurred at station 10 (water depth of 1,531 m) near the Reykjanes Ridge, while the lowest abundance was at station 23 (water depth of 158 m), in Faroe-Shetland Channel (FSC). Interestingly, few stations (2, 3, 4, and 13) located at deeper depths in the Labrador Sea, east of Greenland and Reykjanes Ridge exhibited low TPF, along with the samples at shallow regions (5, 24, and 25), which are generally marked by the lowest TPF (Figures 2A,B). Subsequently, the CaCO3 content in the surface sediments varied from 9% at station 4 (water depth of 2,389 m), near the southwest coast of Greenland, to 74% at station 17 (water depth of 1,514 m), at the western side of the Rockall Plateau. Furthermore, the TPF and CaCO3 content varied concomitantly (Figure 2A).
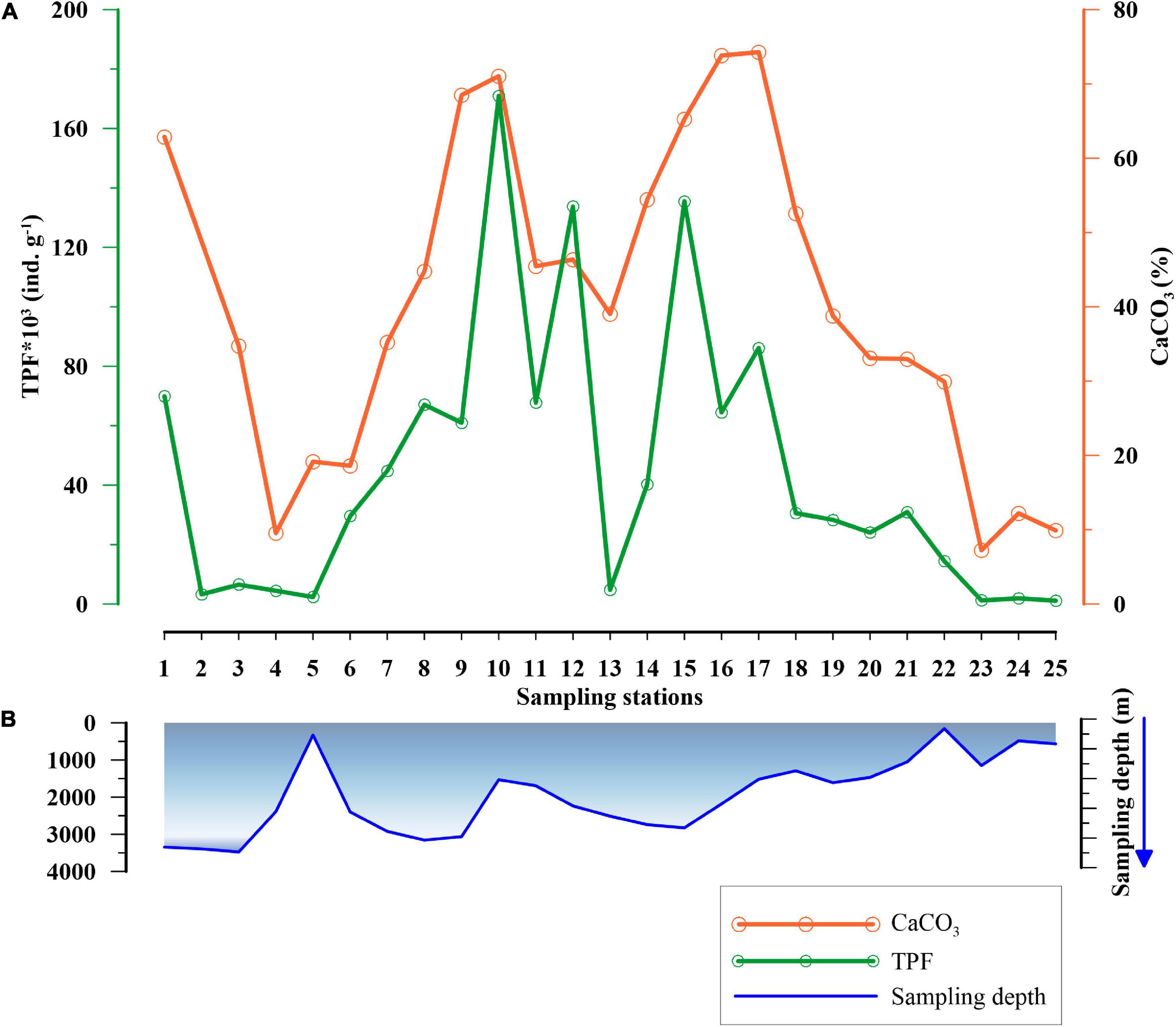
Figure 2. (A) Variation in Total Planktic Foraminiferal (TPF) abundance in numbers per gram sediment (ind. g–1) (in green) and calcium carbonate (CaCO3)% (in orange) at different stations, and (B) the blue curve depicts the sampling depth (m) of the stations in the subpolar North Atlantic Ocean.
We found significant variations in the distribution of planktic foraminifera species along the transect. A total of nine species occurred in our samples: Globigerinita glutinata, Neogloboquadrina pachyderma, Neogloboquadrina incompta, Turborotalita quinqueloba, Globigerina bulloides, Globigerinita uvula, Globorotalia inflata, Globorotalia scitula, and Orbulina sp. (Figure 3). The list of planktic foraminifera species and their ecological information can be found in Supplementary Table 2. Among all, the former six species were the most abundant. Together, they contribute at least 80% to the planktic foraminifera community.
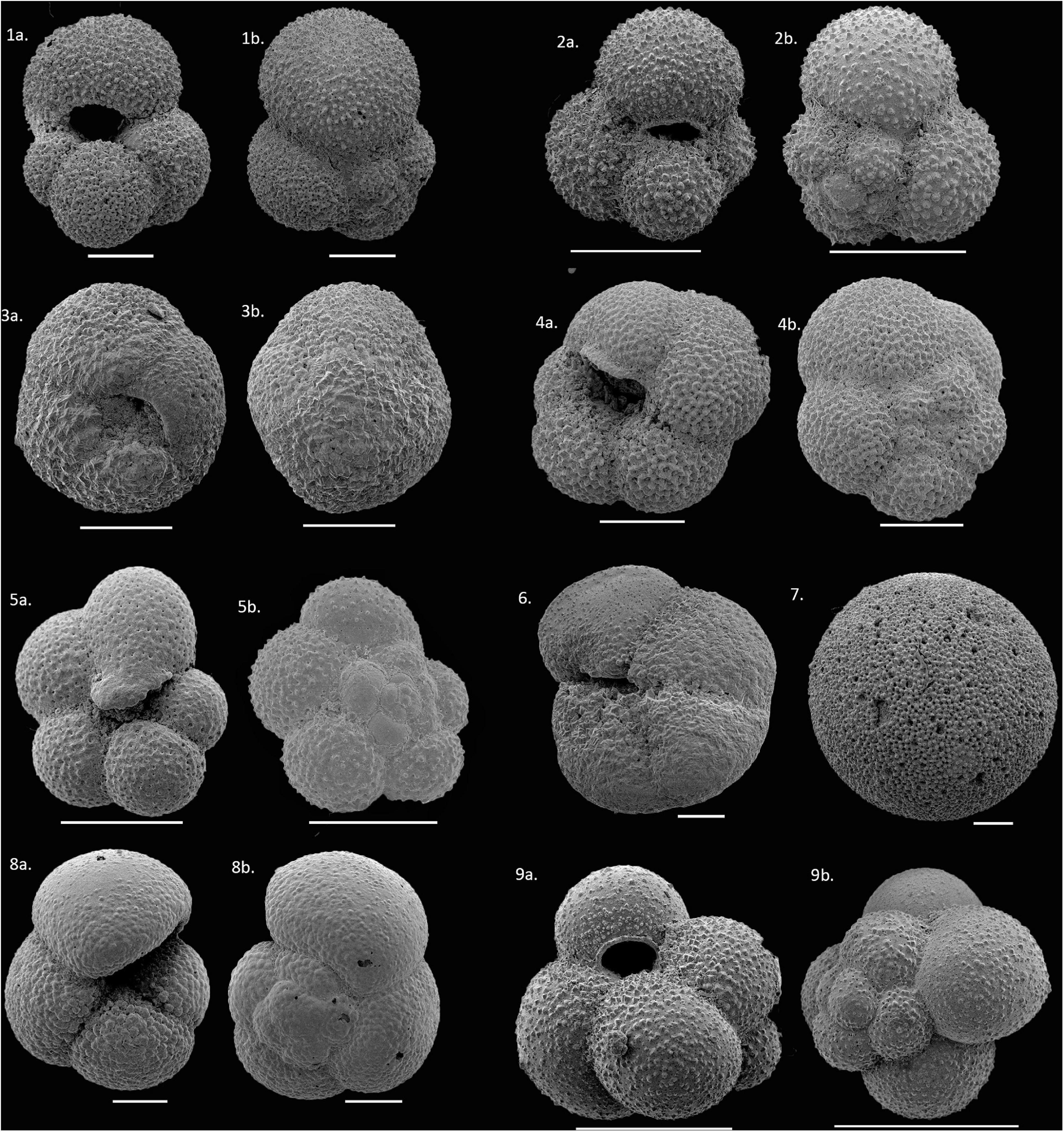
Figure 3. Scanning Electron Micrograph (SEM) images of planktic foraminifera species observed in the present study. (1a,b) Globigerina bulloides (G. bulloides), (2a,b) Globigerinita glutinata (G. glutinata), (3a,b) Neogloboquadrina pachyderma (N. pachyderma), (4a,b) Neogloboquadrina incompta (N. incompta), (5a,b) Turborotalita quinqueloba (T. quinqueloba), (6) Globorotalia scitula (G. scitula), (7) Orbulina sp. (8a,b) Globorotalia inflata (G. inflata), (9a,b) Globigerinita uvula (G. uvula); Scale bars 100 μm.
The former five species were consistently present along the transect except at a few stations; whereas, G. inflata was present only in the eastern part of the transect (absent toward the west of Reykjanes Ridge). However, in the western part of the transect, in the Labrador Sea and Irminger Sea, the sampled stations were dominated by N. pachyderma. Moreover, it is the only species found in the two sampling sites of the Labrador Sea, constituting 100% of the assemblage (Figure 4A).
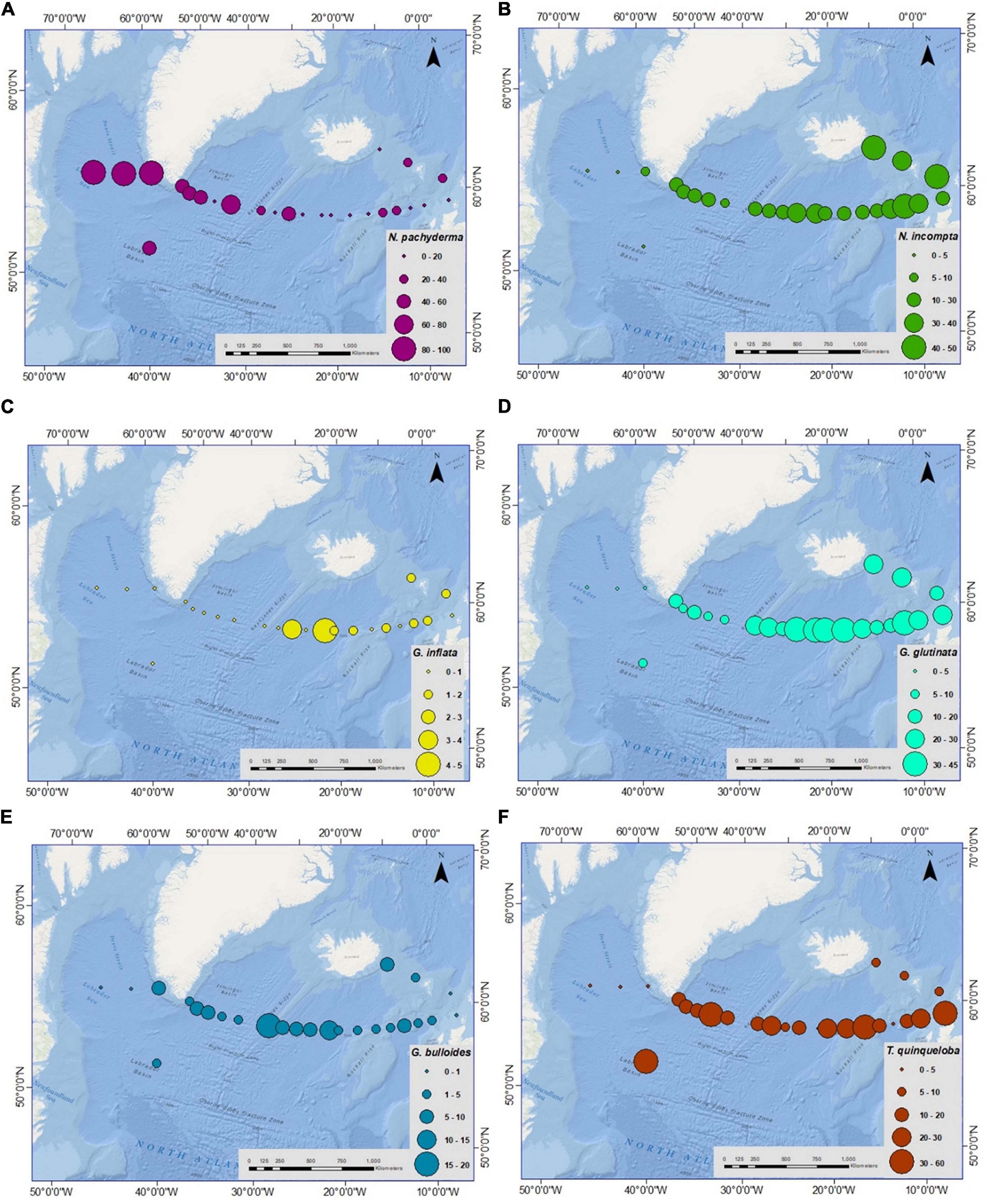
Figure 4. Spatial variation in relative abundance (%) of planktic foraminiferal species (A) Neogloboquadrina pachyderma (N. pachyderma), (B) Neogloboquadrina incompta (N. incompta), (C) Globorotalia inflata (G. inflata), (D) Globigerinita glutinata (G. glutinata), (E) Globigerina bulloides (G. bulloides), (F) Turborotalita quinqueloba (T. quinqueloba) from Labrador Sea to Faroe-Shetland channel. Please note the percentage range for each species is different.
Variations in Relative Abundances of Planktic Foraminifera Species
The relative abundance of planktic foraminifera species varied considerably from west to east along the transect (Figure 4). Neogloboquadrina pachyderma was the most abundant species in the western part of the transect consisting of 100% of the assemblage in sediment fraction of >100 μm in the Labrador Sea (Figure 4A). This species was more abundant in the Labrador and Irminger Seas, and its concentration decreased toward the eastern part of the transect. The species was absent from stations 15, 20, 21, and 22 on the eastern side of the Iceland Basin. The average relative abundance of N. incompta was 22% in the transect with the highest value of 43% (station 20) near the Scottish shelf and nil in the Labrador Sea (stations 2 and 3) (Supplementary Table 3 and Figure 4B). Higher relative abundance was seen in the IFSC (average 41%) followed by the Iceland basin-Rockall Plateau (average 27%) and the lowest in the Irminger Sea (average 12%) and the Labrador Sea (average 2%). Globorotalia inflata was concentrated only in the eastern transect with the highest relative abundance of 5% at stations 25 and 14 in the Iceland-Faroe Ridge and the Iceland Basin (Figure 4C). The average relative abundance of G. inflata was 2% in the eastern part of the transect. Likewise, the average G. glutinata percentage along the transect was 19%, with the highest abundance of 45% (station 14) in the Iceland Basin, although absent in the Labrador Sea (Supplementary Table 3 and Figure 4D). Globigerina bulloides percentage was low in this area, with an average value of 4%. Globigerina bulloides showed the maximum abundance of 20% at station 10 in the Reykjanes Ridge area. While, the abundance of G. bulloides was nil at stations 2, 3, 22, and 23 in the Labrador Sea and near the Scottish shelf (Figure 4E). The relative abundance of T. quinqueloba increased (>25%) in specific sites in the Irminger Sea, Iceland Basin, and off Scotland, with relatively lower percentages in other stations ranging between 0 and 21% (Figure 4F). Globigerinita uvula was present in the samples with very low relative abundance, highest as 5% near the Scottish shelf (station 21). The occurrence of G. uvula was patchy in the transect. Globorotalia scitula and Orbulina sp. were very rare. G. scitula was found only in the eastern Iceland basin (stations 14, 15, and 16) with <1% and near the east coast of Greenland (station 4) with 4%. Orbulina sp. was found only at two stations in the eastern Iceland basin and Iceland-Faroe Ridge (stations 14 and 23).
Ratio Neogloboquadrina pachyderma/Neogloboquadrina incompta Along E–W Transect
The distribution of the species N. pachyderma and N. incompta is significantly influenced by the temperature and salinity of the region. Also, a change in the relative abundance of N. pachyderma and N. incompta is linked to specific summer (Sea Surface Temperature) SST values of the water mass (Žarić et al., 2005). Hence, to document the variation in water mass properties (temperature and salinity) from west to east along the sampled transect, the ratio of N. pachyderma and N. incompta was used. Along the transect, the relative abundance of N. pachyderma was observed to increase significantly from the eastern to the western part. However, N. incompta exhibited an opposite trend with the N. pachyderma abundance from east to west (Figure 5A). To better interpret the variation of these two species with respect to water mass (temperature and salinity) along the transect, we analyzed the ratio of the two species (Figure 5). The ratio of N. pachyderma/N. incompta decreased toward the eastern side of the transect. Also, a higher ratio of >2 was observed toward the western part of the transect, where lower temperature and salinity values were attained (Figure 5B).
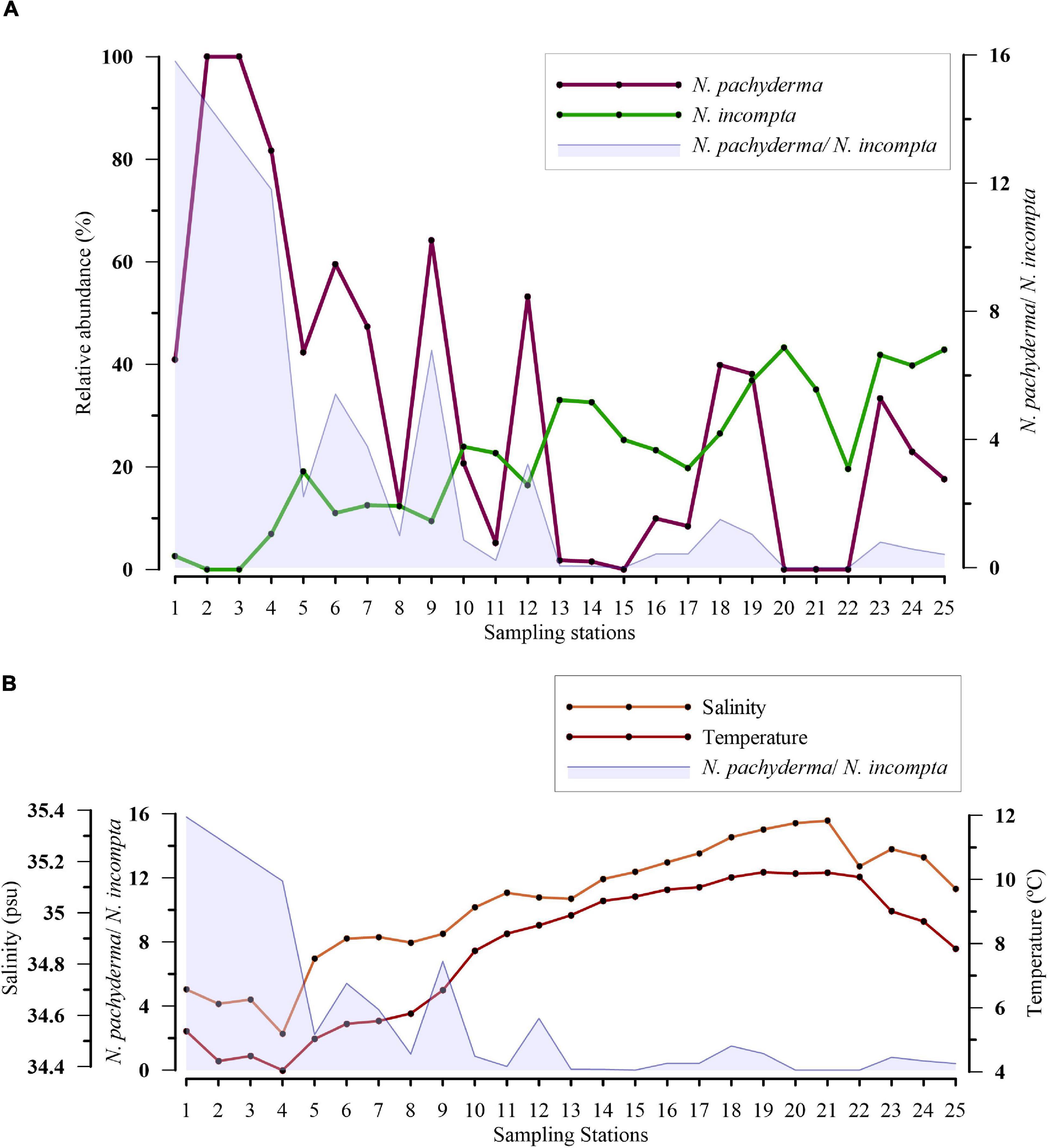
Figure 5. (A) Variation in relative abundance (%) of Neogloboquadrina pachyderma (N. pachyderma) and Neogloboquadrina incompta (N. incompta) along the transect and variation in the ratio of N. pachyderma/N. incompta against the sampled stations is shown in blue; (B) Plot showing a relation between the ratio of N. pachyderma and N. incompta abundance with sea surface temperature and salinity.
Principal Component Analysis
To assess the ecological preferences of the planktic foraminifera and their relation to the environmental parameters in the SPNA ocean, PCA was performed. The first two PCA factors explain 86.98% of the total variance. The first factor explains 60.71%, and the second factor explains 26.27% (Figure 6). Factor 1 has positive loadings on temperature and salinity computed for the first 100 m (average) of the water column, whereas factor 2 has a positive loading with superficial Chl-a. In the PCA analysis, both temperature and salinity vary in the same trend. However, both the parameters have an individual influence on the abundance and distribution of planktic foraminiferal species in the studied area. From the correlation table (Table 1), G. glutinata, N. incompta, and G. inflata exhibit positive values regarding temperature (0–100 m) with r values 0.75, 0.81, and 0.48, respectively, and salinity (0–100 m) with r values 0.67, 0.86, and 0.41, respectively. Neogloboquadrina pachyderma shows a negative correlation with temperature (r = −0.76) and salinity (r = −0.73). Neogloboquadrina incompta shows a negative correlation with water depth. Other species have no significant correlation with any of the environmental parameters.
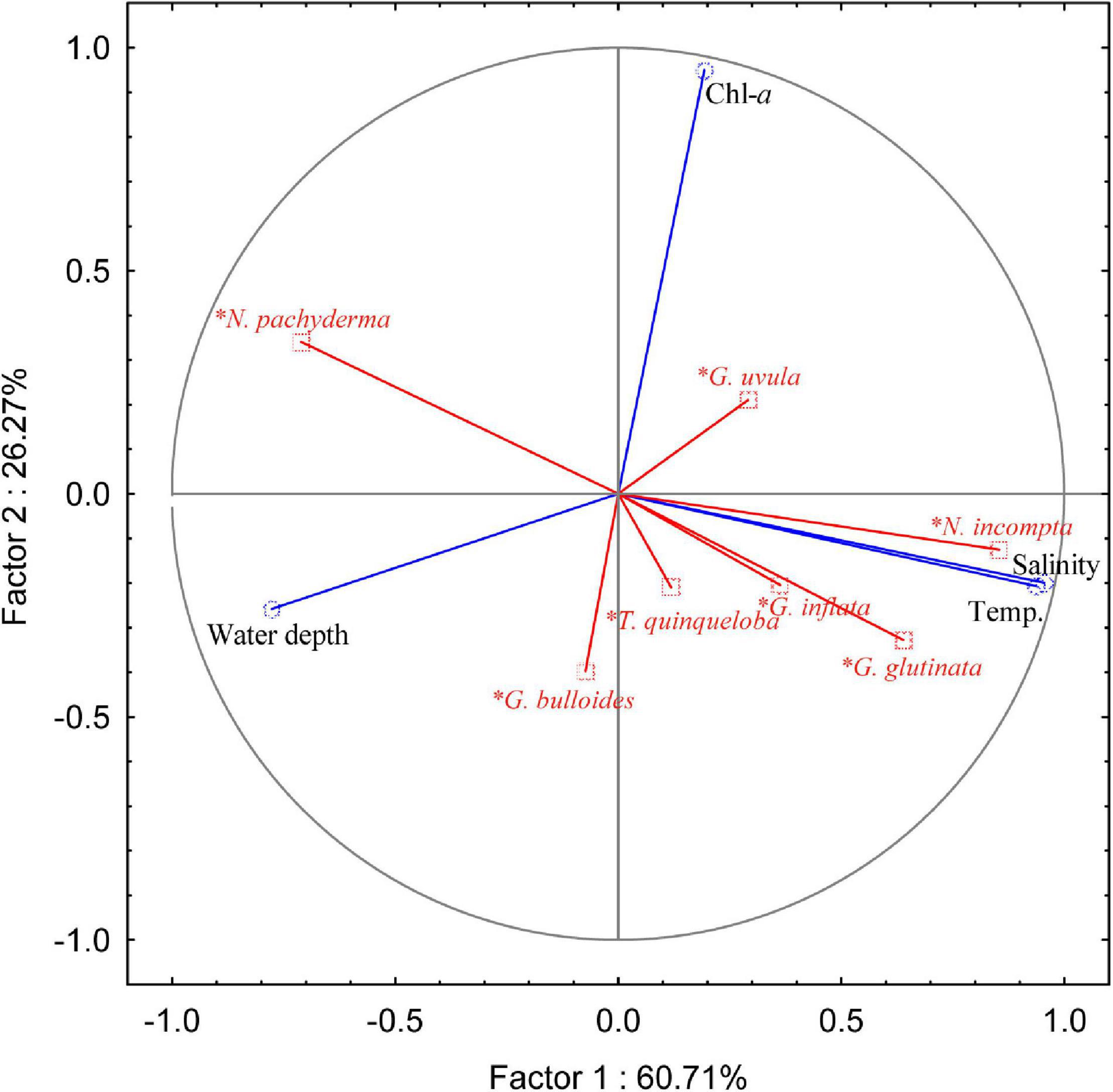
Figure 6. Principal component analysis (PCA) plot of the relative abundance of the different species (N. pachyderma: Neogloboquadrina pachyderma; N. incompta: Neogloboquadrina incompta; G. inflata: Globorotalia inflata; G. glutinata: Globigerinita glutinata; G. bulloides: Globigerina bulloides; T. quinqueloba: Turborotalita quinqueloba; G. uvula: Globigerinita uvula) with different environmental parameters [average temperature (Temp., °C) and salinity (PSU) of the first 100 m of the water column, Chlorophyll-a concentration (Chl-a, mg m– 3) and water depth (m)].

Table 1. Correlation factors for the relative abundance of planktic foraminifera (Neogloboquadrina pachyderma, Neogloboquadrina incompta, Globigerina bulloides, Turborotalita quinqueloba, Globorotalia inflata, Globigerinita glutinata, and Globigerinita uvula) with different environmental parameters [average temperature (Temp., °C) and salinity (PSU) of the first 100 m of the water column, Chlorophyll-a (Chl-a, mg m–3) and water depth (m)].
Cluster Analysis
A hierarchical cluster analysis was performed on the planktic foraminifera dataset to delineate different groups of stations having specific assemblages using the Bray Curtis similarity index (Figure 7). Based on the similarity index, stations along the transect can be divided into three distinct cluster groups I, II, and III. The species percentage discussed in this section refers to the individual species contribution (%) to different clusters, further analyzed from SIMPER analysis.
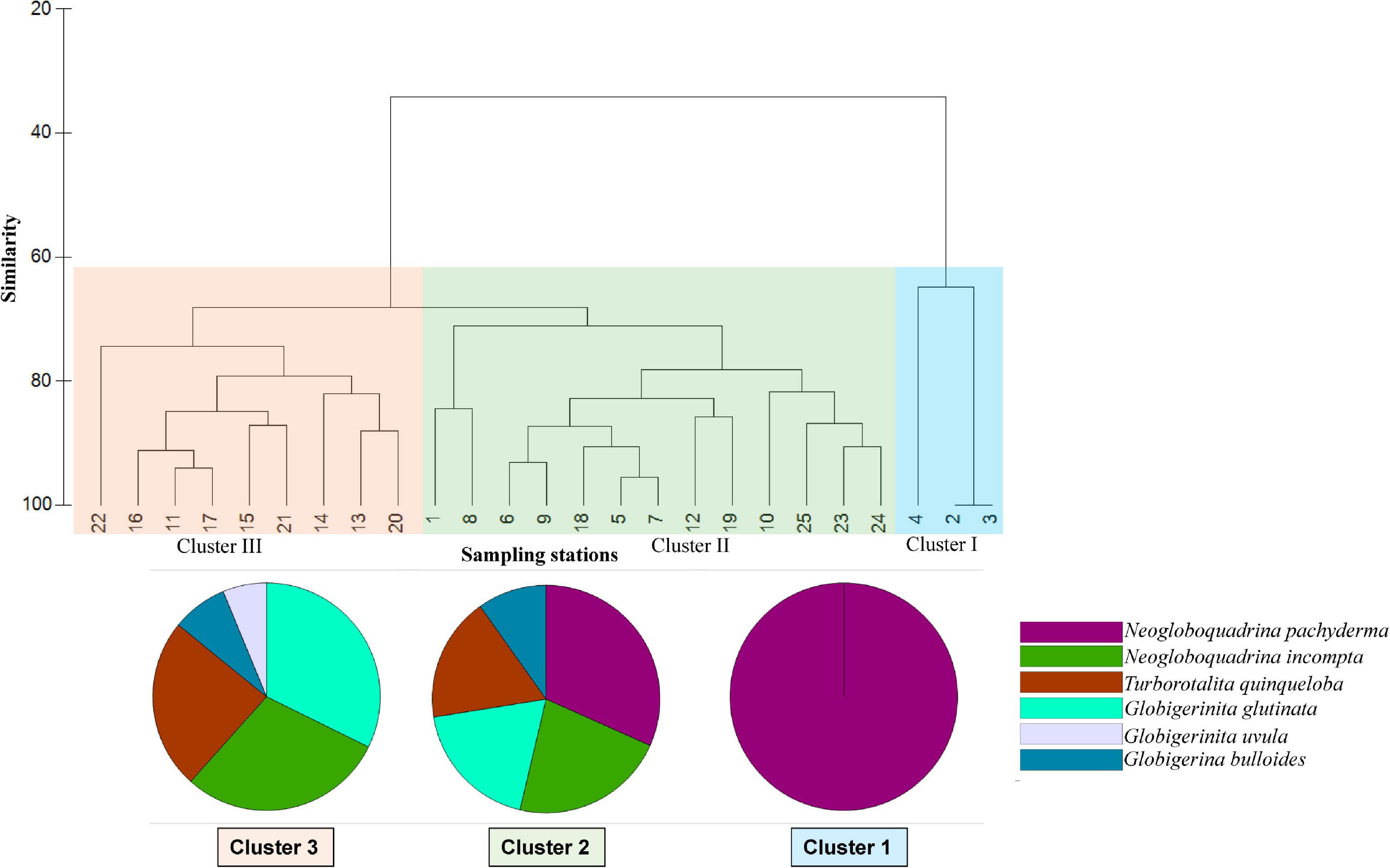
Figure 7. Hierarchical cluster analysis of stations based on foraminiferal assemblages (Bray Curtis similarity). The pie charts show the contribution of different species to different clusters (by SIMPER analysis).
Cluster Group I
Cluster group I includes three stations, 2, 3, and 4, from the central and northeastern Labrador Sea (Figure 7). The planktic foraminifera assemblages associated with this group are dominated by N. pachyderma. Other planktic foraminifera species N. incompta, G. bulloides, T. quinqueloba, and G. scitula, though present in station 4, do not contribute to the similarity percentage of this cluster group. Only N. pachyderma contributes 100% to Cluster I. The average similarity between these samples was 76.63% in the Bray Curtis similarity analysis.
Cluster Group II
Cluster group II includes 13 stations from two separate areas within the transect: (1) western half of transect from Reykjanes Ridge to Irminger Sea and central SPG (stations 1, 5, 6, 7, 8, 9, 10, and 12), (2) eastern/northeastern end of transect including the stations from northernmost Rockall Plateau and Iceland-Faroe-Shetland sill (stations 18, 19, 23, 24, and 25) (Figure 7). Compared to monospecific planktic foraminifera associations of Cluster group I, assemblages from Cluster group II are mixed, consisting of almost all the species found in the study area. The most abundant species are N. pachyderma (30%), N. incompta (21%), G. glutinata (18%), T. quinqueloba (16%), and G. bulloides (9%). The average similarity of the Cluster group II was 78.81% in the samples.
Cluster Group III
Cluster III groups nine stations (11, 13, 14, 15, 16, 17, 20, 21, and 22) on the eastern side of the transect from the Reykjanes Ridge to Rockall Plateau and off Scotland (Figure 7). However, few stations from the eastern part of the transect (stations 12, 18, and 19) are included in Cluster group II. The planktic foraminiferal assemblages in this region consist mainly of G. glutinata (30%), N. incompta (27%), T. quinqueloba (22%), G. bulloides (7%), and G. uvula (6%). The average similarity between the samples in this group is 80.71%.
Discussion
Distribution and Abundance of Planktic Foraminifera in the Subpolar North Atlantic
Planktic foraminifera distribution is majorly governed by the contrasting water mass characteristics in the SPNA ocean. Total planktic foraminifera co-varied with the calcium carbonate content in the surface sediments along the sampled transect from the Labrador Sea to Faroe-Shetland Ridge (Figure 2A). A positive correlation r = 0.72 between the two suggests planktic foraminifera as the main contributor to the biogenic carbonate in the SPNA. A similar observation representing planktic foraminifera as a key component of bottom ocean carbonate was also reported by Honjo and Manganini (1993); Ziveri et al. (1995), and Retailleau et al. (2012). The distribution of planktic foraminifera is primarily based on sea-surface temperature and salinity of the region (Thunell, 1978). However, particularly in the North Atlantic Ocean, SST plays a dominant role (Stangeew, 2001). Along the transect in this study, the lowest planktic foraminifera abundances in the Labrador Sea can be attributed to persistent low annual average (0–100 m) temperature of 3–4°C and salinity of 34.86 PSU. Such low temperature causes a low saturation state for CaCO3 and accounts for a low pH in the Labrador Sea (Azetsu-Scott et al., 2010). These characteristic physical parameters (low temperature, salinity, and pH) of the Labrador Sea are due to the influx of cold and fresh polar water by EGC, fresh Arctic water coming through the Canadian Arctic Archipelago (Melling et al., 2008) and the modified Atlantic water (relatively cooler than North Atlantic Water) coming through IC (Pollard et al., 2004). Furthermore, some parts of the Labrador Sea are influenced by relatively much lower pH water mass coming through the Canadian Arctic Archipelago (Azetsu-Scott et al., 2010). The low pH acts as an additional factor causing low planktic foraminifera abundances as it is detrimental to the calcitic foraminifera shells and limits planktic foraminiferal abundance. The decreasing rate of deep water formation, linked to the current climate change scenario (Rahmstorf et al., 2015; Thornalley et al., 2018), increases the residence time of the cold deep water at the formation site. This also might cause the dissolution of the highly susceptible foraminiferal shells resulting in low planktic foraminifera abundance. Another area with low planktic foraminifera abundances is the shallow water region of Faroe-Shetland Ridge. Planktic foraminifera is more abundant in the open ocean than in neritic zones with shallow water depths (Schmuker, 2000; Retailleau et al., 2009). The water mass near Faroe-Shetland is entrained by a tongue of cold and fresh nutrient-poor Arctic water brought by East Iceland Current (EIC), which can additionally cause a decline in the productivity in the region.
Planktic foraminifera was most abundant in the middle of the transect covering the eastern Iceland Basin to the central Irminger Sea, which is influenced by the modified nutrient-rich Atlantic water. The presence of Subpolar Front (SPF) in this area near Reykjanes Ridge aids in enhanced planktic foraminiferal abundances in the SPNA as frontal regions are characterized by the uplifting of nutrient-rich waters, which allows a greater abundance of phytoplankton and leads to higher primary productivity (Lutjeharms et al., 1985; Kimura et al., 2000). Further, eddy activity in the Irminger Sea and Iceland Basin is responsible for strong mixing at the surface, enriching the surface water with nutrients (Knutsen et al., 2005) which increases primary productivity and hence, the planktic foraminifera abundances in the region.
The planktic foraminiferal number is comparable with the sediment trap and plankton tow datasets previously reported in the SPNA ocean (Schiebel and Hemleben, 2000; Schiebel, 2002). The planktic foraminiferal shells vary from 1.20 × 103 to 1.80 × 105g–1 (present study) with varying sampling depth. Samples from the open ocean at a depth of 2,000 m exhibit comparable numbers with Lundgreen (1996), which represents 1.60 × 105 planktic foraminifera tests year–1 [extrapolated tests day–1 in September from 47°50’N, >100 μm (Table 3 in Schiebel, 2002)]. However, a decrease in flux with depth is associated due to the biologically mediated dissolution of planktic foraminifera through the water column while settling (Schiebel and Hemleben, 2000). Further, the relative abundances found in the multi net samples from the BIOTRANS area and at 57°N, 20–22°W of Schiebel and Hemleben (2000) is comparable to the planktic foraminifera species composition in our study. Hence, the species assemblage in the surface sediment in this area represents the upper water mass assemblages. Pados and Spielhagen (2014) also reported a similar observation from Fram Strait. Moreover, the mean lateral transportation for the smaller species T. quinqueloba and N. pachyderma is 50–100 and 25–50 km in the Fram Strait, respectively (Gyldenfeldt et al., 2000). From this, we can suggest no significant effect of lateral transportation on planktic foraminifera assemblages in the SPNA ocean.
Species Ecological Preferences
The polar to subpolar species N. pachyderma was found to be a single dominant species in the Labrador Sea in the present study. A significant increasing trend was observed in the relative abundance of N. pachyderma toward the western part of the transect in the study area (Figure 4A). Our data show a strong negative correlation of N. pachyderma relative abundance with both temperature (r = −0.76) and salinity (r = −0.73), marking its dominance in the cold fresh Polar water of Labrador and Irminger Seas (Table 1). Previous studies (Kohfeld et al., 1996; Greco et al., 2019) exhibited a number of environmental factors controlling the abundance and distribution of N. pachyderma. This species is observed abundantly in low temperature and low saline regions (Bé and Tolderlund, 1971), which is also evident in our study. Moreover, abundances of N. pachyderma of ≥95 and <50% correspond to a summer temperature of <4 and ≥9°C, respectively (Bé and Tolderlund, 1971). The occurrence of N. pachyderma has been reported to vary as a function of salinity, presence/absence of sea ice, and deep chlorophyll maximum (Stangeew, 2001; Jonkers et al., 2010; Greco et al., 2019). Usually, N. pachyderma abundances show a two-way pulse in its flux in North Atlantic; one in summer at maximum stratification and another in spring (Tolderlund and Bé, 1971; Jonkers et al., 2010). Some studies reported that food availability (Chl-a concentration, as a proxy of phytoplankton biomass) also affects the distribution of N. pachyderma like other planktic foraminifera (Fairbanks and Wiebe, 1980; Kohfeld et al., 1996; Schiebel and Hemleben, 2005). However, our results for SPNA suggest a statistically validated relationship of N. pachyderma abundances with water temperature and salinity but weak dependence on Chl-a concentrations (r = 0.17 in Table 1). Although, it should be noted that the weak correlation of N. pachyderma with Chl-a may be a result of considering only the most superficial layer in this statistical analysis.
In the present study, T. quinqueloba shows a patchy distribution. It is observed maximum in the Irminger Sea and the eastern Iceland Basin in our study. Its occurrence has no significant statistical correlation with temperature and salinity along the sampled transect. Although T. quinqueloba has been previously reported to vary with primary productivity (Volkmann, 2000), its abundance did not correlate with Chl-a concentration in the present study. Meilland et al. (2020) have also documented the same in the Barents Sea opening. This could be due to the specific habitat and food preference of the species, which cannot be represented by Chl-a data alone. T. quinqueloba has been described to be associated with water mass fronts (Hemleben et al., 1989; Husum and Hald, 2012) and are most abundant at regions of mixing of different water masses and enrichment in the nutrients (Johannessen et al., 1994; Husum and Hald, 2012).
Along the sampled transect, N. incompta follows the opposite trend compared to N. pachyderma exhibiting decreasing abundance in the subpolar waters of the Irminger and the Labrador Seas. Its abundance has strong positive correlation with temperature (r = 0.81) and salinity (r = 0.86; Table 1). Also, the negative correlation of N. incompta percentages with water depth (bathymetry) (r = −0.61) supports its higher abundance in shallow waters of the Iceland-Faroe-Shetland Channel. This observation is coherent with other studies wherein N. incompta was abundant in <100 m water depth in eastern North Atlantic (Schiebel et al., 2001; Husum and Hald, 2012). It prefers shallow stratified warm water (>8°C) (Ortiz et al., 1995; Schiebel et al., 2001). Neogloboquadrina incompta is also related to high nutrient and Chl-a concentration (Ortiz et al., 1995; Kuroyanagi and Kawahata, 2004; Kretschmer et al., 2018). However, in the present study, no significant correlation of N. incompta abundances with Chl-a concentration (r = 0.05) was found. This might be due to the changing food habits of the species with respect to the availability of food in this region. Also, we have taken the superficial Chl-a data for the analysis, which could cause a no-correlation with species abundance as N. incompta thrives in 50–150 m depth of the water column.
In the present study, G. glutinata was most abundant in the central part of the Iceland Basin and decreased toward the western and eastern sides of the sampled transect. Globigerinita glutinata is a cosmopolitan species with no specific environmental limits (Schiebel et al., 2017). This species has been shown to dominate planktic foraminifera assemblages in the high latitude areas only recently (Schiebel et al., 2017; Spooner et al., 2020). In the subpolar ocean, the species abundance can increase in summer with shallow thermocline depth depending upon food availability (Stangeew, 2001). Some authors have suggested, it increases with the onset of spring bloom (Ottens, 1992), and few link it with a change in the possible food habitat (Meilland et al., 2020; Spooner et al., 2020). We found that the species occurrence has a positive correlation with temperature (r = 0.75) and salinity (r = 0.67) along the transect showing the species’ affinity toward warmer and more saline water mass, but no correlation with Chl-a. Globigerinita glutinata was also reported to have a strong relationship with seasonality and food availability, especially associated with diatoms (Schiebel and Hemleben, 2000). Our data in this region shows the distribution of G. glutinata is related to the temperature and salinity variation rather than Chl-a concentration.
In the present study, temperate to subpolar species G. bulloides relative abundance shows a weak negative correlation (r = −0.42) with Chl-a concentration and also does not depend on temperature and salinity (Figure 6). Here, G. bulloides is maximum over Reykjanes Ridge and central Iceland Basin. Generally, this species is most abundant in highly productive subpolar and tropical regions and is specifically marked as an upwelling indicator species in the tropics (Bé and Tolderlund, 1971; Schiebel et al., 2001; Salgueiro et al., 2010). However, a negative correlation possibly can be due to a change in the nature of food habitat in the area. Meilland et al. (2020) suggested Chl-a may not always represent a good indicator for foraminiferal distribution, showing no link between Chl-a and the productivity indicator species T. quinqueloba and G. uvula in the Barents Sea. Thus, G. bulloides can also be summed as a ubiquitous subpolar species and does not follow any specific trend in the SPNA ocean.
Globigerinita uvula is a temperate to polar species, constituting 0.50–2% of the assemblages (Schiebel and Hemleben, 2017), which is consistent with our data. Recently, increased abundances of this species in surface water assemblages from subpolar to polar regions (Stangeew, 2001; Retailleau et al., 2011; Meilland et al., 2020) have been noticed, but it was not reflected in the surface sediment assemblages (Meilland et al., 2020) as also seen in our data. As the plankton tow study provides information, particularly during the sampling period, G. uvula abundance in the water column at that particular period can increase depending on the favorable conditions and can be different from surface sediment assemblage. The small-sized-less resistant species may have low preservation potential while settling in sediment, causing an enhancement of the same in the water column and not in sediment. Moreover, the lower settling velocity of this species gives rise to a higher concentration of its tests in the water column. Globigerinita uvula mainly concentrated in <100 μm fraction (Schiebel et al., 2017), might also be a reason for the low abundance of the same in >100 μm in our study. There is no correlation of G. uvula percentages in our data with temperature or salinity. The occurrence of G. uvula presumably co-varies with primary productivity (Volkmann, 2000; Schiebel and Hemleben, 2017) and generally have a seasonal production in spring and early summer (Schiebel et al., 1995). However, it exhibits an insignificant correlation with Chl-a concentrations along the transect. In a recent finding, Meilland et al. (2020) have also shown no significant correlation between Chl-a content and G. uvula occurrence as they showed a more dependency on food composition rather than concentration.
Globorotalia inflata has a low abundance and is present only on the eastern side of the transect. Generally, its occurrence ranges from subpolar to subtropical waters (Schiebel et al., 2017). In the subpolar ocean, its intrusion represents the influence of warmer water coming through the North Atlantic Current (NAC). It thrives at the base of the seasonal thermocline (100–200 m) (Ganssen and Kroon, 2000; Cléroux et al., 2007). G. inflata abundance depends on the surface, and subsurface nutrient enrichment (Schiebel et al., 2017), i.e., eddies and frontal mixing supports its distribution. However, we found only weak positive correlation of G. inflata abundances with temperature (r = 0.47) and salinity (r = 0.41) but no relation to Chl-a concentrations (r = −0.05).
Abundances of the subtropical species G. scitula and Orbulina sp. are rare and very low in SPNA. However, G. scitula and Orbulina sp. have been described as subtropical species and are associated with NAC (Ottens, 1991). Hence, their presence can indicate the entrainment of warm NAC water in the study area. Moreover, the absence of correlation of planktic foraminifera with Chl-a in the study area is probably due to the fact that the chlorophyll data represent only the superficial layer and not the 0–100 m.
Faunal Assemblages
Along the sampled transect in the SPNA Ocean, the stations are grouped into three distinct faunal groups based on hierarchical cluster analysis.
Labrador Sea - Polar Group (Cluster Group I)
The Labrador Sea has the coldest water mass along the transect. It is influenced by the cold surface currents EGC, WGC, LC, and relatively warmer surface current IC. Also, it receives fresh Arctic water through the Canadian Arctic Archipelago. Cluster group I, associated with the Labrador Sea, comprises a single species N. pachyderma (100%) (Figure 7). Neogloboquadrina pachyderma is dominant in subpolar and polar conditions (Bé and Tolderlund, 1971; Volkmann, 2000). A decrease in species diversity and richness often occurs in regions of high environmental stress (Keller and Abramovich, 2009) which is the case in the Labrador Sea. The elevated abundance of N. pachyderma in the region can also be explained by the good preservation of these thick-shelled species as that is more resistant to dissolution than T. quinqueloba and G. uvula (Berger, 1973; Boltovskoy and Wright, 2013).
Study on modern-day planktic foraminifera assemblages in the Labrador Sea is rare. A previous study by Stehman (1972) documented two species, N. pachyderma and G. bulloides, in >200 μm mesh size from the Labrador Sea. Besides, the dominance of single species in this study can be due to an underestimation of the foraminiferal species with smaller size fractions. The size range considered in the present study is >100 μm with relative abundances of N. pachyderma 100%. Conversely, Stangeew (2001) had reported high abundances of small-sized (>63 μm fraction) T. quinqueloba (46.80%), G. uvula (3.50%), along with G. glutinata (20.80%), N. pachyderma (19.60%), N. incompta (2.60%), G. bulloides (6.40%),G. scitula (<1%), and G. inflata (<1%) in the convection region of the Labrador Sea in 0–200 m water column. We compared our surface sediment data of station 3 with the plankton tow results of Stangeew (2001). To ascertain whether the species abundances are size-dependent, we examined the 63–100 μm size fraction in the samples of the Labrador Sea. In this fraction, compared to >100 μm fraction, the smaller-sized species G. uvula were more dominant with 37–80% abundance (Figure 8). The dominance of N. pachyderma in >100 μm fraction and of G. uvula in 63–100 μm fraction infers that size plays a significant role in the analysis of planktic foraminifera assemblages, which was also observed in previous studies (Carstens et al., 1997; Husum and Hald, 2012).
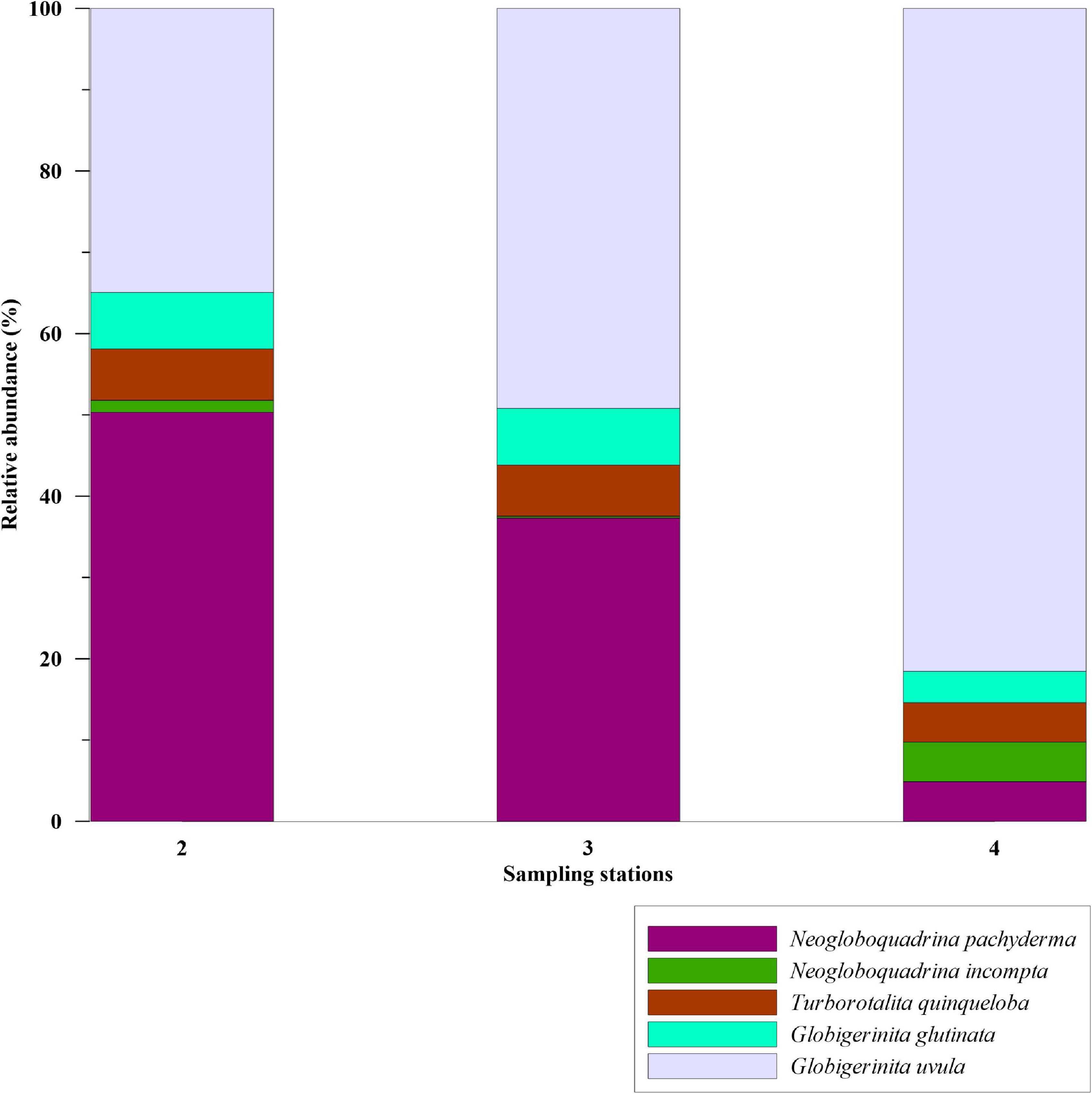
Figure 8. Relative abundance (%) of planktic foraminiferal species in 63–100 μm size fraction from the stations of Labrador Sea, shown in bar plots.
In polar regions, Bé and Tolderlund (1971) reported N. pachyderma to be the most abundant in >200 μm fraction. Whereas, Carstens et al. (1997) and Volkmann (2000) found the species abundance to be reduced to 60% in the fraction of >63 μm and 70% in the fraction of >125 μm, respectively. Also, test sizes of T. quinqueloba in Nordic Seas have been found to differ in the past under varying climatic conditions with shell sizes becoming larger under the Atlantic water influence (Bauch, 1994). As both the size fractions in the Labrador Sea samples from our study are dominant in the cold and fresh water species N. pachyderma and G. uvula, we suggest a stronger influence of fresh melt water and EGC influenced water than the warmer IC water in the Labrador Sea. This can be evident as the recent fresh water/melt water flux increased in the eastern Labrador Sea (Yang et al., 2016; Zhang et al., 2021).
Irminger Sea and Iceland-Faroe-Shetland Subpolar Group (Cluster Group II)
This group includes two areas: (1) the western side of the transect within central and northern subpolar gyre (east of Greenland, Irminger Sea, and Reykjanes Ridge), (2) the eastern side of transect in the Iceland-Faroe-Shetland Channel (IFSC), and on the northernmost Rockall Plateau between the two pathways of NAC. Cluster group II is comprised of mixed assemblages of temperate to subpolar species N. incompta, G. glutinata, and T. quinqueloba, and cold-water species N. pachyderma. On the western part of the transect, the cold fresh water of EGC comes in contact with relatively warm and more saline water of IC (Dickson et al., 2007). Thus, a mixed assemblage is expected in the region. The dominance of N. pachyderma explains this, followed by a significant abundance of T. quinqueloba and N. incompta. While, in the eastern side of the transect, assemblages consist primarily of N. incompta together with T. quinqueloba and N. pachyderma, mainly in the IFSC region. In the IFSC, the Arctic water (lobe of cold water north of Iceland coming in the southern Norwegian Sea) interacts with the warm Atlantic water coming with NAC. Modified North Atlantic Water (MNAW), a cooler water mass than Eastern North Atlantic Water (ENAW), is found in the upper water masses at IFSC and in some parts of the Rockall Plateau (Read, 2000). A mixed assemblage of both cold and warm species represents a mixing of cold and warm water. Planktic foraminifera assemblages of Group II reflect the greater influence of the polar water in the western SPNA.
Central North Atlantic (Iceland Basin, Northwestern Rockall Plateau, Off Scotland) Temperate Group (Cluster Group III)
The region toward the east of the transect, Iceland basin, Rockall Plateau, receives warm water from NAC (Pollard et al., 2004). This group is associated with predominantly temperate and subpolar species assemblages in the central North Atlantic, which exhibit the warmer-water affinity. The most abundant species of this group are G. glutinata, N. incompta, and T. quinqueloba, while G. bulloides and G. uvula are less abundant. In the central Iceland basin, more of N. incompta and G. glutinata represents a warmer water influence. Also, the presence of G. inflata and Orbulina sp. in this group shows the influence of warm subtropical water (Ottens, 1992). Globigerinita uvula’s contribution to the assemblage (6%) shows the presence of fresher melt water at the surface in summer.
Turborotalita quinqueloba percentages are higher at the sites near the NAC pathways in Iceland Basin, near Scotland, and the Reykjanes Ridge. NAC, in this region, acts as a frontal zone, separating the cold fresh subpolar water mass and warm saline subtropical water mass (Read, 2000; Daniault et al., 2016). Turborotalita quinqueloba is a front indicator species; hence, its higher abundance at the eastern Iceland basin and Reykjanes Ridge reflects the proximity of the frontal zone in the studied transect.
Neogloboquadrina pachyderma Versus Neogloboquadrina incompta Ratio as an Indicator for Change in Water Mass Property
Neogloboquadrina pachyderma is a subpolar to polar species with an increase in abundance poleward (Bé and Tolderlund, 1971). While N. incompta, reported earlier as a dextral variety of N. pachyderma, is preferably found in relatively warmer and more saline waters. In the present study, N. incompta represented its association with temperate/subtropical characteristics of NAC in the region, as the PCA plot demonstrated (Figure 6). The abundance of both species depends on physicochemical factors, such as the temperature and salinity of the water mass, presence/absence of sea ice, stratification, and food availability (Jonkers et al., 2010; Greco et al., 2019; Meilland et al., 2020). The highest abundances of N. pachyderma match with an optimum temperature range of −0.50 to 17°C, whereas maximum percentages of N. incompta occur at 8.50–21.40°C (Žarić et al., 2005, based on sediment traps study; Hilbrecht, 1996, based on surface sediments study). The critical temperature for the shift from the dominance of N. pachyderma to N. incompta is at ≥9°C (Žarić et al., 2005). In our study, the highest abundances of N. pachyderma are concentrated in the Irminger and Labrador Seas at an average temperature of 5.54°C and average salinity of 34.96 PSU. Contrary to this, N. incompta is most abundant in the eastern side of the North Atlantic, with maximum abundances at an average temperature of 6.82°C and salinity of 35.05 PSU. The turnover in prevailing abundances of N. pachyderma/N. incompta takes place near Reykjanes Ridge (Figure 5). We suggest a change in water mass properties from the change in assemblages at the western side of Reykjanes Ridge, previously documented by authors from earlier studies (McCartney and Talley, 1982; Read, 2000). This can be illustrated by the ratio between the abundances of N. pachyderma and N. incompta along transect plotted against the temperature and salinity (average of upper 100 m water column) (Figure 5B). The ratio of N. pachyderma and N. incompta was found to be >1 in the western side with the highest values at the temperature of <4.60°C and salinity of <34.80–35 PSU in the present study. Our results on differences in the abundances of N. pachyderma and N. incompta on the transect across SPNA are in agreement with previous data on this species ratio as an indicator of the changes in water mass distribution in Nordic Seas and SPNA (Eynaud, 2011). Updating data on the N. pachyderma/N. incompta ratio improves the use of this tool for paleoceanographic studies, as demonstrated by Eynaud et al. (2009). This is evident with the hydrographic change across the Reykjanes Ridge in the upper water masses.
A Shift in Planktic Foraminiferal Assemblages in the North Atlantic
The recent trends in warming and its associated processes have led to the change in ecology and distribution of planktic foraminifera in the North Atlantic (Jonkers et al., 2019; Meilland et al., 2020). Schiebel et al. (2017) and Meilland et al. (2020) have reported a marked change in planktic foraminifera assemblages and their diversity, with an influx of temperate species increasing their dominance in the region.
We have compared our planktic foraminifera assemblage data with previously published data presenting samples collected in the North Atlantic from Matul et al. (2018), which also includes Pflaumann et al. (2003) dataset, to see if there is any change in planktic foraminiferal assemblages. Their dataset consists of the surface sediment assemblages from the SPNA ocean. We analyzed the average species composition for each region, i.e., I. Irminger Sea II. Iceland Basin and III. Rockall Plateau and IFSC in both datasets. The assemblages observed in the dataset mentioned above and our samples are generally similar in species composition except for the absence of Globorotalia hirsuta and Globorotalia truncatulinoids in the present data.
We compared the planktic foraminifera relative abundance data for each region in both datasets (Figure 9) to illustrate a possible effect of the oceanographic shift on the planktic foraminifera assemblages. The most significant faunal change within the groups was found in the Irminger Sea and Iceland basin. Irminger Sea assemblage is comprised of N. pachyderma, N. incompta, G. glutinata, G. bulloides, and T. quinqueloba in our study. Whereas, Iceland basin and Rockall Plateau-IFSC assemblage consist of all the species of the Irminger Sea assemblage along with G. inflata in the present study. Compared to Matul et al.’s (2018) data, T. quinqueloba percentage has increased, and percentages of G. bulloides and G. glutinata has decreased in our study area in the Irminger Sea. Likewise, in the Iceland basin, T. quinqueloba percentage has increased, and G. glutinata and G. bulloides percentage have decreased in this study in comparison to Matul et al.’s (2018) data. The present study also observed an increased relative abundance of G. glutinata and decreased G. bulloides in Rockall Plateau-IFSC region. Thus, an increase in subpolar species T. quinqueloba in the Irminger Sea and Iceland Basin from our data could indicate an increase in surface productivity and a relative increase in the cold subpolar water influx in the region. Also, a decrease in G. inflata percentage in both the Iceland basin and Rockall-IFSC could indicate a decrease in the influx of warm NAC water to the region (Staines-Urías et al., 2013).
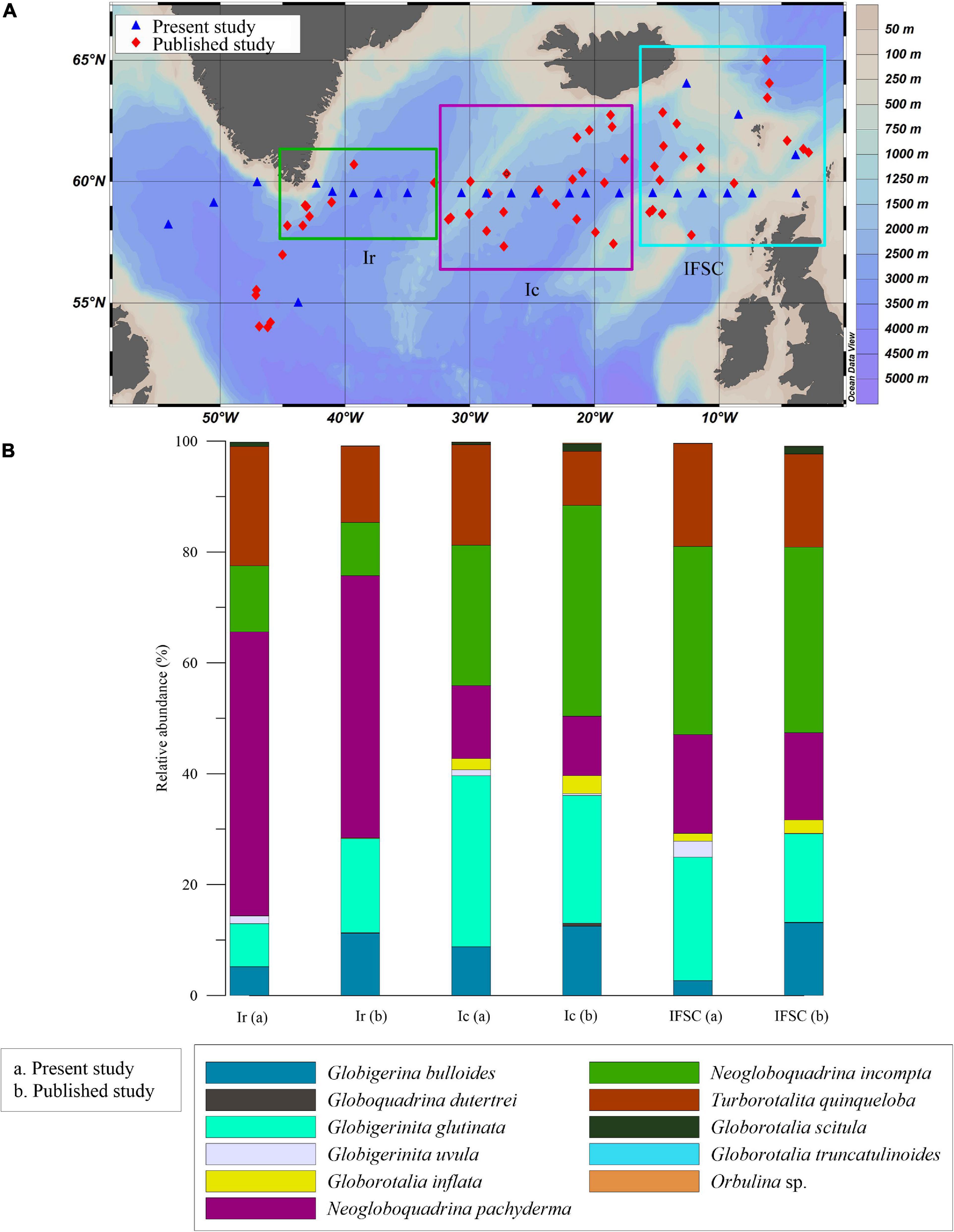
Figure 9. (A) Map showing locations of the samples from Matul et al. (2018) (red diamond) and from our study (blue triangle). (B) Comparison of the relative abundance (%) of planktic species from this study (a) with Matul et al. (2018) (b), shown in the bar chart; Ir, Irminger Sea; Ic, Iceland Basin; IFSC, Rockall Plateau and Iceland-Faroe-Shetland Channel.
The abundance of G. inflata at the eastern side of the transect has been used as a potential indicator of the NAC water influx (Staines-Urías et al., 2013). A lower NAC influx is linked with a strengthened and expanded SPG (more eastward) and vice-versa (Hátún et al., 2005; Staines-Urías et al., 2013). Hence, a decreased influx of G. inflata at the eastern side of the transect, i.e., near Faroes, can be due to strengthened and expanded SPG. Moreover, an ongoing increase in the Greenland Ice sheet melt affects the Irminger Sea and the Labrador Sea the most, increasing fresh water influx (Bamber et al., 2012; Yang et al., 2016). The Labrador Sea assemblage in our study also shows an increase in cold water species N. pachyderma (>100 μm), G. uvula, and T. quinqueloba (>63 μm), indicating a possible drop in temperature and salinity of the upper water column with an increased influence of SPG activity and an increase in freshwater flux. Thus, a slight shift in planktic foraminiferal assemblage in the SPNA ocean was observed in the present dataset. A further study on the present planktic foraminiferal distribution in the water column (plankton tow) in the SPNA ocean will be an important addition to this study.
Conclusion
The present study documents the planktic foraminiferal distribution and species composition in the SPNA Ocean.
(1) In the SPNA Ocean, the recent planktic foraminifera in surface sediments shows a close correlation with the surface-subsurface water mass structure.
(2) Based on cluster analysis, three planktonic foraminifera groups could be identified across the transect in the SPNA Ocean. The polar group is marked by the dominance of N. pachyderma in the Labrador Sea. The mixed group is represented by both warm and cold assemblage (N. pachyderma, N. incompta, G. glutinata, and T. quinqueloba) from the different locations on the western and eastern sides of the transect. Lastly, a warm temperate group included stations from Reykjanes Ridge to Iceland Basin and Rockall Plateau with warm-water assemblage composed of G. glutinata, N. incompta, T. quinqueloba, G. bulloides, and G. uvula.
(3) The ratio N. pachyderma and N. incompta can be used as a proxy to identify the difference between cold Irminger water and relatively warmer Atlantic water in the studied transect.
(4) The distribution of N. pachyderma in the Labrador Sea indicates the presence of cold and fresh water mass. In the Labrador Sea, N. pachyderma was dominant in >100 μm fraction while G. uvula dominated in 63–100 μm fraction. It can be concluded that size is an important factor in studying the planktic foraminiferal abundances and distribution of species.
(5) A comparison of our planktic foraminifera data with the previous dataset reveals a slight shift in planktic foraminiferal assemblage in the SPNA ocean.
Data Availability Statement
The original contributions presented in the study are included in the Supplementary Material, further inquiries can be directed to the corresponding author/s.
Author Contributions
NS carried out the analysis and wrote the original draft of the manuscript along with the conceptualization. SS contributed toward data curation, visualized, conceptualized, wrote, and revised the manuscript. AM provided samples, additional data, and helped to wrote the manuscript. RM supervised the work and reviewed the original draft of the manuscript. AT and NK collected the samples. All authors contributed to the article and approved the submitted version.
Funding
This work was supported by the ESSO-National Centre for Polar and Ocean Research, Ministry of Earth Sciences, India. AM, AT, and NK acknowledge the Russian Science Foundation, Project No. 21-17-00235, with additional funding from the Ministry of Science and Higher Education of the Russian Federation, SIO theme no. 0128-2021-0006 (sediment sampling during cruise).
Conflict of Interest
The authors declare that the research was conducted in the absence of any commercial or financial relationships that could be construed as a potential conflict of interest.
Publisher’s Note
All claims expressed in this article are solely those of the authors and do not necessarily represent those of their affiliated organizations, or those of the publisher, the editors and the reviewers. Any product that may be evaluated in this article, or claim that may be made by its manufacturer, is not guaranteed or endorsed by the publisher.
Acknowledgments
The authors would like to thank the Director, ESSO-NCPOR, Ministry of Earth Sciences (MoES), for supporting the research at NCPOR (Contribution no. J-72/2021-22). NS was grateful to ESSO and NCPOR, for providing research fellowship. The authors thank the crew and participants of the 51st cruise of the RV Akademik Ioffe in 2016 for help in retrieving the sediment samples. Sahina Ghazi and Akshaya are acknowledged for SEM analysis of samples. The authors also extend their sincere gratitude to Polar Micropaleontology and the Past Climate group, NCPOR, for their contribution to this study.
Supplementary Material
The Supplementary Material for this article can be found online at: https://www.frontiersin.org/articles/10.3389/fmars.2021.781675/full#supplementary-material
Footnotes
References
Azetsu-Scott, K., Clarke, A., Falkner, K., Hamilton, J., Jones, E. P., Lee, C., et al. (2010). Calcium carbonate saturation states in the waters of the Canadian Arctic Archipelago and the Labrador Sea. J. Geophys. Res. Oceans 115:C11021. doi: 10.1029/2009JC005917
Bamber, J., Van Den Broeke, M., Ettema, J., Lenaerts, J., Rignot, E., and Bauch, H. A. (2012). Recent large increases in freshwater fluxes from Greenland into the North Atlantic. Geophys. Res. Lett. 39:L19501.
Bauch, H. A. (1994). Significance of variability in Turborotalita quinqueloba (Natland) test size and abundance for paleoceanographic interpretations in the Norwegian-Greenland Sea. Mar. Geol. 121, 129–141. doi: 10.1016/0025-3227(94)90162-7
Bé, A. W. H., and Tolderlund, D. S. (1971). “Distribution and ecology of living planktonic Foraminifera in surface waters of the Atlantic and Indian oceans,” in Micropalaeontology of the Oceans, eds B. M. Funnel and W. R. Riedel (London: Cambridge University Press), 105–149.
Berger, W. H. (1969). Ecologic patterns of living planktonic foraminifera. Deep Sea Res. Oceanograph. Abs. 16, 1–24. doi: 10.1038/s41598-020-77179-8
Berger, W. H. (1973). Deep-sea carbonates. J. Foraminiferal Res. 3, 187–195. doi: 10.2113/gsjfr.3.4.187
Boltovskoy, E., and Wright, R. C. (2013). Recent Foraminifera. Berlin: Springer Science & Business Media.
Brambilla, E., and Talley, L. D. (2008). Subpolar mode water in the northeastern Atlantic: 1. J. Geophys. Res. Ocean. 113, 1–18. doi: 10.1029/2006JC004062
Carstens, J., Hebbeln, D., and Wefer, G. (1997). Distribution of planktic foraminifera at the ice margin in the Arctic (Fram Strait). Mar. Micropaleontol. 29, 257–269. doi: 10.1016/S0377-8398(96)00014-X
Chapman, M. R. (2010). Seasonal production patterns of planktonic foraminifera in the NE Atlantic Ocean: implications for paleotemperature and hydrographic reconstructions. Paleoceanography 25:A1101. doi: 10.1016/j.marmicro.2005.02.008
Clarke, K. R. (1993). Non-parametric multivariate analyses of changes in community structure. Aust. J. Ecol. 18, 117–143. doi: 10.1111/j.1442-9993.1993.tb00438.x
Cléroux, C., Cortijo, E., Duplessy, J. C., and Zahn, R. (2007). Deep-dwelling foraminifera as thermocline temperature recorders. Geochem. Geophy. Geosy. 8:4 doi: 10.1029/2006GC001474
Daniault, N., Mercier, H., Lherminier, P., Sarafanov, A., Falina, A., Zunino, P., et al. (2016). The northern North Atlantic Ocean mean circulation in the early 21st century. Prog. Oceanogr. 146, 142–158. doi: 10.1016/j.pocean.2016.06.007
Dickson, R., Rudels, B., Dye, S., Karcher, M., Meincke, J., and Yashayaev, I. (2007). Current estimates of freshwater flux through Arctic and subarctic seas. Prog. Oceanogr. 73, 210–230. doi: 10.1016/j.pocean.2006.12.003
Espitalié, J., Laporte, J. L., Madec, M., Marquis, F., Leplat, P., Paulet, J., et al. (1977). Rapid method for source rocks characterization and for determination of petroleum potential and degree of evolution. Revue De L InstitutFrancais Du Petrole 32, 23–42.
Eynaud, F. (2011). Planktonic foraminifera in the Arctic: potentials and issues regarding modern and quaternary populations. IOP Conf. Ser. Earth Environ. Sci. IOP Publishing. 14:012005. doi: 10.1088/1755-1315/14/1/012005
Eynaud, F., De Abreu, L., Voelker, A., Schönfeld, J., Salgueiro, E., Turon, J. L., et al. (2009). Position of the Polar Front along the western Iberian margin during key cold episodes of the last 45 ka. Geochem. Geophy. Geosy. 10:Q07U05.
Fairbanks, R. G., and Wiebe, P. H. (1980). Foraminifera and chlorophyll maximum: vertical distribution, seasonal succession, and paleoceanographic significance. Science 209, 1524–1526.
Fossheim, M., Primicerio, R., Johannesen, E., Ingvaldsen, R. B., Aschan, M. M., and Dolgov, A. V. (2015). Recent warming leads to a rapid borealization of fish communities in the Arctic. Nat. Clim. Change. 5, 673–677. doi: 10.1038/nclimate2647
Ganssen, G. M., and Kroon, D. (2000). The isotopic signature of planktonic foraminifera from NE Atlantic surface sediments: implications for the reconstruction of past oceanic conditions. J. Geol. Soc. London. 157, 693–699. doi: 10.1144/jgs.157.3.693
García-Ibáñez, M. I., Pardo, P. C., Carracedo, L. I., Mercier, H., Lherminier, P., Rios, A. F., et al. (2015). Structure, transports and transformations of the water masses in the Atlantic Subpolar Gyre. Prog. Oceanogr. 135, 18–36. doi: 10.1016/j.pocean.2015.03.009
Greco, M., Jonkers, L., Kretschmer, K., Bijma, J., and Kucera, M. (2019). Depth habitat of the planktonic foraminifera Neogloboquadrina pachyderma in the northern high latitudes explained by sea-ice and chlorophyll concentrations. Biogeosciences 16, 3425–3437. doi: 10.5194/bg-16-3425-2019
Gyldenfeldt, A. B. V., Carstens, J., and Meincke, J. (2000). Estimation of the catchment area of a sediment trap by means of current meters and foraminiferal tests. Deep Sea Res. Part II Top. Stud. Oceanogr. 47, 1701–1717. doi: 10.1016/s0967-0645(00)00004-7
Hátún, H., Payne, M. R., Beaugrand, G., Reid, P. C., Sandø, A. B., Drange, H., et al. (2009). Large bio-geographical shifts in the north-eastern Atlantic Ocean: from the subpolar gyre, via plankton, to blue whiting and pilot whales. Prog. Oceanogr. 80, 149–162. doi: 10.1016/j.pocean.2009.03.001
Hátún, H., Sandø, A. B., Drange, H., Hansen, B., and Valdimarsson, H. (2005). Influence of the Atlantic subpolar gyre on the thermohaline circulation. Science 309, 1841–1844. doi: 10.1126/science.1114777
Hemleben, Ch, Spindler, M., and Anderson, R. (1989). Modern Planktonic Foraminifera. New York: Springer.
Hilbrecht, H. (1996). “Extant planktonic foraminifera and the physical environment in the Atlantic and Indian Oceans,” in Mitteilungen Aus Dem Geologischen Institut Der Eidgen, (Zürich: Technischen Hochschule Und Der Universität), 93.
Honjo, S., and Manganini, S. J. (1993). Annual biogenic particle fluxes to the interior of the North Atlantic Ocean; studied at 34 N 21 W and 48 N 21 W. Deep Sea Res. Part II Top. Stud. Oceanogr. 40, 587–607. doi: 10.1016/0967-0645(93)90034-K
Husum, K., and Hald, M. (2012). Arctic planktic foraminiferal assemblages: implications for subsurface temperature reconstructions. Mar. Micropaleontol. 96, 38–47. doi: 10.1016/j.marmicro.2012.07.001
Johannessen, T., Jansen, E., Flatøy, A., and Ravelo, A. C. (1994). “The relationship between surface water masses, oceanographic fronts and paleoclimatic proxies in surface sediments of the Greenland, Iceland, Norwegian Seas,” in Proceedings of the Carbon cycling in the glacial ocean: constraints on the ocean’s role in global change, (Berlin: Springer), 61–85. doi: 10.1007/978-3-642-78737-9_4
Jonkers, L., Brummer, G. J. A., Peeters, F. J. C., Van Aken, H. M., and De Jong, M. F. (2010). Seasonal stratification, shell flux, and oxygen isotope dynamics of leftcoiling N. pachyderma and T. quinqueloba in the western subpolar North Atlantic. Paleoceanography 25, 1–13. doi: 10.1029/2009PA001849
Jonkers, L., Hillebrand, H., and Kucera, M. (2019). Global change drives modern plankton communities away from the pre-industrial state. Nature 570, 372–375. doi: 10.1038/s41586-019-1230-3
Kandiano, E. S., and Bauch, H. A. (2002). Implications of planktic foraminiferal size fractions for the glacial-interglacial paleoceanography of the polar North Atlantic. J. Foraminiferal Res. 32, 245–251. doi: 10.2113/32.3.245
Keller, G., and Abramovich, S. (2009). Lilliput effect in late Maastrichtian planktic foraminifera: response to environmental stress. Palaeogeogr. Palaeoclimatol. Palaeoecol. 284, 47–62. doi: 10.1016/j.palaeo.2009.08.029
Kennett, J. P., and Srinivasan, M. S. (1983). Neogene Planktonic Foraminifera. Pennsylvania: Hutchinson Ross Publ. Go, 265.
Kimura, S., Nakata, H., and Okazaki, Y. (2000). Biological production in meso-scale eddies caused by frontal disturbances of the Kuroshio Extension. ICES J. Mar. Sci. 57, 133–142. doi: 10.1006/jmsc.1999.0564
Knutsen, Ø, Svendsen, H., Øterhus, S., Rossby, T., and Hansen, B. (2005). Direct measurements of the mean flow and eddy kinetic energy structure of the upper ocean circulation in the NE Atlantic. Geophys. Res. Lett 321:14 doi: 10.1029/2005GL023615
Kohfeld, K. E., Fairbanks, R. G., Smith, S. L., and Walsh, I. D. (1996). Neogloboquadrina pachyderma (sinistral coiling) as paleoceanographic tracers in polar oceans: evidence from Northeast Water Polynya plankton tows, sediment traps, and surface sediments. Paleoceanography 11, 679–699. doi: 10.1029/96PA02617
Kretschmer, K., Jonkers, L., Kucera, M., and Schulz, M. (2018). Modeling seasonal and vertical habitats of planktonic foraminifera on a global scale. Biogeosciences 15, 4405–4429. doi: 10.5194/bg-15-4405-2018
Kucera, M. (2007). “Chapter six planktonic foraminifera as tracers of past oceanic environments,” in Methods in late Cenozoic paleoceanography, Development in Marine Geology, eds C. Hillaire-Marcel and A. de Vernal. (New York, NY: Elsevier), 213–262. doi: 10.1016/s1572-5480(07)01011-1
Kuroyanagi, A., and Kawahata, H. (2004). Vertical distribution of living planktonic foraminifera in the seas around Japan. Mar. Micropaleontol. 53, 173–196. doi: 10.1016/j.marmicro.2004.06.001
Locarnini, R. A., Mishonov, A. V., Baranova, O. K., Boyer, T. P., Zweng, M. M., Garcia, H. E., et al. (2018). World Ocean Atlas 2018. Mishonov. Technical. 81:52. doi: 10.1364/OE.443151
Lundgreen, U. (1996). Aminosa”uren im Nordatlantik: partikelzusammensetzung und Remineralisierung. Berichte aus dem Institut für Meereskunde an der Christian-Albrechts-Universität Kiel 283, 1–128. doi: 10.1007/s11306-018-1416-y
Lutjeharms, J. R. E., Walters, N. M., and Allanson, B. R. (1985). “Oceanic frontal systems and biological enhancement,” in Antarctic Nutrient Cycles and Food Webs, eds W. R. Siegfried, P. R. Condy, and R. M. Laws (Berlin: Springer), 11–21. doi: 10.1007/978-3-642-82275-9_3
Mahadevan, A., D’asaro, E., Lee, C., and Perry, M. J. (2012). Eddy-driven stratification initiates North Atlantic spring phytoplankton blooms. Science 337, 54–58. doi: 10.1126/science.1218740
Mallo, M., Ziveri, P., Graham Mortyn, P., Schiebel, R., and Grelaud, M. (2017). Low planktic foraminiferal diversity and abundance observed in a spring 2013 west-east Mediterranean Sea plankton tow transect. Biogeosciences 14, 2245–2266. doi: 10.5194/bg-14-2245-2017
Matul, A., Barash, M. S., Khusid, T. A., Behera, P., and Tiwari, M. (2018). Paleoenvironment Variability during Termination I at the Reykjanes Ridge, North Atlantic. Geoscience 8:375. doi: 10.3390/geosciences8100375
McCartney, M. S., and Talley, L. D. (1982). The subpolar mode water of the North Atlantic Ocean. J. Phys. Oceanogr. 12, 1169–1188. doi: 10.1175/1520-0485(1982)012<1169:tsmwot>2.0.co;2
Meilland, J., Howa, H., Hulot, V., Demangel, I., Salaün, J., and Garlan, T. (2020). Population dynamics of modern planktonic foraminifera in the western Barents Sea. Biogeosciences 17, 1437–1450. doi: 10.5194/bg-17-1437-2020
Melling, M., Agnew, T. A., Falkner, K. K., Greenberg, D. A., Lee, C. M., Munchow, A., et al. (2008). “Fresh-water fluxes via Pacific and Arctic outflows across Canadian Polar Shelf,” in Arctic-Subarctic Ocean Fluxes, Defining the Role of the Northern Seas in Climate, eds R. R. Dickson, J. Meinke, and P. Rhines (Dordrecht: Springer), 193–247.
NASA Goddard Space Flight Center (2018). Moderate-resolution Imaging Spectroradiometer (MODIS) Aqua Chlorophyll Data, 2018: Reprocessing. NASA OB.DAAC. Available online at: https://doi.org/10.5067/AQUA/MODIS/L3B/CHL/2018 [accessed Feb 20, 2019]
Neukermans, G., Oziel, L., and Babin, M. (2018). Increased intrusion of warming Atlantic water leads to rapid expansion of temperate phytoplankton in the Arctic. Glob. Chang. Biol. 24, 2545–2553. doi: 10.1111/gcb.14075
Ortiz, J. D., and Mix, A. C. (1992). “The spatial distribution and seasonal succession of planktic foraminifera in the California Current off Oregon,” in Upwelling systems: Evolution since the Early Miocene. Geological Society, Vol. 64, eds C. P. Summerhayes, W. L. Prell, and K. C. Emeis (London: Special Publications), 197–213.
Ortiz, J. D., Mix, A. C., and Collier, R. W. (1995). Environmental control of living symbiotic and asymbiotic foraminifera of the California Current. Paleoceanography 10, 987–1009. doi: 10.1029/95pa02088
Osman, M. B., Das, S. B., Trusel, L. D., Evans, M. J., Fischer, H., Grieman, M. M., et al. (2019). Industrial-era decline in subarctic Atlantic productivity. Nature 569, 551–555. doi: 10.1038/s41586-019-1181-8
Ottens, J. J. (1991). Planktic foraminifera as North Atlantic water mass indicators. Oceanol. Acta 14, 123–140. doi: 10.1371/journal.pone.0239373
Ottens, J. J. (1992). April and August Northeast Atlantic surface water masses reflected in planktic foraminifera. Netherlands J. Sea Res. 28, 261–283. doi: 10.1016/0077-7579(92)90031-9
Oziel, L., Neukermans, G., Ardyna, M., Lancelot, C., Tison, J. L., Wassmann, P., et al. (2017). Role for Atlantic inflows and sea ice loss on shifting phytoplankton blooms in the Barents Sea. J. Geophys. Res. Oceans 122, 5121–5139. doi: 10.1002/2016JC012582
Pados, T., and Spielhagen, R. F. (2014). Species distribution and depth habitat of recent planktic foraminifera in Fram Strait, Arctic Ocean. Polar Res. 33:483 doi: 10.3402/polar.v33.22483
Parker, F. L. (1960). Living planktonic foraminifera from the Equatorial and Southeast Pacific. Science reports of the Tohoku University. 2nd series, Geology. Special 4, 71–82.
Parker, F. L., and Berger, W. H. (1971). Faunal and solution patterns of planktonic foraminifera in surface sediments of the South Pacific. Deep Sea Res. Oceanograph. ABS. 18, 73–107. doi: 10.1016/0011-7471(71)90017-9
Pflaumann, U., Sarnthein, M., Chapman, M., d’Abreu, L., Funnell, B., Huels, M., et al. (2003). Glacial North Atlantic: Sea-surface conditions reconstructed by GLAMAP 2000. Paleoceanography 18:1065
Pollard, R. T., Griffiths, M. J., Cunningham, S. A., Read, J. F., Pérez, F. F., and Ríos, A. F. (1996). Vivaldi 1991 - A study of the formation, circulation and ventilation of Eastern North Atlantic Central Water. Prog. Oceanogr. 37, 167–172. doi: 10.1016/S0079-6611(96)00008-0
Pollard, R. T., Read, J. F., Holliday, N. P., and Leach, H. (2004). Water masses and circulation pathways through the Iceland basin during Vivaldi 1996. J. Geophys. Res. C. Ocean 109, 1–10. doi: 10.1029/2003JC002067
CLIMAP Project Members. (1976). The surface of the ice-age earth. Science 191, 1131–1137. doi: 10.1126/science.191.4232.1131
Rahmstorf, S., Box, J. E., Feulner, G., Mann, M. E., Robinson, A., and Rutherford, et al. (2015). Exceptional twentieth-century slowdown in Atlantic Ocean overturning circulation. Nat. Clim. Change. 5, 475–480. doi: 10.1038/nclimate2554
Read, J. F. (2000). CONVEX-91: water masses and circulation of the Northeast Atlantic subpolar gyre. Prog. Oceanogr. 48, 461–510. doi: 10.1016/S0079-6611(01)00011-8
Rebotim, A., Voelker, A. H. L., Jonkers, L., Waniek, J. J., Meggers, H., Schiebel, R., et al. (2017). Factors controlling the depth habitat of planktonic foraminifera in the subtropical eastern North Atlantic. Biogeosciences 14, 827–859. doi: 10.5194/bg-14-827-2017
Retailleau, S., Eynaud, F., Mary, Y., Abdallah, V., Schiebel, R., and Howa, H. (2012). Canyon heads and river plumes: how might they influence neritic planktonic foraminifera communities in the SE Bay of Biscay? J. Foraminiferal Res. 42, 257–269. doi: 10.2113/gsjfr.42.3.257
Retailleau, S., Howa, H., Schiebel, R., Lombard, F., Eynaud, F., Schmidt, S., et al. (2009). Planktic foraminiferal production along an offshore–onshore transect in the south-eastern Bay of Biscay. Cont. Shelf Res. 29, 1123–1135. doi: 10.1016/j.csr.2008.12.021
Retailleau, S., Schiebel, R., and Howa, H. (2011). Population dynamics of living planktic foraminifers in the hemipelagic southeastern Bay of Biscay. Mar. Micropaleontol. 80, 89–100. doi: 10.1016/j.marmicro.2011.06.003
Salgueiro, E., Voelker, A. H., de Abreu, L., Abrantes, F., Meggers, H., and Wefer, G. (2010). Temperature and productivity changes off the western Iberian margin during the last 150 ky. Quat. Sci. Rev. 29, 680–695.
Schiebel, R. (2002). Planktic foraminiferal sedimentation and the marine calcite budget. Global Biogeochem Cy. 16, 3–21.
Schiebel, R., and Hemleben, C. (2000). Interannual variability of planktic foraminiferal populations and test flux in the eastern North Atlantic Ocean (JGOFS). Deep Sea Res. Part II Top. Stud. Oceanogr. 47, 1809–1852. doi: 10.1016/S0967-0645(00)00008-4
Schiebel, R., and Hemleben, C. (2005). Modern planktic foraminifera. Paläontologische Zeitschrift 79, 135–148. doi: 10.1007/BF03021758
Schiebel, R., and Hemleben, C. (2017). Planktic Foraminifers in the Modern Ocean. Berlin: Springer, 1–358.
Schiebel, R., Hiller, B., and Hemleben, C. (1995). Impacts of storms on recent planktic foraminiferal test production and CaCO3 flux in the North Atlantic at 47° N, 20° W (JGOFS). Mar. Micropaleontol. 26, 115–129.
Schiebel, R., Spielhagen, R. F., Garnier, J., Hagemann, J., Howa, H., Jentzen, A., et al. (2017). Modern planktic foraminifers in the high-latitude ocean. Mar. Micropaleontol. 136, 1–13. doi: 10.1016/j.marmicro.2017.08.004
Schiebel, R., Waniek, J., Bork, M., and Hemleben, C. (2001). Planktic foraminiferal production stimulated by chlorophyll redistribution and entrainment of nutrients. Deep Sea Res. Part I Oceanogr. Res. Pap. 48, 721–740. doi: 10.1016/S0967-0637(00)00065-0
Schlitzer, R. (2015). Data Analysis and Visualization with Ocean Data View. CMOS Bull. SCMO 43, 9–13.
Schmuker, B. (2000). The influence of shelf vicinity on the distribution of planktic foraminifera south of Puerto Rico. Mar. Geol. 166, 125–143. doi: 10.1016/S0025-3227(00)00014-1
Spooner, P. T., Thornalley, D. J., Oppo, D. W., Fox, A. D., Radionovskaya, S., Rose, N. L., et al. (2020). Exceptional 20th century ocean circulation in the Northeast Atlantic. Geophys. Res. Lett. 47:e2020GL087577. doi: 10.1029/2020GL087577
Staines-Urías, F., Kuijpers, A., and Korte, C. (2013). Evolution of subpolar North Atlantic surface circulation since the early Holocene inferred from planktic foraminifera faunal and stable isotope records. Quat. Sci. Rev. 76, 66–81. doi: 10.1016/j.quascirev.2013.06.016
Stangeew, E. (2001). Distribution and Isotopic Composition of Living Planktonic Foraminifera N. pachyderma (sinistral) and T. quinqueloba in the High Latitude North Atlantic. Ph.D. thesis, Kiel: Christian-Albrechts Universität.
Stehman, C. F. (1972). Planktonic foraminifera in Baffin Bay. Davis Strait and the Labrador Sea. Atl. Geol. 8, 13–19.
Taylor, J. R., and Ferrari, R. (2011b). Shutdown of turbulent convection as a new criterion for the onset of spring phytoplankton blooms. Limnol. Oceanogr. 56, 2293–2307. doi: 10.4319/lo.2011.56.6.2293
Taylor, J. R., and Ferrari, R. (2011a). Ocean fronts trigger high latitude phytoplankton blooms. Geophys. Res. Lett 38:2011 doi: 10.1029/2011GL049312
Thornalley, D. J., Oppo, D. W., Ortega, P., Robson, J. I., Brierley, C. M., Davis, R., et al. (2018). Anomalously weak Labrador Sea convection and Atlantic overturning during the past 150 years. Nature 556, 227–230. doi: 10.1038/s41586-018-0007-4
Thunell, R. C. (1978). Distribution of recent planktonic foraminifera in surface sediments of the Mediterranean Sea. Mar. Micropaleontol. 3, 147–173. doi: 10.1016/0377-8398(78)90003-8
Thunell, R. C., and Reynolds, L. A. (1984). Sedimentation of planktonic foraminifera; seasonal changes in species flux in the Panama Basin. Micropaleontology 30, 243–262.
Tolderlund, D. S., and Bé, A. W. (1971). Seasonal distribution of planktonic foraminifera in the western North Atlantic. Micropaleontology 17, 297–329. doi: 10.1126/science.209.4464.1524
Volkmann, R. (2000). Planktic foraminifers in the outer Laptev Sea and the Fram Strait—Modern distribution and ecology. J. Foraminiferal Res. 30, 157–176. doi: 10.2113/0300157
Yang, Q., Dixon, T. H., Myers, P. G., Bonin, J., Chambers, D., Van Den Broeke, M. R., et al. (2016). Recent increases in Arctic freshwater flux affects Labrador Sea convection and Atlantic overturning circulation. Nat. Commun. 7, 1–8. doi: 10.1038/ncomms10525
Žarić, S., Donner, B., Fischer, G., Mulitza, S., and Wefer, G. (2005). Sensitivity of planktic foraminifera to sea surface temperature and export production as derived from sediment trap data. Mar. Micropaleontol. 55, 75–105. doi: 10.1016/j.marmicro.2005.01.002
Zhang, J., Weijer, W., Steele, M., Cheng, W., Verma, T., and Veneziani, M. (2021). Labrador Sea freshening linked to Beaufort Gyre freshwater release. Nat. Commun. 12, 1–8. doi: 10.1038/s41467-021-21470-3
Ziveri, P., Thunell, R. C., and Rio, D. (1995). Export production of coccolithophores in an upwelling region: results from San Pedro Basin, Southern California Borderlands. Mar. Micropaleontol. 24, 335–358. doi: 10.1016/0377-8398(94)00017-h
Keywords: planktic foraminifera, subpolar North Atlantic, cluster analysis, subpolar gyre, water mass
Citation: Sahoo N, Saalim SM, Matul A, Mohan R, Tikhonova A and Kozina N (2022) Planktic Foraminiferal Assemblages in Surface Sediments From the Subpolar North Atlantic Ocean. Front. Mar. Sci. 8:781675. doi: 10.3389/fmars.2021.781675
Received: 23 September 2021; Accepted: 17 December 2021;
Published: 09 February 2022.
Edited by:
Teresa Sofia Giesta da Silva, Marine and Freshwater Research Institute, IcelandReviewed by:
Lucilla Capotondi, Institute of Marine Science, National Research Council (CNR), ItalyIria Sala, University of Strathclyde, United Kingdom
Matthew Walker, University of Leeds, United Kingdom
Copyright © 2022 Sahoo, Saalim, Matul, Mohan, Tikhonova and Kozina. This is an open-access article distributed under the terms of the Creative Commons Attribution License (CC BY). The use, distribution or reproduction in other forums is permitted, provided the original author(s) and the copyright owner(s) are credited and that the original publication in this journal is cited, in accordance with accepted academic practice. No use, distribution or reproduction is permitted which does not comply with these terms.
*Correspondence: Nibedita Sahoo, bmliZWRpdGEuZ2VvOTJAZ21haWwuY29t; Syed Mohammad Saalim, c2Ffc2FhbGltMjAwNkBob3RtYWlsLmNvbQ==