- 1Department of Life and Environmental Sciences, Faculty of Science and Technology, Bournemouth University, Poole, United Kingdom
- 2School of Biological and Marine Sciences, Faculty of Science and Engineering, University of Plymouth, Devon, United Kingdom
- 3Artecology Ltd., Isle of Wight, United Kingdom
Artificial coastal structures (ACSs) are primarily designed to provide services for human use, such as flood defence or shipping, and are generally poor for marine biodiversity. Consequently, there has been significant research effort to enhance these hard structures to increase biodiversity and habitat availability via eco-engineering. On seawalls and breakwaters, this has included the creation of habitats for benthic species found on natural rocky shores, including the provision of cracks, crevices and water retaining features, such as artificial rockpools. When sediment retention in these features has occurred, it has often been deemed detrimental to the overarching aim of the intervention. Yet, it is soft sediment habitat that is impacted the most through coastal construction. As ecological enhancement of a flood defence scheme, nine concrete retrofit rockpools were installed at three different tidal elevations between mean high water neap tide and mean tide level on steel sheet piling on the Arun Estuary in Littlehampton Harbour, United Kingdom, which naturally filled with mud 1 year after installation. To explore how analogous the faunal assemblages and sediment profile of rockpool mud were to two local mudflats, core samples were taken and analysed for species richness, abundance, biomass, assemblage structure, median grain size, and organic matter content. More benthic species were observed in the artificial rockpool than in the local mudflats. Although the rockpools were placed at higher tidal levels than the lower shore mudflat, their assemblage structure and species richness were more similar to the lower shore mudflat at the base of the sheet piling than the upper shore mudflat. This study demonstrates that retained sediment within eco-engineered features on hard ACSs can create habitat for benthic assemblages. Providing sediment-retentive features on ACSs has the potential to provide a novel eco-engineering option that may be appropriate for some heavily modified waterbodies on sheltered, depositional coasts.
Introduction
Coastal squeeze threatens developed coasts with habitat loss as the intertidal area is reduced and steepened between a fixed high tide mark on artificial coastal structures (ACSs) and sea level rise (Doody, 2004; Schleupner, 2008; Pontee, 2011), which is predicted to significantly decrease biodiversity within coastal regions (Hawkins, 2012; Hawkins et al., 2016). ACSs can physically reduce soft-sediment habitat from their construction footprint (Bugnot et al., 2021) and may also contribute to the alteration of natural dynamic soft-bottomed and hydrological processes (Dugan et al., 2018) and the subsequent impacts on benthic species diversity and community structure (Martin et al., 2005; Heery et al., 2017; Critchley and Bishop, 2019).
It is known that ACSs are not analogous habitats to natural intertidal reefs in terms of habitat availability and topographical complexity (Moschella et al., 2005; Chapman and Underwood, 2011; Aguilera et al., 2014) as well as community structure, species richness and biodiversity (Connell and Glasby, 1999; Chapman, 2003; Moschella et al., 2005; McKinney, 2006; Glasby et al., 2007; Vaselli et al., 2008; Pister, 2009). Consequently, there has been significant effort to increase biodiversity and habitat availability via eco-engineering (Firth et al., 2014; Strain et al., 2017; O’Shaughnessy et al., 2019), where habitat is integrated into ACSs (Bergen et al., 2001; Mitsch and Jorgensen, 2003; Odum and Odum, 2003). The predominant aims of eco-engineering on ACSs thus far have been to increase topographical and structural complexity (MacArthur et al., 2019), either via texture (Coombes et al., 2015) or through the creation of microhabitats (Martins et al., 2010, 2016; Loke et al., 2017), and moisture retentive features, such as drill-cored tide pools and retrofitted artificial rockpools (Browne and Chapman, 2014; Hall et al., 2019; Chee et al., 2020).
To date, eco-engineering has focused exclusively on mitigating habitat loss of natural intertidal reefs with the addition of interventions to retain seawater for biota that would normally occur on hard substrate. Yet, it is soft-sediment habitat that is often impacted the most through construction (Airoldi et al., 2005; Firth et al., 2016), from the seabed offshore (Miller et al., 2013) to the coastal benthos (Martin et al., 2005; Bulleri and Chapman, 2010). When sedimentation in eco-engineering features has occurred, it is deemed incidental and potentially detrimental to the overarching aim of the design (sensu Hall et al., 2018; Waltham and Sheaves, 2018). However, in natural ecosystems, sediment provides a substrate for infauna and potential ecosystem services (Barbier et al., 2011; Costanza et al., 2014; Dissanayake et al., 2018), such as organic matter sequestration and nutrient recycling (Cook et al., 2004a,b, Watson et al., 2020). At the time of writing, there were no published studies that quantified the biotic assemblages inhabiting marine sediment accumulated in eco-engineering installations, either by design or incidentally.
Coastal structures disproportionately impact the species richness of muddy sediments compared to sandy sediments, particularly the abundance of infauna such as nereid worms and bivalves (Critchley and Bishop, 2019). Many of these species contribute to valuable ecosystem processes and services. For example, the ragworm Hediste diversicolor (Müller, 1776) is important prey for birds and fish (McLusky and Elliott, 2004) and bioturbation caused by burrowing activity is known to influence biogeochemical cycles (Davey and Watson, 1995; Gunnarsson et al., 1999; Garcia-Arberas and Rallo, 2002). Sediment characteristics, such as total organic matter and median grain size, can be determining factors in species richness and community structure of intertidal infauna (Ellingsen, 2002; Ysebaert and Herman, 2002; Coblentz et al., 2014, 2015). Species size distribution can be indicative of effective recruitment and post-recruitment survival, which is important for maintenance of populations (Hunt and Scheibling, 1997; Dethier et al., 2012; Beal et al., 2018).
Muddy sediments are more likely to occur in sheltered locations, such as estuaries and harbours, where additional stressors co-occur such as greater nutrient loading (Jordan et al., 2018). The installation of eco-engineered features on ACSs, such as on harbour walls and wharves, in these areas are more likely to retain sediment. Therefore, understanding how soft-sediment assemblages compare with natural mudflat habitats is beneficial. In 2018, nine eco-engineered artificial rockpools (known commercially as Vertipools™), were installed on steel sheet piling in Littlehampton Harbour, West Sussex, United Kingdom. It is one of the first known examples of this type of intervention retrofitted to steel coastal structures. The intention was to add complexity to the harbour wall constructed from steel sheet piling and to provide maximum opportunity for colonisation, with the artificial rockpools known to retain mostly seawater at low tide (sensu Hall et al., 2019) and become colonised by benthos characteristic of intertidal rocky shores. However, all artificial rockpools filled to the brim with estuarine mud. To determine what faunal assemblages were found in the rockpool mud and to compare how analogous these artificial habitats were compared to existing local mudflats, sediment samples were taken from both the artificial rockpools and two local mudflat sites. The main research question was, how do biotic assemblages and the sediment characteristics of the artificial rockpool mud compare to local mudflats?
Materials and Methods
Study Site
Littlehampton Harbour is a heavily modified waterbody situated on the south-east coast of England (50.809596 N, 0.54879069 W) at the mouth of the River Arun (Figure 1). It is a small, busy port popular with recreational boating. Its eastern shore is predominantly comprised of steel sheet piling and floating pontoons, with a greater presence of gently sloping mudflats on the western shore. At low water, intertidal mudflats are exposed, and vessels are limited to a narrow channel in the centre of the river. The harbour entrance has a southerly aspect, which is sheltered from prevailing south-westerly winds by West Beach and the presence of a breakwater that juts out past the river mouth. Tidal streams are exceptionally strong reaching 6 knots (3 m s–1) with ebb flows increased after heavy rain. The study site is approximately 1 km north of the harbour entrance. Mean spring tidal range is 5.5 m.
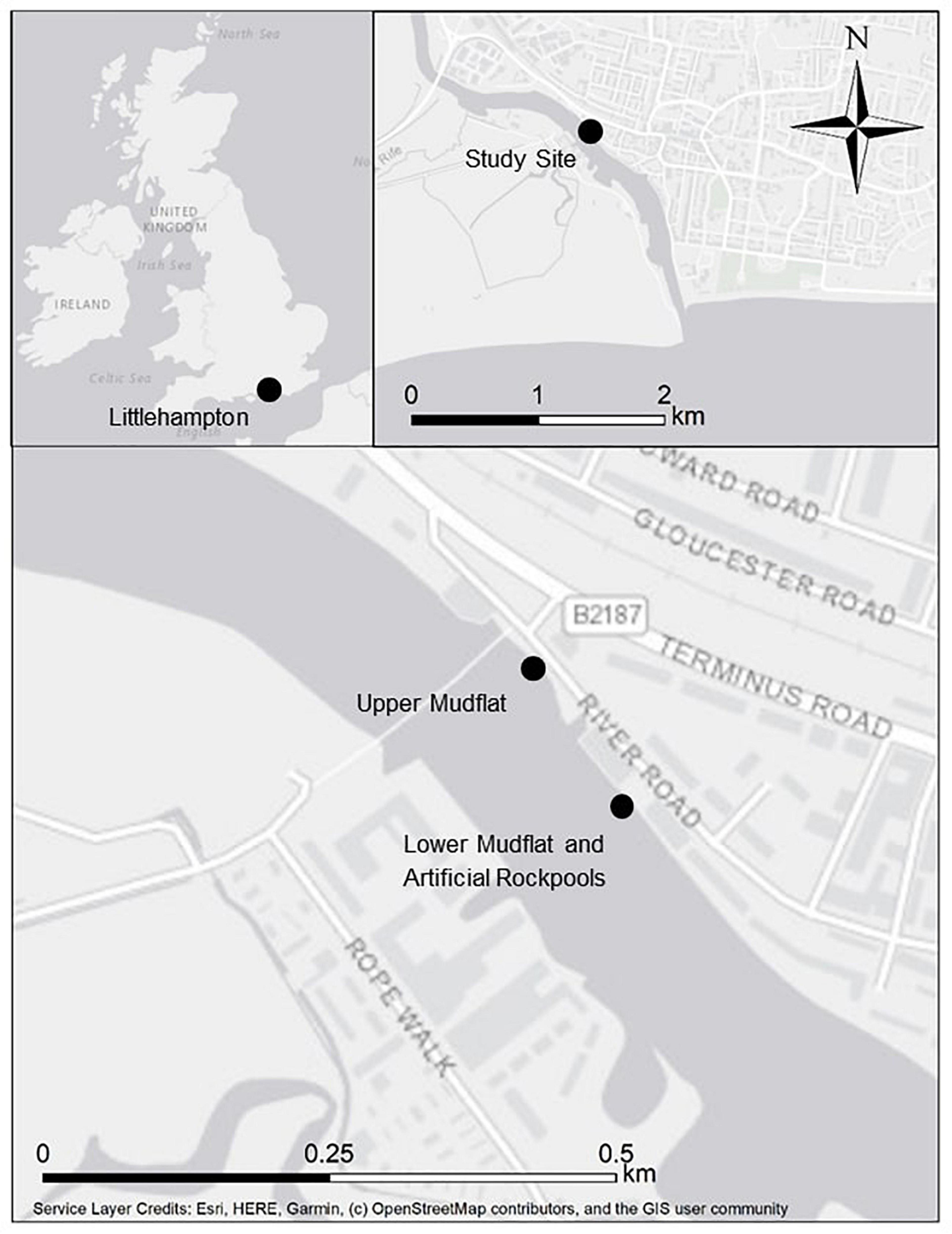
Figure 1. Location of survey sites at Littlehampton Harbour on the River Arun, south coast of England.
Artificial Rockpools
As part of flood defence improvements between 2013 and 2018, new vertical steel sheet pile flood defence walls, capped with concrete coping, were installed along the Eastern Bank of Littlehampton Harbour (Figure 1). A section of this sheet piling included the installation of nine artificial concrete rockpools arranged in three columns of three rockpools at different tidal heights. All rockpools were fixed within the “in-pans” (concave inlets in the structure) of the steel sheet piling (Figure 2). The lowest rockpool in all columns was 40 cm from the toe (± 10 cm) with approximately 50 cm height between each rockpool. The top artificial rockpools were fixed to a height approximating to Mean High Water Neap Tide Level (Table 1). The artificial rockpools were commissioned by the Environment Agency and manufactured by Artecology Ltd. (Sandown, United Kingdom), based on their Vertipool™ Compton design. They were fitted to the steel sheet piling in 2018 as it was constructed, with a custom stainless-steel bracket inlaid into the low-carbon concrete artificial rockpools. The dimensions of the artificial rockpools were 15 cm (h) × 31 cm (w) × 25 cm (d). Maximum mud depth of rockpool interior when full was 6 cm. The experimental design of this study was constrained by the number (n = 9) and arrangement of the artificial rockpools as they were not installed for research purposes.
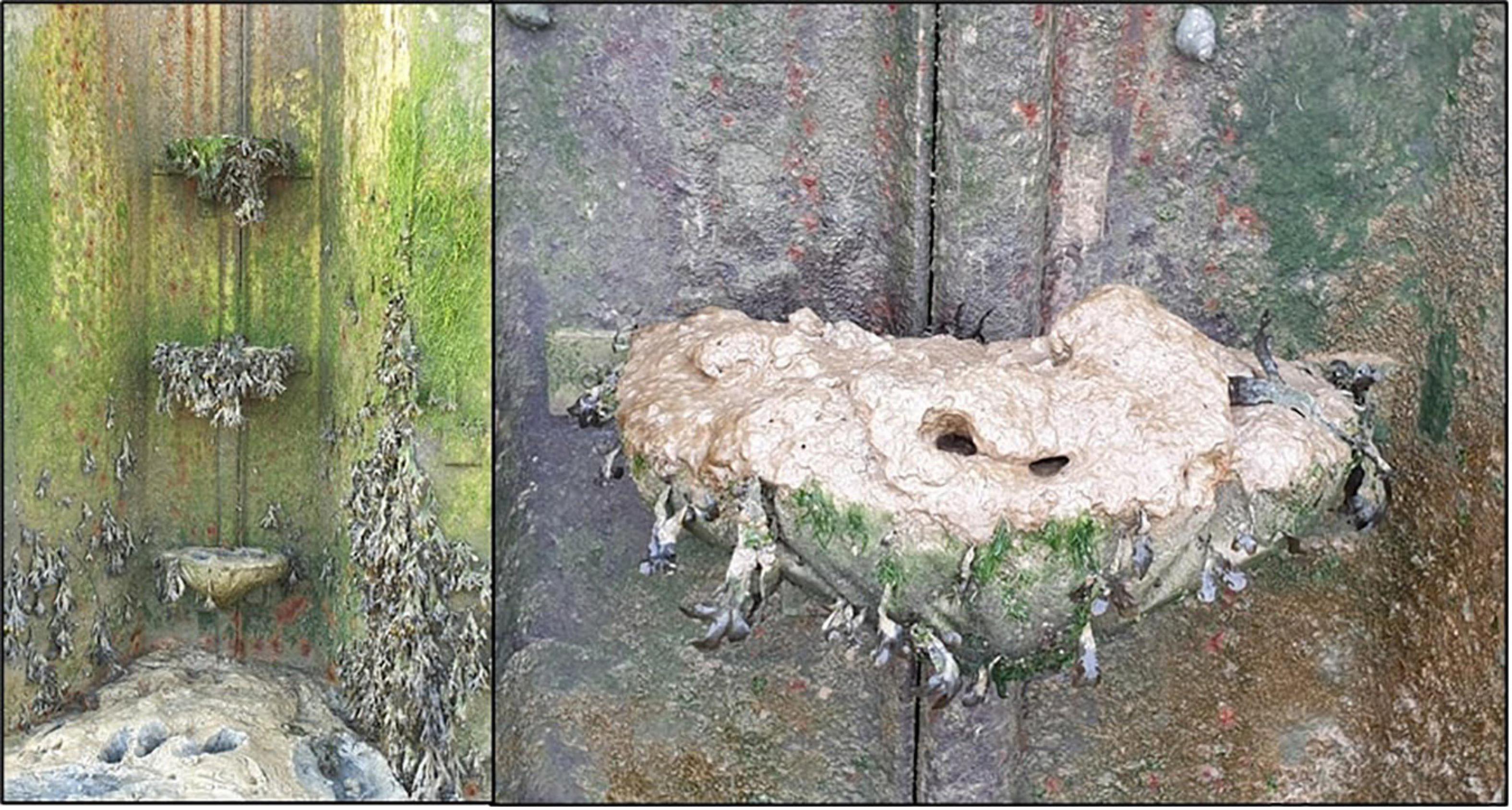
Figure 2. (Left) One of the three columns of artificial rockpools. The rockpools were fixed to the in-pan of the steel sheet piling. The top rockpool was fixed at approximately Mean High Water Neap Tide Level. The foot of the sheet piling was approximately Mean Tide Level. (Right) An artificial rockpool on the steel sheet piling filled with mud, photographed 2.5 years after installation.
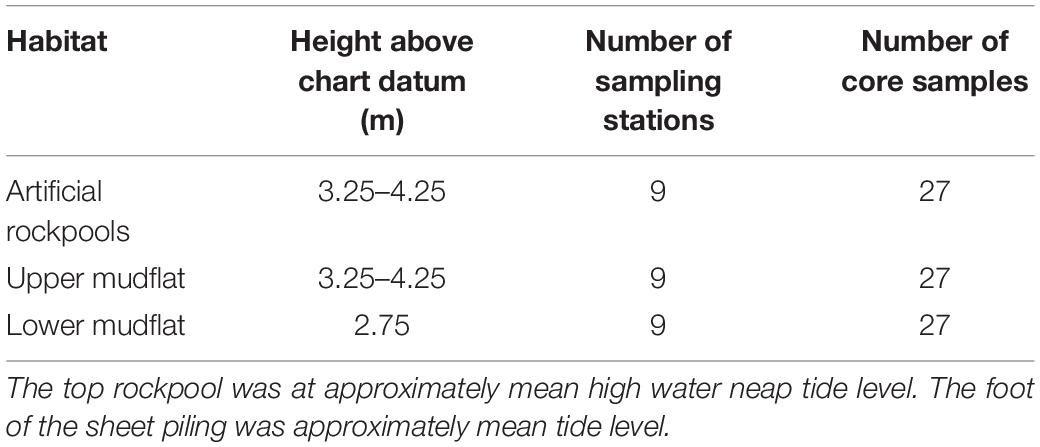
Table 1. Tidal height and number of core samples taken from artificial rockpools and mudflat habitats.
Survey Method
The artificial rockpools and mudflats were both sampled on the same day in September 2020, two and a half years after installation, commencing on an ebbing tide 1 h prior to low water when all habitats were emersed, and finishing 2 h post low water. Three sediment cores were taken from each artificial rockpool using a 6 cm diameter corer to a depth of 5 cm. The core size, sampling depth and number of cores was constrained by the size of the artificial rockpool. A single core was taken and retained in an airtight re-sealable bag for sediment analysis and stored in a freezer (−18°C) the same day to prevent decomposition of organic material. Two additional cores from each artificial rockpool were combined and sieved on site using a 0.5 mm sieve to retain macrofauna, which were transferred to tubs and preserved with 10% formal saline (Fisher Chemical, Loughborough, United Kingdom). The combined cores from each rockpool total an area of 0.075 m2.
The lower mudflat below the artificial rockpools and an upper mudflat 100 m northwest (Figure 1) were both sampled as reference sites. These are also referred to as “natural” mudflats, however, as the estuary has been highly modified, these are potentially contaminated, and the benthic assemblages may differ to mudflats at unmodified locations. Alternative and additional reference sites within the harbour were inaccessible, and sites further away presented the issue of confounding variability. The lower mudflat at the toe of the sheet piling was sampled due to its close proximity but is at a lower tidal height (approximately Mean Tide Level) than the artificial rockpools and upper mudflat. Core samples were also taken at the approximate tidal heights (Mean High Water Neap Tide Level) of the artificial rockpools (±15 cm) at an upper shore mudflat. Due to the sheet piling restricting access to upper shore mudflats, the closest upper mudflat was approximately 100 m upstream where the sheet piling terminated (Figure 1). Three cores (6 cm diameter and 5 cm depth) were taken randomly at each of nine sampling stations on the lower mudflat at the toe of the sheet piling (n = 9) and at nine sampling stations on the upper mudflat at a shore height equivalent to the artificial rockpools (n = 9). To replicate the sampling method used for the artificial rockpools, one core was taken for sediment analysis, and two cores were taken and combined for faunal analysis at each of the nine sampling stations for both mudflats (Table 1).
The sediment samples were defrosted, homogenised and subsampled for sediment analysis. Organic content was determined by drying a homogenised subsample in a Memmert UN55 drying oven (Memmert, Büchenbach, Germany) at 100°C for 48 h, and then placing in a Carbolite Gero CWF chamber furnace (Carbolite Co Ltd., Sheffield, United Kingdom) at 450°C for 12 h and measuring the loss of mass on ignition (% LOI) (Luczak et al., 1997). Water content was determined as the loss of weight following drying. Particle size analysis was conducted using a Mastersizer 3000 laser diffractometer (Malvern Panalytical, Malvern, United Kingdom) with subsamples of the dried sediment (each sample produced five median grain size [D50 – sieve opening which 50% of particles pass through (Yilmaz, 2019)] readings which were used in data analysis (Shakeel et al., 2020).
The biotic samples were rinsed of preservative and viewed under a Leica M165C stereo microscope (Leica, Wetzlar, Germany). Fauna were picked and placed in tubes containing 70% Industrial Methylated Spirit (IMS) (Fisher Chemical, Loughborough, United Kingdom) for longer term storage as voucher specimens. Identification of individuals was made using appropriate keys to lowest taxonomic resolution. All taxonomic authorities provided are as shown in the World Register of Marine Species (WorMS – www.marinespecies.org) accessed December 2021. Organisms without a head and empty shells were discounted. Biomass was obtained by drying organisms in a Memmert UN55 drying oven at 100°C until a constant weight was achieved. Species with shells were left intact as specimens were too small to remove tissue manually. To ensure preservation did not cause changes in weight, organisms were weighed immediately upon removal from preservation fluid to reduce exposure times. The size-frequency of ragworm H. diversicolor was determined in each habitat to compare recruitment potential. The total length of all sampled ragworms were measured using a BMZ-07 stage micrometer calibrated with a BMZ-06 × 10 measuring eyepiece graticule (Brunel Microscopes Ltd., Chippenham, United Kingdom). The peristomium width and total body length from the prostomium to pygidium (when entire specimen was present) was measured. Although metric L3 (sum of the lengths of the prostomium, peristomium and the first chaetiger) is often preferred operationally in condition assessments, Total Length was consistently highly correlated with wet weight (Durou et al., 2007) and considered appropriate for this comparative investigation. Body length data for incomplete specimens was extrapolated from a linear trendline of peristomium width and total length plotted on a scatter graph of complete specimens.
Data Analysis
Data were divided into their respective habitats: artificial rockpool, lower mudflat and upper mudflat. For comparison with the natural mudflats, data from the nine rockpools were treated as a single sample set to compare with the nine sampling stations for each mudflat habitat. Due to low replication (n = 3), analysis of tidal height was not performed on rockpool data. Plymouth Routines in Multivariate Ecological Research (PRIMER-e, version 1.0.6) was used to detect similarities in assemblages between the habitats (Clarke and Gorley, 2006). Species abundance data were square root transformed, to prevent common species being weighted over rare species, and a Bray-Curtis similarity matrix was created between samples. A single factor PERMANOVA design (habitat: artificial rockpool, lower mudflat, upper mudflat) was used to assess the difference in assemblages using species abundance data (Anderson, 2005). Multidimensional scaling (MDS) plots were produced to visually demonstrate the similarity between sample assemblages for habitats and MVDISP was used to provide dispersion indices. SIMPER was used to identify where dissimilarity occurred between habitats and which taxa were most responsible for these differences in assemblage structure. The DIVERSITY function was used to calculate Shannon Weiner diversity index (Clarke and Gorley, 2006).
R version 3.6.3 (R Core Team, 2016) and the “car” (Fox and Weisberg, 2019) and “userfriendlyscience” (Peters, 2016) packages were used to run one-way analysis of variance (ANOVA) tests for average moisture content, organic matter content, median grain size, species richness, abundance, and total biomass with habitat as a fixed factor. Data were tested for normality with Shapiro Wilkes test and were square root transformed if p ≤ 0.05. Tukey post hoc tests were run to determine the direction of significant interactions as indicated by the one-way ANOVAs. A Levene’s test identified unequal variances (p ≤ 0.05) in Shannon Weiner diversity indices, and so a Welch’s ANOVA and Games Howell post hoc test was run instead. ANOVA results are presented as degrees of freedom (df), the F statistic (F), and the p value (p).
Results
Sediment Profile Analysis
Artificial rockpool samples were visibly wetter. A one-way ANOVA revealed the average moisture content (% of weight) of the habitats was significantly different (df 2,23, F = 13.05, p ≤ 0.001). Pairwise tests showed moisture content of artificial rockpool sediment (39%) was significantly greater than both lower (p ≤ 0.01) and upper (p ≤ 0.001) mudflats (Figure 3). The organic content (% loss on ignition, Figure 3) was also significantly different (one-way ANOVA, df 2, 23, F = 6.579, p ≤ 0.01) between habitats and was significantly higher in the artificial rockpools than the lower mudflat (p ≤ 0.01). The one-way ANOVA for median grain size showed a significant difference between median grain sizes of habitats (df 2, 24, F = 350.09, p ≤ 0.001). Median grain size was significantly smaller in the rockpool sediment compared to both mudflats (p ≤ 0.001). Mean median grain size ranges between 48 μm for rockpool sediment and 56 μm for lower mudflat sediment. All habitat samples are predominantly comprised of silt/mud (≤63 μm) (Wentworth, 1922).
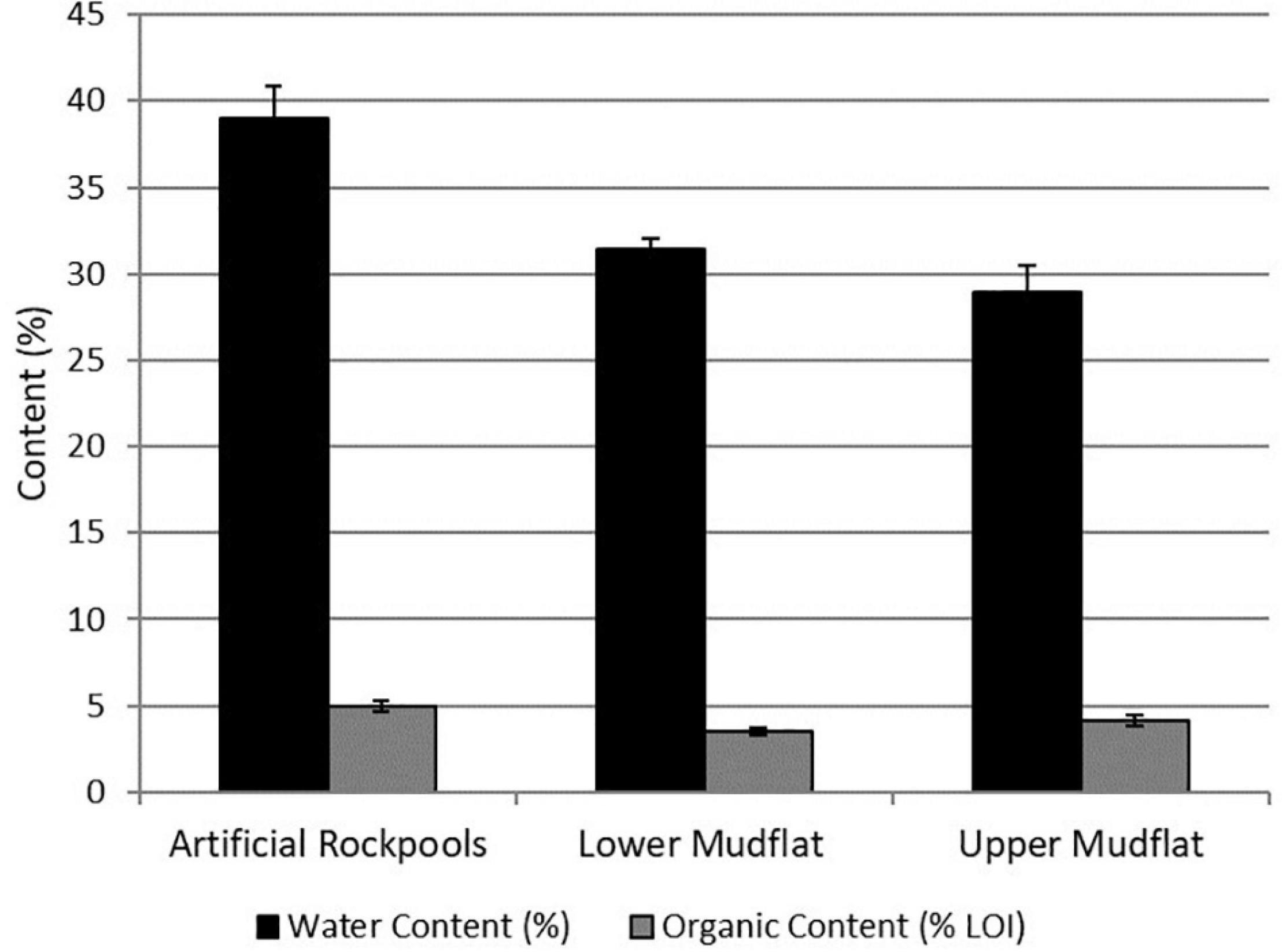
Figure 3. Mean water and organic content in the soft-bottomed habitats expressed as percentage. Error bars show ± Standard Error (n = 9).
Faunal Analysis
A total of thirteen taxa were identified across all habitats, with the gastropod snail Peringia ulvae (Pennant, 1777) numerically abundant in all habitats. The presence of other taxa was variable depending on the habitat (Table 2). No non-native species were identified in the sediment. However, the non-native barnacle Austrominius modestus (Darwin, 1854) was identified on the rockpool exterior and was also present on the sheet piling.
The artificial rockpool habitat contained eleven taxa, the highest for all habitats, with three taxa found only in the artificial rockpool sediment in very low abundances: Actiniidea indet., Cirratulidae sp. and Corophium volutator (Pallas, 1766). Annelid worms were the most abundant taxonomic group. Mean species richness was five (Figure 4) and ranged between four and seven. Specimens of the bivalve Scrobicularia plana (Da Costa, 1778), found in all habitats, were all juveniles (< 15 mm shell length). The lower mudflat habitat contained seven taxa with one species found only within this habitat: the polychaete Capitella capitata (Fabricius, 1780). Species richness ranged from five to seven species in samples and the lower mudflat had the highest mean species richness of all habitats with 6.4 species (Figure 4). The upper mudflat habitat contained eight species of which the gastropod P. ulvae was the most numerically abundant. Annelid worms were absent from most sample stations from the upper mudflat. Mean species richness was the lowest of all habitats with 3.2 species (Figure 4).
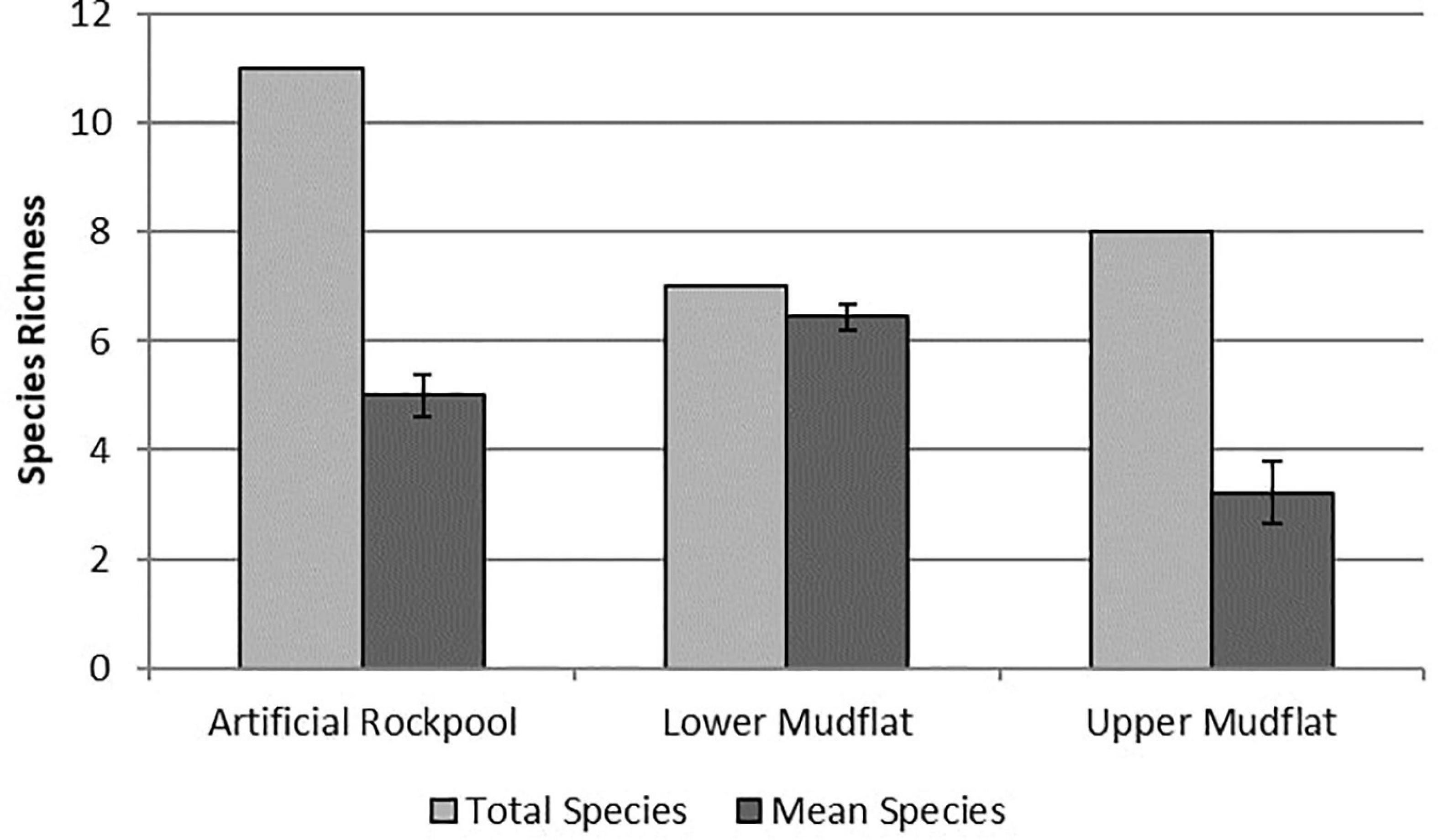
Figure 4. Species richness for soft-bottomed habitats. “Total species” indicates the number of different taxa sampled in a habitat, whereas “mean species” is the average number of species per sample station/rockpool within that habitat (n = 9). Error bars show ± Standard Error.
The one-way ANOVA showed a significant difference between habitats for species richness (df 2,24, F = 14.89, p ≤ 0.001) and abundance (df 2,24, F = 8.08, p ≤ 0.01). Tukey post hoc tests (Supplementary Table 2) revealed significant differences between upper mudflat-lower mudflat (p ≤ 0.001) and upper mudflat-rockpools (p ≤ 0.05) for species richness, and between upper mudflat-lower mudflat (p ≤ 0.01) and rockpools-lower mudflat (p ≤ 0.05) for abundance.
The upper mudflat was least diverse with a mean Shannon Weiner diversity index (H’) of 0.67 (± 0.11 standard error) and showed the greatest range (0 – 0.89). The lower mudflat ranked most diverse (H’ = 1.38 ± 0.06 standard error) and showed the smallest range (1.01–1.54). The artificial rockpools ranked in the middle (H’ = 1.17 ± 0.08 standard error) but their diversity indices were closest to the lower mudflat. A Welch’s ANOVA demonstrated significant difference between the diversity indices of the different habitats (F = 11.207, p ≤ 0.01) and a Games Howell post hoc test revealed significant differences between upper mudflat-lower mudflat (p ≤ 0.01) and upper mudflat-rockpools (p ≤ 0.01).
The lower mudflat sediment contained the greatest total biomass (159 g m–2), with a mean biomass of 18 g m–2, dominated by H. diversicolor (Figure 5). Artificial rockpool sediment closely followed with a total and mean biomass of 134 g m–2 and 15 g m–2, respectively, dominated again by H. diversicolor. Upper mudflat sediment contained the least total and mean biomass with 55 g m–2 and 6 g m–2, respectively, dominated by gastropods. In samples where ragworms were present, biomass was likely to be underestimated due to 38% of H. diversicolor specimens appearing incomplete. Hence, the biomass results should be interpreted with caution. One-way ANOVAs revealed there was no significant difference (df 2,24, F = 2.36, p ≥ 0.116) between the total biomass of the different habitats. The lower mudflat and artificial rockpool mud assemblages were most similar to the littoral sediment biotope “H. diversicolor and Streblospio shrubsolii (Buchanan, 1890) in littoral sandy mud” (LS.LMu.UEst.Hed.Str) (Connor et al., 2004).
The H. diversicolor size class with the highest proportion for the artificial rockpools was 11–20 mm (Figure 6). The lower mudflat had a greater proportion of the smallest size class (1–10 mm) than the artificial rockpools, but both were predominantly comprised of H. diversicolor between 1 and 30 mm. Artificial rockpools had a slightly higher proportion of the larger size classes than the lower mudflat. The upper mudflat had very low abundances of H. diversicolor and their sizes did not exceed 30 mm.
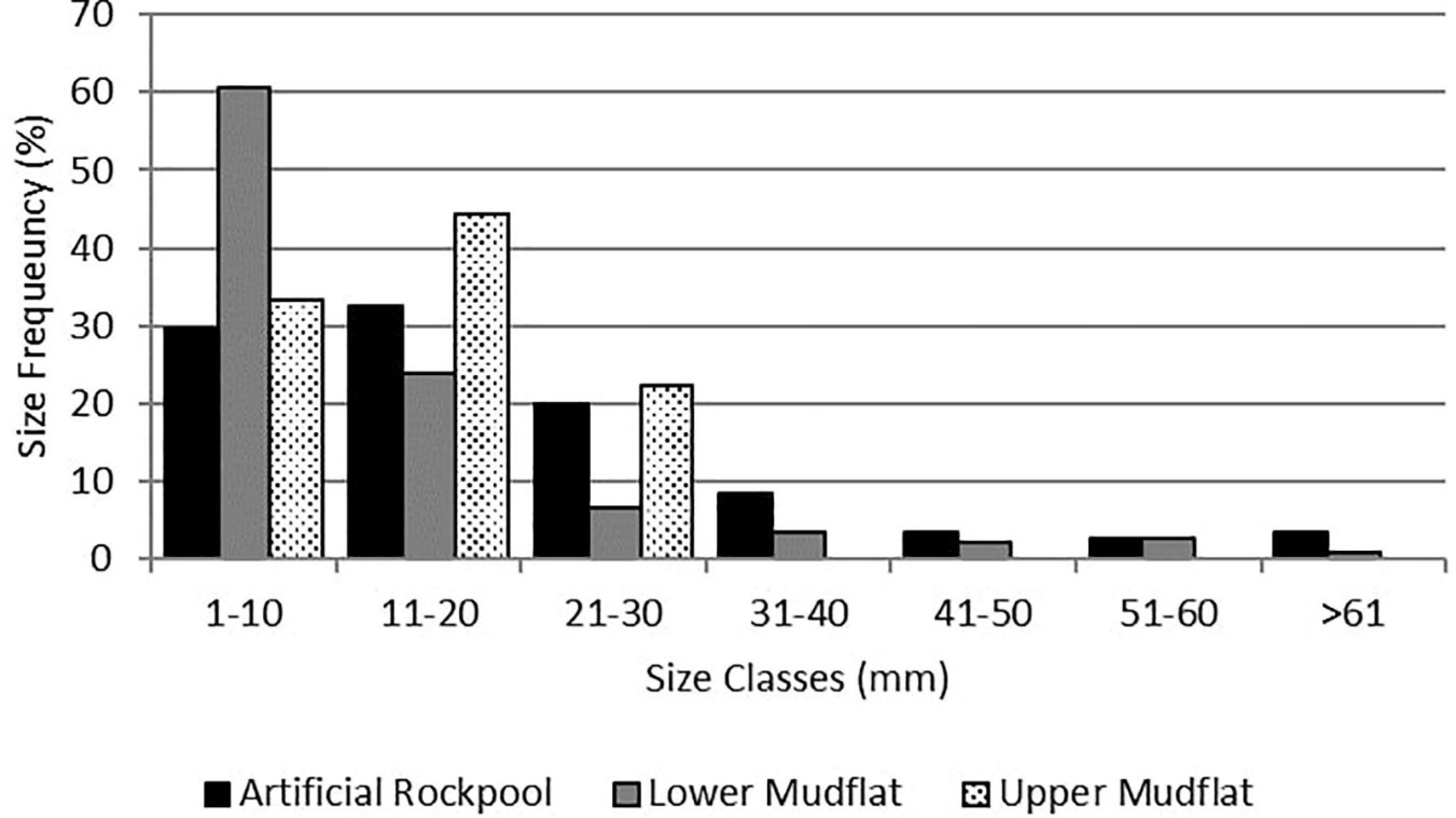
Figure 6. Size frequency (total length) of the ragworm Hediste diversicolor (Müller, 1776) in the artificial rockpools (n = 153), lower mudflat (n = 164), and upper mudflat (n = 10).
PERMANOVA pairwise tests (number of permutations = 9999) revealed significant differences in species abundance data between all habitats (p ≤ 0.001) (Table 3).
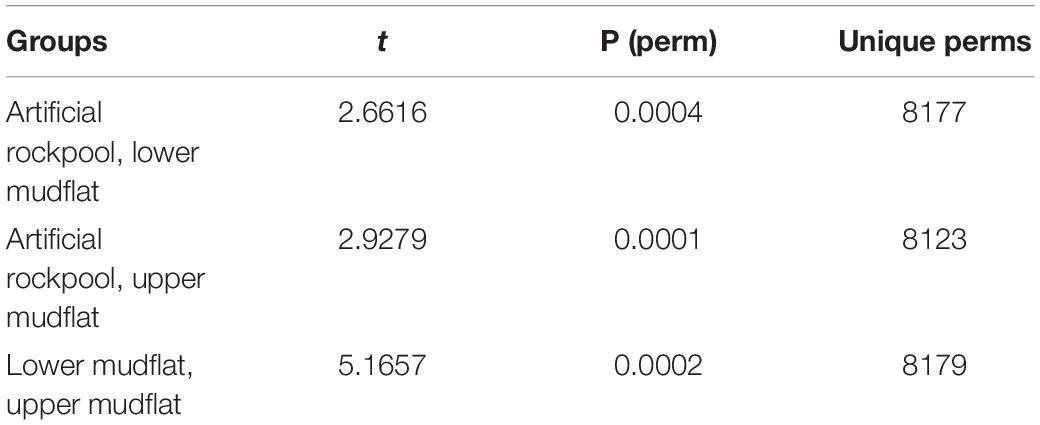
Table 3. Pairwise tests for PERMANOVA between soft-bottomed habitats on species abundance data (Number of permutations = 9999).
Multidimensional scaling plots of the soft-bottomed habitat species abundance data showed the habitat plots as relatively discrete groupings with little overlap (Figure 7). The lower mudflats plots are tightly clustered, which was reflected by a low dispersion index (0.568). The artificial rockpool and upper mudflat plots, however, were more dispersed (dispersion indices are 1.281 and 1.151, respectively); indicating within-habitat artificial rockpool and upper mudflat samples were not as similar as within-habitat samples of the lower mudflat. This was reflected by the pairwise PERMANOVA, which showed that the average similarity within the habitats was highest for the lower mudflat (77.4) and upper mudflat (60.0) and lowest for the artificial rockpool (56.0). The MDS artificial rockpool plots sat between both the mudflat habitats but were plotted slightly closer to the lower mudflat plots, indicating a greater similarity than with the upper mudflat plots. Similarity between artificial rockpools and lower mudflat was reflected by the pairwise test, which indicated average similarity of 54.6 between artificial rockpool and lower mudflat assemblages and 40.7 between artificial rockpool and upper mudflat assemblages.
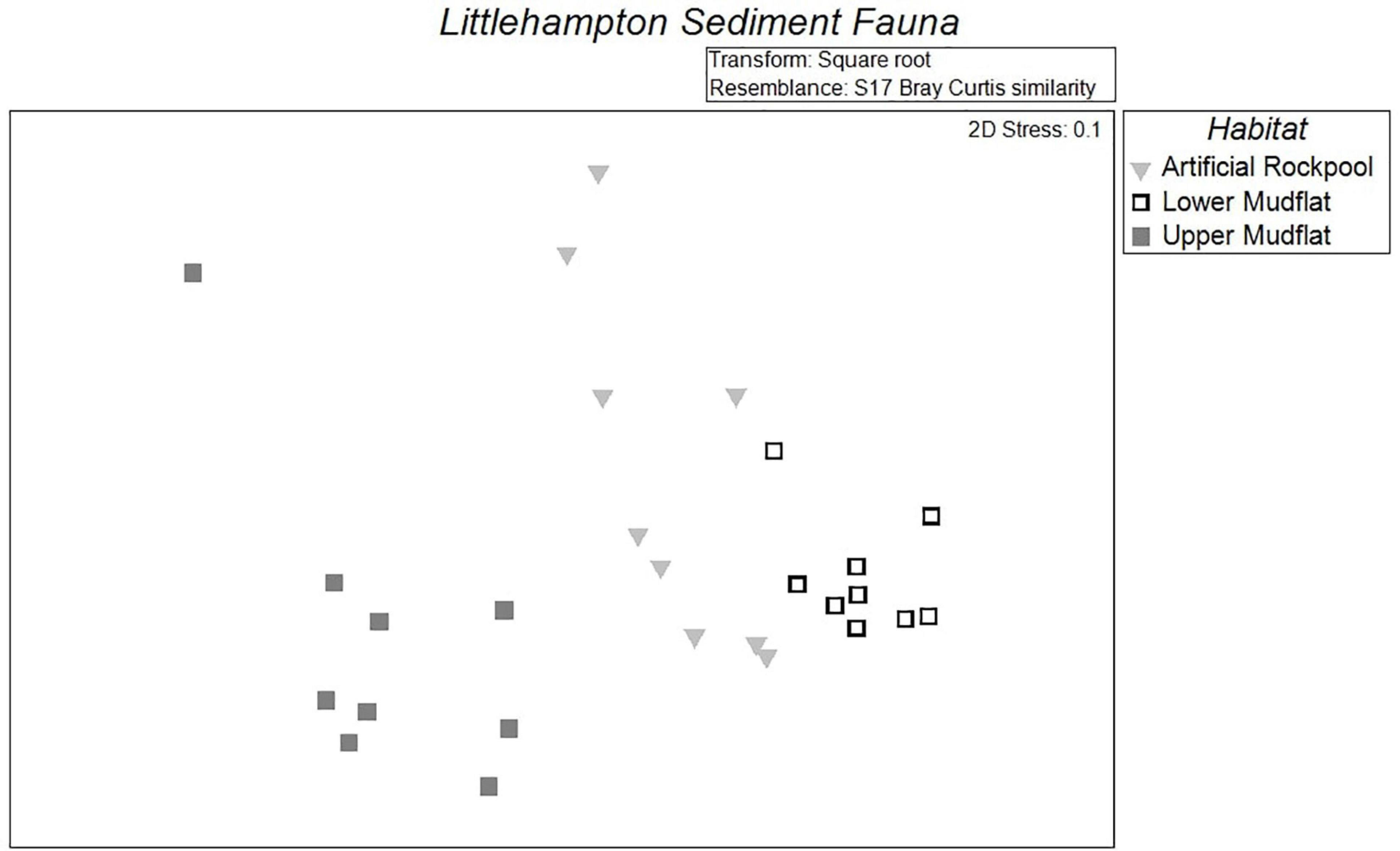
Figure 7. Multidimensional scaling (MDS) plots demonstrating the similarities of assemblages in the different soft-bottom habitats: artificial rockpool, lower mudflat, and upper mudflat (n = 9).
A SIMPER analysis of the taxa which contributed most to the differences between habitat assemblages revealed that annelid worms consistently played key roles in all comparisons (Supplementary Table 1). The polychaete S. shrubsolli contributed the most with 30.8% and 30.5% of dissimilarity between artificial rockpools and lower mudflat, and between lower mudflat and upper mudflat, respectively. Between the artificial rockpool and upper mudflat habitat, the ragworm H. diversicolor and the gastropod P. ulvae contributed 25.4% and 22.4% to dissimilarity, respectively. All other taxa between habitats contributed less than 20%.
Discussion
Although small-scale, to the best of the authors of this present work’s knowledge, this is the first study to demonstrate that estuarine mud retained within artificial rockpools can provide adequate habitat for benthic fauna, comparable to adjacent natural soft-sediment mudflats. Many infaunal species can be found at depths exceeding the depth sampled in this study (5 cm) (Hines and Comtois, 1985; Meadows et al., 1990; Cardoso et al., 2010), which was constrained by the maximum depth of the rockpools. Burrowing behaviour may also depend on the season and size of individuals, for example, larger ragworms may burrow to a depth more than 25 cm in winter, particularly in sandier sediments (Esselink and Zwarts, 1989). Temporal variation in soft-sediment assemblages is well documented (Garcia-Arberas and Rallo, 2002; Ysebaert and Herman, 2002; Magni et al., 2006; Knott et al., 2018) therefore sampling over multiple depth, seasons and years would have been beneficial. However, this would cause significant disturbance to developing habitats within the artificial rockpools.
The limited volume of sediment retained in the artificial rockpools of this study may modify predator-prey or competitive relationships, as well as limiting fitness and growth (Strain et al., 2017). However, if scaled-up, enhancements specially designed to retain estuarine sediment may offer considerable potential to provide habitat and prevent biodiversity loss within heavily modified waterbodies. Although the soft-bottom infauna assemblages of the artificial rockpools differed to the local mudflats in species richness, abundance, diversity indices and assemblage structure, considering the patchy, variable nature of benthic assemblages, the rockpools nevertheless created a relatively analogous habitat to the top few centimetres of natural mudflat habitat. Considering the depth sampled, the faunal assemblages of the artificial rockpools were fairly typical of disturbed, organically enriched estuarine mudflat assemblages recorded in European estuaries and elsewhere (van der Linden et al., 2012). Although sediment characteristics (median grain size and organic matter content) were statistically significantly different between the rockpools and mudflats, the magnitude of this difference was likely to be relatively inconsequential. All habitats were silt-based (Wentworth, 1922), and organic matter ranged across all habitats by ∼1.5%, remaining within organic matter values expected within enriched, estuarine mud (Hossain et al., 2014; Perkins et al., 2014; Coblentz et al., 2015).
The artificial rockpool assemblage structure and Shannon Weiner diversity index were more similar to that of the lower mudflat than the upper mudflat, despite the artificial rockpools being at similar shore heights to the area sampled at the upper mudflat. Additionally, the Shannon Weiner diversity index suggests that the artificial rockpool habitats were more diverse than the upper mudflats. Although their position in the tidal range was higher than the lower mudflats, the artificial rockpool mud drainage was restricted and so the mud appeared to remain more saturated at low tide. Reduced desiccation stress may enhance survival of intertidal organisms, which may be particularly pertinent with rising air temperatures (IPCC, 2014; Maggi et al., 2016).
The assemblage structure within the artificial rockpools appeared to be more variable than the lower mudflat, as indicated by the PERMANOVA similarity values and MDS plots. This was likely due to the fact that they were discrete habitat units, as opposed to being a contiguous habitat like the mudflats, which may also augment the effects of shore height on what is able to colonise the artificial rockpool mud. Tidal height differences between the rockpools may also have contributed to this variability though was not tested due to low replication. The higher number of taxa that were present in the artificial rockpools may be because each artificial rockpool was colonised independently of each other, and by different species. Subsequently, this made the comparison of species diversity between the artificial rockpools and mudflats challenging as they operated at different spatial scales with each artificial rockpool representing a discrete “island” and the mudflats representing a large, contiguous area (Rosenzweig, 1995).
High abundances of small H. diversicolor individuals in the artificial rockpools suggested recent recruitment. The mechanism by which the artificial rockpool mud was colonised by infaunal species is unknown but may occur through adult organisms migrating from the mudflats, larval settlement, or through the incidental deposition of individuals that have been dislodged from bedload transport. Aberson et al. (2011) determined that H. diversicolor can disperse via swimming into raised sediment, though this tends to occur in ragworms < 6 cm in length. It is possible that the steel sheet piling creates a favourable depositional environment within the in-pans, as greater sediment accretion was observed to have occurred at the piling toe within the in-pans. However, this is dependent on a multitude of interacting environmental conditions and will not occur everywhere all the time, as scour at the toe of piling structures is also well documented (Heery et al., 2017).
In a future where sea level rise meets increasingly developed coastlines, coastal squeeze limits the intertidal zone to a narrow vertical area on ACSs, leading to a net loss of intertidal soft-bottomed habitat (Doody, 2004; Schleupner, 2008; Pontee, 2011). As evidenced in this study, it is possible to provide sediment-retentive features on vertical ACSs that offer intertidal habitat that is relatively analogous to estuarine mudflats, particularly where there is risk of soft-bottomed habitat loss. It was also noted that the exterior of the artificial rockpools were well colonised, with the brown alga Fucus spiralis Linnaeus, 1753 and high abundances of barnacles, compared to comparatively sparse coverage of the same species on the sheet piling, which warrants further investigation. Increased abundance present on retrofitted eco-engineering interventions has been noted elsewhere on the south coast of the United Kingdom (Hall et al., 2019). Therefore, these types of interventions may also provide novel habitat on their exterior, maximising their benefits to enhancing faunal colonisation on ACSs. Concerns have been raised that ACSs have introduced non-local assemblages, such as native rocky shore species and invasives, to an area where they were previously uncommon (Bulleri and Chapman, 2010; Airoldi et al., 2015; Perkins et al., 2015). Sediment-retentive interventions may promote and conserve the colonisation of local soft-bottomed species.
Further Study and Application
This study demonstrates proof of concept that sediment can be retained in vertically elevated eco-engineered features which can provide a relatively analogous mudflat habitat containing an assemblage of infauna that typically occur in temperate estuarine mudflats (van der Linden et al., 2012). Further study would benefit from replication on a larger scale with multiple sampling campaigns to consolidate the results, and to install deeper rockpools (e.g., 10–30 cm) that would enable infaunal colonisation and sampling to greater depths. The exterior/underside of the sediment retaining feature may also be designed to provide habitat for species that prefer hard substrata, maximising the value of a sediment-retaining eco-engineering intervention (Hall et al., 2019). Additionally, there may be opportunity to trial the efficacy of other soft-bottomed habitats, such as elevated seagrass, saltmarsh, or reed beds by seeding retained mud with young plants, particularly in locations where these habitats are experiencing loss. Further study should also include close collaboration with coastal engineers and asset owners to determine both long-term and large-scale feasibility of adding these interventions to sheet piling, and how they may affect corrosion rates and loading stress. This collaboration is essential to the success of eco-engineering schemes and eventual incorporation of such interventions in planning and policy (Naylor et al., 2012; Perkins et al., 2015). At this pilot stage, it is unclear what additional benefits artificial mudflat habitats may provide; for example, food provision for birds and fish, or use for commercial hand-gathering of ragworms as bait (Scaps, 2002).
The vertical extension of soft-bottomed habitats via eco-engineering may assist with meeting the enhancement requirements that are increasingly required of new construction. However, the incorporation of sediment-retaining interventions should not be used to justify new-build coastal structures when alternatives, such as soft-engineering, haven’t already been considered, and further pilot testing is required (Firth et al., 2020). It should be noted that Littlehampton harbour, like many recreational marinas (Heery et al., 2017), is exceptionally sheltered and sediment retention is unlikely to occur on medium to high exposure shores, and therefore sediment retaining interventions will not be appropriate for every proposed site. Prior to adding such features on an existing coastal structure or integrating them into planning, it would be prudent to determine local sediment budgets and the depositional environment of the proposed site. For example, Waltham and Sheaves (2018) recorded mean yearly net sediment accumulation of between 17.5 mm yr–1 and 22.5 mm yr–1 in eco-engineered rockpools deployed intertidally on the coast of Townsville, Australia. However, in the present study, full siltation of the artificial rockpools was observed after just 1 year (60–70 mm). It is recommended that the local soft-sediment environment should be sampled to provide an indication of the infauna that could be expected to colonise the sediment-retaining features on the structure itself. Any intervention intended for sediment retention would not be appropriate on an ACS that is expected to facilitate soft-sediment erosion, as it is unlikely they would perform as expected.
Conclusion
The pilot installation of nine artificial rockpools on steel sheet piling have demonstrated they can enhance the structure by retaining soft sediment, that creates a habitat relatively analogous to an estuarine mudflat, albeit very small-scale. These results show that eco-engineering interventions can create both hard substrate and soft-sediment habitats on vertical ACSs, which provides an additional enhancement option for ACSs and the potential to develop design templates for new ACSs that might offer integral habitat-forming features. However, further pilot testing is required to design eco-engineering features specifically designed to retain soft sediments on hard infrastructure in heavily modified water bodies. Collaboration with coastal practitioners would be essential to maximise the efficacy of such schemes.
Data Availability Statement
The raw data supporting the conclusions of this article will be made available by the authors, without undue reservation.
Author Contributions
JB wrote the manuscript. RS, AH, IB, NG, and RH reviewed numerous drafts, provided edits, and improved feedback on the manuscript. All authors contributed to the article and approved the submitted version.
Funding
This work was supported by the MARINEFF project of the Interreg VA France (Channel Manche) England programme, co-funded by the European Regional Development Fund.
Conflict of Interest
IB and NG are employed by Artecology Ltd.
The remaining authors declare that the research was conducted in the absence of any commercial or financial relationships that could be construed as a potential conflict of interest.
Publisher’s Note
All claims expressed in this article are solely those of the authors and do not necessarily represent those of their affiliated organizations, or those of the publisher, the editors and the reviewers. Any product that may be evaluated in this article, or claim that may be made by its manufacturer, is not guaranteed or endorsed by the publisher.
Acknowledgments
We are grateful to the Environment Agency (capital schemes SSD PCM Peter Borsberry/Oliver Sykes and National Environmental and Sustainability team Tania White) for having commissioned and installed the artificial rockpools and providing feedback on the manuscript (Emma Swan, Paula Goodeve, Peter Borseberry, Richard Charman, Tania White, and Mike Best); Environment Agency Estuary Edges team; Littlehampton Harbour Authority and Mackley, as part of Team van Oord, for installation; assistance of the Littlehampton Harbourmaster team for providing us with access and assistance for fieldwork; contributions of co-authors to this manuscript; and reviewers whose feedback has improved subsequent drafts.
Supplementary Material
The Supplementary Material for this article can be found online at: https://www.frontiersin.org/articles/10.3389/fmars.2021.780720/full#supplementary-material
References
Aberson, M. J. R., Bolam, S. G., and Hughes, R. G. (2011). The dispersal and colonisation behaviour of the marine polychaete Nereis diversicolor (O.F. Muller) in south-east England. Hydrobiologia 672, 3–14. doi: 10.1007/s10750-011-0752-y
Aguilera, M. A., Broitman, B. R., and Thiel, M. (2014). Spatial variability in community composition on a granite breakwater versus natural rocky shores: lack of microhabitats suppresses intertidal biodiversity. Mar. Pollut. Bull. 87, 257–268. doi: 10.1016/j.marpolbul.2014.07.046
Airoldi, L., Abbiati, M., Beck, M. W., Hawkins, S. J., Jonsson, P. R., Martin, D., et al. (2005). An ecological perspective on the deployment and design of low-crested and other hard coastal defence structures. Coast. Eng. 52, 1073–1087. doi: 10.1016/j.coastaleng.2005.09.007
Airoldi, L., Turon, X., Perkol-Finkel, S., and Rius, M. (2015). Corridors for aliens but not for natives: effects of marine urban sprawl at a region scale. Divers Distrib. 21, 755–768. doi: 10.1111/ddi.12301
Anderson, M. J. (2005). Permanova Permutational multivariate analysis of variance, a computer program. New Zealand: University of Auckland.
Barbier, E. B., Hacker, S. D., Kennedy, C., Koch, E. W., Stier, A. C., and Silliman, B. R. (2011). The value of estuarine and coastal ecosystem services. Ecol. Monogr. 81, 169–193. doi: 10.1890/10-1510.1
Beal, B. F., Coffin, C. R., Randall, S. F., Goodenow, C. A., Peppreman, K. E., Ellis, B. W., et al. (2018). Spatial variability in recruitment of an infaunal bivalve: experimental effects of predator exclusion on the soft-shell clam (Mya arenaria L.) along three tidal estuaries in southern Maine. USA J. Shellf. Res. 37, 1–27. doi: 10.2983/035.037.0101
Bergen, S. D., Bolton, S., and Fridley, J. (2001). Design principles for ecological engineering. Ecol. Eng. 18, 201–210. doi: 10.1016/S0925-8574(01)00078-7
Browne, M. A., and Chapman, M. G. (2014). Mitigating against the loss of species by adding artificial intertidal pools to existing seawalls. Mar. Ecol. Prog. Ser. 497, 119–129. doi: 10.3354/meps10596
Buchanan, F. (1890). Hekaterobranchus shrubsolii, a new genus and species of the family Spionidae. Q. J. Microsc. Sci. Lond. New Ser. 122(XXXI, Part 2), 175–200, lates XXI–XXII.
Bugnot, A. B., Mayer-Pinto, M., Airoldi, L., Heery, E. C., Johnston, E. L., Critchley, L. P., et al. (2021). Current and projected global extent of marine built structures. Nat 4, 33–41. doi: 10.1038/s41893-020-00595-1
Bulleri, F., and Chapman, M. G. (2010). The introduction of coastal infrastructure as a driver of change in marine environments. J. Appl. Ecol. 47, 26–35. doi: 10.1111/j.1365-2664.2009.01751.x
Cardoso, I., Granadeiro, J. P., and Cabral, H. (2010). Benthic macroinvertebrates’ vertical distribution in the Tagus estuary (Portugal): the influence of tidal cycle. Estuar. Coast. Shelf. Sci. 86, 580–586. doi: 10.1016/j.ecss.2009.11.024
Chapman, M. G. (2003). Paucity of mobile species on constructed seawalls: effects of urbanisation on biodiversity. Mar. Ecol. Prog. Ser. 264, 21–29. doi: 10.3354/meps264021
Chapman, M. G., and Underwood, A. J. (2011). Evaluation of ecological engineering of “armoured” shorelines to improve their value as habitat. J. Exp. Mar. Biol. Ecol. 400, 302–313. doi: 10.1016/j.jembe.2011.02.025
Chee, S. Y., Wee, J. L. S., Wong, C., Yee, J. C., Yusup, Y., and Mujahid, A. (2020). Drill-cored artificial rock pools can promote biodiversity and enhance community structure on coastal rock revetments at reclaimed coastlines of Penang. Malaysia Trop. Conserv. Sci. 13, 1–15. doi: 10.1177/1940082920951912
Clarke, K. R., and Gorley, R. N. (2006). PRIMER v6: User Manual/Tutorial (Plymouth Routines in Multivariate Ecological Research). Plymouth: PRIMER-E.
Coblentz, K. E., Henkel, J. R., Sigel, B. J., and Taylor, C. M. (2014). Technical note: the use of laser diffraction particle size analyzers for inference on infauna-sediment relationships. Estuar. Coast. 38, 699–702. doi: 10.1007/s12237-014-9837-y
Coblentz, K. E., Henkel, J. R., Sigel, B. J., and Taylor, C. M. (2015). Influence of sediment characteristics on the composition of soft-sediment intertidal communities in the northern Gulf of Mexico. PeerJ 3:e1014. doi: 10.7717/peerj.1014
Connell, S. D., and Glasby, T. M. (1999). Do urban structures influence local abundance and diversity of subtidal epibiota? a case study from Sydney Harbour, Australia. Mar. Environ. Res. 47, 373–387. doi: 10.1016/S0141-1136(98)00126-3
Connor, D. W., Allen, J. H., Golding, N., Howell, K. L., Lieberknecht, L. M., Northen, K. O., et al. (2004). The Marine Habitat Classification for Britain and Ireland Version 04.05 in The Marine Habitat Classification for Britain and Ireland Version 15.03. Joint Nature Conservation Committee. Available online at: https://mhc.jncc.gov.uk/resources#version0405.
Cook, P. L. M., Revill, A. T., Butler, E. C. V., and Eyre, B. D. (2004a). Carbon and nitrogen cycling on intertidal mudflats of a temperate Australian estuary. II. Nitrogen cycling. Mar. Ecol. Prog. Ser. 280, 39–54. doi: 10.3354/meps280039
Cook, P. L. M., Revill, A. T., Clemenston, L. A., and Volkman, J. K. (2004b). Carbon and nitrogen cycling on intertidal mudflats of a temperate Australian estuary. III. Sources of organic matter. Mar. Ecol. Prog. Ser. 280, 55–72. doi: 10.3354/meps280055
Coombes, M. A., Claudia La Marca, E., Naylor, L. A., and Thompson, R. C. (2015). Getting into the groove: opportunities to enhance the ecological value of hard coastal infrastructure using fine-scale surface textures. Ecol. Eng. 77, 314–323. doi: 10.1016/j.ecoleng.2015.01.032
Costanza, R., de Groot, R., Sutton, P., van der Ploeg, S., Anderson, S. J., Kubiszewski, I., et al. (2014). Changes in the global value of ecosystem services. Glob. Environ. Change 26, 152–158. doi: 10.1016/j.gloenvcha.2014.04.002
Critchley, L. P., and Bishop, M. J. (2019). Differences in soft-sediment infaunal communities between shorelines with and without seawalls. Estuar. Coast. 42, 1127–1137. doi: 10.1007/s12237-019-00527-z
Da Costa, M. E. (1778). Historia Naturalis Testaceorum Britanniæ, or, The British Conchology: Containing the Descriptions and Other Particulars of Natural History of the Shells of Great Britain and Ireland: Illustrated with Figures, in English and in French. London: Millan, White, Emsley & Robson, i–xii, 1–254, i–vii, I–XVII.
Darwin, C. (1854). A Monograph on the Sub-Class Cirripedia with Figures of All the Species. The Balanidae, (or Sessile Cirripedia); the Verricidae, etc., etc., etc. London: The Ray Society, i–viii+1–684, ls.1–30.
Davey, J. T., and Watson, P. G. (1995). The activity of Nereis diversicolor (Polychaeta) and its impact on nutrient fluxes in estuarine waters. Ophelia 41, 55–70. doi: 10.1080/00785236.1995.10422037
Dethier, M. N., Ruesink, J., Berry, H., and Sprenger, A. G. (2012). Decoupling of recruitment from adult clam assemblages along an estuarine shoreline. J. Exp. Mar. Biol. Ecol. 42, 48–54. doi: 10.1016/j.jembe.2012.04.009
Dissanayake, N. G., Frid, C. L. J., Drylie, T. P., and Caswell, B. A. (2018). Ecological functioning of mudflats: global analysis reveals both regional differences and widespread conservation of functioning. Mar. Ecol. Prog. Ser. 604, 1–20. doi: 10.3354/meps12728
Doody, P. J. (2004). Coastal squeeze – an historical perspective. J. Coast. Conserv. 10, 129–138. doi: 10.1006/ecss.1998.0417
Dugan, J. E., Emery, K. A., Alber, M., Alexander, C. R., Byers, J. E., Gehman, A. M., et al. (2018). Generalizing ecological effects of shoreline armoring across soft sediment environments. Estuar. Coast. 41:254. doi: 10.1007/s12237-017-0254-x
Durou, C., Mouneyrac, C., and Amirad-Triquest, C. (2007). Environmental quality assessment in estuarine ecosystems: use of biometric measurements and fecundity of the ragworm Nereis diversicolor (Polychaeta, Nereididae). Water Res. 42, 2157–2165. doi: 10.1016/j.watres.2007.11.028
Ellingsen, K. A. (2002). Soft-sediment benthic biodiversity on the continental shelf in relation to environmental variability. Mar. Ecol. Prog. Ser. 232, 15–27. doi: 10.3354/meps232015
Esselink, P., and Zwarts, L. (1989). Seasonal trend in burrow depth and tidal variation in feeding activity of Nereis diversicolor. Mar. Ecol. Prog. Ser. 56, 243–254.
Fabricius, O. (1780). Fauna Groenlandica, Systematice Sistens Animalia Groenlandiae Occidentalis Hactenus Indagata, Quoad Nomen Specificum, Triviale, Vernaculumque; Synonyma Auctorum Plurium, Descriptionem, Locum, Victum, Generationem, Mores, Usum, Capturamque Singuli, Prout Detegendi Occasio Fuit, Maximaque Parte Secundum Proprias Observationes. Lipsiae: Ioannis Gottlob Rothe, xvi+452.
Firth, L. B., Airoldi, L., Bulleri, F., Challinor, S., Chee, S. Y., Evans, A. J., et al. (2020). Greening of grey infrastructure should not be used as a Trojan horse to facilitate coastal development. J. Appl. Ecol. 2020, 1–7. doi: 10.1111/1365-2664.13683
Firth, L. B., Knights, A. M., Bridger, D., Evans, A. J., Mieszkowska, N., Moore, P., et al. (2016). “Ocean Sprawl: Challenges and Opportunities for Biodiversity Management in a Changing World,” in Oceanography and Marine Biology: An Annual Review, eds R. N. Hughes, D. J. Hughes, I. P. Smith, and A. C. Dale (Oxford: Taylor and Francis).
Firth, L. B., Schofield, M., White, F. J., Skov, M. W., and Hawkins, S. J. (2014). Biodiversity in intertidal rock pools: informing engineering criteria for artificial habitat enhancement in the built environment. Mar. Environ. Res. 102, 122–130. doi: 10.1016/j.marenvres.2014.03.016
Fox, J., and Weisberg, S. (2019). An R Companion to Applied Regression, Third edition. Thousand Oaks, CA: Sage.
Garcia-Arberas, L., and Rallo, A. (2002). Life cycle, demography and secondary production of the polychaete Hediste diversicolor in a non-polluted estuary in the Bay of Biscay. Mar. Ecol. 23, 237–251. doi: 10.1046/j.1439-0485.2002.02766.x
Glasby, T. M., Connell, S. D., Holloway, M. G., and Hewitt, C. L. (2007). Nonindigenous biota on artificial structures: could habitat creation facilitate biological invasions? Mar. Biol. 151, 887–895. doi: 10.1007/s00227-006-0552-5
Gunnarsson, J. S., Hollertz, K., and Rosenberg, R. (1999). Effects of organic enrichment and burrowing activity of the polychaete Nereis diversicolor on the fate of tetrachlorobiphenyl in marine sediments. Environ. Toxicol. Chem. 18, 1149–1156.
Hall, A. E., Herbert, R. J. H., Britton, J. R., Boyd, I. M., and George, N. C. (2019). Shelving the coast with Vertipools: retrofitting artificial rock pools on coastal structures as mitigation for coastal squeeze. Front. Mar. Sci. 6:456. doi: 10.3389/fmars.2019.00456
Hall, A. E., Herbert, R. J. H., Britton, J. R., and Hull, S. L. (2018). Ecological enhancement techniques to improve habitat heterogeneity on coastal defence structures. Estuar. Coast. Shelf. Sci. 210, 68–78. doi: 10.1016/j.ecss.2018.05.025
Hawkins, S. J. (2012). Marine conservation in a rapidly changing world. Aquat. Conserv. 22, 281–287. doi: 10.1002/aqc.2239
Hawkins, S. J., Evans, A. J., Firth, L. B., Jenner, M. J., Herbert, R. J. H., Adams, L. C., et al. (2016). “Impacts and effects of oceanwarming on intertidal rock habitats. Full report,” in Explaining Ocean Warming: Causes, Scale, Effects and Consequences, eds D. Laffoley and J. M. Baxter (Switzerland: IUCN).
Heery, E. C., Bishop, M. J., Critchley, L. P., Bugnot, A. B., Airoldi, L., Mayer-Pinto, M., et al. (2017). Identifying the consequences of ocean sprawl for sedimentary habitats. J. Exp. Mar. Biol. Ecol. 492, 31–48. doi: 10.1016/j.jembe.2017.01.020
Hines, A. H., and Comtois, K. L. (1985). Vertical distribution of infauna in sediments of a subestuary of central Chesapeake Bay. Estuaries 8:1351490. doi: 10.2307/1351490
Hossain, M. B., Marshall, D. J., and Venkatramanan, S. (2014). Sediment granulometry and organic matter content in the intertidal zone of the Sungai Brunei estuarine system, northwest coast of Borneo. Carp. J. Earth Environ. 9, 231–239.
Hunt, H. L., and Scheibling, R. E. (1997). Role of early post-settlement mortality in recruitment of benthic marine invertebrates. Mar. Ecol. Prog. Ser. 155, 269–301.
IPCC (2014). “Core Writing Team,” in Climate Change 2014: Synthesis Report. Contribution of Working Groups I, II and III to the Fifth Assessment Report of the Intergovernmental Panel on Climate Change, eds R. K. Pachauri and L. A. Meyer (Geneva: IPCC).
Jordan, T. E., Weller, D. E., and Pelc, C. E. (2018). Effects of local watershed land use on water quality in Mid-Atlantic coastal bays and subestuaries of the Chesapeake Bay. Estuar. Coast. 41, 38–53. doi: 10.1007/s12237-017-0303-5
Knott, K. E., Thonig, A., Heiskanen, S., Winding Hansen, B., and Banta, G. T. (2018). Seasonal variation in diversity of marine benthic invertebrates leads to a positive species-genetic diversity correlation (SGDC). Mar. Ecol. Prog. Ser. 592, 129–140. doi: 10.3354/meps12520
Linnaeus, C. (1753). Species Plantarum, Exhibentes Plantas Rite Cognitas ad Genera Relatas cum Differentiis Specificis, Nominibus Trivialibus, Synonymis Selectis, Locis Natalibus, Secundum Systema Sexuale Digestas. Stockholm: Impensis Laurentii Salvii.
Linnaeus, C. (1758). Systema Naturae per Regna Tria Naturae, Secundum Classes, Ordines, Genera, Species, cum Characteribus, Differentiis, Synonymis, Locis. Editio Decima, Reformata [10th revised edition], Vol. 1. Holmiae: Laurentius Salvius, 824.
Loke, L. H. L., Bouma, T. J., and Todd, P. A. (2017). The effects of manipulating microhabitat size and variability on tropical seawall biodiversity: field and flume experiments. J. Exp. Mar. Biol. Ecol. 492, 113–120. doi: 10.1016/j.jembe.2017.01.024
Luczak, C., Janquin, M. A., and Kupka, A. (1997). Simple standard procedure for the routine determination of organic matter in marine sediment. Hydrobiologia 345, 87–94.
MacArthur, M., Naylor, L. A., Hansom, J. D., Burrows, M. T., Loke, L. H. L., and Boyd, I. (2019). Maximising the ecological value of hard coastal structures using textured formliners. Ecol. Eng. X 1:2. doi: 10.1016/j.ecoena.2019.100002
Maggi, E., Cappiello, M., Del Corso, A., Lenzarini, F., Peroni, E., and Benedetti-Cecchi, L. (2016). Climate-related environmental stress in intertidal grazers: scaling-up biochemical responses to assemblage-level processes. PeerJ 4:e2533. doi: 10.7717/peerj.2533
Magni, P., Como, S., Montani, S., and Tsutsumi, H. (2006). Interlinked temporal changes in environmental conditions, chemical characteristics of sediments and macrofaunal assemblages in an estuarine intertidal sandflat (Seto Inland Sea, Japan). Mar. Bio. 149, 1185–1197. doi: 10.1007/s00227-006-0298-0
Martin, D., Bertasi, F., Colangelo, M. A., de Vries, M., Frost, M., Hawkins, S. J., et al. (2005). Ecological impact of coastal defence structures on sediment and mobile fauna: evaluating and forecasting consequences of unavoidable modifications of native habitats. Coast. Eng. 52, 1027–1051. doi: 10.1016/j.coastaleng.2005.09.006
Martins, G. M., Jenkins, S. R., Neto, A. I., Hawkins, S. J., and Thompson, R. C. (2016). Long-term modifications of coastal defences enhance marine biodiversity. Environ. Conserv. 43, 109–116. doi: 10.1017/S0376892915000284
Martins, G. M., Thompson, R. C., Neto, A. I., Hawkins, S. J., and Jenkins, S. R. (2010). Enhancing stocks of the exploited limpet Patella candei d’Orbigny via modifications in coastal engineering. Biol. Conserv. 143, 203–211. doi: 10.1016/j.biocon.2009.10.004
McKinney, M. L. (2006). Urbanisation as a major cause of biotic homogenization. Biol. Conserv. 127, 247–260. doi: 10.1016/j.biocon.2005.09.005
McLusky, D. S., and Elliott, M. (2004). The Estuarine Ecosystem, Ecology, Threats and Management. Oxford: Oxford University Press.
Meadows, P. S., Tait, J., and Hussain, S. A. (1990). Effects of estuarine infauna on sediment stability and particle sedimentation. Hydrobiologia 190, 263–266. doi: 10.1007/BF00008194
Miller, R. G., Hutchinson, Z. L., Macleod, A. K., Burrows, M. T., Cook, E. J., Last, K. S., et al. (2013). Marine renewable energy development: assessing the Benthic Footprint at multiple scales. Front. Ecol. Environ. 11:433–440. doi: 10.1890/120089
Mitsch, W. J., and Jorgensen, S. E. (2003). Ecological engineering: a field whose time has come. Ecol. Eng. 20, 363–377. doi: 10.1016/j.ecoleng.2003.05.001
Moschella, P. S., Abbiati, M., Aberg, P., Airoldi, L., Anderson, J. M., Bacchiocchi, F., et al. (2005). Low-crested coastal defence structures as artificial habitats for marine life: using ecological criteria in design. Coast. Eng. 52, 1053–1071. doi: 10.1016/j.coastaleng.2005.09.014
Müller, O. F. (1776). Zoologiæ Danicæ Prodromus, seu Animalium Daniæ et Norvegiæ Indigenarum Characteres, Nomina, et Synonyma Imprimis Popularium. Havniæ: Hallageri, xxxii+274.
Naylor, L., Coombes, M. A., Venn, O., Roast, S. D., and Thompson, R. C. (2012). Facilitating ecological enhancement of coastal infrastructure: the role of policy, people and planning. Environ. Sci. Policy 22, 36–46. doi: 10.1016/j.envsci.2012.05.002
Odum, H. T., and Odum, B. (2003). Concepts and method of ecological engineering. Ecol. Eng. 20, 339–361. doi: 10.1016/j.ecoleng.2003.08.008
O’Shaughnessy, K. A., Hawkins, S. J., Evans, A. J., Hanley, M. E., Lunt, P., Thompson, R. C., et al. (2019). Design catalogue for eco-engineering of coastal artificial structures: a multifunctional approach for stakeholders and end-users. Urban Ecosyst. 2019:924. doi: 10.1007/s11252-019-00924-z
Pallas, P. S. (1766). Miscellanea Zoologica Quibus Novae Imprimis Atque Obscurae Animalium Species Describuntur et Observationibus Iconibusque Illustrantur. Petrum van Cleef. Hagí Comitum, xii+224;14ls.
Pennant, T. (1777). British Zoology, Crustacea. Mollusca. Testacea, Vol. IV., London: Benjamin White, i–viii,1–154, lates1–93.
Perkins, M. J., Ng, T. P. T., Dudgeon, D., Bonebrake, T. C., and Leung, K. M. Y. (2015). Conserving intertidal habitats: what is the potential of ecological engineering to mitigate impacts of coastal structures? Estuar. Coast. Shelf. Sci. 167, 504–515. doi: 10.1016/j.ecss.2015.10.033
Perkins, T. L., Clements, K., Baas, J. H., Jago, C. F., Jones, D. L., Malham, S. K., et al. (2014). Sediment composition influences spatial variation in the abundance of human pathogen indicator bacteria within an estuarine environment. Plos One 2014:112951. doi: 10.1371/journal.pone.0112951
Peters, G. J. (2016). _userfriendlyscience: Quantitative Analysis Made Accessible_. R package version 0.7.2. https://cran.r-project.org/web/packages/userfriendlyscience/index.html
Pister, B. (2009). Urban marine ecology in southern California: the ability of riprap structures to serve as intertidal rocky habitat. Mar. Biol. 156, 861–873. doi: 10.1007/s00227-009-1130-4
Pontee, N. I. (2011). Reappraising coastal squeeze: a case study from north-west England. Marit. Engin. 164, 127–138. doi: 10.1680/maen.2011.164.3.127
R Core Team (2016). R: A language and environment for statistical computing. R Foundation for Statistical Computing. Vienna: R Core Team.
Rosenzweig, M. L. (1995). Species Diversity in Space and Time. Cambridge: Cambridge University Press.
Scaps, P. (2002). A review of the biology, ecology and potential use of the common ragworm Hediste diversicolor (O.F. Muller) (Annelida: Polychaeta). Hydrobiologia 470, 203–218. doi: 10.1023/A:1015681605656
Schleupner, C. (2008). Evaluation of coastal squeeze and its consequences for the Caribbean island Martinique. Ocean Coast. Manag. 51, 383–390. doi: 10.1016/j.ocecoaman.2008.01.008
Shakeel, A., Safar, Z., Ibanez, M., van Paassen, L., and Chassagne, C. (2020). Flocculation of clay suspensions by anionic and cationic polyelectrolytes: a systematic analysis. Minerals 10:999. doi: 10.3390/min10110999
Strain, E. M. A., Olabarria, C., Mayer-Pinto, M., Cumbo, V., Morris, R. L., Bugnot, A. B., et al. (2017). Eco-engineering urban infrastructure for marine and coastal biodiversity: which interventions have the greatest ecological benefit? J. Appl. Ecol. 55, 426–441. doi: 10.1111/1365-2664.12961
van der Linden, P., Patricio, J., Marchini, A., Cid, N., Neto, J. M., and Marques, J. C. (2012). A biological trait approach to assess the functional composition of subtidal benthic communities in an estuarine ecosystem. Ecol. Indic. 20, 121–133. doi: 10.1016/j.ecolind.2012.02.004
Vaselli, S., Bulleri, F., and Benedetti-Cecchi, L. (2008). Hard coastal-defence structures as habitats for native and exotic rocky-bottom species. Mar. Environ. Res. 66, 395–403. doi: 10.1016/j.marenvres.2008.06.002
Waltham, N. J., and Sheaves, M. (2018). Eco-engineering rock pools to a seawall in a tropical estuary: microhabitat features and fine sediment accumulation. Ecol. Eng. 120, 631–636. doi: 10.1016/j.ecoleng.2018.05.010
Watson, S. C. L., Preston, J., Beaumont, N. J., and Watson, G. J. (2020). Assessing the natural capital value of water quality and climate regulation in temperate marine systems using a EUNIS biotope classification approach. Sci. Total Environ. 744:140688. doi: 10.1016/j.scitotenv.2020.140688
Yilmaz, S. (2019). A new approach for the testing method of coal grindability. Adv. Powder Technol. 30, 1932–1940. doi: 10.1016/j.apt.2019.06.012
Keywords: green infrastructure, ecological enhancement, habitat mitigation, coastal squeeze, benthic fauna, eco-engineering
Citation: Bone JR, Stafford R, Hall AE, Boyd I, George N and Herbert RJH (2022) Estuarine Infauna Within Incidentally Retained Sediment in Artificial Rockpools. Front. Mar. Sci. 8:780720. doi: 10.3389/fmars.2021.780720
Received: 21 September 2021; Accepted: 13 December 2021;
Published: 05 January 2022.
Edited by:
Rochelle Diane Seitz, William & Mary’s Virginia Institute of Marine Science, College of William & Mary, United StatesReviewed by:
Susana M. F. Ferreira, Polytechnic Institute of Leiria, PortugalSu Yin Chee, Universiti Sains Malaysia, Malaysia
Copyright © 2022 Bone, Stafford, Hall, Boyd, George and Herbert. This is an open-access article distributed under the terms of the Creative Commons Attribution License (CC BY). The use, distribution or reproduction in other forums is permitted, provided the original author(s) and the copyright owner(s) are credited and that the original publication in this journal is cited, in accordance with accepted academic practice. No use, distribution or reproduction is permitted which does not comply with these terms.
*Correspondence: Jessica R. Bone, jbone@bournemouth.ac.uk