- 1Key Laboratory of Agro-Forestry Environmental Processes and Ecological Regulation of Hainan Province, Hainan University, Haikou, China
- 2College of Ecology and Environment, Hainan University, Haikou, China
- 3State Key Laboratory of Marine Environmental Science, Xiamen University, Xiamen, China
- 4Institute of Ocean Engineering, Tsinghua Shenzhen International Graduate School, Tsinghua University, Shenzhen, China
- 5Hainan Key Laboratory of Tropical Eco-Circular Agriculture, Environment and Plant Protection Institute, Chinese Academy of Tropical Agricultural Sciences, Haikou, China
- 6Hainan Danzhou Tropical Agro-Ecosystem National Observation and Research Station, Danzhou, China
Microplastics are regarded as ubiquitous pollutants in the ocean and have attracted worldwide concerns. Benzo[a]pyrene (B[a]P), one of typical polycyclic aromatic hydrocarbons (PAHs), is commonly detected in marine environment. Once coexisted, the microplastics and B[a]P may interact with each other and result in combined toxicity to organisms, and these remain to be systematically elucidated. Thus, this study aims to investigate (i) the effects of single micro-sized polystyrene (mPS), polyethylene terephthalate (mPET), and B[a]P on cell growth of Chaetoceros muelleri; and (ii) the interaction of microplastics and B[a]P, and their combined effects on C. muelleri. The results showed that both single microplastics and B[a]P at a high concentration of 150 μg/L inhibited the growth of C. muelleri. For single treatment of microplastics, stronger inhibition effects on microalgae was caused by mPET than that of mPS, with the highest IR of 25.23 and 11.17%, respectively. This may be attributed to the obvious surface roughness of mPET. By comparison, the combined effects of microplastics and B[a]P significantly inhibited the growth of C. muelleri as compared with the single treatment of B[a]P (P < 0.05). Synergistic effect was found in the combination of microplastics with B[a]P at high concentrations of 150 μg/L. Interestingly, the antagonistic effect on C. muelleri was observed in the combined treatment of microplastics and B[a]P at low concentrations of 10 μg/L. In addition, the pollutants reduced the content of photosynthetic pigments in microalgal cells. The SOD and MDA content of microalgae increased in the early stage of exposure to pollutants (e.g., Days 1 and 5), but decreased in the later stage (Day 15) compared with the control group. The decreased superoxide dismutase (SOD) and catalase (CAT) activity in single and composite systems may indicate that the antioxidative enzymatic system of microalgae has been inhibited or destroyed. This study will be helpful to further explore the ecological threats of microplastics and PAHs to the marine ecosystem.
Introduction
Large quantities production (3.59 trillion tons in 2018) of plastics (EuropePlastics, 2019) would result in the accumulation of ubiquitous microplastics (<5 mm) in the environment (Jambeck et al., 2015; Fu et al., 2020). Microplastics are characterized as small size, large surface-to-volume ratio, mostly porous, rough surface, and abundant functional groups, and these features enable them to easily adsorb other contaminants such as polycyclic aromatic hydrocarbons (PAHs), heavy metals, pathogens, and others (Wright et al., 2013; Fu et al., 2019). The interaction of microplastics and other pollutants may alter their environmental behavior, toxicity, bioavailability, and may pose threats to the ecosystem and even human beings (Zhang et al., 2018; Yang et al., 2020).
Recently, more and more attention has been paid on the combination of microplastics and coexisting pollutants and their toxicological effects on marine organisms. For instance, some previous studies pointed out that microplastics (e.g., micro-sized polystyrene, polyethylene and polyamide) can strongly adsorb nonylphenol and therefore alleviate its toxicity to Chlorella pyrenoidosa (Yang et al., 2020). Moreover, a low level of mPS (10 μm, 2 μg/L) could decrease the toxicity of phenanthrene on the marine medaka Oryzias melastigma at its early developmental stage (Li Y. et al., 2020). Similarly, polystyrene divinylbenzene microspheres at a size of 97 μm did not magnify the acute effect of pyrene on the tropical fish Lates calcarifer (Guven et al., 2018). However, some contrary results were reported in other studies, for example, it was found that mPS significantly enhanced the bioaccumulation and toxicity of phenanthrene on Daphnia magna (Ma et al., 2016). Meanwhile, synergistic toxicities on microalgae were demonstrated in the combination of microplastics (red fluorescent polymer microspheres), procainamide, and doxycycline mixtures (Prata et al., 2018). Various factors may contribute to the difference, for example, the physical and chemical properties of microplastics, the tolerance of model organisms to pollutants, and the octanol water distribution coefficient and concentration of other pollutants (Zhu et al., 2018; Li et al., 2019; Yi et al., 2019; Yang et al., 2020). Under such circumstances, more systematic studies are required to elucidate the different combined toxicity of microplastics and coexisting pollutants on aquatic organisms.
B[a]P has a strong ability to enter and accumulate in the cells of aquatic organisms and causing various abnormalities and disorders due to its aromatic fused ring and lipophilicity (Derakhshesh et al., 2019). Previous studies showed that B[a]P is capable of influencing cell growth, effective quantum yield, and photosynthesis of phytoplankton (Li et al., 2021). For example, after 72 h of exposure to B[a]P (1–10 μg/L), the growth of Skeletonema costatum decreased significantly. However, Akashiwo sanguinea is more sensitive to B[a]P, and its growth decreased significantly at a quite lower concentration of 0.1 μg/L (Kim et al., 2004). Moreover, the interaction of coexisted B[a]P and microplastics would occur, especially the lipophilic B[a]P has strong affinity to suspended materials and sediments in the aquatic environment (Yu et al., 2018). Therefore, the microplastics in the environment are likely to become perfect carriers of B[a]P, increasing the bioavailability of B[a]P and enrichment in organisms along the food chain (Wang et al., 2021). However, further research on their combined toxicity to aquatic organisms is needed.
Microalgae are widely applied in ecotoxicological analytical tests due to their short generation time, easy operation and sensitivity to pollutants (Prata et al., 2019). Marine diatom Chaetoceros muelleri, as one of the most diverse phytoplanktons, was selected as the model organism to elucidate the single and combined effects of B[a]P, microplastics polystyrene (mPS) and polyethylene terephthalate (mPET). The parameters such as cell number, growth inhibition rate, scanning electron microscope (SEM), superoxide dismutase (SOD) and catalase (CAT) of C. muelleri were measured to clarify the toxic effects of microplastics, B[a]P and their combined effects on microalgae.
Materials and Methods
Preparation of Microplastics
The microplastics (i.e., mPS and mPET) were purchased from Aladdin industrial corporation (Shanghai, China) and No. 8863 Huarun (Changzhou, China). The surface structure of mPS and mPET were observed using scanning electron microscopy (SEM, S3000). The functional groups of mPS and mPET were determined by using a Fourier Transform Infrared Spectrometer (FTIR, TENSOR27), respectively. The size of microplastics was measured by using a laser particle size analyzer (Masterizer 3000, Malvern Instruments Ltd., United Kingdom).
Microalgal Strain and Medium
Microalgae C. muelleri was obtained from the College of Oceanography, Hainan University. The f/2 Guillard medium (Guillard, 1975) was prepared with natural seawater (filtered by 0.45 μm membrane) that was collected from the Xinbu Island in Hainan. The medium and flasks used in this study were sterilized at 121°C using an autoclave for 30 min (LDZM-60L-II, Shanghai Shenan Medical Instrument Factory). The initial microalgal concentration was maintained at approximately 1 × 105 cells/mL. Microalgae were cultivated in an Erlenmeyer flask (250 mL) under cool continuous white fluorescent lights (5,000 lx) with a light/dark cycle of 12 h/12 h. All the cultures were randomly placed in an incubator to achieve equal illumination. The temperature was kept at 25 ± 1°C, and all cultures were shaken every 12 h to prevent the aggregation and sedimentation of cells.
Toxicity Test of Pollutants on Microalgae
The concentration range of B[a]P was based on the pollution in the partial sea area of China, for example, an average concentration of B[a]P was 663 ng/L in Daya Bay (Shenzhen, China) while the total PAHs could reach up to 10, 984 ng/L (Qiu et al., 2004). Therefore, 10 μg/L B[a]P was selected to simulate the baseline of PAHs concentration in the natural environment, and also as the lowest concentration of toxicological tests to C. muelleri in the preliminary experiment of this study. Higher concentrations of 50, 100, and 150 μg/L B[a]P were set to simulate severely polluted areas. The preliminary results showed that B[a]P at concentrations of 10, 50, and 100 μg/L did not significantly inhibit the growth of C. muelleri, while B[a]P at 150 μg/L reached an obvious inhibitory effect. Therefore, the concentration of B[a]P was set at 10 and 150 μg/L while microplastics at 200 mg/L (was determined by our previous study) were used for the following experiment. More details are presented in Table 1.
Completely dissolved B[a]P (Shanghai Aladdin Biochemical Technology Co., Ltd., AR) in dimethyl sulfoxide (DMSO, AR, Xilong Science Co., Ltd.) was used to produce a concentrated stock solution (2 g/L). The solution used for the toxicology test was obtained by adding an appropriate volume of the stocks into filtered seawater (0.45 μm). In the chronic toxicity test, the DMSO content is less than 0.005% and has no inhibition on microalgae (Gong et al., 2017). In addition, our preliminary experiments also demonstrated that the same volume of DMSO has no effect on the cell growth of C. muelleri.
The microplastics solution (200 mg/L) was prepared by weighing 0.03 g and adding them to the medium (150 mL) and then reaching evenly distributed state via ultrasonic treatment.
Toxicity Test of Single Microplastics and B[a]P on Microalgae
Microalgal inoculum was added to the medium supplemented with microplastics (i.e., mPS and mPET) and B[a]P, respectively, and then the cultures were placed in a light incubator for 15 days of cultivation. The experimental design is summarized in Table 1. All cultivation conditions were the same as those in Section “Microalgal Strain and Medium.” All treatments were tested in replicates, and sterile conditions were maintained to avoid contamination in the test.
Combined Effects of Microplastics and B[a]P on Microalgae
The combined effects of mPS or mPET at 200 mg/L and B[a]P at concentrations of 10 and 150 μg/L were investigated (Table 1). All cultivation conditions were maintained the same as those in Section “Microalgal Strain and Medium.”
Analytical Methods
Cell Number Density and Growth Inhibition Ratio
According to the guideline 201 of the Organization for Economic Cooperation and Development (OECD), the IR of C. muelleri was determined by counting cell numbers. The cell number density of C. muelleri was determined every 2 days, at 400× magnification using a hemocytometer under the microscope (OLYMPUS, DP71). Each sample was counted three times to obtain the mean value.
The microalgal growth inhibition ratio was calculated based on cell number density (Equation 1):
where Igi is the growth inhibition rate at the time i; Cci and Cei are the cell number density of the control and the treatment at the time i.
Assessment of Microplastics and B[a]P Interactive Effects on C. muelleri Growth
Abbott’s model is commonly used to evaluate the potential interactions between microalgae and B[a]P combined system, which assumes the independent effects of components in the mixture. The model treats any deviation from this assumption as a symbol of the interaction between the mixture components (Gisi, 1996; Mwamba et al., 2016). Based on the measured individual toxicity of microplastics (TMPs) and B[a]P (TB[a]P), Abbott’s model was used to predict their combined toxicity (Tpre), assuming an independent action of microplastics and B[a]P occurs in the mixture (Equation 2):
where Tpre is the combined toxicity of microplastics and B[a]P predicted using Abbott’s model; TMPs and TB[a]P is the measured individual toxicity of microplastics and B[a]P, respectively.
Then, the ratio of the combined toxicity measured in the experiment (Tobs) and the value predicted by Abbott’s model (Tpre) will be further calculated to check the existence of potential interaction between microplastics and B[a]P. For example, if the ratio of Tobs and Tpre (i.e., Tobs/Tpre) is equal to 1, indicating an additivity effect is achieved between microplastics and B[a]P, while the value of Tobs/Tpre > 1 or <1 indicates a synergistic or antagonistic effect is achieved for their combination, respectively.
Content of Photosynthetic Pigment
The content of photosynthetic pigment such as chlorophyll a, b, and carotenoid was measured by using the method of Song et al. (2020). Briefly, 2 mL of 90% methanol was added in some microalgal cells (2 mL) collected by centrifugation (10 min, 5,000 rpm). Then, the mixture was placed in the refrigerator overnight at 4°C for 24 h. Finally, the supernatant was obtained by centrifugation (10,000 rpm, 10 min) for measuring the absorbance at wavelengths of 470, 652, and 665 nm by using an ultraviolet-visible spectrophotometer (UV1800, Aoxi). The content of chlorophyll a, chlorophyll b and carotenoid was calculated according to the following formula.
where Ca, Cb, and Cc represents the content of chlorophyll a, chlorophyll b and carotenoid, respectively; A665, A652, and A470 indicate the absorbance of C. vulgaris at 470, 652, and 665 nm, respectively.
Antioxidant Enzyme
Antioxidant enzymes such as SOD and CAT of microalgae were determined using test kits (Solarbio Science & Technology Co., Ltd.). The samples were taken on the Days 1, 5, and 15. Briefly, some microalgal cells collected by centrifugation (10 min, 5,000 rpm) were added with extraction solution (∼5 × 106 cells were added with 1 mL extraction solution) for further break cells by ultrasound (200 W, 3 s, 30 times). Finally, the supernatant was obtained by centrifugation (8,000 g, 10 min, 4°C) for measuring the absorbance at wavelength of 560 nm for SOD and 240 nm for CAT. The content of SOD and CAT was indicated as U/104 cells.
Interaction Between Microplastics and C. muelleri
The coexisting microplastics with microalgae in the culture were observed by naked eyes and recorded every day, while the surface structures of microplastics were measured using SEM at Day 15. Briefly, the microplastics were collected by centrifugation (8,000 rpm, 10 min) and resuspended in a phosphate buffer (0.05 M, pH 7.2) (Mao et al., 2018). Then, the microplastics were fixed using 2.5% glutaraldehyde for 24 h and were further observed and measured using SEM. Cell damage was determined by using the Calcein/PI cell viability and cytotoxicity test kit (Beyotime Biotechnology Co., Ltd.).
Statistical Analysis
Excel 2010 and Origin 2018 were used for data analysis and plotting. The data were expressed as average ± standard error in the study. Different treatments were tested significantly through one-way analysis of variance with Duncan comparisons (SPSS 23.0).
Results
Properties of Microplastics
The size distribution of microplastics is shown in Table 2, and the average size of mPET was slightly greater than that of mPS. As shown in Figures 1A,B, mPS floated on the surface of culture, while mPET sank to the bottom. The precipitation of mPET results from a larger density as compared to mPS. Figures 1C,D showed that many microalgal cells adhered to the surface of microplastics and form heterogeneous aggregations. The coexisting microalgal cells may affect the structures and characteristics of microplastics (Endo and Koelmans, 2016). The mPS is a glassy polymer with a weakly polar and weak π electron donor, and concave structure can be observed via SEM (Figure 1E). By comparison, mPET is a crystalline polymer with more amorphous regions, and plenty of micro-pores can be observed on the rough surface (Figure 1F). Moreover, the functional groups of the two microplastics are different, for example, mPS has a stronger intensity of hydroxyl groups (−OH, ∼3,400 cm–1), carbon-carbon double bond (C = C, ∼1,642 cm–1), carbonyl group (C = O, ∼1,710 cm–1) and C-O stretching of phenolic (C-O, ∼1,260 cm–1), but with a weakened intensity of methylene (CH2, ∼2,926 cm–1) as compared with those of mPET (Figure 2).
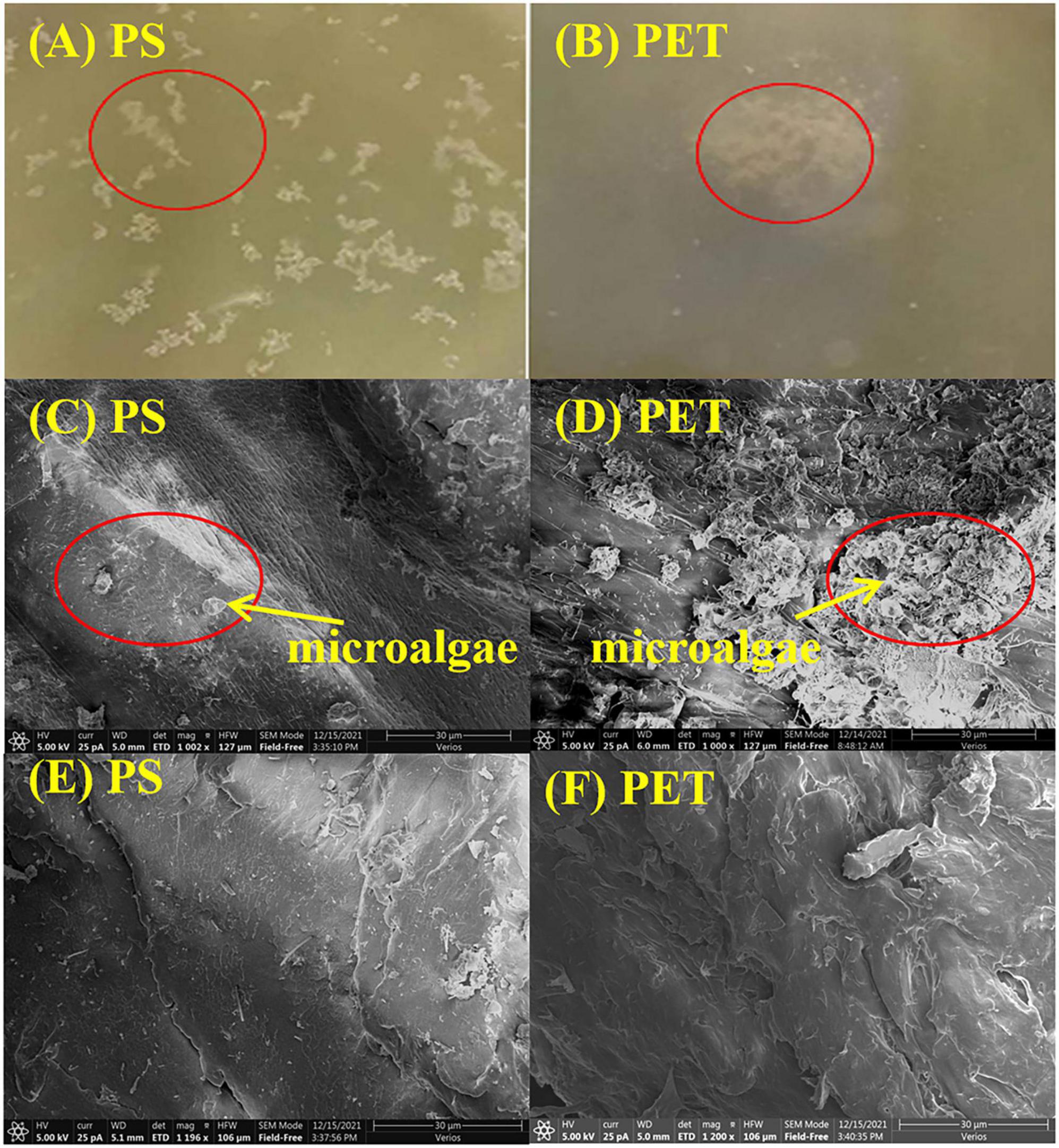
Figure 1. Morphology of (A) mPS and (B) mPET in the culture of Chaetoceros muelleri observed by naked eyes; C. muelleri cells attached to (C) mPS and (D) mPET observed by SEM; SEM images of (E) mPS, and (F) mPET.
Effects of Microplastics and B[a]P on Microalgae
Effects of Microplastics on Cell Growth of C. muelleri
As shown in Figures 3, 4, both microplastics have negative impacts on microalgae, with an increasing IR at the beginning of growth and then decreased in the later stage. The cell density of C. muelleri exposed to mPET (2.42 × 106 cells/mL) is less than mPS (2.55 × 106 cells/mL), and both were lower than the control (2.6 × 106 cells/mL). In addition, no significant difference (P > 0.05) was reached for the cell density of microalgae in the control and the treatment with mPS. However, a significant difference (P < 0.05) was found with mPET as compared with the control.
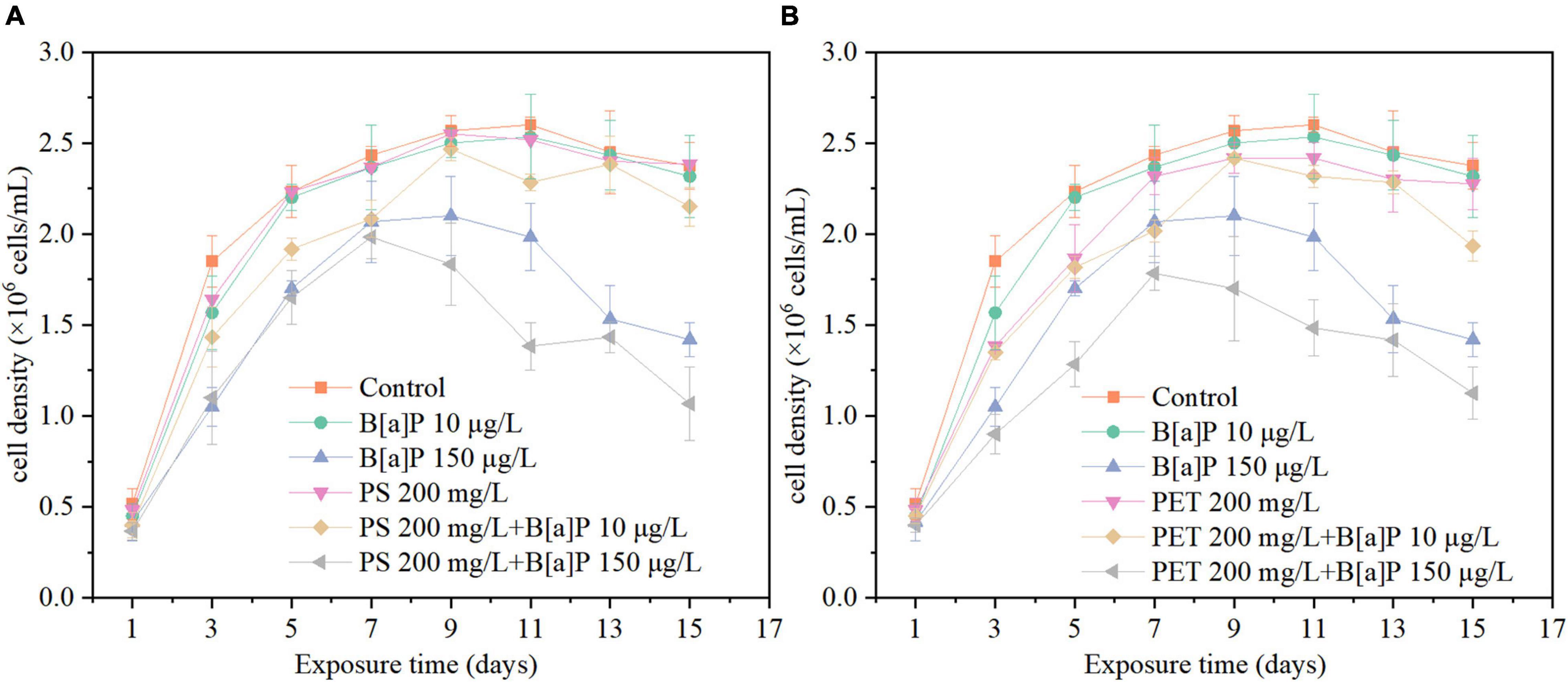
Figure 3. The single and combined effects of microplastics [(A) mPS and (B) mPET] and B[a]P on the cell density of C. muelleri.
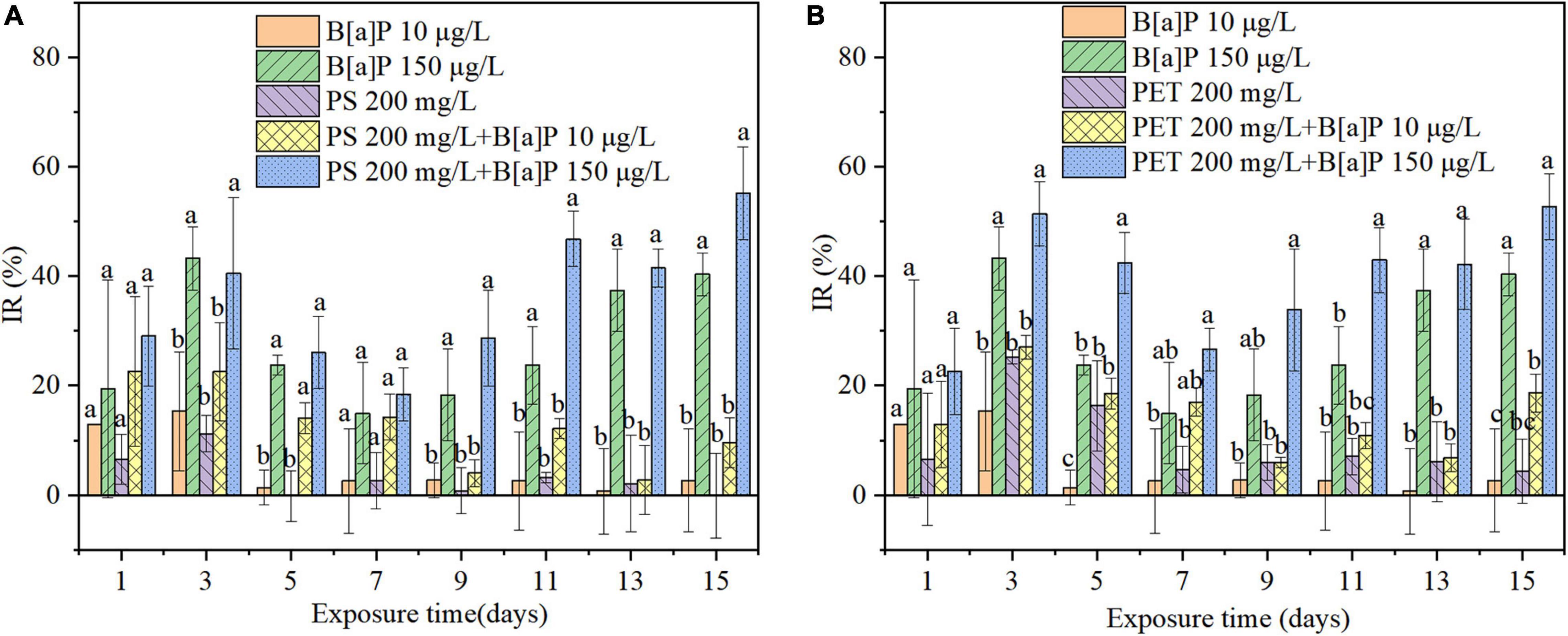
Figure 4. The single and combined effects of microplastics [(A) mPS and (B) mPET] and B[a]P on the IR of C. muelleri. Different letters indicate significant differences among treatments (P < 0.05).
Overall, mPET has stronger inhibition on the growth of microalgae than mPS, for example, the highest IR caused by mPET was 25.23% on the third day, which was significantly (P < 0.05) higher than that of mPS (11.17%) (Figure 4).
Effects of B[a]P on Cell Growth of C. muelleri
As also shown in Figure 3, in the 15 days of cultivation, the cell growth in terms of cell density (Figure 3) of C. muelleri was slightly inhibited by B[a]P at a concentration of 10 μg/L, but significantly inhibited at 150 μg/L (P < 0.05) compared to the control. The maximal cell density obtained in the treatment with B[a]P of 10 and 150 μg/L were 2.53 × 106 and 2.10 × 106 cells/mL (Figure 3), respectively. Correspondingly, the IR increased with the concentration of B[a]P on microalgae under the investigated condition, for instance, the IR caused by 10 and 150 μg/L B[a]P reached their own maximum value of 15.32 and 43.24% on Day 3, respectively (Figure 4).
Combined Effects of Microplastics and B[a]P on Cell Growth of C. muelleri
As shown in Figures 3, 4, the cell density of all combined treatments of microplastics and B[a]P was lower than that of the single treatments. For instance, the highest IR of 55.18% was caused by the combined group of 150 μg/L B[a]P + 200 mg/L mPS, which was higher than that of single 150 μg/L B[a]P (IR = 40.34%) and mPS (IR = 1.26%) after 15-day cultivation, respectively (Figure 4). Similarly, the IR reached up to 52.66% in the combined group of 150 μg/L B[a]P + 200 mg/L mPET, which was also higher than that of single 150 μg/L B[a]P (IR = 40.34%) and mPET (IR = 4.36%) (Figure 4).
Furthermore, the combined toxicities of mPET and B[a]P on microalgae were greater than the combinations of mPS and B[a]P in terms of cell density and IR. It is consistent with the toxicity of microplastics to C. muelleri (Figures 3, 4). Additionally, the combined inhibitory effects on the growth of C. muelleri increased with the concentration and single toxicity of pollutants (e.g., mPET, mPS, and B[a]P). For instance, the maximal cell density (1.98 × 106 cells/mL) achieved in the combined group of 150 μg/L B[a]P + 200 mg/L mPS, was significantly lower than that of combination of 10 μg/L B[a]P + 200 mg/L mPS (2.47 × 106 cells/mL) (P < 0.05). The IR of the combined group of B[a]P and mPS was less than the combined group of B[a]P and mPET.
Photosynthetic Pigment Content of Microalgae
Overall, the content of photosynthetic pigment was decreased when the microalgae exposed to either single or combined microplastics and B[a]P (Figure 5 and Supplementary Figure 1). For single system, the inhibitory effect of high concentration of B[a]P was greater than that of low concentration of B[a]P. In addition, the inhibitory effect of PET was greater than that of PS. This is consistent with the results of growth inhibition. In the combined system, it is strange that the addition of microplastics alleviated the inhibitory effect of B[a]P on photosynthetic pigment of microalgae. For example, the photosynthetic pigment content of the culture exposed to single B[a]P (150 μg/L) was 1.97 mg/L, which was lower than that of cultures exposed to combined pollutants of PS + B[a]P 150 μg/L (2.19 mg/L) and PET + B[a]P 150 μg/L (3.36 mg/L) on Day 15 of cultivation.
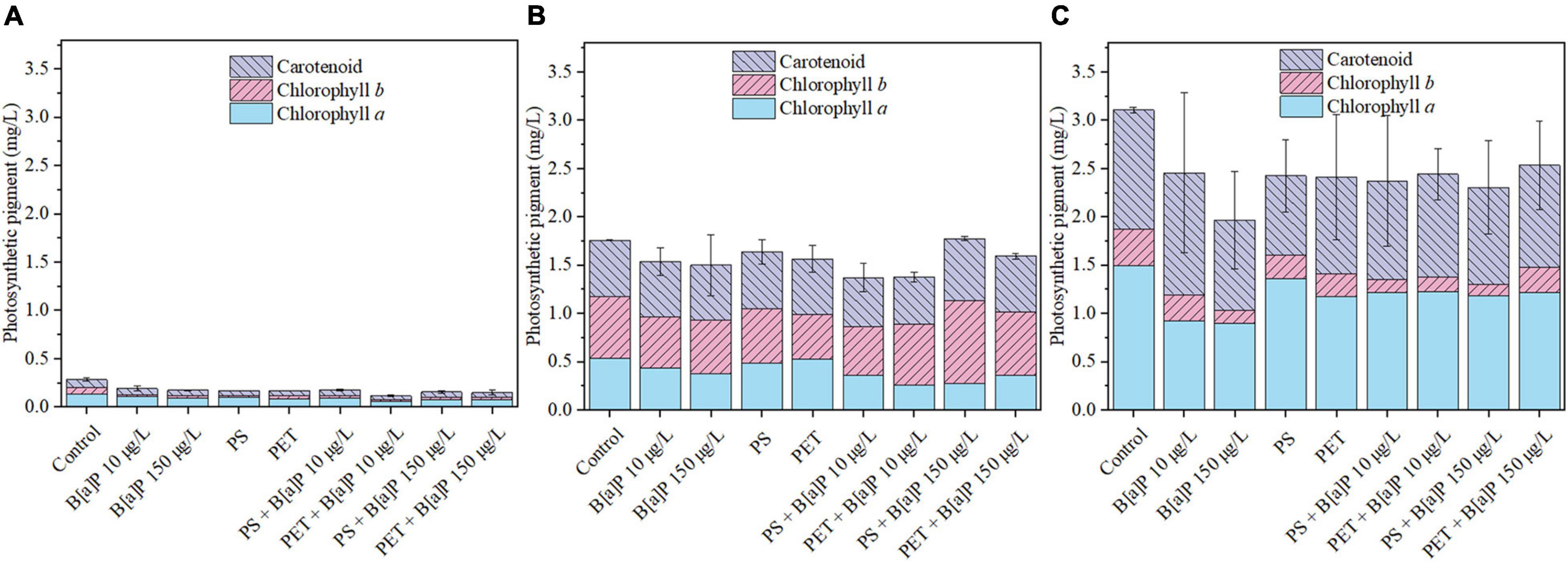
Figure 5. The single and combined effects of microplastics (mPS and mPET) and B[a]P on the photosynthetic pigment of C. muelleri in (A) day 1, (B) day 5, and (C) day 15.
Antioxidant Enzymes of Microalgae
The antioxidative defense system of C. muelleri in terms of SOD and CAT was also determined on the Days 1, 5, and 15 of cultivation in this study (Figure 6). In the early stage of pollutants exposure (Days 1 and 5), the pollutants stimulated the production of SOD and CAT of microalgae compared with the control group. As shown in a single toxicity test, the SOD and CAT content of the culture exposed to B[a]P increased with its concentration. For single microplastics, the SOD content of microalgal culture exposed to mPET was higher than that of mPS, but the CAT content of microalgal culture exposed to mPET was lower than that of mPS. The results may indicate that the type of microplastics was one of the important factors affecting the secretion of SOD and CAT by microalgae. As compared with the control, the SOD contents of the cultures exposed to the four combined treatments were significantly increased (P < 0.05) on Day 5, and the CAT on Day 1 was significantly increased (P < 0.05). Besides, the SOD and CAT content in the combined of microplastics and B[a]P was higher than that of single microplastics or B[a]P. Moreover, it should be mentioned that, for the combined treatment, the production of enzymes such as SOD and CAT was mainly affected by the concentration of B[a]P at the early period of pollutants exposure (Days 1–5), that is, the higher the concentration of B[a]P, the higher the enzyme content can be achieved.
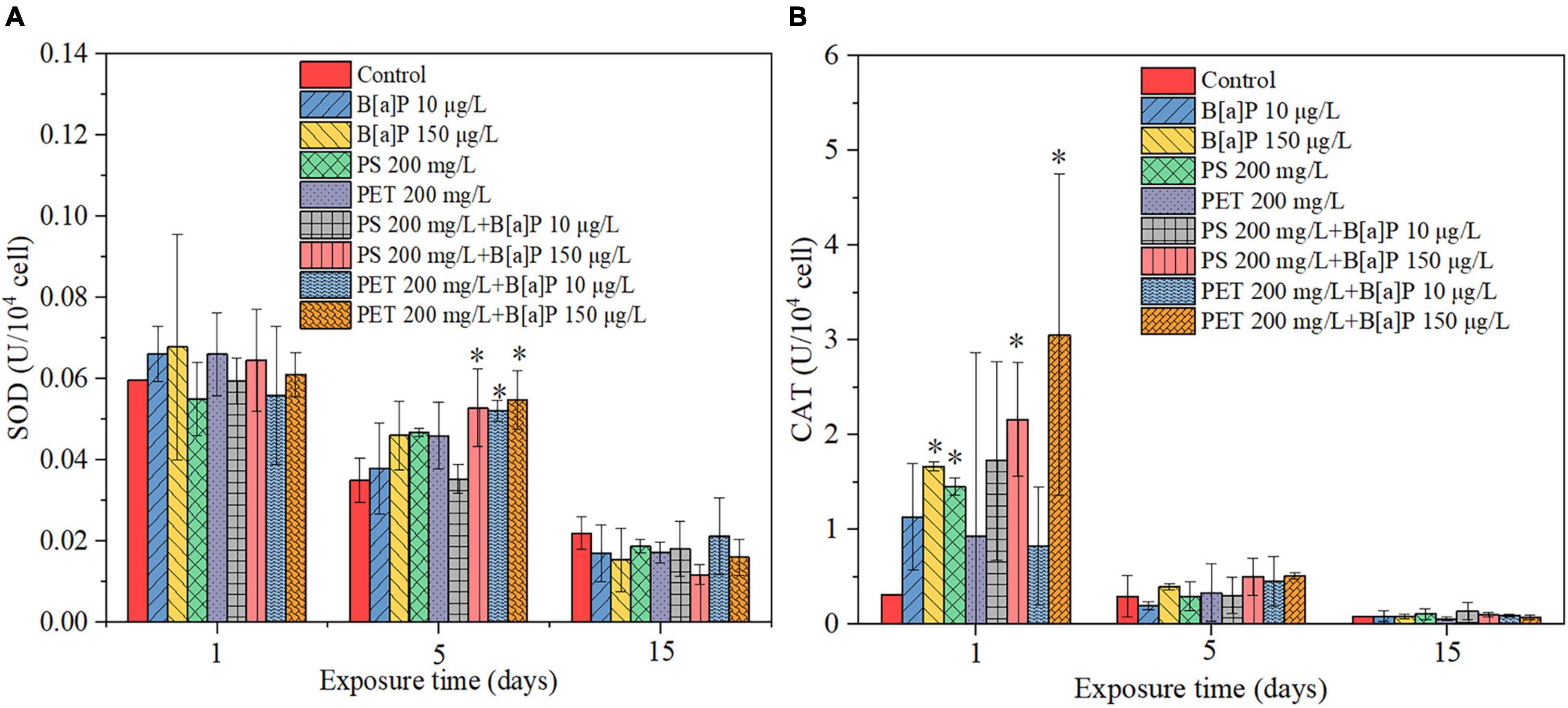
Figure 6. (A) SOD and (B) CAT activity of C. muelleri to single microplastics, B[a]P, and their combinations. * indicates that significant differences compared to the control (P < 0.05).
On the contrary, in the late period of pollutants exposure (Day 15), the pollutants slightly inhibited the production of oxidative response kinase such as SOD and CAT, but no significant difference (P > 0.05) was reached as compared with that of the control.
Discussion
Effects of Microplastics and B[a]P on Cell Growth of Microalgae
Single Microplastics
The growth of C. muelleri exposed to microplastics can be generally divided into two stages. At the first stage (e.g., Days 1–3), the cell density of the cultures supplemented with microplastics decreased with time, corresponding to an increasing IR. This may be attributed to that unfavorable environmental conditions caused by microplastics (Zhang et al., 2017). Meanwhile, it can also result in damaged microalgal cell membrane in terms of some holes and hollows in this study (Figure 7 and Supplementary Figure 2). At the second stage (e.g., Days 4–15), the IR of microalgae decreased since the cells gradually adapted to the existence of microplastics and the unfavorable environment. Therefore, the microalgal biomass and photosynthesis can be restored via thickening cell wall during the later exposure period of microplastics. These can effectively alleviate the cell damage caused by microplastics accordingly (Mao et al., 2018).
Meanwhile, this study found that mPET exhibited stronger inhibitory effect on microalgal growth compared to mPS. The toxicity may be derived from the differences in the physical and chemical properties of microplastics. For example, the mPET has greater surface roughness than that of mPS, and this would result in stronger toxic effects on microalgae. Previous study also reported that the surface roughness of microplastics can inhibit microalgal growth, and a rougher surface would lead to stronger inhibition to microalgae (Wang et al., 2021). Meanwhile, this study also found that mPET was completely immersed in the culture, offering a wider contact area to microalgal cells. This was confirmed by the intensive contact between microplastics with cells that can be observed via the SEM images (Figure 1). This would limit the mass transfer of microalgae with extracellular environment and further aggravate cell damage.
The main inhibition mechanism of microplastics on microalgae is shown in Figure 8. In general, microplastics may be led to damages to the cell wall and membrane structures, lipid peroxidation, toxicities to organelles and even DNA, which is commonly indicated by an increase of SOD enzyme, CAT enzyme, reactive oxygen species (ROS), malondialdehyde (MDA), respectively (Mao et al., 2018; Nava and Leoni, 2021).
Furthermore, it is reported that the presence of microplastics can increase the expression levels of microalgae genes involved in the sugar synthesis pathway, and trigger microalgal cells to secrete extracellular polymeric substances (EPS). Then, it can promote the heterogeneous aggregation between microalgae and microplastics, through establishing hydrogen bonds or electrostatic interactions (Chen et al., 2012; Fu et al., 2019). These aggregates would further limit the light transfer and mass transfer between cells and the environment. Thus, the photosynthesis of microalgae will be restricted in terms of a decreased chlorophyll fluorescence parameter (Fv/Fm) or photosynthesis pigment (Bhattacharya et al., 2010; Li S. et al., 2020; Lang et al., 2022). Additionally, the contaminants adsorbed or the additives released by microplastics can transfer to microalgal cells when they interact or aggregate. They can negatively impact the growth of microalgae particularly when the cell damage occurred (Prata et al., 2018).
Single B[a]P
In this study, B[a]P at low concentration (10 μg/L) did not inhibit the growth of C. muelleri, which may relate to that microalgal cells can adapt to the toxic environment with low concentration. Particularly, the cells in the growth stable period could gradually adapt to the presence of pollutants. For example, Olmos-Espejel et al. (2012) found that B[a]P was metabolized by Selenastrum capricornutum and the concentration of B[a]P in the solution gradually decreased with time. It may be inferred that the potential capacity of microalgae for detoxifying organic pollutants can be enhanced by bioabsorption, bio-uptake and biodegradation (Sutherland and Ralph, 2019). However, once reaching a high concentration of B[a]P, the ability of microalgae for alleviating pollutants (e.g., B[a]P) would be restricted and therefore the growth of microalgae can be inhibited (Wu et al., 2019). For example, B[a]P at high concentration (e.g., 150 μg/L) result in obvious inhibitory effects to C. muelleri in this study, which was consistent with the results of previous study (Shen et al., 2012).
Combined Toxicity of Microplastics and B[a]P
This study found that the presence of microplastics could enhance the toxicity of B[a]P on microalgae as compared with the single toxicity caused by B[a]P. The results of Abbott’s model indicated that the single pollutants (i.e., microplastics, B[a]P) and their combinations induced different toxicities to microalgae (Table 3). Synergistic effect (i.e., exceeding the additive effects of single pollutants that is predicted by Abbott’s model) was observed between microplastics with B[a]P at high concentrations, but antagonistic effect was observed at low exposure level of B[a]P. In general, the degree of combined toxicity is associated with the amount of B[a]P accumulated in microalgae (Mwamba et al., 2016). Correspondingly, microplastics may also drive the toxic effect of B[a]P on microalgae.
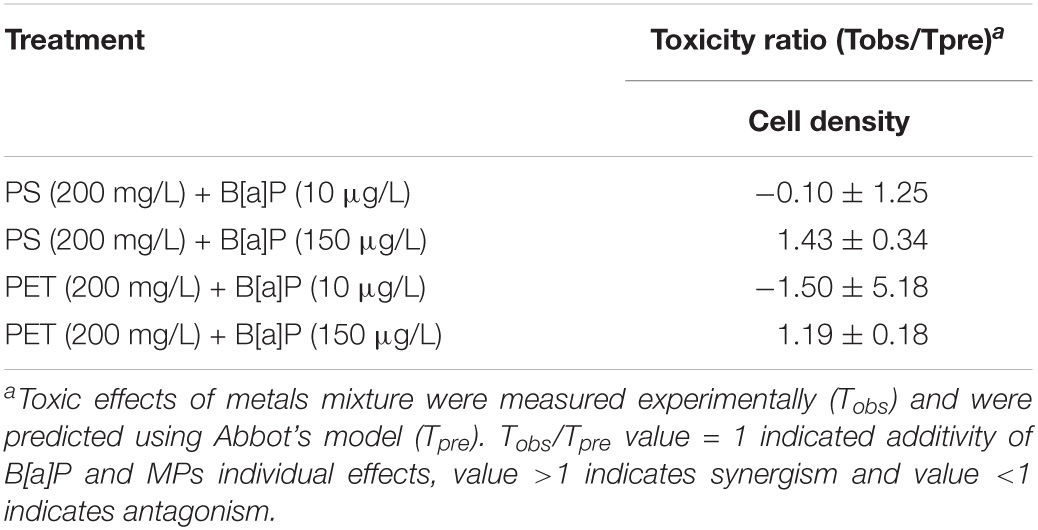
Table 3. Interactive effects of microplastics and B[a]P on cell density in 15 days treated in C. muelleri.
On the one hand, microplastics can provide space for the colonization of microalgae, and meanwhile offer active sites for the adsorption of B[a]P (Gao et al., 2021). Under such circumstances, their coexistence may lead to close contact between microalgae and B[a]P on microplastics, resulting in cell damage to microalgae (Mao et al., 2018). Particularly, the absorption and/or toxicity of B[a]P to cells can be greatly aggravated when the cell structure is destroyed, owing to no protective shield to cells. Similar results were observed in some previous studies (Prata et al., 2018; Yi et al., 2019).
Furthermore, it should be mentioned that some studies reported that the combination of some organic pollutants with microplastics could decrease their combined toxicities on microalgae (Zhu et al., 2018) (Table 4). While the biotoxicity and bioaccumulation may be attributed to the tested species, polymer type, the type and concentration of pollutants (Bellas and Gil, 2020). Take the concentration of pollutants as an example, Li et al. (2019) demonstrated that the combined effect of mPS (0.1 μm) and dibutyl phthalate (DBP, 0.25–16 mg/L) on Chlorella pyrenoidosa varied with their concentration ranges, reaching an antagonistic effect between all the tested concentrations of mPS and DBP at low concentrations (e.g., 0.25 mg/L). However, the combined effects changed with the increasing concentrations of DBP, reaching synergistic effect between DBP and mPS less than 10 mg/L, while antagonistic effect for the combination of DBP with mPS exceeding 10 mg/L.
To further explore the toxicity mechanisms, the functional groups of microplastics were tested and analyzed, it is found that mPS is more aromatic and this would increase its affinity for pollutants (Fu et al., 2019). Other studies also reported that mPS offered a greater adsorption capacity of sulfamethoxazole (12 mg/L) than that of mPET, however, the mechanisms were not specified (Guo et al., 2019). This further explained the fact that mPS exhibited stronger toxicity to cells compared to mPET. Besides, it is interesting that the combined toxicity of mPET and B[a]P on cell growth of microalgae was more serious than that of mPS in this study. This may be attributed to that the weak adsorption capacity of mPET leading to a higher concentration of B[a]P in the environment (Fu et al., 2019), and therefore causing a strong inhibitory effect. It should be mentioned that the elucidation of specific toxic mechanisms requires further studies on other factors such as the crystallinity of microplastics, surface chemical structure and surface charge properties, and the types of pollutants.
Effects of Microplastics and B[a]P on Photosynthetic Pigment
Photosynthetic pigments play an important role in the photosynthesis of microalgae. In addition, the content of chlorophyll a can reflect environmental stress and toxicity (Lang et al., 2022). Therefore, this study determined the content of photosynthetic pigment (chlorophyll a, chlorophyll b and carotenoids) in microalgae. Previous studies have shown that the pollutants can inhibit the synthesis of chlorophyll a. For example, Lang et al. (2022) found that after 96 h of exposure, the inhibition ratio of PS (25–200 mg/L) on the content of chlorophyll a reached 20.11, 22.34, 22.10, and 25.45%, respectively. Triclosan (TCS) (0.7 μg/mL) significantly reduced the chlorophyll content of cyanobacterium (Anabaena sp. PCC7120) (Verdú et al., 2021). The above findings are consistent with this study. The inhibitory effect on the photosynthetic pigment of microalgae may also be the cause of the inhibitory effect on the microalgal growth.
For single microplastics or B[a]P, the content of photosynthetic pigment may be related to the concentration and type of pollutants, in other words, the higher the concentration of pollutants, the greater their inhibition on C. muelleri. Similar results were also reported in previous studies. For instance, Liu et al. (2021) found that triphenyl phosphate (TPhP, 0.08, 0.4, and 0.8 mg/L) have the greatest inhibition to chlorophyll a at high concentration (0.8 mg/L) on marine diatom Phaeodactylum tricornutum. In addition, the inhibition of PET on photosynthetic pigment was greater than PS, which may be because the light transmittance of PET is less than that of PS (Li, 1996), and this may be also weaken the synthesis of photosynthetic pigment. The combined of microplastics and B[a]P had a stronger inhibitory effect on C. muelleri, but their inhibitory effect on photosynthetic pigment was weakened compared with single microplastics or B[a]P. This may be attributed to that when the external pressure on the microalgae reaches a certain critical point, the microalgae cells would trigger a regulatory mode of defense to adapt to adverse conditions by reducing chlorophyll degradation or inhibiting biosynthesis (Agathokleous et al., 2020). Similarly, Chae et al. (2019) found that micro-sized polyethylene spheres had a slight inhibitory effect (no significant effect, P > 0.05) on the chlorophyll content of microalgae at low concentrations (50–150 mg/L), but it significantly promoted (P < 0.05) the chlorophyll content at high concentrations (200–350 mg/L).
Effects of Microplastics and B[a]P on Antioxidant Enzymes
In general, SOD plays the role in antioxidant via transforming the O2– into H2O2 in cells (Mittler, 2002). The stimulatory effect results from short-term exposure to the low dosage of toxic substances (Calabrese et al., 2012), is commonly characterized as hormesis response in terms of increasing SOD activities. For instance, some studies reported that the SOD content of microalgae would increase when they were exposed to microplastics (Yang et al., 2020; Wang et al., 2021). This may be due to the production of ROS stimulated by rough surface structure of microplastics (Song et al., 2020). This study also found that the single or combined pollutants (e.g., mPS, mPET, and B[a]P) actually increased the SOD of microalgae as compared with the control in the first 5 days of cultivation. By comparison, the pollutants inhibited the SOD content on the last day of the experiment. This may be attributed to the stimulating effects to microalgae from the evolution of large amount of ROS under the stressed condition (Bellingeri et al., 2020). Once the accumulation of ROS in microalgal cells reached a super high level, the cellular ability to remove ROS via producing enough oxidative response kinases (i.e., SOD and CAT) would be restricted and even destroyed. Thus, the antioxidative enzymatic system may collapse accompanying with abnormal secretion of antioxidative enzymes. This was also demonstrated by a previous study that some organisms can eliminate excess ROS via increasing the production of SOD to cope with mild stress from copper, but the content of SOD would decrease when the stress is further aggravated (Papadimitriou and Loumbourdis, 2002).
Furthermore, CAT can be used to remove H2O2 from cells when H2O2 is at a high level (Mittler, 2002). Some studies pointed out that the CAT of microalgae can be increased when the cells being exposed to copper and microplastics (Manimaran et al., 2012; Zhang et al., 2021). However, the CAT content of microalgal cultures exposed to either single or combined pollutants increased in the first 5 ays, but decreased on Day 15 of cultivation compared with the control in this study, which was consistent with the results of SOD content. Similar results were observed in the study of Lozano et al. (2014), which the CAT activity in Cylindrotheca closterium was slightly decreased when being exposed to copper, but no details about the mechanisms. Overall, more profound studies are required to further elucidate the role of antioxidative enzymes and their fluctuations with the changes of stressed conditions in the future.
Conclusion
This study investigated the single and combined effects of microplastics (mPS and mPET) and B[a]P on microalgae C. muelleri. The results showed that single mPET or B[a]P at high concentration (i.e., 150 μg/L) have significantly inhibition on cell density of microalgae, but mPS or B[a]P at low concentration (i.e., 10 μg/L) have no significant inhibition to cells. The aggregation of mPET and microalgal cells was observed in the culture, which may be one of contributors to the negative impact on microalgae. The interaction between microplastics and B[a]P is closely related to the concentration and toxicity of single pollutants. The synergistic effect of microplastics and B[a]P at high concentrations might be partially attributed to the ability of microplastics to be vector of B[a]P entering microalgal cells. This work would help to improve the understanding of the combined toxicity of microplastics and organic pollutants such as B[a]P in the marine environment. More studies regarding the interaction mechanisms of microplastics with B[a]P are required to be elucidated in the future.
Data Availability Statement
The original contributions presented in the study are included in the article/Supplementary Material, further inquiries can be directed to the corresponding author.
Author Contributions
YS and HQ provided the methodology, conducted the experiments, and wrote the manuscript. YH, MG, and JL performed the investigation. MC and XZ participated writing—review and editing. MC performed data processing. CG provided resources. DF and ZW provided the methodology. LP provided the resources, administrated the project, supervised the experiments, and participated in the writing original draft. All authors contributed to the article and approved the submitted version.
Funding
This study was supported by the Natural Science Foundation of Hainan Province, China (Grant No. 2019RC043), the National Natural Science Foundation of China (41766003), and Start-up funding from Hainan University [kyqd(zr)1719].
Conflict of Interest
The authors declare that the research was conducted in the absence of any commercial or financial relationships that could be construed as a potential conflict of interest.
Publisher’s Note
All claims expressed in this article are solely those of the authors and do not necessarily represent those of their affiliated organizations, or those of the publisher, the editors and the reviewers. Any product that may be evaluated in this article, or claim that may be made by its manufacturer, is not guaranteed or endorsed by the publisher.
Supplementary Material
The Supplementary Material for this article can be found online at: https://www.frontiersin.org/articles/10.3389/fmars.2021.779321/full#supplementary-material
References
Agathokleous, E., Feng, Z., and Peñuelas, J. (2020). Chlorophyll hormesis: are chlorophylls major components of stress biology in higher plants? Sci. Total. Environ. 726:138637. doi: 10.1016/j.scitotenv.2020.138637
Bellas, J., and Gil, I. (2020). Polyethylene microplastics increase the toxicity of chlorpyrifos to the marine copepod Acartia tonsa. Environ. Pollut 260:114059. doi: 10.1016/j.envpol.2020.114059
Bellingeri, A., Casabianca, S., Capellacci, S., Faleri, C., Faleri, C., Paccagnini, E., et al. (2020). Impact of polystyrene nanoparticles on marine diatom Skeletonema marinoi chain assemblages and consequences on their ecological role in marine ecosystems. Environ. Pollut. 262:114268. doi: 10.1016/j.envpol.2020.114268
Bhattacharya, P., Lin, S., Turner, J. P., and Ke, C. P. (2010). Physical adsorption of charged plastic nanoparticles affects algal photosynthesis. J. Phys. Chem. C 114, 16556–16561. doi: 10.1021/jp1054759
Calabrese, E. J., Iavicoli, I., and Calabrese, V. (2012). Hormesis: why it is important to biogerontologists. Biogerontology 13, 215–235. doi: 10.1007/s10522-012-9374-7
Chae, Y., Kim, D., and An, Y. (2019). Effects of micro-sized polyethylene spheres on the marine microalga Dunaliella salina: focusing on the algal cell to plastic particle size ratio. Aquat. Toxicol. 216:105296. doi: 10.1016/j.aquatox.2019.105296
Chen, P., Powell, B. A., Mortimer, M., and Ke, P. C. (2012). Adaptive interactions between Zinc Oxide nanoparticles and Chlorella sp. Environ. Sci. Technol. 46, 12178–12185. doi: 10.1021/es303303g
Derakhshesh, N., Salamat, N., Movahedinia, A., Hashemitabar, M., and Bayati, V. (2019). Exposure of liver cell culture from the orange-spotted grouper, Epinephelus coioides, to benzo[a]pyrene and light results in oxidative damage as measured by antioxidant enzymes. Chemosphere 226, 534–544. doi: 10.1016/j.chemosphere.2019.03.181
Endo, S., and Koelmans, A. A. (2016). “Sorption of hydrophobic organic compounds to plastics in the marine environment: equilibrium,” in Hazardous Chemicals Associated with Plastics in the Marine Environment. The Handbook of Environmental Chemistry, Vol. 78, eds H. Takada and H. Karapanagioti (Cham: Springer), doi: 10.1007/698_2016_11
EuropePlastics (2019). Plastics–The Facts 2019: An Analysis of European Plastics-Facts-2018. Production, Demand and Waste Data. Belgium: Plastics Europe.
Fu, D., Chen, C. M., Qi, H., Fan, Z., Wang, Z., Peng, L., et al. (2020). Occurrences and distribution of microplastic pollution and the control measures in China. Mar. Pollut. Bull. 153:110963. doi: 10.1016/j.marpolbul.2020.110963
Fu, D., Zhang, Q., Fan, Z., Qi, H., Wang, Z., and Peng, L. (2019). Aged microplastics polyvinyl chloride interact with copper and cause oxidative stress towards microalgae Chlorella vulgaris. Aquat. Toxicol. 216:105319. doi: 10.1016/j.aquatox.2019.105319
Gao, L., Fu, D., Zhao, J., Wu, W., Wang, Z., Su, Y., et al. (2021). Microplastics aged in various environmental media exhibited strong sorption to heavy metals in seawater. Mar. Pollut. Bull. 169:112480. doi: 10.1016/j.marpolbul
Garrido, S., Linares, M., Campillo, J. A., and Albentosa, M. (2019). Effect of microplastics on the toxicity of chlorpyrifos to the microalgae Isochrysis galbana, clone t-ISO. Ecotoxicol. Environ. Saf. 173, 103–109. doi: 10.1016/j.ecoenv.2019.02.020
Gong, W., Zhu, L., Jiang, T., and Han, C. (2017). The occurrence and spatial-temporal distribution of tetrabromobisphenol A in the coastal intertidal zone of Qingdao in China, with a focus on toxicity assessment by biological monitoring. Chemosphere 185, 462–467. doi: 10.1016/j.chemosphere.2017.07.033
Guillard, R. R. L. (1975). “Culture of phytoplankton for feeding marine invertebrates,” in Culture of Marine Invertebrate Animals, eds W. L. Smith and M. H. Chanley (New York, NY: Plenum Press), 26–60. doi: 10.1007/978-1-4615-8714-9_3
Guo, X., Chen, C., and Wang, J. (2019). Sorption of sulfamethoxazole onto six types of microplastics. Chemosphere 228, 300–308. doi: 10.1016/j.chemosphere.2019.04.155
Guven, O., Bach, L., Munk, P., Dinh, K. V., Mariani, P., and Nielsen, T. G. (2018). Microplastic does not magnify the acute effect of PAH pyrene on predatory performance of a tropical fish (Lates calcarifer). Aquat. Toxicol. 198, 287–293. doi: 10.1016/j.aquatox.2018.03.011
Jambeck, J. R., Geyer, R., Wilcox, C., Siegler, T. R., Perryman, M., Andrady, A., et al. (2015). Plastic waste inputs from land into the ocean. Science 347, 768–771. doi: 10.1126/science.1260352
Kim, S., Shin, K., Moon, C. H., Park, D. W., and Chang, M. (2004). Effects of benzo(a)pyrene on growth and photosynthesis of phytoplankton. Korean J. Environ. Biol. 22, 54–62.
Lang, X., Ni, J., and He, Z. (2022). Effects of polystyrene microplastic on the growth and volatile halocarbons release of microalgae Phaeodactylum tricornutum. Mar. Pollut. Bullet. 174:113197. doi: 10.1016/j.marpolbul.2021.113197
Li, F., Jiang, L., Zhang, T., Qiu, J., Lv, D., Su, T., et al. (2021). Combined effects of seawater acidification and benzo(a)pyrene on the physiological performance of the marine bloom-forming diatom Skeletonema costatum. Mar. Environ. Res. 169:105396. doi: 10.1016/j.marenvres.2021.105396
Li, R. (1996). Research on the comprehensive utilization of PS plastics. J. Chengde Teach. Coll. Natl. 1, 136–139. doi: 10.16729/j.cnki.jhnun.1996.s1.063
Li, S., Wang, P., Zhang, C., Zhou, X., Yin, Z., Hu, T., et al. (2020). Influence of polystyrene microplastics on the growth, photosynthetic efficiency and aggregation of freshwater microalgae Chlamydomonas reinhardtii. Sci. Total Environ. 714:136767. doi: 10.1016/j.scitotenv.2020.136767
Li, Y., Wang, J., Yang, G., Lu, L., Zheng, Y., Zhang, Q., et al. (2020). Low level of polystyrene microplastics decreases early developmental toxicity of phenanthrene on marine medaka (Oryzias melastigma). J. Hazard. Mater. 385:121586. doi: 10.1016/j.jhazmat.2019.121586
Li, Z., Yi, X., Zhou, H., Chi, T., and Yang, K. (2019). Combined effect of polystyrene microplastics and dibutyl phthalate on the microalgae Chlorella pyrenoidosa. Environ. Pollut 257:113604. doi: 10.1016/j.envpol.2019.113604
Liu, Q., Tang, X., Zhang, X., Tong, X., Sun, Z., and Zhang, X. (2021). Mechanistic understanding of the toxicity of triphenyl phosphate (TPhP) to the marine diatom Phaeodactylum tricornutum: targeting chloroplast and mitochondrial dysfunction. Environ. Pollut. 295:118670. doi: 10.1016/j.envpol.2021.118670
Lozano, P., Trombini, C., Crespo, E., Blasco, J., and Moreno-Garrido, I. (2014). ROI-scavenging enzyme activities as toxicity biomarkers in three species of marine microalgae exposed to model contaminants (copper, Irgarol and atrazine). Ecotox. Environ. Saf. 104, 294–301. doi: 10.1016/j.ecoenv.2014.03.021
Ma, Y., Huang, A., Cao, S., Sun, F., Wang, L., Guo, H., et al. (2016). Effects of nanoplastics and microplastics on toxicity, bioaccumulation, and environmental fate of phenanthrene in fresh water. Environ. Pollut. 219, 166–173. doi: 10.1016/j.envpol.2016.10.061
Manimaran, K., Karthikeyan, P., Ashokkumar, S., Ashok Prabu, V., and Sampathkumar, P. (2012). Effect of copper on growth and enzyme activities of marine diatom, Odontella mobiliensis. Bull. Environ. Contam. Toxicol. 88, 30–37. doi: 10.1007/s00128-011-0427-4
Mao, Y., Ai, H., Chen, Y., Zhang, Z., Zeng, P., Kang, L., et al. (2018). Phytoplankton response to polystyrene microplastics: perspective from an entire growth period. Chemosphere 208, 59–68. doi: 10.1016/j.chemosphere.2018.05.170
Mittler, R. (2002). Oxidative stress, antioxidants and stress tolerance. Trends Plant. Sci. 7, 405–410. doi: 10.1016/S1360-1385(02)02312-9
Mwamba, T. M., Ali, S., Ali, B., Lwalaba, J. L., Liu, H., Farooq, M. A., et al. (2016). Interactive effects of cadmium and copper on metal accumulation, oxidative stress, and mineral composition in Brassica napus. Int. J. Environ. Sci. Te. 13, 2163–2174. doi: 10.1007/s13762-016-1040-1
Nava, V., and Leoni, B. (2021). A critical review of interactions between microplastics, microalgae and aquatic ecosystem function. Water Res. 188, 116476. doi: 10.1016/j.watres.2020.116476
Olmos-Espejel, J., García de Llasera, M. P., and Velasco-Cruz, M. (2012). Extraction and analysis of polycyclic aromatic hydrocarbons and benzo[a]pyrene metabolites in microalgae cultures by off-line/on-line methodology based on matrix solid-phase dispersion, solid-phase extraction and high-performance liquid chromatography. J. Chromatogr. A 1262, 138–147. doi: 10.1016/j.chroma.2012.09.015
Papadimitriou, E., and Loumbourdis, N. S. (2002). Exposure of the frog Rana ridibunda to Copper:impact on two biomarkers, lipid peroxidation, and gulathione. Bull. Environ. Contam. Toxicol. 69, 885–891. doi: 10.1007/s00128-002-0142-2
Prata, J. C., da Costa, J. P., Lopes, I., Duarte, A. C., and Rocha-Santos, T. (2019). Effects of microplastics on microalgae populations: a critical review. Sci. Total. Environ. 665, 400–405. doi: 10.1016/j.scitotenv.2019.02.132
Prata, J. C., Lavorante, B. R. B. O., Montenegro, B. S. M. M. D. C., and Guilhermino, L. (2018). Influence of microplastics on the toxicity of the pharmaceuticals procainamide and doxycycline on the marine microalgae Tetraselmis chuii. Aquat. Toxicol. 197, 143–152. doi: 10.1016/j.aquatox.2018.02.015
Qiu, Y. W., Zhou, J. L., Maskaoui, K., Hong, H. S., and Wang, Z. D. (2004). Distribution of polycyclic aromatic hydrocarbons in water and sediments from Daya bay and their ecological hazard assessment. J. Tropic. Oceangr. 23, 72–80. doi: 10.1016/S0269-7491(02)00215-4
Shen, C., Li, Y., and Pan, L. (2012). [Effects of benzo[α]pyrene on cell growth and characteristics in marine microalgae] in Chinese. Mar. Environ. Sci. 31, 510–514. doi: 10.3969/j.issn.1007-6336.2012.04.011
Song, C., Liu, Z., Wang, C., Li, S., and Kitamura, Y. (2020). Different interaction performance between microplastics and microalgae: the bio-elimination potential of Chlorella sp. L38 and Phaeodactylum tricornutum MASCC-0025. Sci. Total. Enviro. 723:138146. doi: 10.1016/j.scitotenv.2020.138146
Sutherland, D. L., and Ralph, P. J. (2019). Microalgal bioremediation of emerging contaminants – Opportunities and challenges. Water Res. 164, 114921. doi: 10.1016/j.watres.2019.114921
Verdú, I., González-Pleiter, M., Leganés, F., Rosal, R., and Fernández-Piñas, F. (2021). Microplastics can act as vector of the biocide triclosan exerting damage to freshwater microalgae. Chemosphere 266:129193. doi: 10.1016/j.chemosphere.2020.129193
Wang, Z., Fu, D., Gao, L., Qi, H., Su, Y., and Peng, L. (2021). Aged microplastics decrease the bioavailability of coexisting heavy metals to microalga Chlorella vulgaris. Ecotoxicol. Environ. Saf. 217:112199. doi: 10.1016/j.ecoenv
Wright, S. L., Thompson, R. C., and Galloway, T. S. (2013). The physical impacts of microplastics on marine organisms: a review. Environ. Pollut. 178, 483–492. doi: 10.1016/j.envpol.2013.02.031
Wu, Y., Guo, P., Zhang, X., Zhang, Y., Xie, S., and Deng, J. (2019). Effect of microplastics exposure on the photosynthesis system of freshwater algae. J. Hazard. Mater. 374, 219–227. doi: 10.1016/j.jhazmat.2019.04.039
Yang, W., Gao, X., Wu, Y., Wan, L., and Zhang, W. (2020). The combined toxicity influence of microplastics and nonylphenol on microalgae chlorella pyrenoidosa. Ecotox. Environ. Saf. 195:110484. doi: 10.1016/j.ecoenv.2020.110484
Yi, X., Chi, T., Li, Z., Wang, J., Yu, M., Wu, M., et al. (2019). Combined effect of polystyrene plastics and triphenyltin chloride on the green algae Chlorella pyrenoidosa. Environ. Sci. Pollut. Res 26, 15011–15018. doi: 10.1007/s11356-019-04865-0
Yu, N., Ding, Q., Li, E., Qin, J. G., Chen, L., and Wang, X. (2018). Growth, energy metabolism and transcriptomic responses in Chinese mitten crab (Eriocheir sinensis) to benzo[α]pyrene (BaP) toxicity. Aquat. Toxicol. 203, 150–158. doi: 10.1016/j.aquatox.2018.08.014
Zhang, C., Chen, X., Wang, J., and Tan, L. (2017). Toxic effects of microplastic on marine microalgae Skeletonema costatum: interactions between microplastic and algae. Environ. Pollut. 20, 1282–1288. doi: 10.1016/j.envpol.2016.11.005
Zhang, Q., Qu, Q., Lu, T., Ke, M. J., Zhu, Y. C., Zhang, M., et al. (2018). The combined toxicity effect of nanoplastics and glyphosate on microcystis aeruginosa growth. Environ. Pollut. 243, 1106–1112. doi: 10.1016/j.envpol.2018.09.073
Zhang, W., Sun, S., Du, X., Han, Y., Tang, Y., Zhou, W., et al. (2021). Toxic impacts of microplastics and tetrabromobisphenol A on the motility of marine microalgae and potential mechanisms of action. Gondwana Res. doi: 10.1016/j.gr.2021.08.011
Keywords: microplastics, benzo[a]pyrene, Chaetoceros muelleri, combined toxicity, oxidative stress
Citation: Su Y, Qi H, Hou Y, Gao M, Li J, Cai M, Zhu X, Chen M, Ge C, Fu D, Wang Z and Peng L (2022) Combined Effects of Microplastics and Benzo[a]pyrene on the Marine Diatom Chaetoceros muelleri. Front. Mar. Sci. 8:779321. doi: 10.3389/fmars.2021.779321
Received: 18 September 2021; Accepted: 30 December 2021;
Published: 03 February 2022.
Edited by:
Jian Zhao, Ocean University of China, ChinaReviewed by:
Bin Xia, Yellow Sea Fisheries Research Institute, Chinese Academy of Fishery Sciences (CAFS), ChinaFei-fei Liu, Shandong University, China
Copyright © 2022 Su, Qi, Hou, Gao, Li, Cai, Zhu, Chen, Ge, Fu, Wang and Peng. This is an open-access article distributed under the terms of the Creative Commons Attribution License (CC BY). The use, distribution or reproduction in other forums is permitted, provided the original author(s) and the copyright owner(s) are credited and that the original publication in this journal is cited, in accordance with accepted academic practice. No use, distribution or reproduction is permitted which does not comply with these terms.
*Correspondence: Licheng Peng, bGNwZW5nQGhhaW5hbnUuZWR1LmNu
†These authors have contributed equally to this work and share first authorship